- 1Clinic of Orthopedics and Trauma Surgery, Heidelberg University Hospital, Heidelberg, Germany
- 2Institute of Structural Mechanics and Dynamics in Aerospace Engineering, University of Stuttgart, Stuttgart, Germany
Achilles tendon lengthening (ATL) is frequently used in the treatment of foot deformities. However, there is currently no objective method to determine the optimal muscle length during surgery. We developed an intraoperative approach to evaluate the passive and active forces of the triceps surae muscle group before and after ATL and aimed to test the following hypotheses: 1) the ankle passive range of motion (ROM) increases, 2) passive muscle forces decrease post-ATL, and 3) forces measured from patients with non-neurological and neurological conditions demonstrate different characteristics. Passive forces at various ankle joint positions were measured in ten patients (11.3 ± 3.0 years old) pre- and post-ATL using a force transducer attached to the Achilles tendon. In six patients, active isometric forces were measured by stimulating the triceps surae supramaximally. Passive forces decreased by 94.3% (p < 0.0001), and ROM increased by 89.4% (p < 0.0001) post-ATL. The pre-ATL passive forces were 70.8% ± 15.1% lower in patients with idiopathic foot deformities than in patients with neurological conditions (p < 0.001). The peak active force of 209.8 ± 114.3 N was achieved at an ankle angle of 38.3° ± 16.0°, where the passive force was 6.3 ± 6.7 N. The inter-individual variability was substantial in both groups. In conclusion, the hypotheses posed were supported. The present findings suggest that muscle passive and active force production as well as the inter-individual variability should be considered when planning further treatment.
Introduction
Skeletal muscles need continuous stretching impulses to grow and develop properly (Huijing and Jaspers, 2005). If this growth stimulus is disrupted by involuntary and prolonged muscle contractions, permanent muscle shortening can occur, resulting in bone and joint deformities. One cause of contraction formation is spasticity, often observed in patients with different underlying neurological conditions such as cerebral palsy (CP). These velocity-dependent repetitive muscle contractions can lead to limitations in force exertion and joint range of motion (ROM) (Farmer and James, 2001; Wren et al., 2005). In the lower limb, triceps surae muscle spasticity resulting in foot deformities such as pes equinus or pes equinovarus are common manifestations (Horsch et al., 2019; Bloom and Sabharwal, 2022) with possible deterioration of the patients’ ability to stand or walk independently (Prabhu et al., 2013; Attias et al., 2016). Muscle shortening and associated joint movement problems are not limited to patients with spastic CP and neurological conditions. Foot deformities due to permanent contractures can occur without neurological origin (Engström and Tedroff, 2018). Pes equinus appears in toe-walking children and the majority of patients with congenital talipes equinovarus (CTEV) are considered idiopathic (Ruzbarsky et al., 2016). Both idiopathic and neurological forms may require surgical intervention but the approach and clinical outcome differ (Gurnett et al., 2008; Brierty et al., 2021). The exact definition of a functionally relevant and impairing equinus foot is still controversial (Horsch et al., 2019; Horsch et al., 2022).
Achilles tendon lengthening (ATL) is a frequently used component in the treatment of pes equinus and recurrent CTEV (Yngve and Chambers, 1996; Rutz et al., 2020). This surgical procedure aims to increase the ROM and improve the patient’s ability to stand and walk by enabling the patient to roll over their feet from heel to toe (Sutherland and Cooper, 1978; Dietz et al., 2006). There are several methods to lengthen the Achilles tendon and the choice depends on the amount of lengthening required, clinical examination, and underlying disease (Dietz et al., 2006; Rutz et al., 2020).
Currently, there is no objective method to quantify the amount of tendon lengthening required to reduce tension without critical muscle weakening. Instead, surgeons rely on their haptic impressions and intuition to determine “the dose of lengthening”. Thus, the risk of over- or under-correction is not negligible (Borton et al., 2001; Dietz et al., 2006; Firth et al., 2013). A few preoperative methods exist to quantify the needed amount of lengthening (Pilloni et al., 2019; Ozyalvac et al., 2020). Yet, these indirect methods are limited in terms of estimating the outcome. Therefore, there is a need for a direct approach that would give surgeons immediate objective feedback during surgery. In this study, we aimed to investigate the use of an intraoperative approach (e.g., Ateş et al., 2013; Ates et al., 2014, 2016; Yucesoy et al., 2017; Ates et al., 2018; Kaya et al., 2018) to measure the passive and active isometric forces of the triceps surae muscle group in relation to different ankle joint positions as well as quantify the effects of Achilles tendon lengthening (ATL) surgery. We hypothesized that i) the maximum dorsiflexion (DF) angle and passive ankle ROM increase, ii) passive muscle forces decrease significantly post-ATL, and iii) muscle forces measured from patients with the neurological disease are different from patients with idiopathic foot deformities.
Materials and methods
Patients
Ten patients (11.3 ± 3.0 years old, average body weight = 51.2 ± 22.2 kg at the time of surgery) who underwent surgery for their foot deformity after indication in our hospital’s pediatric orthopedic outpatient clinic were included. Five of the patients had an idiopathic foot deformity without a diagnosed neurological disease (here referred to as “non-neurologic”), five had a diagnosed underlying disease (four cerebral palsy, one HMSN Type IV = hereditary motor and sensory neuropathy, type IV = Refsum disease). Before the experiments, the patients and/or their parents or legal guardians provided written informed consent. The anthropometric data were collected (Table 1).
Intraoperative procedures
The experimental procedures were approved by the local ethics committee. All procedures were performed in agreement with the guidelines of the Helsinki declaration. The patients received an Achilles tendon lengthening (ATL) performed as a Z-plasty (Yngve and Chambers, 1996) under general anesthesia. The Achilles tendon was made visible with a 6–7 cm posteromedial incision. The tendon was exposed from the calcaneus (insertion) to the musculotendinous junction. Before any other surgical intervention was performed, a sterilized s-shaped buckle force transducer (BFT) (dimensions: width = 12 mm, length = 20 mm, and height = 9 mm; maximal force range = 500 N; for test range 0–200 N: accuracy <3% (<0.2% below 100 N); resolution = 0.6 N and high linearity (R2 = 0.99, peak non-linearity = 1.3%)) was mounted onto the tendon and secured (Figure 1). Prior to use, BFTs were calibrated using bovine tendon strips and sterilized. The BFT was then connected to an amplifier (NI 9237, National Instruments, USA) and a data acquisition system (NI cDAQ-9174, National Instruments, USA).
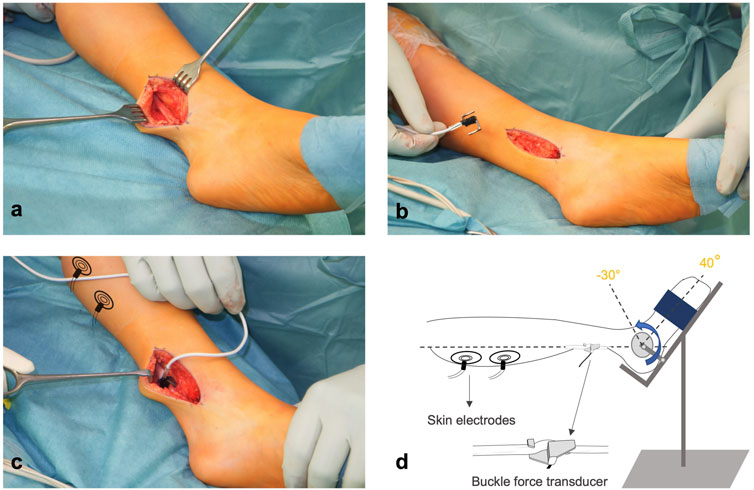
FIGURE 1. Intraoperative experimental setup. (A) Achilles Tendon is exposed under general anesthesia and (B,C) the s-shaped buckle force transducer is attached. (D) Two electrodes are placed on the skin over the triceps surae muscle for active force measurement. The foot is mounted on an apparatus with a heavy ground plate and an ankle angle adjuster. The foot is fixed in the apparatus to collect isometric force data.
For active muscle force measurements, two sterilized skin electrodes (standard ECG electrodes) were placed on the gastrocnemii muscle bellies and connected to a constant current high voltage source (cccVBioS, TEKNOFIL, Istanbul, Turkey). The patient’s foot was mounted on the device (Figure 1D) designed to fix the foot at the adjusted ankle positions. This device included a heavy ground plate to prevent movement during active force measurements. An attached goniometer allowed a precise angle adjustment. The center of foot rotation and the apparatus were carefully aligned.
Collection of passive and active forces
Preconditioning: The triceps surae muscle group was preconditioned by activating with a supramaximal transcutaneous electrical stimulation (with a bipolar rectangular signal, 200 mA, 50 Hz) at the longest and the shortest possible muscle lengths -corresponding to the patient’s maximal plantarflexion (PF) and dorsiflexion (DF) respectively-repeatedly until the measured active forces did not change at the measured position.
Pre-ATL: Triceps surae was activated with two short pulses followed by a pulse train for 1,000 ms to induce tetanic contraction and a subsequent twitch. BFT recorded passive and active isometric forces from the Achilles tendon at fixed positions of ankle angle from the patient’s maximal PF to DF in steps of 10°. Figure 2 shows the superimposed examples of force-time traces collected from one patient’s triceps surae muscle group at different ankle angles. After each contraction, the muscle was given a 2-min break to recover.
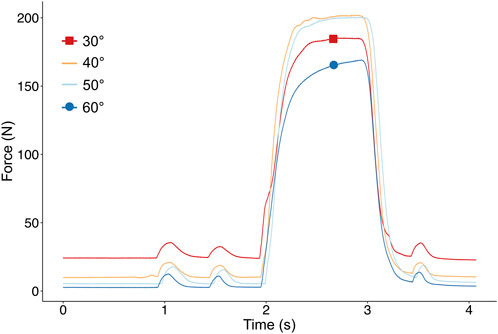
FIGURE 2. Example of superimposed force-time traces collected from the Achilles Tendon of one patient at different ankle angles. After two pulsatile stimulations, the muscle is stimulated supramaximally to reach a tetanic plateau and the maximal muscle force.
After pre-ATL measurements were completed, the BFT was removed and the surgeon performed the Z-plasty: The tendon was divided by a vertical incision using a scalpel. After reaching the desired length, the scalpel was turned by 90° to cut through half of the tendon. The remaining half was cut on the opposite end of the vertical section to create a Z-shaped incision. After applying the correction, the two free ends of the tendon were then sutured with a modified Bunnell technique (Bunnell, 1922) using an absorbable, braided suture (Ethicon, Vicryl 1, Polyglactin 910, Johnson and Johnson, New Brunswick, New Jersey, USA) while the patient’s foot was held in a plantigrade position (0°).
Post-ATL: After completing the Z-plasty, the BFT was mounted onto the tendon again. The passive forces in this new condition were measured throughout the full ROM. The still fragile sutures made post-ATL active measurements impossible.
Data processing and statistics
Passive force (Fp) was averaged over a period of 1,000 ms and fitted in relation to the ankle angle (AA) with an exponential function using a least squares criterion:
a0, a1, and a2 are the coefficients determined during the fitting process. A linear function was used if the exponential function did not describe the collected passive force-ankle angle data.
Active force (FA) -calculated by subtracting passive muscle force (FP) from total muscle force-was averaged over a 400 ms period within the tetanic plateau and fitted with a polynomial function using a least squares criterion:
a0, a1, a2, and an are the coefficients determined in the fitting process, AA = ankle angle. The degree of the polynomial function was chosen based on the best-fit criteria so that the fitted function adequately described the particular set of force-angle data. The fitted force data were used to determine the mean passive and total muscle forces for each ankle angle as well as the peak muscle force and its corresponding angle.
To evaluate force production capacity independent from muscle length, passive and active forces were normalized to the lower leg length of individual patients.
Student’s t-test was used to compare the maximum DF and ROM before and after the ATL. Two-way ANOVA for repeated measures (factors: pre-vs post-ATL; ankle angle) was applied to compare 1) pre- and post-ATL passive forces and 2) the passive muscle forces between neurological and non-neurological patients. Post-hoc tests for pairwise comparisons were applied to locate the differences. Differences were considered significant at p < 0.05.
Results
The patients’ ROM pre- and post-ATL are summarized in Table 2. The post-ATL ROM (62.5° ± 9.6°) was 89.4% higher than the pre-ATL ROM (33.0° ± 9.0°) (p < 0.0001). The increase in ROM was due to the change in maximum DF, as maximum PF remained unaffected. The mean maximum DF increased by 27.1% from 19° ± 13.0° pre-ATL to −10.5° ± 5.2° post-ATL (p < 0.0001) (Figure 3).
Passive forces showed an exponential increase with increasing muscle length. Pre- and post-ATL passive forces were averaged for all ten patients (Figure 4A). ANOVA showed significant effects for both factors (pre- and post-ATL forces (p < 0.0001) and ankle angle (p < 0.001)) with no significant interactions. Post-ATL passive force drop ranged from 90.4% to 95.6% at the measured joint positions; on average 94.3% ± 2.0%, indicating an absolute force reduction of 42.5 N (95% conf. int. = −56.2 to −28.7 N). For normalized passive forces, ANOVA showed significant effects of both factors (pre- and post-ATL forces (p < 0.0001) and ankle angle (p < 0.001)) with no significant interactions.
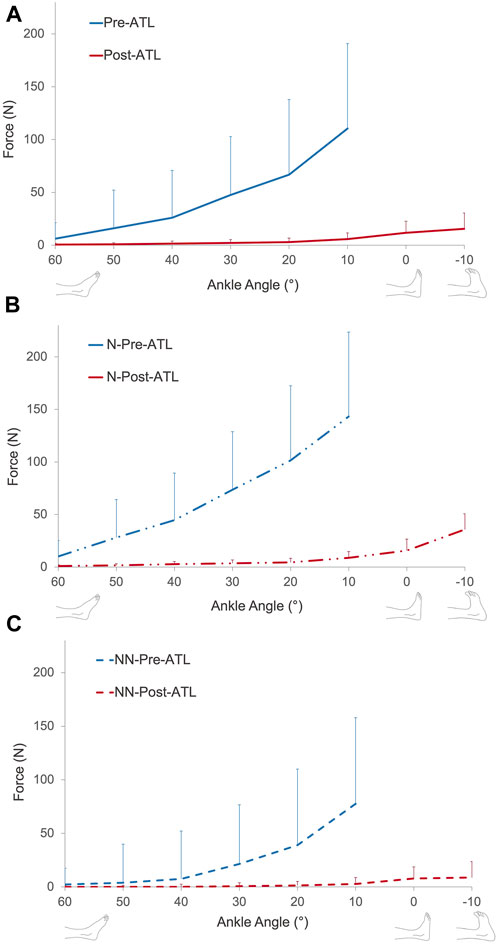
FIGURE 4. (A) Muscle passive force-ankle angle characteristics of all patients pre- and post-ATL. Muscle passive force-ankle angle characteristics of patients with (B) and without (C) neurological conditions pre- and post-ATL. N = neurologic patient, NN = non-neurological condition.
When patients were classified according to their neurological condition (Figures 4B, C), the pre-ATL passive forces were 45.8%–86.1% (at different joint positions) lower for patients with a non-neurological condition compared to the patients with neurological disease (p = 0.0016). The mean difference of −70.8% ± 15.1% corresponded to an absolute value of −43.0 N (95% conf. int. = −17.2 N to −68.0 N). The difference between the two groups (−77.2% ± 18.5%) remained after the ATL (p < 0.01). However, the absolute difference (−3.5 N, 95% conf. int. −1.1 N to −5.9 N) was less pronounced. Normalization did not cause a major change in comparison: The normalized passive forces pre-ATL were 52.3%–84.4% (at different joint positions) lower for patients with a non-neurological condition compared to the patients with neurological disease (Figure 5, p = 0.0013). Post-ATL, the difference in normalized forces remained (−82.5% ± 17.1%) between the two groups (p < 0.01).
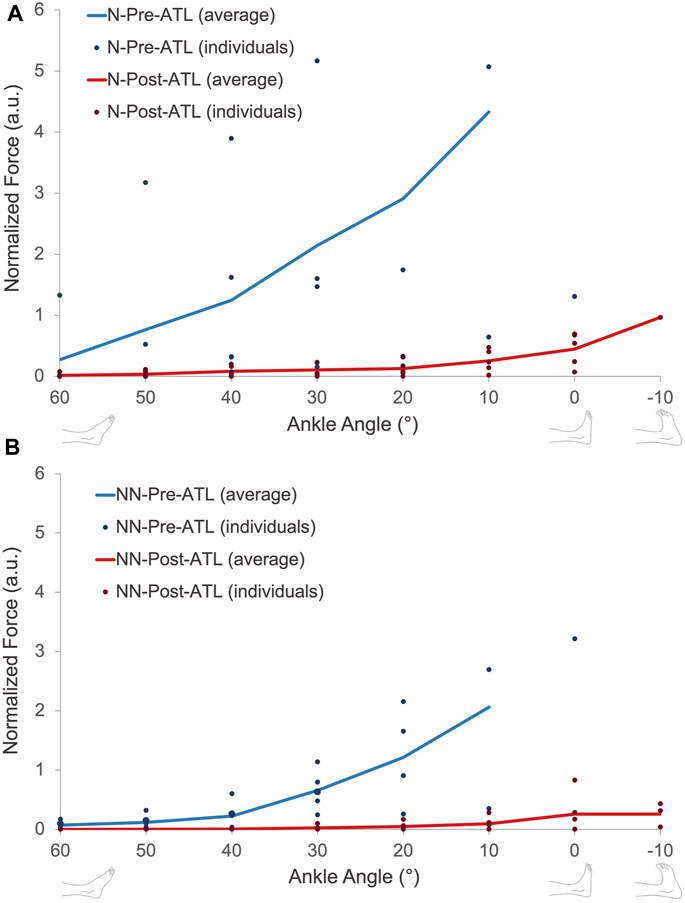
FIGURE 5. Normalized passive force-ankle angle characteristics of patients with (A) and without (B) neurological conditions pre- and post-ATL. N = neurologic patient, NN = non-neurological condition.
We were able to measure the active force production of the triceps surae muscle group for six of the patients (Figure 6A). The peak value of active force production was 209.8 ± 114.3 N, ranges between 58.3 N and 404.5 N. Maximal active force production was achieved at 25°, 60°, 45°, 15°, 40°, and 45° PF angles (38.3° ± 16.0°) and the corresponding passive forces were 7.2 N, 0.7 N, 5.6 N, 19.0 N, 0.8 N, and 4 N (6.3 ± 6.7 N). Normalization of active forces pre-ATL did not cause any major change in the force production characteristics of patients tested (Figure 6B).
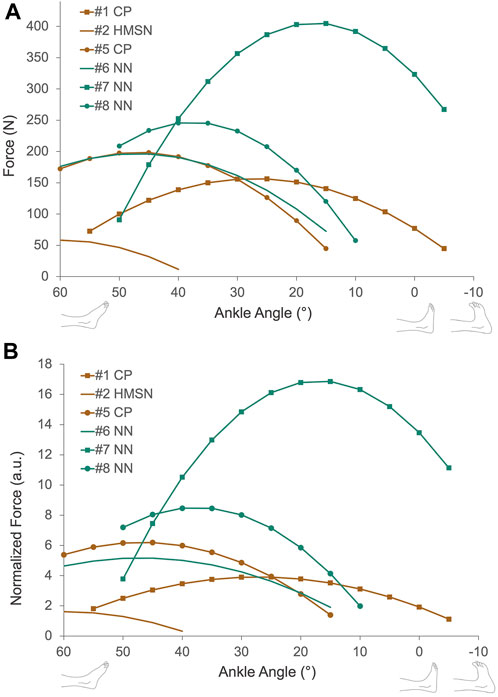
FIGURE 6. (A) Muscle active force-ankle-angle characteristics collected from six patients pre-ATL. Active force is calculated by subtracting passive force from the total measured force. (B) Normalized active force-ankle-angle characteristics pre-ATL. Active forces were normalized to the patient’s lower leg length. CP = Cerebral palsy, HMSN = hereditary motor and sensory neuropathy, NN = non-neurological condition.
Discussion
The main objective of the ATL procedure is to increase the ankle ROM and the length range of muscle force exertion. The surgery aims to attain at least a plantigrade foot position (0°) to improve the function (Dietz et al., 2006). Our present results show that these were achieved for all patients tested. Muscle-lengthening interventions were also expected to reduce passive and active muscle forces (Ates et al., 2013). Consistent with the expectation in passive state, we found a significant decrease in passive muscle forces. Therefore, the hypotheses posed were supported. The observed differences between the muscles of patients with neurological and non-neurological conditions and the possible alterations in active force production are discussed in the following sections.
Passive muscle forces
Pre- and post-ATL passive forces showed a linear to exponential increase from DF to PF joint positions, corresponding to the increasing lengths of the triceps surae muscle group. The passive mechanical characteristics are consistent with previous findings in animal (Gordon et al., 1966; Ter Keurs et al., 1978) and human muscles (Rassier et al., 1999; Kreulen and Smeulders, 2008; de Bruin et al., 2014). After the ATL, the targeted reduction in passive muscle forces was achieved in both groups and this facilitated medium passive tension to shift approximately 30° toward the DF position.
Importantly, even though the present findings were statistically meaningful, the observed inter-subject variability was remarkable pre-ATL. Previous studies investigating exclusively patients with CP have also reported great variability (Smeulders et al., 2004; Ates et al., 2014; Yucesoy et al., 2017). The severity of the disease, differences in treatment approaches, and difficulties in the fine description of the severity may be some of the reasons. Differences in etiologies, particularly in patients with non-neurological conditions, may also explain the variability in part: Idiopathic foot deformities were classified as “non-neurological”, but the exact pathophysiology of the contractures remains unknown and may vary (Gurnett et al., 2008). In patients with CP, muscular changes such as a decrease in muscle volume and length (Barber et al., 2012), and an increase in connective tissue (Booth et al., 2001; de Bruin et al., 2014) have been reported as a result of disruption in the neural signal and abnormality in motor control (Ates et al., 2020). We found that inter-individual variance persisted even after normalization of the forces to the muscle length (Figure 5). Presently, there was no muscle volume (or thickness and physiological cross-sectional area) information collected for the patients recruited, so it is not possible to entirely rule out the effect of muscle volume in variance between individuals. However, muscle volume is expected to determine the force amplitude since it is associated with the amount of active force production but not necessarily the ankle angle range where forces are generated. Thus, the normalization of muscle volume may not cause dramatic shape changes in the mechanical characteristics which highlight possible differential changes in the intrinsic muscle properties rather than muscle size as adaptation factors of muscle passive force-ankle angle characteristics. Sarcomeric protein titin has a key role in myofilament integrity (Swist et al., 2020) and passive resistance where its two regions, corresponding to the I-band of a sarcomere, Ig domains, and PEVK were reported to show different mechanical properties; of the former being stiffer in moderate sarcomere stretches and latter being stiffer at higher stretches (Linke and Granzier, 1998). The amount and material properties of sarcomeric titin may alter in various conditions (e.g., van der Pijl et al., 2020). An earlier study reported that titin mass measured for gastrocnemius and soleus muscles was greater for the patients with CP compared to the typically developing children. (Mathewson et al., 2014). However, the authors could not find a correlation between titin size and muscle fiber passive stiffness. Importantly, this previous study also emphasized the heterogeneity of the data collected from the patients with CP being much higher. Together with these, our findings suggest that the mechanical adaptation of myofibers is not well-understood and needs more detailed investigations. It should also be noted that although titin is not a contractile element, it might as well affect the active muscle characteristics since titin is known to signal the active force production hence, regulating the muscle force exertion depending on sarcomere length (Linke et al., 2002; Rode et al., 2009; Herzog et al., 2012). Yet, the need for relating structural changes of sarcomeric proteins and intramuscular connective tissues with the force production and the resultant mechanical effects of tendon surgery is valid. There is also a data scarcity for patients with idiopathic foot deformities. The factors other than neurological conditions in the development of muscle shortening (Kruse et al., 2021) need to be further investigated using intraoperative designs and histological studies.
Active force measurements
The active force-ankle angle characteristics shown presently (Figure 6) are consistent with the findings of previous studies in animal and human muscles (Gordon et al., 1966; Dulhunty and Franzini-Armstrong, 1977; Rassier et al., 1999; Smeulders et al., 2004; Kreulen and Smeulders, 2008; Ateş et al., 2013). In contrast to the major difference in passive forces found between neurological and non-neurological patients, five patients with non-neurological conditions and CP showed a similar shape of the active force-angle curve, with maximal force production at their medium muscle lengths (15°–45° ankle angle) and spanning both ascending and descending limbs of the curve. The only distinct characteristic was observed for the force excursion curve of the HMSN-patient performing at the descending limb only (with a maximum force at 60° PF). No published data is showing active mechanical characteristics of human lower leg muscles in health however, previous studies reporting the force-knee angle characteristics of hamstring muscles of patients with CP indicated a qualitative similarity to the shape of the active force curve of healthy hamstrings (Ateş et al., 2013; Ates et al., 2016; Kaya et al., 2019). In agreement with these, our present results indicate a comparably wide range of active muscle force production for patients with CP pre-ATL. As maximal force production was achieved around the optimal muscle lengths where the medium passive force was generated, a good overlap between muscle filaments (Gordon et al., 1966) is anticipated pre-ATL. This might mean that the affected muscle per se does not necessarily need to be lengthened for a wide range of active force production. The key to improved joint movement would be a shift of optimal muscle length to DF positions as observed post-ATL but with a minimal loss in active force production (Delp and Zajac, 1992). Previous studies reported major active force reductions (Ates et al., 2013) due to aponeurotomy and muscle lengthening surgeries since these interventions directly interfere with the force-transmitting components of muscles by cutting the connection of muscle fibers to the tendon through neighboring muscle fibers and aponeurosis (Yucesoy et al., 2013). Therefore, the surgery targeting the tendon for lengthening rather than the muscle itself is supported to be a better solution for the targeted patient group.
The mechanical changes induced by the ATL have multiple effects on patients’ gait. First, increased maximal dorsiflexion in the stance and swing phases leads to improved ankle kinematics (Hemo et al., 2006; Ma et al., 2021). This effect can be attributed to the reduction in tendon tension and restored dorsiflexion. In addition to the changes in kinematics, there are evident improvements in kinetics (ankle moment and power) (McMulkin et al., 2016; Putz et al., 2018) which could be explained by the ability of related muscles to generate force over a greater ROM post-ATL due to increased tendon length. Hence, the increase in dorsiflexion and a wider range of force generation could lead to better walking ability reported after ATL procedures (Hemo et al., 2006; McMulkin et al., 2016; Putz et al., 2018; Ma et al., 2021).
Feasibility and clinical relevance of intraoperative measurements
In the tendon lengthening surgery, as presented, objective force data can help to determine the best position for the refixation of the tendons with the right tension to minimize the loss in active force production (Lieber et al., 1996). The implementation of this new technique in surgery routines can initially be challenging. However, once employed, it is a straightforward method that provides surgeons with immediate objective information. Passive data can be collected within 5 minutes. Active force data collection, however, can take about 20 min as the muscle needs recovery periods between consecutive measurements. Though, the active force data is still relevant since with the present knowledge, the passive force cannot be used directly as a predictor of the characteristics and magnitude of active force production. For this to be feasible, there is a need for more data to be collected and reliable muscle models that are validated for specific conditions and diseases.
Both passive and active force production showed high inter-subject variability, even within the same group of patients. Until further research and results on the etiology and pathophysiology of muscle shortening are available to allow a more precise classification, force data should be collected for each patient individually. Particularly for patients with idiopathic foot deformities, this study provides important results on force production and tendon tension that can be further built upon.
More importantly, the presented method is not necessarily limited to the measurement of triceps surae forces. A similar approach can be used, for example, to measure tibialis anterior and tibialis posterior muscle forces during tendon transfers. Therefore, in tendon transfer and muscle lengthening surgery, the surgeon’s intuition and experience can be supplemented with objective force data. Consequently, our findings suggest that the method improved in this study 1) can be used to determine the new optimal muscle length and 2) would provide comparability and thus improve surgical outcomes.
Data availability statement
The raw data supporting the conclusion of this article will be made available by the authors, without undue reservation.
Ethics statement
The studies involving human participants were reviewed and approved by Heidelberg University Hospital Ethics Committee. Written informed consent to participate in this study was provided by the participants and legal guardian/next of kin.
Author contributions
All authors listed have made a substantial, direct, and intellectual contribution to the work and approved it for publication.
Funding
This research was funded by the Bundesministerium für Bildung und Forschung (BMBF, Federal Ministry of Education and Research) through the project “3DFoot” (01EC1907B).
Acknowledgments
We thank Dr. Sebastian Wolf for his help during the implementation of the project. We acknowledge the help of student assistants Lena Koch, Gregor Schlaucher, Mona Gläsle, and master student Jennifer Hiller from the University of Stuttgart during data collection.
Conflict of interest
The authors declare that the research was conducted in the absence of any commercial or financial relationships that could be construed as a potential conflict of interest.
Publisher’s note
All claims expressed in this article are solely those of the authors and do not necessarily represent those of their affiliated organizations, or those of the publisher, the editors and the reviewers. Any product that may be evaluated in this article, or claim that may be made by its manufacturer, is not guaranteed or endorsed by the publisher.
References
Ates, F., Brandenburg, J. E., and Kaufman, K. R. (2020). Effects of selective dorsal rhizotomy on ankle joint function in patients with cerebral palsy. Front. Pediatr. 8, 75. doi:10.3389/fped.2020.00075
Ates, F., Ozdeslik, R. N., Huijing, P. A., and Can, A., Y. (2013). Muscle lengthening surgery causes differential acute mechanical effects in both targeted and non-targeted synergistic muscles. J. Electromyogr. Kinesiol. 23, 1199–1205. doi:10.1016/j.jelekin.2013.05.010
Ates, F., Temelli, Y., and Yucesoy, C. A. (2018). Effects of antagonistic and synergistic muscles' co-activation on mechanics of activated spastic semitendinosus in children with cerebral palsy. Hum. Mov. Sci. 57, 103–110. doi:10.1016/j.humov.2017.11.011
Ateş, F., Temelli, Y., and Yucesoy, C. A. (2013). Human spastic Gracilis muscle isometric forces measured intraoperatively as a function of knee angle show no abnormal muscular mechanics. Clin. Biomech. (Bristol, Avon) 28, 48–54. doi:10.1016/j.clinbiomech.2012.08.012
Ates, F., Temelli, Y., and Yucesoy, C. A. (2014). Intraoperative experiments show relevance of inter-antagonistic mechanical interaction for spastic muscle's contribution to joint movement disorder. Clin. Biomech. (Bristol, Avon) 29, 943–949. doi:10.1016/j.clinbiomech.2014.06.010
Ates, F., Temelli, Y., and Yucesoy, C. A. (2016). The mechanics of activated semitendinosus are not representative of the pathological knee joint condition of children with cerebral palsy. J. Electromyogr. Kinesiol 28, 130–136. doi:10.1016/j.jelekin.2016.04.002
Attias, M., Chevalley, O., Bonnefoy-Mazure, A., De Coulon, G., Cheze, L., and Armand, S. (2016). Effects of contracture on gait kinematics: A systematic review. Clin. Biomech. (Bristol, Avon) 33, 103–110. doi:10.1016/j.clinbiomech.2016.02.017
Barber, L., Barrett, R., and Lichtwark, G. (2012). Medial gastrocnemius muscle fascicle active torque-length and Achilles tendon properties in young adults with spastic cerebral palsy. J. Biomech. 45, 2526–2530. doi:10.1016/j.jbiomech.2012.07.018
Bloom, T., and Sabharwal, S. (2022). Surgical management of foot and ankle deformities in cerebral palsy. Clin. Podiatr. Med. Surg. 39, 37–55. doi:10.1016/j.cpm.2021.09.001
Booth, C. M., Cortina-Borja, M. J., and Theologis, T. N. (2001). Collagen accumulation in muscles of children with cerebral palsy and correlation with severity of spasticity. Dev. Med. Child. Neurol. 43, 314–320. doi:10.1017/s0012162201000597
Borton, D. C., Walker, K., Pirpiris, M., Nattrass, G. R., and Graham, H. K. (2001). Isolated calf lengthening in cerebral palsy. Outcome analysis of risk factors. J. Bone Jt. Surg. Br. 83, 364–370. doi:10.1302/0301-620x.83b3.10827
Brierty, A., Walsh, H. P. J., Jeffries, P., Graham, D., Horan, S., and Carty, C. (2021). Dynamic muscle-tendon length following zone 2 calf lengthening surgery in two populations with equinus gait: Idiopathic Toe Walkers and Cerebral Palsy. Clin. Biomech. (Bristol, Avon) 84, 105323. doi:10.1016/j.clinbiomech.2021.105323
De Bruin, M., Smeulders, M. J., Kreulen, M., Huijing, P. A., and Jaspers, R. T. (2014). Intramuscular connective tissue differences in spastic and control muscle: A mechanical and histological study. PLoS One 9, e101038. doi:10.1371/journal.pone.0101038
Delp, S. L., and Zajac, F. E. (1992). Force- and moment-generating capacity of lower-extremity muscles before and after tendon lengthening. Clin. Orthop. Relat. Res. 284, 247–259. doi:10.1097/00003086-199211000-00035
Dietz, F. R., Albright, J. C., and Dolan, L. (2006). Medium-term follow-up of Achilles tendon lengthening in the treatment of ankle equinus in cerebral palsy. Iowa Orthop. J. 26, 27–32.
Dulhunty, A. F., and Franzini-Armstrong, C. (1977). The passive electrical properties of frog skeletal muscle fibres at different sarcomere lengths. J. Physiol. 266, 687–711. doi:10.1113/jphysiol.1977.sp011788
Engström, P., and Tedroff, K. (2018). Idiopathic toe-walking: Prevalence and natural history from birth to ten years of age. J. Bone Jt. Surg. Am. 100, 640–647. doi:10.2106/JBJS.17.00851
Farmer, S. E., and James, M. (2001). Contractures in orthopaedic and neurological conditions: A review of causes and treatment. Disabil. Rehabil. 23, 549–558. doi:10.1080/09638280010029930
Firth, G. B., Mcmullan, M., Chin, T., Ma, F., Selber, P., Eizenberg, N., et al. (2013). Lengthening of the gastrocnemius-soleus complex: An anatomical and biomechanical study in human cadavers. J. Bone Jt. Surg. Am. 95, 1489–1496. doi:10.2106/JBJS.K.01638
Gordon, A. M., Huxley, A. F., and Julian, F. J. (1966). The variation in isometric tension with sarcomere length in vertebrate muscle fibres. J. Physiol. 184, 170–192. doi:10.1113/jphysiol.1966.sp007909
Gurnett, C. A., Boehm, S., Connolly, A., Reimschisel, T., and Dobbs, M. B. (2008). Impact of congenital talipes equinovarus etiology on treatment outcomes. Dev. Med. Child Neurology 50, 498–502. doi:10.1111/j.1469-8749.2008.03016.x
Hemo, Y., Macdessi, S. J., Pierce, R. A., Aiona, M. D., and Sussman, M. D. (2006). Outcome of patients after Achilles tendon lengthening for treatment of idiopathic toe walking. J. Pediatr. Orthop. 26, 336–340. doi:10.1097/01.bpo.0000217743.44609.44
Herzog, W., Duvall, M., and Leonard, T. R. (2012). Molecular mechanisms of muscle force regulation: A role for titin? Exerc Sport Sci. Rev. 40, 50–57. doi:10.1097/JES.0b013e31823cd75b
Horsch, A., Gotze, M., Geisbusch, A., Beckmann, N., Tsitlakidis, S., Berrsche, G., et al. (2019). Prevalence and classification of equinus foot in bilateral spastic cerebral palsy. World J. Pediatr. 15, 276–280. doi:10.1007/s12519-019-00238-2
Horsch, A., Petzinger, L., Ghandour, M., Putz, C., Renkawitz, T., and Gotze, M. (2022). Defining equinus foot in cerebral palsy. Child. (Basel) 9, 956. doi:10.3390/children9070956
Huijing, P. A., and Jaspers, R. T. (2005). Adaptation of muscle size and myofascial force transmission: A review and some new experimental results. Scand. J. Med. Sci. Sports 15, 349–380. doi:10.1111/j.1600-0838.2005.00457.x
Kaya, C. S., Bilgili, F., Akalan, N. E., Temelli, Y., Ates, F., and Yucesoy, C. A. (2019). Intraoperative experiments combined with gait analyses indicate that active state rather than passive dominates the spastic gracilis muscle's joint movement limiting effect in cerebral palsy. Clin. Biomech. (Bristol, Avon) 68, 151–157. doi:10.1016/j.clinbiomech.2019.06.005
Kaya, C. S., Temelli, Y., Ates, F., and Yucesoy, C. A. (2018). Effects of inter-synergistic mechanical interactions on the mechanical behaviour of activated spastic semitendinosus muscle of patients with cerebral palsy. J. Mech. Behav. Biomed. Mater 77, 78–84. doi:10.1016/j.jmbbm.2017.08.040
Kreulen, M., and Smeulders, M. J. (2008). Assessment of Flexor carpi ulnaris function for tendon transfer surgery. J. Biomech. 41, 2130–2135. doi:10.1016/j.jbiomech.2008.04.030
Kruse, A., Rivares, C., Weide, G., Tilp, M., and Jaspers, R. T. (2021). Stimuli for adaptations in muscle length and the length range of active force exertion-A narrative review. Front. Physiol. 12, 742034. doi:10.3389/fphys.2021.742034
Lieber, R. L., Pontén, E., Burkholder, T. J., and Fridén, J. (1996). Sarcomere length changes after flexor carpi ulnaris to extensor digitorum communis tendon transfer. J. Hand Surg. Am. 21, 612–618. doi:10.1016/S0363-5023(96)80012-8
Linke, W. A., and Granzier, H. (1998). A spring tale: New facts on titin elasticity. Biophys. J. 75, 2613–2614. doi:10.1016/S0006-3495(98)77706-9
Linke, W. A., Kulke, M., Li, H., Fujita-Becker, S., Neagoe, C., Manstein, D. J., et al. (2002). PEVK domain of titin: An entropic spring with actin-binding properties. J. Struct. Biol. 137, 194–205. doi:10.1006/jsbi.2002.4468
Ma, N., Sclavos, N., Passmore, E., Thomason, P., Graham, K., and Rutz, E. (2021). Three-dimensional gait analysis in children undergoing gastrocsoleus lengthening for equinus secondary to cerebral palsy. Med. Kaunas. 57, 98. doi:10.3390/medicina57020098
Mathewson, M. A., Chambers, H. G., Girard, P. J., Tenenhaus, M., Schwartz, A. K., and Lieber, R. L. (2014). Stiff muscle fibers in calf muscles of patients with cerebral palsy lead to high passive muscle stiffness. J. Orthop. Res. 32, 1667–1674. doi:10.1002/jor.22719
Mcmulkin, M. L., Gordon, A. B., Tompkins, B. J., Caskey, P. M., and Baird, G. O. (2016). Long term gait outcomes of surgically treated idiopathic toe walkers. Gait Posture 44, 216–220. doi:10.1016/j.gaitpost.2015.12.013
Ozyalvac, O. N., Akpinar, E., Gur, V., Beng, K., Yagmurlu, M. F., and Bayhan, A. I. (2020). A formula to predict the magnitude of Achilles tendon lengthening required to correct equinus deformity. Med. Princ. Pract. 29, 75–79. doi:10.1159/000501603
Pilloni, G., Pau, M., Costici, F., Condoluci, C., and Galli, M. (2019). Use of 3D gait analysis as predictor of Achilles tendon lengthening surgery outcomes in children with cerebral palsy. Eur. J. Phys. Rehabil. Med. 55, 250–257. doi:10.23736/S1973-9087.18.05326-1
Prabhu, R. K., Swaminathan, N., and Harvey, L. A. (2013). Passive movements for the treatment and prevention of contractures. Cochrane Database Syst. Rev. 28, Cd009331. doi:10.1002/14651858.CD009331
Putz, C., Mertens, E. M., Wolf, S. I., Geisbüsch, A., Niklasch, M., Gantz, S., et al. (2018). Equinus correction during multilevel surgery in adults with cerebral palsy. Foot Ankle Int. 39, 812–820. doi:10.1177/1071100718765161
Rassier, D. E., Macintosh, B. R., and Herzog, W. (1999). Length dependence of active force production in skeletal muscle. J. Appl. Physiol. (1985) 86, 1445–1457. doi:10.1152/jappl.1999.86.5.1445
Rode, C., Siebert, T., and Blickhan, R. (2009). Titin-induced force enhancement and force depression: A 'sticky-spring' mechanism in muscle contractions? J. Theor. Biol. 259, 350–360. doi:10.1016/j.jtbi.2009.03.015
Rutz, E., Mccarthy, J., Shore, B. J., Shrader, M. W., Veerkamp, M., Chambers, H., et al. (2020). Indications for gastrocsoleus lengthening in ambulatory children with cerebral palsy: A delphi consensus study. J. Child. Orthop. 14, 405–414. doi:10.1302/1863-2548.14.200145
Ruzbarsky, J. J., Scher, D., and Dodwell, E. (2016). Toe walking: Causes, epidemiology, assessment, and treatment. Curr. Opin. Pediatr. 28, 40–46. doi:10.1097/MOP.0000000000000302
Smeulders, M. J., Kreulen, M., Hage, J. J., Huijing, P. A., and Van Der Horst, C. M. (2004). Intraoperative measurement of force-length relationship of human forearm muscle. Clin. Orthop. Relat. Res. 418, 237–241. doi:10.1097/00003086-200401000-00041
Sutherland, D. H., and Cooper, L. (1978). The pathomechanics of progressive crouch gait in spastic diplegia. Orthop. Clin. North Am. 9, 143–154. doi:10.1016/s0030-5898(20)30887-7
Swist, S., Unger, A., Li, Y., Voge, A., Von Frieling-Salewsky, M., Skarlen, A., et al. (2020). Maintenance of sarcomeric integrity in adult muscle cells crucially depends on Z-disc anchored titin. Nat. Commun. 11, 4479. doi:10.1038/s41467-020-18131-2
Ter Keurs, H. E., Iwazumi, T., and Pollack, G. H. (1978). The sarcomere length-tension relation in skeletal muscle. J. Gen. Physiol. 72, 565–592. doi:10.1085/jgp.72.4.565
Van Der Pijl, R. J., Hudson, B., Granzier-Nakajima, T., Li, F., Knottnerus, A. M., Smith, J., et al. (2020). Deleting titin's C-terminal PEVK exons increases passive stiffness, alters splicing, and induces cross-sectional and longitudinal hypertrophy in skeletal muscle. Front. Physiol. 11, 494. doi:10.3389/fphys.2020.00494
Wren, T. A., Rethlefsen, S., and Kay, R. M. (2005). Prevalence of specific gait abnormalities in children with cerebral palsy: Influence of cerebral palsy subtype, age, and previous surgery. J. Pediatr. Orthop. 25, 79–83. doi:10.1097/00004694-200501000-00018
Yngve, D. A., and Chambers, C. (1996). Vulpius and Z-lengthening. J. Pediatr. Orthop. 16, 759–764. doi:10.1097/00004694-199611000-00011
Yucesoy, C. A., Seref-Ferlengez, Z., and Huijing, P. A. (2013). In muscle lengthening surgery multiple aponeurotomy does not improve intended acute effects and may counter-indicate: An assessment by finite element modelling. Comput. Methods Biomech. Biomed. Engin 16, 12–25. doi:10.1080/10255842.2011.599803
Keywords: triceps surae, idiopathic foot deformity, in vivo muscle mechanics, muscle lengthening surgery, cerebral palsy
Citation: Brendecke E, Tsitlakidis S, Götze M, Hagmann S and Ates F (2023) Quantifying the effects of achilles tendon lengthening surgery: An intraoperative approach. Front. Physiol. 14:1143292. doi: 10.3389/fphys.2023.1143292
Received: 12 January 2023; Accepted: 23 February 2023;
Published: 06 March 2023.
Edited by:
Brad Palmer, University of Vermont, United StatesReviewed by:
Brent Momb, University of Massachusetts Amherst, United StatesYuan Wang, University of Vermont, United States
Copyright © 2023 Brendecke, Tsitlakidis, Götze, Hagmann and Ates. This is an open-access article distributed under the terms of the Creative Commons Attribution License (CC BY). The use, distribution or reproduction in other forums is permitted, provided the original author(s) and the copyright owner(s) are credited and that the original publication in this journal is cited, in accordance with accepted academic practice. No use, distribution or reproduction is permitted which does not comply with these terms.
*Correspondence: Filiz Ates, ZmlsaXouYXRlc0Bpc2QudW5pLXN0dXR0Z2FydC5kZQ==