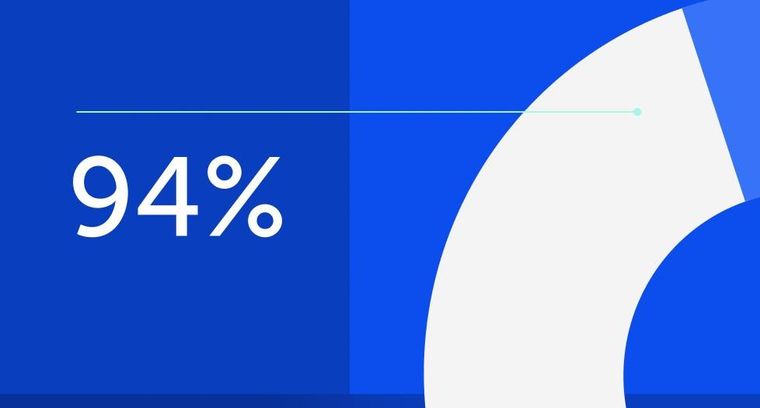
94% of researchers rate our articles as excellent or good
Learn more about the work of our research integrity team to safeguard the quality of each article we publish.
Find out more
ORIGINAL RESEARCH article
Front. Physiol., 09 May 2023
Sec. Computational Physiology and Medicine
Volume 14 - 2023 | https://doi.org/10.3389/fphys.2023.1136545
This article is part of the Research TopicRecent Advances in the Design and Preclinical Evaluation of Ventricular Assist DevicesView all 9 articles
Purpose: Blood damage has been associated with patients under temporary continuous-flow mechanical circulatory support. To evaluate the side effects caused by transit blood pumping, in vitro hemocompatibility testing for blood damage in pumps is considered a necessary reference before clinical trials.
Methods: The hemocompatibility of five extracorporeal centrifugal blood pumps was investigated comprehensively, including four commercial pumps (the Abbott CentriMag, the Terumo Capiox, the Medos DP3, and the Medtronic BPX-80) and a pump in development (the magAssist MoyoAssist®). In vitro, hemolysis was tested with heparinized porcine blood at nominal operating conditions (5 L/min, 160 mmHg) and extreme operating conditions (1 L/min, 290 mmHg) using a circulation flow loop. Hematology analyses concerning the blood cell counts and the degradation of high-molecular-weight von Willebrand factor (VWF) during 6-h circulation were also evaluated.
Results: Comparing the in vitro hemocompatibility of blood pumps at different operations, the blood damage was significantly more severe at extreme operating conditions than that at nominal operating conditions. The performance of the five blood pumps was arranged in different orders at these two operating conditions. The results also demonstrated superior hemocompatibility of CentriMag and MoyoAssist® at two operating conditions, with overall low blood damage at hemolysis level, blood cell counts, and degradation of high-molecular-weight VWF. It suggested that magnetic bearings have an advantage in hemocompatibility compared to the mechanical bearing of blood pumps.
Conclusion: Involving multiple operating conditions of blood pumps in in vitro hemocompatibility evaluation will be helpful for clinical application. In addition, the magnetically levitated centrifugal blood pump MoyoAssist® shows great potential in the future as it demonstrated good in vitro hemocompatibility.
Centrifugal blood pumps have been widely used, as ventricular assist devices (VADs) or as part of the extracorporeal membrane oxygenation (ECMO) circuit, in extracorporeal life support to drive blood in the circuit (Bottrell et al., 2014; Wu et al., 2021a). As a temporary continuous-flow mechanical circulatory support device, a centrifugal blood pump is considered clinically favorable (Nishida et al., 1993; Bennett et al., 2004). Despite the effectiveness of centrifugal blood pumps in prolonging the lives of heart failure patients, it has been reported that patients being bridged could develop a series of blood damage-related clinical hemocompatibility complications (Eckman and John, 2012; Kirklin et al., 2017). The complications could be the consequence of the side effect of pre-sheared blood in the blood pumps (Borchardt et al., 2012; Sun et al., 2020). When heart failure patients are treated with VADs, high-level non-physiological shear stress created by the pumps can damage blood components, including blood cells and protein in the plasma (Fraser et al., 2012; Chen et al., 2019).
To evaluate these side effects, hemocompatibility testing for overall blood damage caused by a centrifugal blood pump is considered a necessary reference before clinical trials. In vitro and in vivo tests are two commonly used ways of evaluating the hemocompatibility of blood pumps. However, scientific head-to-head comparisons cannot be made using a few samples because animal blood and hemodynamic differences can cause pumps to operate at different conditions. Thus, while performing a head-to-head hemocompatibility comparison test, an in vitro test is also recommended in preclinical studies. Using the in vitro hemolysis test, the red blood cell trauma caused by a developing blood pump can be quantitatively analyzed and evaluated, referring to key indicators in terms of the normalized hemolysis index (NIH) and modified hemolysis index (MIH) (Sobieski et al., 2012). Many studies demonstrated that compared to mechanical bearings, blood pumps using non-contacting bearings with advanced technologies like hydrodynamics suspension and/or magnetic levitation could alleviate damage to blood components (Ranganath et al., 2020; Wu et al., 2021b). In practice, blood damage-associated adverse events, e.g., hemolysis and in-pump thrombosis, were reduced (Kosaka et al., 2009; Bourque et al., 2016). The incidence of clinical complication gastrointestinal (GI) bleeding remains high (Bansal et al., 2019), which has been proven as a consequence of the loss of high-molecular-weight von Willebrand factor (VWF) after continuous-flow mechanical circulatory support in clinics (Stockschlaeder et al., 2014; Bartoli et al., 2015). Therefore, it is necessary to involve VWF damage in the hemocompatibility test of centrifugal blood pumps.
Although extracorporeal centrifugal blood pumps were initially designed for a specific nominal operating condition, they could be manipulated into a broader operation range in a clinic due to the various settings, the resistance of different pipelines, or under the worst-case clinical scenario (Schöps et al., 2021). Moreover, neonatal or pediatric patients receiving ECMO and patients undergoing extracorporeal CO2 removal represented a lower blood flow rate than conventional ECMO (Schöps et al., 2021). It has been verified that blood damage in blood pumps varies widely under different operating conditions (Gross-Hardt et al., 2019; Kuck et al., 2021). In particular, adverse events such as hemolysis, clotting, and GI bleeding complications were frequently reported at low blood flow rate operations (Gross-Hardt et al., 2019; Schöps et al., 2021). Hence, the overall blood damage in centrifugal blood pumps at a more comprehensive operating condition range should also be investigated to provide a more thorough evaluation, especially the extreme work condition (low flow rate and high-pressure head) (Chan et al., 2015; Radley et al., 2019).
In this study, comprehensive perspectives on blood damage from five centrifugal blood pumps were investigated, including four commercial pumps and a pump in development. The comparison of three mainstream commercial extracorporeal blood pumps and a new fully maglev extracorporeal blood pump with the clinical standard CentriMag was conducted. The influence of different operating conditions on hemocompatibility was also evaluated. Here, we present our study on the in vitro hemolysis level of five blood pumps at nominal operating conditions (5 L/min, 160 mmHg) and extreme operating conditions (1 L/min, 290 mmHg) using a circulation flow loop. The 160-mmHg pressure head at nominal operating conditions was due to the resistance of the peripheral tubes used in clinical practice. The extreme operating condition was chosen to reflect the worst-case clinical scenario. Hematology analysis concerning change in the number of white blood cells and platelets over time was conducted. The degradation of high-molecular-weight VWF multimers during 6-h circulation was also evaluated.
The following extracorporeal centrifugal blood pumps were included in this study: the CentriMag magnetically levitated centrifugal pump (Abbott, Thoratec, Pleasanton, CA, United States), the Capiox centrifugal pump (Terumo Corp., Tokyo, Japan), the Deltastream DP3 diagonal rotary pump (Medos Medizintechnik AG, Stolberg, Germany), the Bio-pump BPX-80 (Medtronic, Inc., Minneapolis, MN, United States), and the MoyoAssist® device (magAssist., Inc., Suzhou, China). The Deltastream DP3 is a rotary blood pump with combined characteristics of an axial and a radial design. All the blood pumps are commercially used devices, except magAssist MoyoAssist®. MoyoAssist® is a magnetically levitated extra-VAD that could provide bi-ventricular support for patients with acute heart failure (Li et al., 2022). Different from CentriMag and MoyoAssist®, Capiox, DP3, and BPX-80 are mechanical bearing centrifugal pumps. The maximum flow rate of CentriMag, BPX-80, and MoyoAssist® is 10 L/min, while that of Capiox and DP3 is 8 L/min. The maximum operating pressures of CentriMag, Capiox, DP3, BPX-80, and MoyoAssist® are 600 mmHg, 800 mmHg, 600 mmHg, 1,100 mmHg, and above 600 mmHg, respectively.
Domestic porcine blood with 0.4% heparin sodium anticoagulant was purchased from Suzhou Frankenman Medical Devices Co., Ltd. The activated coagulation time (ACT) of blood was kept greater than 300 s (ISO, 2016). The in vitro blood circulation loop was built referring to the American Society for Testing and Materials (ASTM) standard F1841-97 (Figure 1) (ASTM, 2019). For each set of tests, four loops were operated simultaneously, and the blood was taken from one pig. Due to the limited blood volume per porcine model, the loop was designed smaller than the ASTM standard, and the option of centrifuging blood for controlling the hematocrit was not performed. The hematocrit (Hct) of blood in the loop was controlled at 21% ± 4%, and the total blood volume was 310 ± 10 mL. The total hemoglobin at time zero was 8.3 ± 1.0 g/dL. The blood pumps were tested under stable hemodynamic conditions for 6 h on nominal (5 ± 0.25 L/min, 160 ± 5 mmHg) and extreme (1 ± 0.05 L/min, 290 ± 8 mmHg) operations, respectively. The flow rate was measured using an ultrasonic flow sensor (T402, Transonic Systems Inc., Ithaca, NY, United States). The differential pressure across the pump was measured using pressure sensors (DPT-01, Nora, Shenzhen, China). All the test loops and a separate static control blood bag were immersed in the thermostatic water bath to maintain a blood temperature of 37°C ± 1°C. During the experiment, blood samples were collected through the sample port at 0, 30, 60, 120, 180, 240, 300, and 360 min. The blood samples at time zero were obtained from blood that circulated for 5 min to ensure complete mixing. The first 2 mL of the blood sample was discarded, followed by a second volume of 3 mL. Blood samples from the static control blood bag were collected in the same way at 0, 120, 240, and 360 min.
FIGURE 1. Schematic diagram and picture of the blood circulation loop. The loop setup allows for control of temperature, flow rate, and pressure head.
Blood samples were centrifuged twice at ×1,500 g for 10 min, and the supernatants were collected to measure plasma-free hemoglobin. Samples were diluted 10 times with 0.01% Na2CO3 solution, and then, the triplicate was added to a 96-well labeled plate (Jet Biofil, Guangzhou, China). The absorbance was measured using the SpectraMax Paradigm Multi-Mode Microplate Reader (Molecular Devices, California, United States) at three wavelengths of 380 nm, 415 nm, and 450 nm. The absorbance of each sample at three wavelengths of 415 nm, 380 nm, and 450 nm was denoted as A415, A380, and A450, and the absorbance difference of each sample was calculated according to
where Y represents the absorbance difference of each sample. Then, the value Y was substituted back into the standard formula
Here,
ΔpfHb: increase of the plasma-free hemoglobin concentration (g/L)
Hb: total blood hemoglobin concentration at time zero (g/dL)V: blood volume in the loop (mL)
Q: flow rate (L/min)Hct: hematocrit (%)
T: sampling time (min)
The porcine blood samples from both the circulation loop and static control blood bag were collected for hematology analysis at time points 0, 120, 240, and 360 min. The total numbers of white blood cells and platelets in the samples were determined using the clinical automatic hematology system (ADVIA 2120i, Siemens Healthcare Diagnostics Inc., Erlangen, Germany).
Blood samples were centrifuged at 15,000 g for 5 min to collect the supernatant and remove debris. The VWF multimers in plasma samples were separated by gel-electrophoresis for 3.5 h and transferred onto a 0.45-µm polyvinylidene difluoride membrane (Immobilon-P; Millipore Corporation, Bedford, MA, United States) overnight. Then, primary antibody (Polyclonal Rabbit anti-Human von Willebrand factor, Cell Signaling Technology, Boston, United States) and secondary antibody (Polyclonal Rabbit anti-Rabbit lgG HRP-linked antibody, Massachusetts, United States) tests were performed to detect the VWF multimers’ molecular weight distribution on the film. The whole process included five main steps: SDS agarose gel preparation, electrophoresis, Western blot, immunolocalization, and visualization. After the visualization step, ImageJ software was used to process the VWF bands’ image obtained from the Kodak film (Rayco Medical Products Company Limited for Carestream Health, Xiamen, China) development. The high-molecular-weight VWF multimers section was selected to acquire the gray value corresponding to each sample. This value did not represent the actual molecular weight of VWF. The loss percentage of high molecular weight VWF multimers was expressed as
where Hsample is the gray value of high molecular weight bands corresponding to each blood sample and Hcontrol is the gray value of high molecular weight bands corresponding to each blood sample at time zero.
Three valid measurements were obtained from repeated tests of the MoyoAssist® and CentriMag centrifugal blood pumps. Due to the limitations of the experimental conditions, two accurate repeated measurements were obtained for BPX-80 on the nominal operation and Capiox on the extreme operation. Since DP3 and BPX-80 were only used for a single test on extreme operation, no statistical analysis was performed. The averages and standard deviations were calculated using data analysis software (Origin version 9, OriginLab, Northampton, MA, United States). Due to multiple measurements taken from each blood pump, statistical analysis was carried out using one-way ANOVA. A value of p < 0.05 (**) was considered to be statistically significant.
The result of in vitro hemolysis in terms of NIH and MIH for all the test blood pumps is shown in Figure 2. The hemolysis values of all the test pumps at extreme operating conditions were 6–13 times higher than those of the nominal operating conditions. At both nominal and extreme operating conditions, the averages of NIH and MIH ranked from low to high were MoyoAssist®, CentriMag, Capiox, DP3, and BPX-80. There was a significant difference among the five devices at the nominal operating condition (p = 0.01), with a significant difference between MoyoAssist® and DP3 (p = 0.03), MoyoAssist® and BPX-80 (p = 0.02), and CentriMag and BPX-80 (p = 0.03). No significant difference was demonstrated among MoyoAssist®, CentriMag, and Capiox at extreme operating conditions. DP3 and BPX-80, on extreme operation, were not statistically comparable since they were only conducted for a single test. However, they presented approximately 1–4 folds higher levels of hemolysis than the other three devices.
FIGURE 2. Averages of (A) NIH and (B) MIH for all the centrifugal blood pumps. The sample sizes were MoyoAssist® (n = 3; n = 3), CentriMag (n = 3; n = 3), DP3 (n = 3; n = 1), Capiox (n = 3; n = 2), and BPX-80 (n = 2; n = 1), representing nominal operating conditions and extreme operating conditions. Porcine blood subjected to circulation loops for 6 h. Hemolysis was measured and calculated in accordance with ASTM. A value of p < 0.05 (**) was considered to be statistically significant.
The result of white blood cell and platelet counts every 2 hours for all the test blood pumps is shown in Figure 3. Due to the differences in the data baseline of blood samples, the ratios of each sample to the sample at time zero were presented. On nominal operation, there was a significant decrease in the white blood cell count over time for CentriMag and Capiox (p < 0.05) (Figure 3A). The number of white blood cells was reduced by more than 10% for both of them after 6-h circulation. No significant decrease in white blood cell numbers over time was observed for the other three devices. On extreme operation, there was a significant decrease over time for all the blood pumps (p < 0.05) (Figure 3C). The number of white blood cells was reduced by 10%–15% after a 6-h in vitro hemolysis test under extreme operating conditions. It indicated more damage to white blood cells at a low flow rate and high pressure head. In addition, no significant difference in white blood cell numbers was observed between the static control sample and blood pump samples at 360 min for MoyoAssist® and Capiox. Since Capiox showed a significant decrease in white blood cell count over time on nominal operation, MoyoAssist® performed best among the five devices at white blood cell counting. For the platelet count, there was no significant change over the testing period for all the blood pumps at both nominal and extreme operating conditions (Figures 3B, D). There was also no significant difference in platelet numbers between the static control sample and all the blood pump samples at 360 min.
FIGURE 3. Comparison of temporal changes among control and centrifugal blood pumps on (A) white blood cells at nominal operating conditions, (B) platelets at nominal operating conditions, (C) white blood cells at extreme operating conditions, and (D) platelets at extreme operating conditions. The sample sizes were the same as those for the hemolysis test. Porcine blood was subjected to a circulation loop for 6 h and measured by automatic hematology analysis every 2 h. Results expressed as mean ± SD, % relative to each time zero samples. A value of p < 0.05 (**) was considered to be statistically significant.
For the study of high-molecular-weight VWF degradation, blood samples at time points 0, 30, 60, 120, 180, 240, 300, and 360 min were tested. During the 6-h test, a part of the high-molecular-weight VWF cut off to be small molecules under high shear stress. The representative image of VWF multimer bands obtained by immunoblotting is shown in Figure 4. The section in the dotted box represented the high-molecular-weight VWF multimer bands. The result indicated a gradual loss of high-molecular-weight VWF multimers over time for all the test blood pumps at both nominal and extreme operating conditions. Based on the representative images, the degradation of high-molecular-weight VWF multimers (%) was calculated (Eq. 4) and is shown in Figure 5. On nominal operation, the most severe degradation of VWF occurred in the first 2 h of the experiment (Figure 5A). It started to fluctuate after that. The degradation of high-molecular-weight VWF multimers was less than 20% during the entire test period. There was a significant difference among the five devices at the time point 360 min (p = 0.001). BPX-80 demonstrated a significant difference with the other four devices (p < 0.05), with at least 6% more degradation of high-molecular-weight VWF. On extreme operation, there was a significant increase in high-molecular-weight VWF degradation over time for all the test blood pumps (Figure 5B). Meanwhile, all the degradation of high-molecular-weight VWF multimers was more than 20% during the entire test period. It was obviously more severe than the VWF damage under nominal operation. There was a significant difference among MoyoAssist®, CentriMag, and Capiox at time point 360 min (p = 0.001). Capiox demonstrated a significant difference with the other two devices (p < 0.05), with 10% more degradation of high-molecular-weight VWF.
FIGURE 4. Representative immunoblot gel image of VWF multimers for all the centrifugal blood pumps at (A) nominal operating conditions and (B) extreme operating conditions. The sample sizes were the same as those for the hemolysis test. Porcine blood was subjected to circulation loops for 6 h and then measured by immunoblotting.
FIGURE 5. Degradation of high-molecular weight VWF multimers for all the centrifugal blood pumps at (A) nominal operating conditions and (B) extreme operating conditions. Results expressed as mean ± SD, % relative to each time zero sample. A value of p < 0.05 (**) was considered to be statistically significant.
In this study, the blood damage caused by four commercial extracorporeal centrifugal blood pumps widely used in clinics was comprehensively investigated. Experimental research on the influence of different operating conditions was conducted. The blood compatibility of a full maglev extracorporeal blood pump in development was also evaluated. All the centrifugal blood pumps were operated at nominal operating conditions (5 L/min, 160 mmHg) and extreme operating conditions (1 L/min, 290 mmHg) for clinical relevance. We did not consider conducting the experiments for right-sided support conditions (a high flow rate and low-pressure head) because the hemolysis will be lower under a lower pressure head. We considered the results under the worst case (a low flow rate and high-pressure head) more valuable. Hemolysis, calculated by the amount of plasma-free hemoglobin, is a reliable marker for red blood cell damage. The variable coefficient of NIH and MIH could be acceptable as in other certified labs. The hemolysis results of commercial pumps were consistent with the data in the literature (Hijikata et al., 2008; Schibilsky et al., 2020). MoyoAssist® and CentriMag demonstrated lower hemolysis levels might be due to their non-contacting bearings associated with lower shear stress in pumps. The reason for the lower hemolysis of Capiox than DP3 and BPX-80 may be the lower operating pump speed (max. 3,000 rpm) that cause lower shear stress than the other two devices. White blood cells and platelets play an essential role in infection and thrombosis. Thus, their damage could contribute to adverse events in the clinic. The phenomenon of slightly increased platelet counts (false-positive) in the automatic hematology analyzer has been confirmed to be associated with the release of microparticles from white blood cells (Radley et al., 2019).
It is known that the VWF damage caused by centrifugal blood pumps is a major factor in GI bleeding. Under non-physiological shear stress, the high-molecular-weight multimers of VWF will easily be degraded into small molecules and thus cannot participate in the coagulation process. The high-molecular-weight VWF decreased by less than 20% during the test period for all the blood pumps on nominal operation, which is consistent with the published data on CentriMag. The result indicated that the loss of high-molecular-weight VWF over time exists in all the centrifugal blood pumps; thus, it needs to be paid attention to in preclinical testing. Interestingly, BPX-80, which performed much worse than the other blood pumps in hemolysis, demonstrated low VWF damage at extreme operation. This phenomenon may be related to the different damage mechanisms of red blood cells and VWF (Mei et al., 2022a; Mei et al., 2022b). The shear stress threshold for the damage to VWF was much lower than the damage to red blood cells (Nascimbene et al., 2016). Other factors also affect the degradation of high-molecular-weight VWF besides high shear stress, such as a surface that easily adheres to VWF and unfolds the chain segments. Therefore, the blood pump with higher shear stress may cause more hemolysis but not necessarily yield more damage to VWF. In addition, the rate of VWF degradation was the fastest at the beginning, followed by a gradual decline. It suggested that VWF damage induced by non-physiological shear stress is rapid and irreversible.
Comparing the in vitro hemocompatibility of blood pumps at different operations, the blood damage was more severe at extreme operating conditions than that at nominal operating conditions. It was consistent with the published data (Chan et al., 2015). At the same flow rate, the hemolysis index increased with the increase of pressure head. In addition, the hemolysis index decreased with the increase in flow rate at the same pressure head. Another research showed lower hemolysis in pediatric LVAD operating conditions than in adult LVAD operating conditions, with about half of the flow rate and 70% of the pressure head (Kuck et al., 2021). The order of most to least hemolysis remained the same as the nominal operating condition. However, the order of most to least VWF damage was not the same at different operations. This may also be related to the different damage mechanisms of red blood cells and VWF, as mentioned in the previous paragraph. The more severe blood damage in blood pumps at extreme operating conditions could be associated with more adverse events in clinics. Therefore, special attention should be paid to the hemocompatibility performance of extracorporeal centrifugal blood pumps at extreme operation to reduce adverse events in clinical practice.
Third-generation VAD utilizing non-contacting bearings with hydrodynamic suspension and/or magnetic levitation technology has been developed to reduce blood damage-associated adverse events. Compared to mechanical bearing, a non-contacting bearing allows for a bigger secondary flow path and, thus, lower shear stress distributed in the blood pump. Therefore, pumps with non-contacting bearings were supposed to perform better than those with mechanical bearings in in vitro hemocompatibility. CentriMag and MoyoAssist® involved in this study are magnetically levitated centrifugal pumps, while Capiox, DP3, and BPX-80 are mechanical bearing centrifugal pumps. The results demonstrated the superior hemocompatibility of CentriMag and MoyoAssist®, with overall low blood damage at the hemolysis level, blood cell counts, and degradation of high-molecular-weight VWF. They were expected to reduce adverse events associated with blood damage in clinical practice.
The CentriMag centrifugal blood pump has a long successful history of clinical use and caused low blood damage during in vitro testing (Zhang et al., 2006; Chan et al., 2015). In this study, as the only pump in development, MoyoAssist® demonstrated even lower white blood cell damage than CentriMag. The findings suggested that non-contacting bearings with magnetic levitation have an absolute advantage in comprehensive hemocompatibility compared to mechanical bearings in blood pumps. Since the MoyoAssist® blood pump shows good performance in in vitro blood damage testing, it has great potential in the future.
In addition, the blood circulation loop built in this study consisted of 1 m of 3/8″-diameter tubing. The total blood volume in the loop was 310 ± 10 mL. It could be considered as a downscaled test loop with a lower priming volume according to the old version of the ASTM standard (2017). However, a mini test-loop was proved to significantly better differentiate the blood pumps with fewer adverse effects by the loop itself (Woelke et al., 2020). A 160-mL test loop provided significantly higher plasma-free hemoglobin increase and consistently stronger VWF degradation than a 480-mL test loop. Therefore, to test as many circuits as possible simultaneously, a downscaled circulation loop may be useful to evaluate in vitro blood damage.
In this study, all the centrifugal blood pumps were evaluated by in vitro models with well-controlled experimental conditions. Clinical scenarios integrate a plethora of variables, especially patient-related, which are associated with phenomena such as hemolysis, leukopenia, and altered hemostasis. Therefore, the results from an in vitro study may be different from clinical practice. In addition, the low sample size was due to the limited pump heads and the small number of animals as the blood source. Repeated tests at extreme operating conditions were not conducted for DP3 and BPX-80. In addition, all the centrifugal blood pumps were tested at operating conditions for the extracorporeal VAD application. In the future, the in vitro blood damage tests will be conducted at ECMO operating conditions to reflect the hemocompatibility for cardiopulmonary support application. The platelet activation will also be measured to evaluate the risk of clot formation.
In this study, the hemocompatibility of five extracorporeal centrifugal blood pumps was investigated comprehensively. Compared to mechanical bearing blood pumps, the magnetically levitated centrifugal pumps CentriMag and MoyoAssist® demonstrated an advantage in hemocompatibility under both nominal and extreme operations. As a pump in development, the MoyoAssist® blood pump has good in vitro hemocompatibility. In addition, the blood damage was more severe at extreme operating conditions than that at nominal operating conditions. The performance of the five blood pumps was arranged in different orders at these two operating conditions. Therefore, including multiple operating conditions of blood pumps in in vitro hemocompatibility evaluation will be helpful for clinical application.
The original contributions presented in the study are included in the article/supplementary material; further inquiries can be directed to the corresponding authors.
ND, PL, and P-LH conceived and designed the experiments; XM, WG, MZ, NH, and QJ performed the experiments; XM and LZ analyzed the data; LZ drafted the manuscript; PL, WG, TW, P-LH, and US revised the paper. All authors reviewed and approved the manuscript. All authors contributed to the article and approved the submitted version.
The authors disclosed receipt of the following financial support for the research, authorship, and/or publication of this article: This work was supported by the Young Overseas High-Level Talents Program, the Organization Department of the Central Committee of the CPC, and the National Natural Science Foundation of China (grant number 31700817).
P-LH is the founder and CEO of magAssist, Inc.
The remaining authors declare that the research was conducted in the absence of any commercial or financial relationships that could be construed as a potential conflict of interest.
All claims expressed in this article are solely those of the authors and do not necessarily represent those of their affiliated organizations, or those of the publisher, the editors, and the reviewers. Any product that may be evaluated in this article, or claim that may be made by its manufacturer, is not guaranteed or endorsed by the publisher.
ASTM (2019). ASTM F1841-97 standard practice for assessment of hemolysis in continuous flow blood pumps. United States: ASTM International. doi:10.1520/F1841-19.clinical
Bansal, A., Uriel, N., Colombo, P. C., Narisetty, K., Long, J. W., Bhimaraj, A., et al. (2019). Effects of a fully magnetically levitated centrifugal-flow or axial-flow left ventricular assist device on von Willebrand factor: A prospective multicenter clinical trial. J. Hear Lung Transpl. 38, 806–816. doi:10.1016/j.healun.2019.05.006
Bartoli, C. R., Restle, D. J., Zhang, D. M., Acker, M. A., and Atluri, P. (2015). Pathologic von Willebrand factor degradation with a left ventricular assist device occurs via two distinct mechanisms: Mechanical demolition and enzymatic cleavage. J. Thorac. Cardiovasc Surg. 149, 281–289. doi:10.1016/j.jtcvs.2014.09.031
Bennett, M., Horton, S., Thuys, C., Augustin, S., Rosenberg, M. B. C., and Brizard, C. (2004). Pump-induced haemolysis: A comparison of short-term ventricular assist devices. Perfusion 19, 107–111. doi:10.1191/0267659104pf729oa
Borchardt, R., Schlanstein, P., Mager, I., Arens, J., Schmitz-Rode, T., and Steinseifer, U. (2012). In vitro performance testing of a pediatric oxygenator with an integrated pulsatile pump. ASAIO J. 58, 420–425. doi:10.1097/MAT.0b013e318251dc70
Bottrell, S., Bennett, M., Augustin, S., Thuys, C., Schultz, B., Horton, A., et al. (2014). A comparison study of haemolysis production in three contemporary centrifugal pumps. Perfusion 29, 411–416. doi:10.1177/0267659113509000
Bourque, K., Cotter, C., Dague, C., Harjes, D., Dur, O., Duhamel, J., et al. (2016). Design rationale and preclinical evaluation of the HeartMate 3 left ventricular assist system for hemocompatibility. ASAIO J. 62, 375–383. doi:10.1097/MAT.0000000000000388
Chan, C. H., Pieper, I. L., Hambly, R., Radley, G., Jones, A., Friedmann, Y., et al. (2015). The CentriMag centrifugal blood pump as a benchmark for in vitro testing of hemocompatibility in implantable ventricular assist devices. Artif. Organs 39, 93–101. doi:10.1111/aor.12351
Chen, Z., Sun, A., Wang, H., Fan, Y., and Deng, X. (2019). Non-physiological shear stress-induced blood damage in ventricular assist device. Med. Nov. Technol. Devices 3, 100024. doi:10.1016/j.medntd.2019.100024
Eckman, P. M., and John, R. (2012). Bleeding and thrombosis in patients with continuous-flow ventricular assist devices. Circulation 125, 3038–3047. doi:10.1161/CIRCULATIONAHA.111.040246
Fraser, K. H., Zhang, T., Taskin, M. E., Griffith, B. P., and Wu, Z. J. (2012). A quantitative comparison of mechanical blood damage parameters in rotary ventricular assist devices: Shear stress, exposure time and hemolysis index. J. Biomech. Eng. 134, 081002. doi:10.1115/1.4007092
Gross-Hardt, S., Hesselmann, F., Arens, J., Steinseifer, U., Vercaemst, L., Windisch, W., et al. (2019). Low-flow assessment of current ECMO/ECCO2R rotary blood pumps and the potential effect on hemocompatibility. Crit. Care 23, 348–349. doi:10.1186/s13054-019-2622-3
Hijikata, W., Shinshi, T., Asama, J., Li, L., Hoshi, H., Takatani, S., et al. (2008). A magnetically levitated centrifugal blood pump with a simple-structured disposable pump head. Artif. Organs 32, 531–540. doi:10.1111/j.1525-1594.2008.00576.x
ISO (2016). ISO 18242: 2016 cardiovascular implants and extracorporeal systems — centrifugal blood pumps. Berlin, Germany: Springer.
Kirklin, J. K., Pagani, F. D., Kormos, R. L., Stevenson, L. W., Blume, E. D., Myers, S. L., et al. (2017). Eighth annual INTERMACS report: Special focus on framing the impact of adverse events. J. Hear Lung Transpl. 36, 1080–1086. doi:10.1016/j.healun.2017.07.005
Kosaka, R., Maruyama, O., Nishida, M., Yada, T., Saito, S., Hirai, S., et al. (2009). Improvement of hemocompatibility in centrifugal blood pump with hydrodynamic bearings and semi-open impeller: In vitro evaluation. Artif. Organs 33, 798–804. doi:10.1111/j.1525-1594.2009.00817.x
Kuck, L., Simmonds, M. J., and Chan, C. H. H. (2021). Ex vivo assessment of erythrocyte tolerance to the HeartWare ventricular assist device operated in three discrete configurations. Artif. Organs 45, E146–E157. doi:10.1111/aor.13877
Li, P., Wu, T., Hsu, P. L., Wei, X., and Dong, N. (2022). 30-Day in vivo study of a fully maglev extracorporeal ventricular assist device. Artif. Organs 46, 2171–2178. doi:10.1111/aor.14317
Mei, X., Lu, B., Zhong, M., Zhu, Y., Zhang, L., and Ge, W. (2022). The influence of surface roughness on the damage of von Willebrand Factor under shear flow condition. Int. J. Artif. Organs 45, 412–420. doi:10.1177/03913988211056961
Mei, X., Zhong, M., Ge, W., and Zhang, L. (2022). Mathematical models for shear-induced blood damage based on vortex platform. Int. J. Artif. Organs 45, 397–403. doi:10.1177/03913988211003587
Nascimbene, A., Neelamegham, S., Frazier, O. H., Moake, J. L., and Dong, J. F. (2016). Acquired von Willebrand syndrome associated with left ventricular assist device. Blood 127, 3133–3141. doi:10.1182/blood-2015-10-636480
Nishida, H., Yamaki, F., Nakatani, H., Endo, M., Koyanagi, H., Oshiyama, H., et al. (1993). Development of the Terumo Capiox centrifugal pump and its clinical application to open heart surgery: A comparative study with the roller pump. Artif. Organs 17, 323–327. doi:10.1111/j.1525-1594.1993.tb00588.x
Radley, G., Pieperlaura, I., and Robinson, C. R. (2019). In vitro benchmarking study of ventricular assist devices in current clinical use. J. Card. Fail 00, 1–10.
Ranganath, N. K., Rashidi, M., Antaki, J. F., Phillips, K. G., Kon, Z. N., Smith, D. E., et al. (2020). Mechanical blood-immersed bearings in continuous-flow rotary blood pumps. ASAIO J. 66, 343–347. doi:10.1097/MAT.0000000000000994
Schibilsky, D., Takatani, S., Schibilsky, B., Graf, T., da Silva, D. M., Wendel, H. P., et al. (2020). Hemocompatibility of new magnetically-levitated centrifugal pump technology compared to the CentriMag adult pump. Sci. Rep. 10, 22055–22058. doi:10.1038/s41598-020-78709-0
Schöps, M., Groß-Hardt, S. H., Schmitz-Rode, T., Steinseifer, U., Brodie, D., Clauser, J. C., et al. (2021). Hemolysis at low blood flow rates: Iin-vitro and in-silico evaluation of a centrifugal blood pump. J. Transl. Med. 19, 2. doi:10.1186/s12967-020-02599-z
Sobieski, M. A., Giridharan, G. A., Ising, M., Koenig, S. C., and Slaughter, M. S. (2012). Blood trauma testing of CentriMag and RotaFlow centrifugal flow devices: A pilot study. Artif. Organs 36, 677–682. doi:10.1111/j.1525-1594.2012.01514.x
Stockschlaeder, M., Schneppenheim, R., and Budde, U. (2014). Update on von Willebrand factor multimers: Focus on high-molecular-weight multimers and their role in hemostasis. Blood Coagul. Fibrinolysis 25, 206–216. doi:10.1097/MBC.0000000000000065
Sun, W., Wang, S., Chen, Z., Zhang, J., Arias, K., et al. (2020). Impact of high mechanical shear stress and oxygenator membrane surface on blood damage relevant to thrombosis and bleeding in a pediatric ECMO circuit. Artif. Organs 44, 717–726. doi:10.1111/aor.13646
Woelke, E., Klein, M., Mager, I., Schmitz-Rode, T., Steinseifer, U., Arens, J., et al. (2020). Miniaturized test loop for the assessment of blood damage by continuous-flow left-ventricular assist devices. Ann. Biomed. Eng. 48, 768–779. doi:10.1007/s10439-019-02404-z
Wu, P., Huo, J., Dai, W., Wu, W. T., Yin, C., and Li, S. (2021). On the optimization of a centrifugal maglev blood pump through design variations. Front. Physiol. 12, 699891–699910. doi:10.3389/fphys.2021.699891
Wu, P., Xiang, W., Yin, C., and Li, S. (2021). The design and evaluation of a portable extracorporeal centrifugal blood pump. Front. Physiol. 12, 766867–7. doi:10.3389/fphys.2021.766867
Keywords: centrifugal pump, ECMO, hemolysis, von Willebrand factor, blood damage
Citation: Li P, Mei X, Ge W, Wu T, Zhong M, Huan N, Jiang Q, Hsu P-L, Steinseifer U, Dong N and Zhang L (2023) A comprehensive comparison of the in vitro hemocompatibility of extracorporeal centrifugal blood pumps. Front. Physiol. 14:1136545. doi: 10.3389/fphys.2023.1136545
Received: 03 January 2023; Accepted: 20 April 2023;
Published: 09 May 2023.
Edited by:
Zengsheng Chen, Beihang University, ChinaReviewed by:
Guruprasad A. Giridharan, University of Louisville, United StatesCopyright © 2023 Li, Mei, Ge, Wu, Zhong, Huan, Jiang, Hsu, Steinseifer, Dong and Zhang. This is an open-access article distributed under the terms of the Creative Commons Attribution License (CC BY). The use, distribution or reproduction in other forums is permitted, provided the original author(s) and the copyright owner(s) are credited and that the original publication in this journal is cited, in accordance with accepted academic practice. No use, distribution or reproduction is permitted which does not comply with these terms.
*Correspondence: Nianguo Dong, ZG9uZ25pYW5ndW9AaG90bWFpbC5jb20=; Liudi Zhang, bGl1ZGlAc3VkYS5lZHUuY24=
Disclaimer: All claims expressed in this article are solely those of the authors and do not necessarily represent those of their affiliated organizations, or those of the publisher, the editors and the reviewers. Any product that may be evaluated in this article or claim that may be made by its manufacturer is not guaranteed or endorsed by the publisher.
Research integrity at Frontiers
Learn more about the work of our research integrity team to safeguard the quality of each article we publish.