- 1Centre for Heart Lung Innovation, St. Paul’s Hospital, Vancouver, BC, Canada
- 2Department of Anesthesiology, Pharmacology and Therapeutics, University of British Columbia, Vancouver, BC, Canada
Asthma affects an estimated 262 million people worldwide and caused over 461,000 deaths in 2019. The disease is characterized by chronic airway inflammation, reversible bronchoconstriction, and airway remodeling. Longitudinal studies have shown that current treatments for asthma (inhaled bronchodilators and corticosteroids) can reduce the frequency of exacerbations, but do not modify disease outcomes over time. Further, longitudinal studies in children to adulthood have shown that these treatments do not improve asthma severity or fixed airflow obstruction over time. In asthma, fixed airflow obstruction is caused by remodeling of the airway wall, but such airway remodeling also significantly contributes to airway closure during bronchoconstriction in acute asthmatic episodes. The goal of the current review is to understand what is known about the heterogeneity of airway remodeling in asthma and how this contributes to the disease process. We provide an overview of the existing knowledge on airway remodeling features observed in asthma, including loss of epithelial integrity, mucous cell metaplasia, extracellular matrix remodeling in both the airways and vessels, angiogenesis, and increased smooth muscle mass. While such studies have provided extensive knowledge on different aspects of airway remodeling, they have relied on biopsy sampling or pathological assessment of lungs from fatal asthma patients, which have limitations for understanding airway heterogeneity and the entire asthma syndrome. To further understand the heterogeneity of airway remodeling in asthma, we highlight the potential of in vivo imaging tools such as computed tomography and magnetic resonance imaging. Such volumetric imaging tools provide the opportunity to assess the heterogeneity of airway remodeling within the whole lung and have led to the novel identification of heterogenous gas trapping and mucus plugging as important predictors of patient outcomes. Lastly, we summarize the current knowledge of modification of airway remodeling with available asthma therapeutics to highlight the need for future studies that use in vivo imaging tools to assess airway remodeling outcomes.
Introduction
Asthma affects an estimated 262 million people worldwide and caused over 461,000 deaths in 2019 (Vos et al., 2020). The disease is characterized by chronic airway inflammation, bronchial hyperresponsiveness (which form the targets of all pharmacological asthma therapeutics), and airway remodeling which involves all tissues of the airway wall (Holgate, 2008). Longitudinal studies have shown that current treatments for asthma (inhaled bronchodilators and corticosteroids) can reduce the frequency of exacerbations, but do not modify disease outcomes over time (Phelan et al., 2002; Sears et al., 2003). While patients can take precautions to avoid asthma-trigger exposure, inevitably 75% of patients report having asthma flare-ups and up to 45% regularly have a hard time breathing with day-to-day activities (The Lung Association, 2016). In this review, we emphasize the importance of studying the structural changes that occur with airway remodeling in asthma and how they relate to disease outcomes. We examine new evidence that has been generated using current ex vivo and in vivo imaging techniques to understand how heterogeneous airway remodeling influences airway closure and patient outcomes. Lastly, we review current therapeutics and their effect on features of airway remodeling.
Asthma phenotypes
To further understand asthma pathology, the field has focused on clinical asthma phenotypes which have been generated from recognizable clusters of demographic, clinical, or pathophysiological characteristics of patients with asthma. Some of the most common asthma phenotypes are early-onset or late-onset allergic asthma, non-allergic asthma, smoking-related, obesity-related, or aspirin-exacerbated respiratory disease (AERD) (Kuruvilla et al., 2019). Most recently with immune profiling, the following Th2-high and Th2-low asthma endotypes have also been proposed. Within the Th2-high asthma endotype, there is higher airway eosinophilia, which is a good predictor of the patient’s responsiveness to inhaled corticosteroids (ten Brinke et al., 2004). Although it is clear that there is a strong relationship between Th2 cytokine levels, eosinophilic inflammation and asthma, the inflammatory response of the asthmatic individual is heterogeneous and not all individuals with asthma have eosinophilic inflammation. More recently, a systematic review showed that approximately 50% of asthma cases are Th2-low asthma, driven by Th1 and Th17 cells and neutrophilic inflammation (Douwes et al., 2002; Vock et al., 2010; Kuruvilla et al., 2019). Th2-low asthma is less sensitive or resistant to corticosteroid treatment due to the dysregulation of glucocorticoid receptors (Raundhal et al., 2015; Banuelos et al., 2017; Ramakrishnan et al., 2019; Al Heialy et al., 2020; Hong et al., 2022).
To date, endotyping the inflammatory profile within a patient with asthma has been important clinically to understand which patients will be the most likely to respond to inhaled corticosteroids or who will benefit from the treatment with biologics. However, independent of the asthma phenotype or endotype, the underlying pathologies of asthma are chronic inflammation, reversible bronchoconstriction, and airway remodeling. In asthma, airway remodeling of the conducting airway walls results in fixed airflow obstruction, but airway remodeling also significantly contributes to airway closure during bronchoconstriction during an acute asthmatic episode (Kuwano et al., 1993). Several longitudinal studies that have followed children to adulthood have shown that with increasing severity of the disease, fixed airflow obstruction is a persistent feature of the disease (Strachan et al., 1996; Phelan et al., 2002; Sears et al., 2003). Further, despite the use of inhaled corticosteroids and bronchodilators, it has been shown longitudinally that there is no improvement in fixed airflow obstruction over time if present in childhood (Strachan et al., 1996; Phelan et al., 2002; Sears et al., 2003). Thus, the goal of the current review is to understand what is known about the heterogeneity of airway remodeling in asthma and how this contributes to the disease process.
Airway remodeling
Airway remodeling was first described in cases of fatal asthma by Huber and Koessler (1922). Airway remodeling in asthma refers to the structural changes that occur in both the large and small conducting airways and include loss of epithelial integrity, goblet cell and submucosal gland enlargement, basement membrane thickening, subepithelial fibrosis, increased smooth muscle mass, angiogenesis, and decreased cartilage integrity as highlighted in Figure 1 (Huber and Koessler, 1922; Haraguchi et al., 1999; Noble et al., 2002). Since then, features of airway remodeling have been documented for all stages of asthma severity and have been linked to reduced lung function, airway hyperresponsiveness, and the greater use of asthma medications (Holgate, 2008; Mostaço-Guidolin et al., 2019; Osei et al., 2020). The vast majority of what we understand about airway remodeling in asthma is derived from cross-sectional biopsy studies of the large conducting airways or post-mortem pathology on adult fatal asthmatics (Laitinen et al., 1985; Li and Wilson, 1997; Wilson and Li, 1997; Barbato et al., 2003; Payne et al., 2003; Tsartsali et al., 2011). It was previously proposed that chronic Th2 inflammation leads to a chronic cycle of injury resulting in airway remodeling over the lifetime of an individual with asthma (Mosmann et al., 1986; Le Gros et al., 1990; Metcalfe et al., 1997). However, recent studies have now shown that airway remodeling is not present at birth (Tsartsali et al., 2011), but features of remodeling are present by the age of 2–4 years of life, often before atopic inflammation is observed or a clinical diagnosis of asthma is made (Dunnill, 1960; Roche et al., 1989). Further, a systematic review of 39 studies examining the relationship between inflammation and airway remodeling concluded “Failure to demonstrate eosinophilic inflammation in children in the absence of airway remodeling is contrary to the hypothesis that inflammation causes these changes” (Castro-Rodriguez et al., 2018). Lastly, assuming airway remodeling is not reversible as is seen in rodent models, one would assume that airway remodeling would increase over time with persistent inflammation. However, Broekema et al. demonstrated in a large cohort of adult asthmatics with active asthma or a history of asthma, that independent of asthma status and medication use, the extent of airway remodeling and fixed airflow obstruction did not change over a 3-year time period (Broekema et al., 2011). Thus, the hypothesis that airway remodeling occurs over the lifetime of an asthmatic individual into adulthood in response to inflammation does not appear to be true. With that in mind, below we review the current knowledge of each airway remodeling feature associated with asthma.
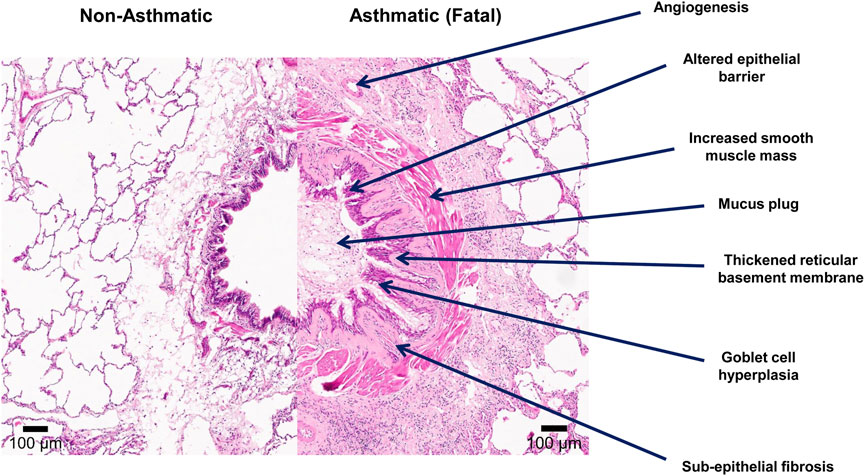
FIGURE 1. Features of airway remodeling in asthma. Lung tissue was formalin-fixed paraffin-embedded (FFPE) and stained with Hematoxylin and Eosin stains. The left image in the panel demonstrates a conducting airway from a non-asthmatic individual (11 years) with no history of respiratory disease. The right image demonstrates a conducting airway of a fatal asthmatic individual (15 years) showing features of airway remodeling including: an altered epithelial barrier with goblet cell hyperplasia, angiogenesis, increased smooth muscle mass, thickened reticular basement membrane, sub-epithelial fibrosis, and mucus plugging of the airway lumen.
Extracellular matrix remodeling
Abnormal thickening of the reticular basement membrane with increased deposition of extracellular matrix (ECM) proteins, fibronectin, collagen types I, III, and V, hyaluronan, laminin α2/β2, tenascin, and versican within the lamina reticularis is one of the hallmarks of airway remodeling in asthma and has been observed in both children and adults with mild to severe and fatal asthma in several histological studies (Roberts, 1995; Laitinen et al., 1997; Elias et al., 1999; Zeiger et al., 1999; Boulet et al., 2000; James et al., 2012a; Burgess et al., 2016). Several clinical studies have demonstrated that structural changes associated with reticular basement membrane thickening, correlate with airway hyperresponsiveness, suggesting abnormal ECM deposition is a fundamental abnormality involved in the pathogenesis of asthma (Ward et al., 2002; Tsurikisawa et al., 2010). More recently, Mostaço-Guidolin et al., using an automated unbiased approach involving the combination of colour segmentation to isolate airway wall features and then uniformly measuring the thickness distribution of each structure using Euclidean Distance Mapping, highlighted that not only is the reticular basement membrane thickened but, that there is greater variance or heterogeneity in the thickening of the reticular basement membrane (Mostaco-Guidolin et al., 2017). Specifically, the study highlighted the heterogeneity of reticular basement membrane remodeling both within a single airway wall and between patients, demonstrating airway remodeling is a dynamic process occurring at different levels or rates with any given airway within the lung (Mostaco-Guidolin et al., 2017). The study further showed that the basement membrane thickening and its heterogeneity in variance were not different between fatal and non-fatal asthmatics, irrespective of age and biological sex.
Increased deposition of collagen I, collagen III, and fibronectin have also been demonstrated in the lamina propria of patients with asthma (Roberts, 1995). Fibroblasts are the primary producer of ECM within the lung, and an increase in myofibroblast number within the lamina propria of conducting airways has been reported in asthmatic patients (Carroll et al., 2000; Boser et al., 2017). Myofibroblasts are ECM synthetic cells and have been suggested to be the cause of increased collagen I, collagen III, and fibronectin within the conducting airways of patients with asthma (James, 2005; Michalik et al., 2018). More recently, using second harmonic imaging, Mostaço-Guidolin et al. showed that the fibrillar collagen deposited within the lamina propria is not only overproduced but is highly disorganized and fragmented in both the large and small conducting airways in children and adults with asthma (Mostaço-Guidolin et al., 2019). The same study also showed that airway fibroblasts from asthmatic patients are defective at producing decorin, an important proteoglycan involved in collagen formation (Mostaço-Guidolin et al., 2019). As fragmented defective collagen can induce myofibroblast formation and stimulate increased ECM synthesis, it has been proposed that the lack of decorin production and disorganized fibrillar collagen formation may induce a feedback loop where fibroblasts are continually stimulated to produce more collagen resulting in a higher level of disorganized collagen and airway remodeling (Mostaço-Guidolin et al., 2019; Snelgrove and Patel, 2019).
Lastly, respiratory viruses may also play a role in increased ECM remodeling in asthma. Rhinovirus (RV) infection of airway smooth muscle cells increases the deposition of ECM proteins fibronectin, perlecan, and collagen IV (Kuo et al., 2011). Further, RV-induced ECM production also induced greater migration rates of airway smooth muscle on the ECM, suggesting that respiratory viruses can induce increased ECM deposition and increased airway smooth muscle mass from increased cell migration.
In summary, basement membrane thickening is an early and universal feature of airway wall remodeling in asthma. It has been proposed that basement membrane thickening may lead to loss of epithelial-fibroblast cross-talk leading to subepithelial fibrosis. Indeed Osei et al. have shown that epithelial-derived interleukin (IL)-1α is an important mediator in epithelial-fibroblast cross-talk and reduces inflammatory cytokine release and ECM production in healthy lung-derived fibroblasts (Osei et al., 2020). Whether the diffusion and activation of epithelial-derived factors such as IL-1α are altered due to ECM accumulation within the basement membrane in the asthmatic lung is still to be determined.
Airway epithelial remodeling
There is now strong evidence within the literature that impairment of epithelial barrier function in asthma is a key player in the disease pathogenesis through the initiation of airway inflammation and remodeling via the release of alarmins (e.g., TSLP, IL-25 and IL-33 expression) (Borish and Steinke, 2011; West et al., 2012; Paplińska-Goryca et al., 2018; Chan et al., 2019). Indeed gene variants in TSLP and IL33 are associated with increased expression of these epithelial alarmins (Gudbjartsson et al., 2009; He et al., 2009; Ferreira et al., 2011; Harada et al., 2011; Li et al., 2015; Shrine et al., 2019), and ex vivo studies have demonstrated higher expression levels of TSLP and IL-33 in airway epithelial cells derived from asthma patients cultured at air-liquid-interface (ALI) compared to healthy controls (Hackett et al., 2013). In response to environmental insults such as respiratory syncytial virus (RSV), particulate matter (PM) 10, or mechanical wounding, the airway epithelium of asthmatic patients has also been shown to be more sensitive compared to healthy controls, releasing greater levels of inflammatory cytokines including IL-6, IL-8 and granulocyte-macrophage colony-stimulating factor (GM-CSF), which are also known to be airway smooth muscle mitogens (Johnson et al., 2004; Hackett et al., 2011). Whereas, in response to rhinovirus infection, the bronchial epithelium of asthmatic patients has been shown to have a deficient innate immune response including decreased interferon-β expression, impaired apoptosis, and increased virus replication (Wark et al., 2005).
In situ studies have also revealed many structural changes within the airway epithelium of asthmatic patients, including disruption of tight junctions and adherens junctions, detachment of ciliated cells, increased numbers of goblet cells, basal cells (CK5+/p63+), and side population progenitor cells (Hackett and Knight, 2007; de Boer et al., 2008; Hackett et al., 2008; Knight et al., 2011; Xiao et al., 2011). In line with these findings, functional studies of airway epithelial cells derived from children and adults with asthma cultured at ALI have also demonstrated decreased expression of tight junction (increased permeability) and adherens junction molecules (e.g., E-cadherin, caveolin-1), and increased numbers of basal cells (Hackett et al., 2009; Hackett et al., 2011; Xiao et al., 2011). It has been shown that infections with respiratory viruses, such as RSV, disrupt epithelial tight junctions by activation of protein kinase D (Rezaee et al., 2013). Studies focused on RV infection have shown infection causes loss of tight junctions (ZO-1) in ALI-cultured airway epithelial cells from asthmatic children, which is more pronounced and sustained compared to airway epithelial cells from children with no asthma (Looi et al., 2016; Looi et al., 2018). A reduction in occludin expression in an NADPH-oxidase-dependent manner has also been implicated in RV infection, inducing barrier dysfunction (Comstock et al., 2011). In addition, RV infection in asthmatic subjects leads to less mitochondrial respiration compared to healthy control subjects, indicating that patients with asthma may have a less efficient immune response to virus infection (Huang et al., 2022). Disruption of airway epithelial barrier function in asthma is further supported by several genome-wide association studies (GWAS) that have found asthma risk genes and genetic loci associated with asthma (Cookson, 2004; Moheimani et al., 2016) that are involved in cell adhesion and airway epithelial barrier function such as PCDH1 (protocadherin-1) (Koppelman et al., 2009), ORMDL3 (orosomucoid-like 3) (Moffatt et al., 2007; Moffatt, 2008; Moffatt et al., 2010), DPP10 (dipeptidyl peptidase 10) (Allen et al., 2003), GPRA (G protein-coupled receptor for asthma susceptibility) (Laitinen et al., 2004) and CDHR3 (cadherin-related family member 3) (Bønnelykke et al., 2014; Basnet et al., 2019). CDHR3, which has been shown to be involved in cell adhesion and polarity, is the receptor for rhinovirus C, thus variants of this gene can modulate the susceptibility to infection (Bønnelykke et al., 2014; Li et al., 2015; Basnet et al., 2019). Although the mechanisms contributing to the loss of airway epithelial barrier function in asthma have not been fully elucidated, the thickening of the epithelium with increased numbers of basal cells may be a remodeling mechanism to support the attachment of the airway epithelium. This is supported by the early work of Evans and Plopper, who demonstrated that the reticular basement membrane is essential for anchoring the basal epithelial cells via hemidesmosomes and that within the conducting airways the height of the reticular basement membrane directly correlates with the height of the airway epithelium and number of basal cells (Evans and Plopper, 1988; Tsartsali et al., 2011). It is therefore not surprising that when measuring the thickness of the reticular basement membrane in asthma, Mostaço-Guidolin et al. showed that airway epithelial thickness directly correlates with reticular basement membrane thickness and that the two show great intra- and inter-subject heterogeneity (Mostaco-Guidolin et al., 2017).
Goblet cell hyperplasia (increased number of cells), metaplasia (change in cell phenotype) and mucus accumulation have been consistently reported in the conducting airways of patients with mild, moderate, severe, and fatal asthma (Kuyper et al., 2003; Wenzel et al., 2012; Malmström et al., 2017; Trejo Bittar et al., 2017; Elliot et al., 2018). In the epithelium of asthmatic patients, goblet cell metaplasia is the main contributor to increased numbers of goblet cells in the small airways rather than goblet cell hyperplasia (Curran and Cohn, 2010). Though there are many proposed mechanisms of goblet cell metaplasia, the main suggested mechanism is through trans-differentiation of club and ciliated cells into goblet cells, rather than the proliferation of pre-existing goblet cells (Lambrecht and Hammad, 2012). The second mechanism is through increased expression of inflammatory mediators which initiate goblet cell metaplasia (Curran and Cohn, 2010). Specifically, epidermal growth factor receptor (EGFR) and IL-13 induce both club and ciliated cells to transition into goblet cells through the transcription factors FoxA2, TTF-1, SPDEF, and GABAAR (deFelice et al., 2003; Whiting, 2003; Wan et al., 2004; Park et al., 2007; Xiang et al., 2007). It has also been proposed that goblet cell hyperplasia, metaplasia, and subsequent mucus over-production may result through epithelial compression during bronchoconstriction and airway hyperresponsiveness (Grainge et al., 2011).
Mucins secreted by goblet cells in the airway epithelium and submucosal glands are important for maintaining lung homeostasis by trapping particulate matter and pathogens, which are then removed from the lung via mucociliary clearance (Symmes et al., 2018). The primary mucins in the human airways are MUC5B and MUC5AC which are secreted throughout the small and large conducting airways, with MUC5B being the dominant secretory mucin in the healthy lung (Okuda et al., 2019). Increased MUC5AC expression has been observed by a number of asthma studies and mucus plugs have been shown to contribute to airflow obstruction in the conducting airways (Bonser and Erle, 2017). MUC5AC and MUC5B gene variants are also predicted to cause increased mucin production in asthma (Rousseau et al., 2007; Shrine et al., 2019). It has been hypothesized that the alterations in mucus composition and organization through changes in mucin gene expression as well as an increase in the number of goblet cells, can result in impaired mucus expectoration due to MUC5AC tethering and increased gel viscoelasticity (Bonser and Erle, 2017).
In summary, these studies have demonstrated that genetic defects or variations within the airway epithelium can cause, drive, or worsen asthma, leading to a defective epithelial barrier that can drive the disease process in response to interactions with the inhaled environment.
Angiogenesis
In 1960, Dunnill et al. first reported on bronchial artery dilation in lung tissue from 20 fatal asthmatic patients (Dunnill, 1960). Since then, it has been documented that an increased density of the bronchial vasculature (Vrugt et al., 2000; Wilson and Robertson, 2002; Tanaka et al., 2003; Barbato et al., 2006) leads to increased blood flow in both patients with asthma and animal models of the disease (Wanner et al., 1990; Csete et al., 1991; Kumar et al., 1998; Mendes et al., 2004), which further correlates with airflow limitation (Li and Wilson, 1997; Orsida et al., 1999; Vrugt et al., 2000), bronchial hyperresponsiveness (Orsida et al., 1999; Kanazawa et al., 2002a; Kanazawa et al., 2002b), and asthma severity (Hoshino et al., 2001a; Hashimoto et al., 2005; Chetta et al., 2007; Grigoraş et al., 2012). It has been proposed that the increased bronchial arterial angiogenesis in the airways of patients with asthma may promote the extravasation of inflammatory cells, the release of inflammatory mediators, and abnormal cell growth and proliferation leading to asthma pathology. More recently, the distal pulmonary arteries and veins of pediatric and adult asthma patients have also been shown to be remodeled with thickened walls resulting from increased deposition of disorganized, fragmented, and thicker collagen fibers, in comparison with control lungs (Mostaço-Guidolin et al., 2021). These data highlight that remodeling of the pulmonary vasculature occurs throughout the lung in asthma and is not restricted to the conducting airways which are the primary site of airway hyperresponsiveness and bronchoconstriction. Further, the deposition of disorganized and fragmented collagen fibers is a key feature of lung remodeling that occurs early in life, regardless of sex or disease severity to both the wall of conducting airways and the pulmonary vasculature.
In summary, future work is needed to understand the role of pulmonary vasculature remodeling in asthma and if it is a driver of the disease process or consequence.
Airway smooth muscle mass
Airway smooth muscle (ASM) hyperresponsiveness is a key feature of acute airflow limitation in asthma and its mechanisms of contraction and relaxation have been a subject of great investigation (Doeing and Solway, 2013; Wang et al., 2020). Increased ASM mass is a hallmark feature of airway wall remodeling in asthma which contributes to the narrowing of the airways and amplifies the effect of smooth muscle shortening (James et al., 1989). In 1993, Carroll et al. showed that the thickness of the ASM layer is increased in both large and small airways in patients with asthma, and there is more ASM mass with increasing disease severity (Carroll et al., 1993). In asthma, expansion of the ASM layer occurs through ASM cell hypertrophy (increased cell size), and hyperplasia (increased cell number) (Ebina et al., 1993; Araujo et al., 2008; James et al., 2012b). It has been proposed that hypertrophy and hyperplasia may be induced by the activation of elevated cytokines in the asthmatic lung, including TGF-β1, EGF, platelet-derived growth factor (PDGF) (Hirst et al., 1996; Panettieri et al., 1996; Cohen et al., 2000; Chen and Khalil, 2006). Additionally, ASM cells derived from asthmatic patients in vitro have been shown to have a significantly higher rate of proliferation compared to ASM cells derived from healthy individuals, which was shown to occur due to the dysregulation of C/EBPα, a transcription factor responsible for inhibition of proliferation through the glucocorticoid receptor (Johnson et al., 2001; Roth et al., 2004). Further, ASM cells from asthmatic patients have increased mitochondrial biogenesis and increased fatty acid consumption, which is thought to drive increased ASM cell proliferation in asthma (Esteves et al., 2021). These changes in ASM mass lead to mechanical changes in the cells ability to relax, contract, and lengthen, which play important roles in luminal narrowing and airflow restriction (Seow et al., 1998; Doeing and Solway, 2013). Lambert and Wiggs established that ASM contraction and consequently, airway narrowing can result in airway mucosal folding (Lambert, 1991; Lambert et al., 1994; Wiggs et al., 1997). This not only provides significant airway stiffness but also significant load against the ASM contraction, particularly in conjunction with the thickening of the airway wall and alterations in ECM deposition (Lambert, 1991; Lambert et al., 1994; Wiggs et al., 1997). During inspiration, radial tethering of the alveolar walls stretches the conducting airways and vessels open. However, in asthma, increased ASM mass causes the ASM to shorten excessively against the elastic loads provided by the lung parenchyma, parallel airways and vessels, and thus results in mucosal folding (Seow et al., 1998). Johnson et al. showed that when human airway epithelial cells are mechanically compressed, mimicking bronchoconstriction, this results in the secretion of ASM cell mitogens which include IL-6, IL-8, monocyte chemoattractant protein-1, and matrix metalloproteinase (MMP)-9 (Johnson et al., 2004). The deformed epithelium also released endothelin-1, which can augment both the basal tone and histamine-induced contraction of ASM cells. Moreover, it has been shown that repeated airway bronchoconstriction leads to higher expression of epithelium-generated TGF-β, which may lead to thickening of the sub-epithelial collagen layer (Grainge et al., 2011). This suggests that bronchoconstriction due to ASM contraction plays a role in inducing basement membrane thickening (Noble et al., 2014). Together, these studies suggest that independent of allergic inflammation, ASM bronchoconstriction and deformation of the airway epithelium may be sufficient for inducing features of airway wall remodeling and asthma progression (Camoretti-Mercado and Lockey, 2021).
It has been previously shown that placing a load on healthy airways stretches the ASM more than the optimal length, causing them to produce less contractile force (Seow et al., 1998). However, as we now know that airways from asthmatic subjects produce significantly greater force, solely an increased amount in ASM mass cannot be responsible for the amount of force produced, which suggests there may be alterations in either the contractile ability of myocytes or myocyte interactions (Brusasco et al., 1998; Seow et al., 1998). Chin et al. demonstrated that the mechanical properties of human tracheal ASM from asthmatic and non-asthmatic subjects were comparable except for increased passive stiffness and attenuated decline in force generation after an oscillatory perturbation (Chin et al., 2012). The authors concluded that these findings may explain the reduced bronchoconstriction induced after a deep inspiration in asthmatic subjects. More recently Ijpma et al. have shown that human ASM from asthma subjects is hyperreactive only in intrapulmonary airways and not in the trachea, suggesting ASM hypersensitivity is dependent on the airway generation within the lung (Ijpma et al., 2020).
More recently the role of ASM in asthma pathology has been shown to extend beyond bronchoconstriction. There is now compelling evidence that ASM is involved in airway inflammation through the release of inflammatory chemokines IL-1β, TNFα or immunomodulators including IL-5, IL-13, TGF-β (Hakonarson et al., 1999; Coutts et al., 2001; Moynihan et al., 2008). ASM additionally releases eotaxin, increasing recruitment of eosinophils, and immune cells such as T-lymphocytes and mass cells have been shown to infiltrate and accumulate within the ASM layer (Ammit et al., 1997; Ghaffar et al., 1999; Moore et al., 2002; Berger et al., 2005; Ramos-Barbón et al., 2010). ASM can also regulate ECM composition through the secretion of matrix metalloproteinase (MMP)-9 and MMP-12 (Araujo et al., 2008). Further, ASM cells have been shown to secrete more collagen I and perlecan, and have decreased laminin α1 and collagen IV expression (Johnson et al., 2004). Additionally, Esteves et al. have recently shown that ASM from asthmatic patients increases RV replication in the epithelium after infection by secreting CCL20, inhibiting the epithelial protein kinase RNA-activated antiviral pathway (Esteves et al., 2022).
In summary, the contribution of ASM in asthma is multifactorial and beyond the effects of bronchoconstriction alone. ASM contraction not only alters the mechanical properties of the airway wall but also contributes to airway remodeling which influences other structural cells of the airway (epithelial, fibroblasts and vasculature) and immune modulation (inflammatory cells).
Heterogeneity of lung ventilation and airway remodeling
While the histological studies described above have provided extensive knowledge on different aspects of airway remodeling in asthma, they have relied on biopsy sampling or autopsy lungs which have several limitations to note. Biopsy samples are limited in the airway wall structures that can be sampled which are primarily the epithelium, basement membrane and laminar reticularis, and the tissue artefacts that can be induced when crunched by the biopsy forceps. Additionally, lung biopsies primarily allow for the larger airways to be sampled and it is the branch point of two daughter airway generations that is sampled which can make sampling of the airway wall very difficult. Lastly, it is very difficult to sample multiple airway generations to assess the heterogeneity of airway remodeling within the lung of asthmatic patients. Autopsy studies on lungs from fatal asthmatics offer a unique opportunity to assess the heterogeneity of airway remodeling within multiple airway generations and across lung height, however, it does not provide the opportunity to assess the spectrum of the asthma syndrome specifically mild and moderate asthma. Below we describe the various approaches that have been used to assess the heterogeneity of airway remodeling and its effects on lung function. The inherent heterogeneity of lung ventilation has long been of great interest, dating back to 1956 when the uneven distribution of ventilation was hypothesized to influence the mechanical properties of the dynamic lung (Otis et al., 1956). This hypothesis was first explored using rat and canine models, which revealed that exposure to methacholine or histamine resulted in bronchial responsiveness that was heterogeneous in individual airways (Ludwig et al., 1987; Saldiva et al., 1992). This finding of heterogenous airway responsiveness was confirmed in human lung explants in 1997 by Minshall et al. who used agarose-filled lungs that were cross-sectioned and stimulated with methacholine (Minshall et al., 1997). To understand ventilation heterogeneity, many computational models have since been developed but many have assumed a simplified airway tree structure that did not provide an accurate model of the human lung (Neelakantan et al., 2022). In 1997, Bates and Thorpe developed a computational model which accounted for asymmetric branching patterns based on Horsfield and Cummings’s data (Horsfield and Cumming, 1968) on the branching of the human lung and stochastic heterogeneity (Thorpe and Bates, 1997). This model demonstrated that random heterogeneous airway narrowing in a human lung can lead to increased lung impedance (Kuwano et al., 1993). Though heterogeneous bronchoconstriction throughout the airway tree was well-established, the question remained if this occurs to the same or greater magnitude in diseased lungs. To answer this question, in 1999 Gillis and Lutchen used a computational lung model to show that when the same heterogeneous airway smooth muscle shortening is applied to a healthy lung and asthmatic lung, the healthy lung experiences mild changes but in the asthmatic lung, this would lead to greater changes in lung resistance (Gillis and Lutchen, 1999). This work demonstrated that airways within the lung of a patient with asthma are predisposed to heterogeneous bronchoconstriction. These data highlight that in asthma, the disease process is not occurring uniformly across the lung and may have spatial and temporal differences. Importantly, it has also been shown that in asthma, baseline ventilation heterogeneity of the conducting airways can predict airway hyperresponsiveness, independent of airway inflammation (Downie et al., 2007). More recently, Pascoe et al. demonstrated heterogeneous remodeling of randomly selected small airways within the lungs patients with fatal and non-fatal asthma compared to donor control lungs (Pascoe et al., 2017). Using their histological measurements of airway remodeling in a computational model of airway narrowing, they showed that heterogeneous airway remodeling can lead to increased airway closure (Pascoe et al., 2017). Direct comparisons have also been made between daughter airways bifurcating from a common parent airway and shown great heterogeneity in parallel daughter airways in response to inhaled methacholine (Carroll et al., 2015). These findings have led to the hypothesis that an individual airway’s remodeling phenotype is determined by its own mechanical and biological properties and is less influenced by its surrounding environment (Carroll et al., 2015). However more recently, Pascoe et al. have developed a mathematical model to demonstrate that remodeled airways are spatially correlated, which may be caused by cycles of bronchoconstriction and mechanotransduction (Pascoe et al., 2020). The study further confirmed this finding in human subjects with and without asthma (Pascoe et al., 2020). These results demonstrate the importance of understanding heterogeneous airway remodeling and its spatial distribution which may be responsible for ventilation heterogeneities in asthma.
In Vivo imaging
The use of computed tomography (CT) scanning and magnetic resonance imaging (MRI) has enabled the 3-dimensional (3D) assessment of the lung structure in vivo in patients with asthma. This has overcome many limitations of histological studies which could only be conducted on lungs from fatal asthma patients, and the lack of airway wall structures sampled in an airway wall biopsy sample. Over the past three decades, numerous studies have measured airway remodeling and air trapping in patients with asthma using both qualitative, semi-quantitative, and quantitative CT/MRI techniques. Gupta and colleagues were the first to describe increased bronchial wall thickening in patients with severe asthma (n = 185) using CT (Gupta et al., 2009). Later, their group and other research groups, showed using quantitative measures including luminal volume and airway wall volume, that the large airways (measurements able to be obtained up to 5–6th generation of airways) (Brillet et al., 2007; Gupta et al., 2014; Grenier et al., 2016) are not only remodeled in patients with severe disease but are also remodeled in patients with mild/moderate asthma (Niimi et al., 2000; Brillet et al., 2013). Prior research has also demonstrated using CT that following methacholine-induced bronchoconstriction, the large conducting airways (>2 mm diameter) narrow more heterogeneously and have a larger decrease in airway lumen area in asthmatic patients compared to control patients (King et al., 2004). CT imaging has also enabled the assessment of disease progression over time within patients. In a longitudinal study, Witt et al. showed that severe asthma subjects have a greater decline in postbronchodilator FEV1% over time compared to those with mild-to-moderate asthma, which inversely correlated with significantly increased wall area percent and wall thickness percent in multiple airway generations, demonstrating the association between lung function loss and airway remodeling over time (Witt et al., 2014). This finding was also confirmed by Awadh et al. who showed that the wall thickness of large conducting airways is associated with lung function loss and disease severity (Awadh et al., 1998).
Several studies have also tried to correlate measures of large airway wall remodeling on CT with histopathological changes within the airway wall. Aysola et al. demonstrated that airway wall thickness at the third generation, correlated with increased epithelial and reticular basement membrane thickness on histology (Aysola et al., 2008). However, Kaminska et al. showed that airway wall thickness did not significantly correlate with the histopathological features of remodeling, including epithelial detachment, airway smooth muscle area, basement membrane thickness, and submucosal fibrosis, observed on biopsy (Kaminska et al., 2009). These findings indicate that there may be large variability in the degree of airway remodeling amongst patients with asthma that is detectable by CT imaging. Indeed, both studies showed variability in airway morphology between airways in the same patients and within-subject groups, which further highlights the need for assessment of the heterogeneity of airway wall remodeling in asthma.
In Vivo imaging of ventilation defects in asthma
Several studies have used expiratory CT scans to analyze areas of low attenuation to identify areas of air trapping in asthmatic subjects (Newman et al., 1994; Stern and Frank, 1994). Air trapping is the retention of excess air within the lung after expiration, usually in distal parts of the lung, which often correlates with airflow limitation (Arakawa et al., 1998). Several methods have been used to quantify gas trapping including the ratio between mean inspiratory and expiratory lung attenuation (E/I) (Gono et al., 2003), lung density < 900 Houndsfield units (HU) at full expiration (Newman et al., 1994), lung density < −900 HU at full expiration (Busacker et al., 2009), and the difference between inspiratory and expiratory attenuation (Tunon-de-Lara et al., 2007). Using image registration, on lung CT scans at multiple volumes, Choi et al. have shown that severe asthmatic subjects not only have reduced air volume, but that air trapping occurs in the lower lobes more compared to the upper lobes (Choi et al., 2013). Using expiratory CT lung scans from the Severe Asthma Research Program (SARP) cohort, Busacker et al. performed a multivariate analysis demonstrating that subjects with air trapping were more likely to have a history of asthma-induced hospitalizations, and longer disease duration, suggesting that air trapping can identify individuals with high risk for a severe disease phenotype (Busacker et al., 2009). Further, Gono et al. have demonstrated that asthmatic subjects with persistent lung function loss, have greater ratios of airway wall thickness to total diameter and percentage wall area of the right bronchus, which correlated with more air-trapping compared to the asthmatic subjects with normal spirometry after bronchodilator inhalation (Gono et al., 2003). Together these studies suggest that increased airway remodeling contributes to air trapping.
MRI with hyperpolarized gas such as Helium or Xenon has been used as a non-invasive technology to provide regional information on ventilation distribution within the human lung (Albert et al., 1994; Bachert et al., 1996; Kern and Vogel-Claussen, 2018). In asthma, MRI has shown that ventilation defects persist in the same location of the lung with repeated bronchoconstriction, demonstrating that areas of airflow obstruction tend to re-occur in the same airways (de Lange et al., 2007). De Lange et al. have also shown that patients with severe asthma have greater ventilation defects detected on MRI compared to those with mild to moderate asthma (de Lange et al., 2007). Interestingly, MRI has revealed ventilation defects exist even in subjects who have asymptomatic asthma with normal spirometry (Altes et al., 2001). This suggests that airflow obstruction and air trapping are occurring at a level that cannot yet be detected by spirometry. Altes et al. have shown that lung ventilation defects observed using MRI correlated with several clinical features of asthma including severity, medication use, decreased lung function (FEV1/FVC), and blood eosinophils (Altes et al., 2016). CT imaging is often used in conjunction with MRI to assess the lung structure in relation to the functionality of the lung. More recently Eddy et al. have combined MRI and CT imaging to identify phenotypic clusters which may have different clinical outcomes as demonstrated in Figure 2A (Eddy et al., 2022). These studies have important implications for how in vivo imaging can be used to support treatment decisions. Importantly, the same ventilation defects that have been observed on hyperpolarized gas MRI images in asthma, correspond to the same regions of air trapping that can be identified on expiratory CT scans (Fain et al., 2008).
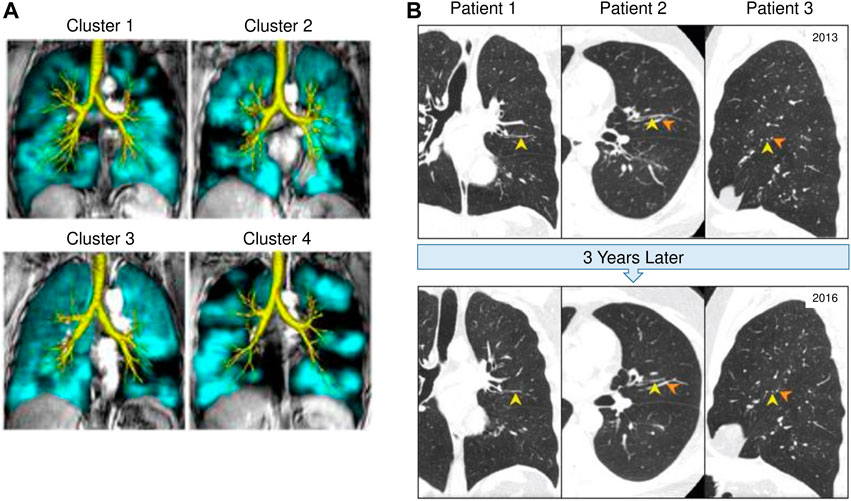
FIGURE 2. In vivo imaging of mucus plugging and ventilation defects in CT and MRI. (A) Lung computed tomography (CT) scans from three different asthma patients (A1, A2, and A3), which demonstrate the persistence of mucus plugs (Yellow arrowheads) in the same airway at baseline (2013) and 3 years later (2016). Orange arrowheads indicate blood vessels. This figure was adapted with permission from Figure 1 by Tang M et al. published in the American Journal of Respiratory and Critical Care Medicine 2022. (B) 129Xe magnetic resonance imaging (MRI) ventilation (teal) and computed tomography (CT) airway tree (yellow) for representative participants from four different asthma clusters. Cluster 1 has moderate heterogeneity as seen on MRI, moderate wall thickening and minimal luminal narrowing on CT. Clinically, cluster 1 has normal obstruction and no gas trapping. Cluster 2 has moderate MRI heterogeneity, significant wall thickening and minimal luminal narrowing. Clinically, this cluster pattern appears in females only, with moderate obstruction and gas trapping. Cluster 3 has moderate MRI heterogeneity with moderate wall thickening and significant luminal narrowing. Clinically, they tend to have more severe asthma and moderate obstruction with minimal gas trapping. Cluster 4 has significant MRI heterogeneity and moderate wall thickening with significant luminal narrowing. Clinically, cluster 4 patterning is male dominant with severe gas trapping and obstruction. Cluster analysis of asthma using MRI in combination with CT imaging may provide useful insight for asthma phenotyping and treatment decisions. This figure is adapted with permission from Figure 3B by Eddy R et al. published in Journal of Magnetic Resonance Imaging 2022. Copyright from RightsLink/John Wiley and Sons.
Mucus plugging
More recently CT has been used to visualize and quantify the presence of mucus plugs in asthmatic patients. In 2018, Dunican et al. analyzed CT scans of 146 adults with asthma and 22 healthy controls in the SARP cohort and established a method of scoring mucus plugging on CT scans based on a bronchopulmonary segment-based scoring system to quantify mucus plugging (Dunican et al., 2018). They showed that 67% of asthmatic subjects with FEV1 less than 60%, had a high mucus score (defined as mucus plugs present in more than four segments) which was also associated with increased sputum eosinophils and eosinophil peroxidase (EPO) levels (Dunican et al., 2018). Subsequently, a longitudinal study by Tang et al. assessed mucus plugs longitudinally using CT scans from the SARP cohort and found that 82% of subjects with mucus plugs at baseline still had mucus plugs at the third-year follow-up scan. Moreover, 65% of visible mucus plugs remained in the same airway segment as demonstrated in Figure 2B (Tang et al., 2022). These data demonstrate that chronic mucus plugs persist over time which emphasizes the need to clear mucus plugs to improve lung function in severe asthma patients (Tang et al., 2022). To evaluate the localization of mucus plugs, Yoshida et al. used a curved, multiplanar reconstruction (MPR) technique, a newly developed technique which provides greater accuracy and visualization than standard CT images (Yoshida et al., 2020). This study showed that when subjects were in the stable phase of asthma, the fourth and fifth-generation bronchi in the lower lobes had a greater frequency of mucus plugs. However, when subjects with asthma were undergoing an exacerbation, the upper lobes had a higher frequency of mucus plus in the fifth and sixth generation bronchi. In addition, Yoshida et al. showed that over 40% of conducting airways of the lower lobes were obstructed by mucus plugs (Yoshida et al., 2020). Lastly, a study involving 44 patients with severe asthma from the SARP cohort, assessed mucus plugging on CT scans, and showed that in the same bronchopulmonary segment where there was a mucus plug present, there were greater ventilation defects (Mummy et al., 2022). Together, these studies suggest that airway remodeling and mucus plugging are important causes of the heterogenous ventilation defects in asthma. It will be important to determine if remodeled airways are associated with mucus plugs and the key cells and mediators driving this process.
In summary, CT and MRI imaging are providing great insight into the heterogeneity of airway remodeling within the asthmatic lung and mucus plugs are chronic, persistent, and prevalent thus there is an urgent need to target mucus plugs to improve air trapping in asthma. Unfortunately, one remaining limitation of CT imaging apart from radiation exposure is the resolution to assess the airway tree at the cellular level to understand the disease pathology. The current resolution of CT (.85–1 mm in diameter) (Hasegawa et al., 2006; Kurashima et al., 2013; Kirby et al., 2018), depending on the radiation dose used does not enable the visualization of the smallest conducting airways (<2 mm in diameter) (McDonough et al., 2011), which are thought to contribute to the greatest impact to airway closure during bronchoconstriction and fixed airflow obstruction (airway remodeling) (Kurashima et al., 2013). More recently, microCT with a spatial resolution of up to 1 μm has enabled volumetric imaging and quantitative assessment of the small conducting airways, respiratory airways, and alveoli (Haefeli-Bleuer and Weibel, 1988; McDonough et al., 2011). To date, microCT has been applied to assess the morphometry of the normal human lung and how it is modified in the pathology of chronic obstructive pulmonary disease (COPD) (McDonough, 2012; Koo et al., 2018), lung cancer (Holbrook et al., 2021), Idiopathic Pulmonary Fibrosis (IPF) (Khalajzeyqami et al., 2022), cystic fibrosis (CF) (Wielpütz et al., 2011), and bronchiectasis (Wurnig et al., 2014). However one limitation of microCT is that it cannot be used in vivo in humans due to the small field of view and higher radiation dose. To date, research on asthma using microCT has been limited to murine models of asthma (Shofer et al., 2007; Lederlin et al., 2012; Paik et al., 2014). These studies have shown that it is possible to monitor airway remodeling (wall thickness, epithelial hyperplasia and smooth muscle hypertrophy) non-invasively in asthmatic mice which may be important for future drug testing. Future work using microCT to assess human samples will be important to bridge the resolution gap to understand the heterogeneity in airway remodeling in both the large and small airways and vessels within the asthmatic lung.
Treatment of airway remodeling
The current 2021 guidelines from the Global Initiative for Asthma (GINA) aim to control symptoms and minimize the risk of exacerbations and persistent airflow limitation (GINA Asthma, 2012). As per the 2021 GINA guidelines, the first line of recommended pharmacological treatments are inhaled low-dose corticosteroids (ICS) to suppress the inflammatory response within the airways, and bronchodilators to relax the smooth muscles during bronchoconstriction (Barnes, 2010). However, there are no current asthma pharmacological treatments that are used primarily to target airway remodeling (Berair and Brightling, 2014). Bronchial thermoplasty is a non-pharmacological approach that uses controlled thermal energy to reduce smooth muscle mass in severe uncontrolled asthma (Dombret et al., 2014), and thus is the only asthma therapy that directly targets airway remodeling. Recent follow-up studies have shown that ACQ scores and airway resistance can be sustained up to 10 years after treatment with bronchial thermoplasty, however, there were no significant changes in FEV1 and patients that undergo this procedure have an increased risk for bronchiectasis (Langton et al., 2018; Langton et al., 2020; Chaudhuri et al., 2021). This could be in part due to the treatment only targeting the large central airways, and not the small conducting airways which are the site of airway narrowing and closure in asthma (Donovan et al., 2018). Langton et al. also demonstrated that following bronchial thermoplasty treatment, not all airways were dilated uniformly (Langton et al., 2021). Thus, while bronchial thermoplasty treatment can be a useful approach for some patients with severe uncontrolled asthma, it has limitations to modify airway remodeling across the entire lung structure and the spectrum of disease severity and endotypes. Below we outline the clinical studies to date, which have investigated the effect of pharmacological treatment on features of airway remodeling.
Inhaled corticosteroids (ICS)
It was first shown in a double-blind randomized controlled trial, that 12 months of fluticasone propionate treatment decreases reticular basement membrane thickness, though there were no effects on collagen I and III deposition (Ward et al., 2002). ICS treatment has also been shown to decrease collagen type III subepithelial deposition in bronchial biopsies (Mattos et al., 2002). However, given that multiple other longitudinal studies show ICS treatment does not affect reticular basement membrane thickness, more research is needed to determine why there is heterogeneity in ECM remodeling to ICS treatment (Jeffery et al., 1992; Boulet et al., 2000; Bergeron et al., 2005). This will be particularly important as the new 2021 GINA guidelines for asthma recommend low-dose ICS along with the use of long-acting bronchodilators.
With regards to airway epithelial damage, Dorsheid et al. showed that treatment with dexamethasone, beclomethasone, budesonide, and triamcinolone on primary airway epithelial cells induced apoptosis (Dorscheid et al., 2001). Several studies have also demonstrated that when ciliated cells are exposed to ICS, their adhesion to the basal lamina is weakened, leading to epithelial shedding (Shebani et al., 2005; Trautmann et al., 2005; Uhlík et al., 2007). In a study investigating the effects of inhalation from a single dose of beclomethasone in a rabbit model, Uhlík et al. showed that the mucus release was accelerated from goblet cells and due to overstimulation they became degenerated and were eventually shed (Uhlík et al., 2007). However, there is some conflicting evidence of how epithelial shedding occurs or loss of barrier function may be induced by ICS use. Carayol et al. demonstrated that dexamethasone treatment was able to recover TNF-α induced reduction of adherens proteins E-cadherin, β and γ-catenin expression (Carayol et al., 2002). Further, Laitinen et al. showed that following 3 months of use of ICS, there was an increased number of ciliated cells and a reduction of goblet cells in biopsies from asthmatic patients (Laitinen et al., 1985). This indicates that the effect of ICS treatment on epithelial damage is time dependent. Indeed, in a longitudinal study looking at bronchial mucosal biopsies from before and after 10 years of ICS treatment, Lundgren et al. showed that though the number of inflammatory cells was reduced post-treatment, only partial recovery of epithelial damage was observed (Lundgren et al., 1988).
There are limited studies on how ICS use affects the pulmonary vasculature in asthma. Notably, Hoshino et al. showed that 6 months of treatment with inhaled beclomethasone dipropionate lead to a significant reduction in vessel number and vascularity within the lamina propria (Hoshino et al., 2001b). However, in a shorter study that lasted 6 weeks of both low-dose and high-dose inhaled fluticasone propionate, only patients administered a high dose of ICS had a decreased number of vessels and an overall vascular area within the airway wall (Chetta et al., 2003).
When assessing the effects of long-term use of ICS at high doses on airway remodeling, it is important to note the side effects of ICS which include impaired growth in children, decreased bone mineral density, and cataracts (Dahl, 2006). As well, longitudinal studies have demonstrated that patients with asthma who are taking ICS and/or bronchodilators continue to have fixed airflow obstruction (Phelan et al., 2002; Sears et al., 2003). Thus, while the use of ICS to manage airway inflammation will be important for sustained long-acting bronchodilator use ICS treatment of airway remodeling may not be feasible, and targeted therapeutics to modulate airway remodeling will be important to improve lung function.
Leukotriene modifiers
In asthma, leukotriene receptor antagonists including zafirlukast and montelukast have been shown to decrease inflammation, improve airway hyperresponsiveness and reduce exacerbations (Horwitz et al., 1998; Montuschi, 2010). Most of the research investigating how leukotriene modifiers modulate airway remodeling has been performed in animal models. In 1993, Wang et al. demonstrated, in ovalbumin (OVA)-sensitized rats, that leukotriene D4 decreased smooth muscle mass in the large airways, as well as airway hypersensitivity. In 2005, Henderson et al. showed that zileuton, a leukotriene synthesis inhibitor, was able to reduce smooth muscle mass, as well as decrease the thickness of the basement membrane and the number of blood vessels in OVA-sensitive mice (Chen et al., 2013). More recently, in OVA-sensitive mice, pranlukast, a leukotriene receptor antagonist, has been shown to decrease several features of airway remodeling, including goblet cell hyperplasia, and collagen deposition by inhibiting TGF-β signaling (Hur et al., 2018). However, the challenge is converting these observations in preclinical models to humans as many induced airway remodeling features are spontaneously reversible in murine models. Future work is required in preclinical human cell models or clinical trials to determine if leukotriene modifiers can be used to modify airway remodeling in asthmatic patients.
Biologics
Humanized antibodies, are immune-modulating drugs, also known as biologics, which are used to target Th2-high inflammation in severe asthma. Omalizumab, the first available humanized antibody for severe asthma inhibits IgE binding to the FcεR receptor present on eosinophils, mast cells, basophils, and dendritic cells (Kumar and Zito, 2022). In clinical trials, it has been shown that omalizumab can reduce total airway wall thickness, the thickness of the reticular basement membrane, and in particular, fibronectin deposition in severe asthmatic airways (Hoshino and Ohtawa, 2012; Riccio et al., 2012; Zastrzeżyńska et al., 2020). However, these reductions were modest with only a 5% decrease in the percent wall area. Mepolizumab is a humanized antibody that selectively binds to IL-5, the primary pro-inflammatory mediator responsible for eosinophilic formation and maturation, elevated in Th2-high asthma endotypes (Cada et al., 2016). Flood-Page et al. have shown that mepolizumab treatment in mild atopic asthmatics (n = 24) results in a reduction of tenascin and lumican, two reticular basement membrane proteins that are upregulated in asthma. The mechanism in which mepolizumab may be modulating the ECM is not clear, though it has been proposed to be due to the reduction of eosinophils producing TGF-β1, reducing myofibroblasts and ECM protein expression (Flood-Page et al., 2003). Benralizumab is another humanized antibody that also targets IL-5 activation (Pelaia et al., 2018). Chachi et al. used a novel computational modelling approach to predict the impact of benralizumab based on eosinophilic count from bronchial biopsies, and predicted using their virtual patient model that benralizumab may reduce ASM however no in vivo data exist (Chachi et al., 2019).
Macrolides
Macrolides are a class of antibiotics that have anti-bacterial, anti-viral, and anti-inflammatory properties which can reduce exacerbations in severe asthma, typically in oral formulation (Brusselle et al., 2013; Wang et al., 2020). Azithromycin is a macrolide that has been shown to reduce ASM cell viability, leading to apoptotic cell death and reduced proliferation (Stamatiou et al., 2009; Stamatiou et al., 2010). In the past few years, it has been shown in both naïve and allergic mice models, azithromycin not only decreases airway remodeling but also ASM thickness in both proximal and distal airways (Kang et al., 2016; Donovan et al., 2020). Another macrolide that has been explored is roxithromycin, which has an autophagic effect on neutrophils and suppresses the production of IL-8, IL-6, and GM-CSF in airway epithelial cells (Kawasaki et al., 1998). Additionally, roxithromycin has been shown to inhibit ASM cell proliferation by suppressing vascular endothelial growth factor (VEGF), and extracellular signal-regulated kinase (ERK) (Pei et al., 2016). In 1994, Shimizu et al. assessed the effectiveness of roxithromycin for improving asthma symptoms and showed that it reduces bronchial hyperresponsiveness in children with asthma (Shimizu et al., 1994). Macrolides provide promising immunomodulatory effects however, their beneficial effects on airway remodeling in vivo have yet to be confirmed.
Mucolytics
Dating back to the 1960s, mucolytics were originally pursued for their ability to clear mucus plugs in muco-obstructive diseases such cystic fibrosis (CF) and chronic obstructive pulmonary disease (COPD) (Ehre et al., 2019). Mucolytics decrease mucus viscosity, enabling greater clearance, whereas expectorants are used to induce the expulsion of mucus from the respiratory tract. Small studies have shown the potential for use of inhaled N-acetylcysteine (NAC), a mucolytic and antioxidant agent (Millman et al., 1985). However, a randomized clinical trial demonstrated that NAC did not have a significant effect on improving asthma exacerbations (Aliyali et al., 2010). Yet, NAC has been shown to improve airway inflammation by modulating claudin 18 (tight junctional protein) expression (Lee et al., 2020). Further studies are needed to determine if NAC is effective for the treatment of asthma mucus plugging. Additionally, existing mucolytics including nacystelyn and methylcysteine hydrochloride, have been administered to CF patients however no studies, to our knowledge, exist for its use in asthma (Rogers, 2002). More recently, other novel mucolytics have been explored for the treatment of mucus plugging in asthma. Tris (2-carboxyethyl) phosphine (TCEP) can rapidly disrupt mucin disulfide bonds, and Morgan et al., have demonstrated its ability to reverse mucus plugging in an allergic mouse model (Morgan et al., 2021). Much more work is needed to provide effective mucolytic therapeutics to improve mucus plug clearance in asthma.
Other therapeutics
More recently, pharmacological targeting of the ASM has been of particular interest. In 2015, Girodet et al. showed in a large double-blinded, randomised study that after 12 months of treatment with gallopamil (an L-type calcium channel blocker normally used to treat coronary heart disease), there was an 18% reduction of ASM thickness in severe asthma at the fourth generation, measured by CT, and additionally, that there was a significant decrease in ASM cell proliferation (Girodet et al., 2015). The following year, Gonem et al. began investigating the effects of fevipiprant, a prostaglandin D2 antagonist in a phase 2 randomized, placebo-controlled trial, which reduced eosinophilic inflammation in the airways by blocking PGD2-driven release of Th2 cytokines and improved lung function (Gonem et al., 2016). Then, analysis of bronchial biopsies from the same patients with asthma in the previous study revealed that fevipiprant reduced airway smooth muscle mass in patients with asthma, likely by decreasing airway eosinophilia and decreasing recruitment of myofibroblasts and fibrocytes (Saunders et al., 2019). Though this drug initially seemed promising for the treatment of persistent severe asthma, in a phase 3 randomised, double-blind, placebo-controlled trial, there was found no significant improvement in lung function (Castro et al., 2021). Finally, the use of thiazolidinediones, oral insulin-sensitizing medications typically used to treat hyperglycemia and type 2 diabetes mellitus, has been examined for asthma treatment. A study by Ward et al. has shown that rosiglitazone has anti-proliferative effects on ASM cells in culture (Ward et al., 2004). Though there are some modest beneficial effects on allergen response, based on the results from initial clinical trials, thiazolidinediones are unlikely to have therapeutic benefit for airway remodeling (Richards et al., 2010; Sandhu et al., 2012).
Thus, much further work is required to assess the responses of current pharmacological agents for asthma on airway remodeling. However, as it has been highlighted by earlier studies, the concept that inflammation and airway remodeling are tied may not be correct and targeted therapies focused on airway remodeling features may be required.
Summary
This review article provides an overview of the existing knowledge on airway remodeling features observed in asthma, including epithelial damage, mucus cell metaplasia, ECM remodeling in both the airways and vessels, angiogenesis, and increased smooth muscle mass. While such studies have provided extensive knowledge on different aspects of airway remodeling, they have relied on biopsy sampling or autopsy lungs which have limitations. Biopsy samples are limited in the airway wall structures that can be sampled and the tissue artefacts that can be induced when crunched by the biopsy forceps. Autopsy studies on lungs from fatal asthmatics offer a unique opportunity to assess the heterogeneity of airway remodeling within a lung, but it does not provide the opportunity to assess the spectrum of the asthma syndrome. In this review, we highlight the potential of in vivo imaging tools such as CT and MRI that are enabling measures of the airway wall in vivo and longitudinally in research studies. Importantly, such volumetric imaging tools provide the opportunity to assess the heterogeneity of airway remodeling within the whole lung and has led to the novel identification of heterogenous gas trapping and mucus plugging as important predictors of patient outcomes. However, still more work needs to be done to now assess how these radiological features can be understood pathologically and then translated to therapeutic targets. Lastly, we summarize the current knowledge of modification of airway remodeling with available asthma therapeutics to highlight the need for future studies that could now use in vivo imaging tools to assess airway remodeling outcomes with therapeutics.
Author contributions
Conceptualization: AH, NA, and T-LH. Writing and original draft preparation: AH, NA, and T-LH. All authors have read and agreed to the published version of the manuscript.
Funding
This study was funded by a Canadian Institutes of Health Research (CIHR) operating grant MOP 130504. AH is supported by a CIHR Canadian Graduate Scholarship-Master’s Program. T-LH is supported by a Tier I Canada Research Chair in Asthma and COPD Lung Pathobiology and Therapeutics.
Conflict of interest
The authors declare that the research was conducted in the absence of any commercial or financial relationships that could be construed as a potential conflict of interest.
Publisher’s note
All claims expressed in this article are solely those of the authors and do not necessarily represent those of their affiliated organizations, or those of the publisher, the editors and the reviewers. Any product that may be evaluated in this article, or claim that may be made by its manufacturer, is not guaranteed or endorsed by the publisher.
References
Al Heialy S., Gaudet M., Ramakrishnan R. K., Mogas A., Salameh L., Mahboub B., et al. (2020). Contribution of IL-17 in steroid hyporesponsiveness in obese asthmatics through dysregulation of glucocorticoid receptors α and β. Front. Immunol. 11, 1724. doi:10.3389/fimmu.2020.01724
Albert M. S., Cates G. D., Driehuys B., Happer W., Saam B., Springer C. S., et al. (1994). Biological magnetic resonance imaging using laser-polarized 129Xe. Nature 370 (6486), 199–201. doi:10.1038/370199a0
Aliyali M., Amiri A. P., Sharifpoor A., Zalli F. (2010). Effects of N-acetylcysteine on asthma exacerbation. Iran. J. Allergy Asthma Immunol. 9, 103–109. Published online.
Allen M., Heinzmann A., Noguchi E., Abecasis G., Broxholme J., Ponting C. P., et al. (2003). Positional cloning of a novel gene influencing asthma from Chromosome 2q14. Nat. Genet. 35 (3), 258–263. doi:10.1038/ng1256
Altes T. A., Powers P. L., Knight-Scott J., Rakes G., Platts-Mills T. A., de Lange E. E., et al. (2001). Hyperpolarized 3He MR lung ventilation imaging in asthmatics: Preliminary findings. J. Magn. Reson Imaging 13 (3), 378–384. doi:10.1002/jmri.1054
Altes T. A., Mugler J. P., Ruppert K., Tustison N. J., Gersbach J., Szentpetery S., et al. (2016). Clinical correlates of lung ventilation defects in asthmatic children. J. Allergy Clin. Immunol. 137 (3), 789–796. doi:10.1016/j.jaci.2015.08.045
Ammit A. J., Bekir S. S., Johnson P. R., Hughes J. M., Armour C. L., Black J. L. (1997). Mast cell numbers are increased in the smooth muscle of human sensitized isolated bronchi. Am. J. Respir. Crit. Care Med. 155 (3), 1123–1129. doi:10.1164/ajrccm.155.3.9116997
Arakawa H., Webb W. R., McCowin M., Katsou G., Lee K. N., Seitz R. F. (1998). Inhomogeneous lung attenuation at thin-section CT: Diagnostic value of expiratory scans. Radiology 206 (1), 89–94. doi:10.1148/radiology.206.1.9423656
Araujo B. B., Dolhnikoff M., Silva L. F. F., Elliot J., Lindeman J. H. N., Ferreira D. S., et al. (2008). Extracellular matrix components and regulators in the airway smooth muscle in asthma. Eur. Respir. J. 32 (1), 61–69. doi:10.1183/09031936.00147807
Awadh N., Muller N. L., Park C. S., Abboud R. T., FitzGerald J. M. (1998). Airway wall thickness in patients with near fatal asthma and control groups: Assessment with high resolution computed tomographic scanning. Thorax 53 (4), 248–253. doi:10.1136/thx.53.4.248
Aysola R. S., Hoffman E. A., Gierada D., Wenzel S., Cook-Granroth J., Tarsi J., et al. (2008). Airway remodeling measured by multidetector CT is increased in severe asthma and correlates with pathology. Chest 134 (6), 1183–1191. doi:10.1378/chest.07-2779
Bachert P., Schad L. R., Bock M., Knopp M. V., EbertM. , Grossmann T., et al. (1996). Nuclear magnetic resonance imaging of airways in humans with use of hyperpolarized 3He. Magn. Reson Med. 36 (2), 192–196. doi:10.1002/mrm.1910360204
Banuelos J., Cao Y., Shin S. C., Lu N. Z. (2017). Immunopathology alters Th17 cell glucocorticoid sensitivity. Allergy 72 (3), 331–341. doi:10.1111/all.13051
Barbato A., Turato G., Baraldo S., Bazzan E., Calabrese F., Tura M., et al. (2003). Airway inflammation in childhood asthma. Am. J. Respir. Crit. Care Med. 168 (7), 798–803. doi:10.1164/rccm.200305-650OC
Barbato A., Turato G., Baraldo S., Bazzan E., Calabrese F., Panizzolo C., et al. (2006). Epithelial damage and angiogenesis in the airways of children with asthma. Am. J. Respir. Crit. Care Med. 174 (9), 975–981. doi:10.1164/rccm.200602-189OC
Basnet S., Bochkov Y. A., Brockman-Schneider R. A., Kuipers I., Aesif S. W., Jackson D. J., et al. (2019). CDHR3 asthma-risk genotype affects susceptibility of airway epithelium to rhinovirus C infections. Am. J. Respir. Cell Mol. Biol. 61 (4), 450–458. doi:10.1165/rcmb.2018-0220OC
Berair R., Brightling C. E. (2014). Asthma therapy and its effect on airway remodelling. Drugs 74 (12), 1345–1369. doi:10.1007/s40265-014-0250-4
Berger P., Girodet P. O., Manuel Tunon-de-Lara J. (2005). Mast cell myositis: A new feature of allergic asthma? Allergy 60 (10), 1238–1240. doi:10.1111/j.1398-9995.2005.00898.x
Bergeron C., Hauber H. P., Gotfried M., Newman K., Dhanda R., Servi R. J., et al. (2005). Evidence of remodeling in peripheral airways of patients with mild to moderate asthma: Effect of hydrofluoroalkane-flunisolide. J. Allergy Clin. Immunol. 116 (5), 983–989. doi:10.1016/j.jaci.2005.07.029
Bønnelykke K., Sleiman P., Nielsen K., Kreiner-Moller E., Mercader J. M., Belgrave D., et al. (2014). A genome-wide association study identifies CDHR3 as a susceptibility locus for early childhood asthma with severe exacerbations. Nat. Genet. 46 (1), 51–55. doi:10.1038/ng.2830
Bonser L. R., Erle D. J. (2017). Airway mucus and asthma: The role of MUC5AC and MUC5B. J. Clin. Med. 6 (12), 112. doi:10.3390/jcm6120112
Borish L., Steinke J. W. (2011). Interleukin-33 in asthma: How big of a role does it play? Curr. Allergy Asthma Rep. 11 (1), 7–11. doi:10.1007/s11882-010-0153-8
Boser S. R., Mauad T., Araújo-Paulino B. B. de, Mitchell I., Shrestha G., Chiu A., et al. (2017). Myofibroblasts are increased in the lung parenchyma in asthma. PLOS ONE 12 (8), e0182378. doi:10.1371/journal.pone.0182378
Boulet L. P., Turcotte H., Laviolette M., NaudF. , Bernier M. C., Martel S., et al. (2000). Airway hyperresponsiveness, inflammation, and subepithelial collagen deposition in recently diagnosed versus long-standing mild asthma. Influence of inhaled corticosteroids. Am. J. Respir. Crit. Care Med. 162 (4), 1308–1313. doi:10.1164/ajrccm.162.4.9910051
Brillet P. Y., Fetita C. I., Beigelman-Aubry C., SarAgAgliA A., Perchet D., PreteuxF. , et al. (2007). Quantification of bronchial dimensions at MDCT using dedicated software. Eur. Radiol. 17 (6), 1483–1489. doi:10.1007/s00330-006-0496-7
Brillet P. Y., Grenier P. A., Fetita C. I., Beigelman-Aubry C., Ould-Hmeidi Y., Ortner M., et al. (2013). Relationship between the airway wall area and asthma control score in moderate persistent asthma. Eur. Radiol. 23 (6), 1594–1602. doi:10.1007/s00330-012-2743-4
Broekema M., Timens W., Vonk J. M., Volbeda F., Lodewijk M. E., Hylkema M. N., et al. (2011). Persisting remodeling and less airway wall eosinophil activation in complete remission of asthma. Am. J. Respir. Crit. Care Med. 183 (3), 310–316. doi:10.1164/rccm.201003-0494OC
Brusasco V., Crimi E., Pellegrino R. (1998). Airway hyperresponsiveness in asthma: Not just a matter of airway inflammation. Thorax 53 (11), 992–998. doi:10.1136/thx.53.11.992
Brusselle G. G., VanderStichele C., Jordens P., Deman R., Slabbynck H., Ringoet V., et al. (2013). Azithromycin for prevention of exacerbations in severe asthma (AZISAST): A multicentre randomised double-blind placebo-controlled trial. Thorax 68 (4), 322–329. doi:10.1136/thoraxjnl-2012-202698
Burgess J. K., Mauad T., Tjin G., Karlsson J. C., Westergren-Thorsson G. (2016). The extracellular matrix – The under-recognized element in lung disease? J. Pathol. 240 (4), 397–409. doi:10.1002/path.4808
Busacker A., Newell J. D., Keefe T., Hoffman E. A., Granroth J. C., Castro M., et al. (2009). A multivariate analysis of risk factors for the air-trapping asthmatic phenotype as measured by quantitative CT analysis. Chest 135 (1), 48–56. doi:10.1378/chest.08-0049
Cada D. J., Bindler R. J., Baker D. E. (2016). Mepolizumab. Hosp. Pharm. 51 (5), 405–414. doi:10.1310/hpj5105-405
Camoretti-Mercado B., Lockey R. F. (2021). Airway smooth muscle pathophysiology in asthma. J. Allergy Clin. Immunol. 147 (6), 1983–1995. doi:10.1016/j.jaci.2021.03.035
Carayol N., Campbell A., Vachier I., Mainprice B., Bousquet J., Godard P., et al. (2002). Modulation of cadherin and catenins expression by tumor necrosis factor- α and dexamethasone in human bronchial epithelial cells. Am. J. Respir. Cell Mol. Biol. 26 (3), 341–347. doi:10.1165/ajrcmb.26.3.4684
Carroll N., Elliot J., Morton A., James A. (1993). The structure of large and small airways in nonfatal and fatal asthma. Am. Rev. Respir. Dis. 147 (2), 405–410. doi:10.1164/AJRCCM/147.2.405
Carroll N. G., Perry S., Karkhanis A., Butt J., James A. L., Green F. H., et al. (2000). The airway longitudinal elastic fiber network and mucosal folding in patients with asthma. Am. J. Respir. Crit. Care Med. 161 (1), 244–248. doi:10.1164/ajrccm.161.1.9805005
Carroll J. R. D., Magnussen J. S., Berend N., Salome C. M., King G. G. (2015). Greater parallel heterogeneity of airway narrowing and airway closure in asthma measured by high-resolution CT. Thorax 70 (12), 1163–1170. doi:10.1136/thoraxjnl-2014-206387
Castro M., Kerwin E., Miller D., Pedinoff A., Sher L., Cardenas P., et al. (2021). Efficacy and safety of fevipiprant in patients with uncontrolled asthma: Two replicate, phase 3, randomised, double-blind, placebo-controlled trials (ZEAL-1 and ZEAL-2). eClinicalMedicine 35, 100847. doi:10.1016/j.eclinm.2021.100847
Castro-Rodriguez J. A., Rodriguez-Martinez C. E., Ducharme F. M. (2018). Daily inhaled corticosteroids or montelukast for preschoolers with asthma or recurrent wheezing: A systematic review. Pediatr. Pulmonol. 53 (12), 1670–1677. doi:10.1002/ppul.24176
Chachi L., Diver S., Kaul H., Rebelatto M. C., Boutrin A., Nisa P., et al. (2019). Computational modelling prediction and clinical validation of impact of benralizumab on airway smooth muscle mass in asthma. Eur. Respir. J. 54 (5), 1900930. doi:10.1183/13993003.00930-2019
Chan B. C. L., Lam C. W. K., Tam L. S., Wong C. K. (2019). IL33: Roles in allergic inflammation and therapeutic perspectives. Front. Immunol. 10, 364. doi:10.3389/fimmu.2019.00364
Chaudhuri R., Rubin A., Sumino K., Lapa E Silva J. R., Niven R., Siddiqui S., et al. (2021). Safety and effectiveness of bronchial thermoplasty after 10 years in patients with persistent asthma (BT10+): A follow-up of three randomised controlled trials. Lancet Respir. Med. 9 (5), 457–466. doi:10.1016/S2213-2600(20)30408-2
Chen G., Khalil N. (2006). TGF-beta1 increases proliferation of airway smooth muscle cells by phosphorylation of map kinases. Respir. Res. 7 (1), 2. doi:10.1186/1465-9921-7-2
Chen W. J., Liaw S. F., Lin C. C., Lin M. W., Chang F. T. (2013). Effects of zileuton on airway smooth muscle remodeling after repeated allergen challenge in Brown Norway rats. Respiration 86 (5), 421–429. doi:10.1159/000353427
Chetta A., Zanini A., Foresi A., Del Donno M., Castagnaro A., D'Ippolito R., et al. (2003). Vascular component of airway remodeling in asthma is reduced by high dose of fluticasone. Am. J. Respir. Crit. Care Med. 167 (5), 751–757. doi:10.1164/rccm.200207-710OC
Chetta A., Zanini A., Torre O., Olivieri D. (2007). Vascular remodelling and angiogenesis in asthma: Morphological aspects and pharmacological modulation. Inflamm. Allergy Drug Targets 6 (1), 41–45. doi:10.2174/187152807780077273
Chin L. Y. M., Bossé Y., Pascoe C., Hackett T. L., Seow C. Y., Paré P. D. (2012). Mechanical properties of asthmatic airway smooth muscle. Eur. Respir. J. 40 (1), 45–54. doi:10.1183/09031936.00065411
Choi S., Hoffman E. A., Wenzel S. E., Tawhai M. H., Yin Y., Castro M., et al. (2013). Registration-based assessment of regional lung function via volumetric CT images of normal subjects vs. severe asthmatics. J. Appl. Physiol. 115 (5), 730–742. doi:10.1152/japplphysiol.00113.2013
Cohen P., Rajah R., Rosenbloom J., Herrick D. J. (2000). IGFBP-3 mediates TGF-beta1-induced cell growth in human airway smooth muscle cells. Am. J. Physiol-Lung Cell Mol. Physiol. 278 (3), L545–L551. doi:10.1152/ajplung.2000.278.3.L545
Comstock A. T., Ganesan S., Chattoraj A., Faris A. N., Margolis B. L., Hershenson M. B., et al. (2011). Rhinovirus-induced barrier dysfunction in polarized airway epithelial cells is mediated by NADPH oxidase 1. J. Virol. 85 (13), 6795–6808. doi:10.1128/JVI.02074-10
Cookson W. (2004). The immunogenetics of asthma and eczema: A new focus on the epithelium. Nat. Rev. Immunol. 4 (12), 978–988. doi:10.1038/nri1500
Coutts A., Chen G., Stephens N., Douglas D., EichholTz T., Khalil N., et al. (2001). Release of biologically active TGF-β from airway smooth muscle cells induces autocrine synthesis of collagen. Am. J. Physiol-Lung Cell Mol. Physiol. 280 (5), L999–L1008. doi:10.1152/ajplung.2001.280.5.L999
Csete M. E., Chediak A. D., Abraham W. M., Wanner A. (1991). Airway blood flow modifies allergic airway smooth muscle contraction. Am. Rev. Respir. Dis. 144 (1), 59–63. doi:10.1164/ajrccm/144.1.59
Curran D. R., Cohn L. (2010). Advances in mucous cell metaplasia: A plug for mucus as a therapeutic focus in chronic airway disease. Am. J. Respir. Cell Mol. Biol. 42 (3), 268–275. doi:10.1165/rcmb.2009-0151TR
Dahl R. (2006). Systemic side effects of inhaled corticosteroids in patients with asthma. Respir. Med. 100 (8), 1307–1317. doi:10.1016/j.rmed.2005.11.020
de Boer W. I., Sharma H. S., Baelemans S. M. I., Hoogsteden H. C., Lambrecht B. N., Braunstahl G. J. (2008). Altered expression of epithelial junctional proteins in atopic asthma: Possible role in inflammation. Can. J. Physiol. Pharmacol. 86 (3), 105–112. doi:10.1139/Y08-004
de Lange E. E., Altes T. A., Patrie J. T., Parmar J., Brookeman J. R., Mugler J. P., et al. (2007). The variability of regional airflow obstruction within the lungs of patients with asthma: Assessment with hyperpolarized helium-3 magnetic resonance imaging. J. Allergy Clin. Immunol. 119 (5), 1072–1078. doi:10.1016/j.jaci.2006.12.659
deFelice M., Silberschmidt D., DiLauro R., Xu Y., Wert S. E., Weaver T. E., et al. (2003). TTF-1 phosphorylation is required for peripheral lung morphogenesis, perinatal survival, and tissue-specific gene expression. J. Biol. Chem. 278 (37), 35574–35583. doi:10.1074/jbc.M304885200
Doeing D. C., Solway J. (2013). Airway smooth muscle in the pathophysiology and treatment of asthma. J. Appl. Physiol. Bethesda Md 1985 114 (7), 834–843. doi:10.1152/japplphysiol.00950.2012
Dombret M. C., Alagha K., Boulet L. P., Brillet P. Y., Joos G., Laviolette M., et al. (2014). Bronchial thermoplasty: A new therapeutic option for the treatment of severe, uncontrolled asthma in adults. Eur. Respir. Rev. 23 (134), 510–518. doi:10.1183/09059180.00005114
Donovan G. M., Elliot J. G., Green F. H. Y., James A. L., Noble P. B. (2018). Unraveling a clinical paradox: Why does bronchial thermoplasty work in asthma? Am. J. Respir. Cell Mol. Biol. 59 (3), 355–362. doi:10.1165/rcmb.2018-0011OC
Donovan G. M., Wang K. C. W., Shamsuddin D., Mann T. S., Henry P. J., Larcombe A. N., et al. (2020). Pharmacological ablation of the airway smooth muscle layer—mathematical predictions of functional improvement in asthma. Physiol. Rep. 8 (11), e14451. doi:10.14814/phy2.14451
Dorscheid D. R., Wojcik K. R., Sun S., Marroquin B., White S. R. (2001). Apoptosis of airway epithelial cells induced by corticosteroids. Am. J. Respir. Crit. Care Med. 164 (10), 1939–1947. doi:10.1164/ajrccm.164.10.2103013
Douwes J., Gibson P., Pekkanen J., Pearce N. (2002). Non-eosinophilic asthma: Importance and possible mechanisms. Thorax 57 (7), 643–648. doi:10.1136/thorax.57.7.643
Downie S. R., Salome C. M., Verbanck S., Thompson B., Berend N., King G. G. (2007). Ventilation heterogeneity is a major determinant of airway hyperresponsiveness in asthma, independent of airway inflammation. Thorax 62 (8), 684–689. doi:10.1136/thx.2006.069682
Dunican E. M., Elicker B. M., Gierada D. S., Nagle S. K., Schiebler M. L., Newell J. D., et al. (2018). Mucus plugs in patients with asthma linked to eosinophilia and airflow obstruction. J. Clin. Invest. 128 (3), 997–1009. doi:10.1172/JCI95693
Dunnill M. S. (1960). The pathology of asthma, with special reference to changes in the bronchial mucosa. J. Clin. Pathol. 13 (1), 27–33. doi:10.1136/jcp.13.1.27
Ebina M., Takahashi T., Chiba T., Motomiya M. (1993). Cellular hypertrophy and hyperplasia of airway smooth muscles underlying bronchial asthma: A 3-D morphometric study. Am. Rev. Respir. Dis. 148 (3), 720–726. doi:10.1164/ajrccm/148.3.720
Eddy R. L., McIntosh M. J., Matheson A. M., McCormack D. G., Licskai C., Parraga G. (2022). Pulmonary MRI and cluster analysis help identify novel asthma phenotypes. J. Magn. Reson Imaging 56 (5), 1475–1486. doi:10.1002/jmri.28152
Ehre C., Rushton Z. L., Wang B., Hothem L. N., Morrison C. B., Fontana N. C., et al. (2019). An improved inhaled mucolytic to treat airway muco-obstructive diseases. Am. J. Respir. Crit. Care Med. 199 (2), 171–180. doi:10.1164/rccm.201802-0245OC
Elias J. A., Zhu Z., Chupp G., Homer R. J. (1999). Airway remodeling in asthma. J. Clin. Invest. 104 (8), 1001–1006. doi:10.1172/JCI8124
Elliot J. G., Noble P. B., Mauad T., Bai T. R., Abramson M. J., McKay K. O., et al. (2018). Inflammation-dependent and independent airway remodelling in asthma. Respirology 23 (12), 1138–1145. doi:10.1111/RESP.13360
Esteves P., Blanc L., Celle A., Dupin I., Maurat E., Amoedo N., et al. (2021). Crucial role of fatty acid oxidation in asthmatic bronchial smooth muscle remodelling. Eur. Respir. J. 58 (5), 2004252. doi:10.1183/13993003.04252-2020
Esteves P., Allard B., Celle A., Dupin I., Maurat E., Ousova O., et al. (2022). Asthmatic bronchial smooth muscle increases rhinovirus replication within the bronchial epithelium. Cell Rep. 38 (13), 110571. doi:10.1016/j.celrep.2022.110571
Evans M. J., Plopper C. G. (1988). The role of basal cells in adhesion of columnar epithelium to airway basement membrane. Am. Rev. Respir. Dis. 138 (2), 481–483. doi:10.1164/ajrccm/138.2.481
Fain S. B., Gonzalez-Fernandez G., Peterson E. T., Evans M. D., Sorkness R. L., Jarjour N. N., et al. (2008). Evaluation of structure-function relationships in asthma using multidetector CT and hyperpolarized He-3 MRI. Acad. Radiol. 15 (6), 753–762. doi:10.1016/j.acra.2007.10.019
Ferreira M. A. R., McRae A. F., Medland S. E., Nyholt D. R., Gordon S. D., Wright M. J., et al. (2011). Association between ORMDL3, IL1RL1 and a deletion on chromosome 17q21 with asthma risk in Australia. Eur. J. Hum. Genet. 19 (4), 458–464. doi:10.1038/ejhg.2010.191
Flood-Page P., Menzies-Gow A., Phipps S., Ying S., Wangoo A., Ludwig M. S., et al. (2003). Anti-IL-5 treatment reduces deposition of ECM proteins in the bronchial subepithelial basement membrane of mild atopic asthmatics. J. Clin. Invest. 112 (7), 1029–1036. doi:10.1172/JCI17974
Ghaffar O., Hamid Q., Renzi P. M., Allakhverdi Z., Molet S., Hogg J. C., et al. (1999). Constitutive and cytokine-stimulated expression of eotaxin by human airway smooth muscle cells. Am. J. Respir. Crit. Care Med. 159 (6), 1933–1942. doi:10.1164/ajrccm.159.6.9805039
Gillis H. L., Lutchen K. R. (1999). Airway remodeling in asthma amplifies heterogeneities in smooth muscle shortening causing hyperresponsiveness. J. Appl. Physiol. 86 (6), 2001–2012. doi:10.1152/JAPPL.1999.86.6.2001/ASSET/IMAGES/LARGE/JAPP05633008X.JPEG
GINA Asthma. Glob strategy asthma manag prev. Published online 2012. Available at: http://ww5.ginaasthma.org/
Girodet P. O., Dournes G., Thumerel M., Begueret H., Dos Santos P., Ozier A., et al. (2015). Calcium channel blocker reduces airway remodeling in severe asthma. A proof-of-concept study. Am. J. Respir. Crit. Care Med. 191 (8), 876–883. doi:10.1164/rccm.201410-1874OC
Gonem S., Berair R., Singapuri A., Hartley R., Laurencin M. F. M., Bacher G., et al. (2016). Fevipiprant, a prostaglandin D2 receptor 2 antagonist, in patients with persistent eosinophilic asthma: A single-centre, randomised, double-blind, parallel-group, placebo-controlled trial. Lancet Respir. Med. 4 (9), 699–707. doi:10.1016/S2213-2600(16)30179-5
Gono H., Fujimoto K., Kawakami S., Kubo K. (2003). Evaluation of airway wall thickness and air trapping by HRCT in asymptomatic asthma. Eur. Respir. J. 22 (6), 965–971. doi:10.1183/09031936.03.00085302
Grainge C. L., Lau L. C. K., Ward J. A., Dulay V., Lahiff G., Wilson S., et al. (2011). Effect of bronchoconstriction on airway remodeling in asthma. N. Engl. J. Med. 364 (21), 2006–2015. doi:10.1056/NEJMoa1014350
Grenier P. A., Fetita C. I., Brillet P. Y. (2016). Quantitative computed tomography imaging of airway remodeling in severe asthma. Quant. Imaging Med. Surg. 6 (1), 76–83. doi:10.3978/j.issn.2223-4292.2016.02.08
Grigoraş A., Căruntu I. D., Grigoraş C. C., Mihăescu T., Amălinei C. (2012). Relationship between immunohistochemical assessment of bronchial mucosa microvascularization and clinical stage in asthma. Romanian J. Morphol. Embryol. Rev. Roum. Morphol. Embryol. 53 (3), 485–490.
Gudbjartsson D. F., Bjornsdottir U. S., Halapi E., Helgadottir A., Sulem P., Jonsdottir G. M., et al. (2009). Sequence variants affecting eosinophil numbers associate with asthma and myocardial infarction. Nat. Genet. 41 (3), 342–347. doi:10.1038/ng.323
Gupta S., Siddiqui S., Haldar P., Raj J. V., Entwisle J. J., Wardlaw A. J., et al. (2009). Qualitative analysis of high-resolution CT scans in severe asthma. Chest 136 (6), 1521–1528. doi:10.1378/chest.09-0174
Gupta S., Hartley R., Khan U. T., Singapuri A., Hargadon B., Monteiro W., et al. (2014). Quantitative computed tomography–derived clusters: Redefining airway remodeling in asthmatic patients. J. Allergy Clin. Immunol. 133 (3), 729–738. doi:10.1016/j.jaci.2013.09.039
Hackett T. L., Knight D. A. (2007). The role of epithelial injury and repair in the origins of asthma. Curr. Opin. Allergy Clin. Immunol. 7 (1), 63–68. doi:10.1097/ACI.0b013e328013d61b
Hackett T. L., Shaheen F., Johnson A., Wadsworth S., Pechkovsky D. V., Jacoby D. B., et al. (2008). Characterization of side population cells from human airway epithelium. Stem Cells 26 (10), 2576–2585. doi:10.1634/stemcells.2008-0171
Hackett T. L., Warner S. M., Stefanowicz D., Shaheen F., Pechkovsky D. V., Murray L. A., et al. (2009). Induction of epithelial-mesenchymal transition in primary airway epithelial cells from patients with asthma by transforming growth factor-beta1. Am. J. Respir. Crit. Care Med. 180 (2), 122–133. doi:10.1164/rccm.200811-1730OC
Hackett T. L., Singhera G. K., Shaheen F., Hayden P., Jackson G. R., Hegele R. G., et al. (2011). Intrinsic phenotypic differences of asthmatic epithelium and its inflammatory responses to respiratory syncytial virus and air pollution. Am. J. Respir. Cell Mol. Biol. 45 (5), 1090–1100. doi:10.1165/rcmb.2011-0031OC
Hackett T. L., de Bruin H. G., Shaheen F., van den Berge M., van Oosterhout A. J., Postma D. S., et al. (2013). Caveolin-1 controls airway epithelial barrier function. Implications for asthma. Am. J. Respir. Cell Mol. Biol. 49 (4), 662–671. doi:10.1165/rcmb.2013-0124OC
Haefeli-Bleuer B., Weibel E. R. (1988). Morphometry of the human pulmonary acinus. Anat. Rec. 220 (4), 401–414. doi:10.1002/ar.1092200410
Hakonarson H., Maskeri N., Carter C., Chuang S., Grunstein M. M. (1999). Autocrine interaction between IL-5 and IL-1beta mediates altered responsiveness of atopic asthmatic sensitized airway smooth muscle. J. Clin. Invest. 104 (5), 657–667. doi:10.1172/JCI7137
Harada M., Hirota T., Jodo A. I., Hitomi Y., Sakashita M., Tsunoda T., et al. (2011). Thymic stromal lymphopoietin gene promoter polymorphisms are associated with susceptibility to bronchial asthma. Am. J. Respir. Cell Mol. Biol. 44 (6), 787–793. doi:10.1165/rcmb.2009-0418OC
Haraguchi M., Shimura S., Shirato K. (1999). Morphometric analysis of bronchial cartilage in chronic obstructive pulmonary disease and bronchial asthma. Am. J. Respir. Crit. Care Med. 159 (3), 1005–1013. doi:10.1164/ajrccm.159.3.9712144
Hasegawa M., Nasuhara Y., Onodera Y., Makita H., Nagai K., Fuke S., et al. (2006). Airflow limitation and airway dimensions in chronic obstructive pulmonary disease. Am. J. Respir. Crit. Care Med. 173 (12), 1309–1315. doi:10.1164/rccm.200601-037OC
Hashimoto M., Tanaka H., Abe S. (2005). Quantitative analysis of bronchial wall vascularity in the medium and small airways of patients with asthma and COPD. Chest 127 (3), 965–972. doi:10.1378/chest.127.3.965
He J. Q., Hallstrand T. S., Knight D., Chan-Yeung M., Sandford A., Tripp B., et al. (2009). A thymic stromal lymphopoietin gene variant is associated with asthma and airway hyperresponsiveness. J. Allergy Clin. Immunol. 124 (2), 222–229. doi:10.1016/j.jaci.2009.04.018
Hirst S. J., Barnes P. J., Twort C. H. (1996). PDGF isoform-induced proliferation and receptor expression in human cultured airway smooth muscle cells. Am. J. Physiol-Lung Cell Mol. Physiol. 270 (3), L415–L428. doi:10.1152/ajplung.1996.270.3.L415
Holbrook M. D., Clark D. P., Patel R., Qi Y., Bassil A. M., Mowery Y. M., et al. (2021). Detection of lung nodules in micro-CT imaging using deep learning. Tomography 7 (3), 358–372. doi:10.3390/tomography7030032
Holgate S. T. (2008). Pathogenesis of asthma. Clin. Exp. Allergy 38 (6), 872–897. doi:10.1111/j.1365-2222.2008.02971.x
Hong L., Herjan T., Bulek K., Xiao J., Comhair S. A. A., Erzurum S. C., et al. (2022). Mechanisms of corticosteroid resistance in type 17 asthma. J. Immunol. Author Choice 209 (10), 1860–1869. doi:10.4049/jimmunol.2200288
Horsfield K., Cumming G. (1968). Morphology of the bronchial tree in man. J. Appl. Physiol. 24 (3), 373–383. doi:10.1152/jappl.1968.24.3.373
Horwitz R. J., McGILL K. A., Busse W. W. (1998). The role of leukotriene modifiers in the treatment of asthma. Am. J. Respir. Crit. Care Med. 157 (5), 1363–1371. doi:10.1164/ajrccm.157.5.9706059
Hoshino M., Ohtawa J. (2012). Effects of adding omalizumab, an anti-immunoglobulin E antibody, on airway wall thickening in asthma. Respir. Int. Rev. Thorac. Dis. 83 (6), 520–528. doi:10.1159/000334701
Hoshino M., Takahashi M., Aoike N. (2001). Expression of vascular endothelial growth factor, basic fibroblast growth factor, and angiogenin immunoreactivity in asthmatic airways and its relationship to angiogenesis. J. Allergy Clin. Immunol. 107 (2), 295–301. doi:10.1067/mai.2001.111928
Hoshino M., Takahashi M., Takai Y., Sim J., Aoike N. (2001). Inhaled corticosteroids decrease vascularity of the bronchial mucosa in patients with asthma. Clin. Exp. Allergy J. Br. Soc. Allergy Clin. Immunol. 31 (5), 722–730. doi:10.1046/j.1365-2222.2001.01071.x
Huang M., Ding M., Radzikowska U., Rodriguez-Coira J., Stocker N., Tan G., et al. (2022). Distinct metabolic reprogramming of airway epithelium in asthma in response to infection with rhinovirus. ERJ Open Res. 8. doi:10.1183/23120541.LSC-2022.200
Huber Hl, Koessler Kk (1922). The pathology of bronchial asthma. Arch. Intern Med. 30 (6), 689–760. doi:10.1001/archinte.1922.00110120002001
Hur J., Kang J. Y., Rhee C. K., Kim Y. K., Lee S. Y. (2018). The leukotriene receptor antagonist pranlukast attenuates airway remodeling by suppressing TGF-β signaling. Pulm. Pharmacol. Ther. 48, 5–14. doi:10.1016/j.pupt.2017.10.007
Ijpma G., Kachmar L., Panariti A., Matusovsky O. S., Torgerson D., Benedetti A., et al. (2020). Intrapulmonary airway smooth muscle is hyperreactive with a distinct proteome in asthma. Eur. Respir. J. 56 (1), 1902178. doi:10.1183/13993003.02178-2019
James A. L., Pare P. D., Hogg J. C. (1989). The mechanics of airway narrowing in asthma. Am. Rev. Respir. Dis. 139 (1), 242–246. doi:10.1164/AJRCCM/139.1.242
James A. L., Maxwell P. S., Pearce-Pinto G., Elliot J. G., Carroll N. G. (2012). The relationship of reticular basement membrane thickness to airway wall remodeling in asthma. Am. J. Respir. Crit. Care Med. 166 (12 I), 1590–1595. doi:10.1164/RCCM.2108069
James A. L., Elliot J. G., Jones R. L., Carroll M. L., Mauad T., Bai T. R., et al. (2012). Airway smooth muscle hypertrophy and hyperplasia in asthma. Am. J. Respir. Crit. Care Med. 185 (10), 1058–1064. doi:10.1164/rccm.201110-1849OC
James A. (2005). Airway remodeling in asthma. Curr. Opin. Pulm. Med. 11 (1), 1–6. doi:10.1097/01.mcp.0000146779.26339.d8
Jeffery P. K., Godfrey R. W., Ädelroth E., Nelson F., Rogers A., Johansson S. A. (1992). Effects of treatment on airway inflammation and thickening of basement membrane reticular collagen in asthma: A quantitative light and electron microscopic study. Am. Rev. Respir. Dis. 145, 890–899. doi:10.1164/ajrccm/145.4_Pt_1.890
Johnson P. R. A., Roth M., Tamm M., HughesM. , Ge Q., KinG G., et al. (2001). Airway smooth muscle cell proliferation is increased in asthma. Am. J. Respir. Crit. Care Med. 164 (3), 474–477. doi:10.1164/ajrccm.164.3.2010109
Johnson P. R. A., Burgess J. K., Underwood P. A., Au W., Poniris M. H., Tamm M., et al. (2004). Extracellular matrix proteins modulate asthmatic airway smooth muscle cell proliferation via an autocrine mechanism. J. Allergy Clin. Immunol. 113 (4), 690–696. doi:10.1016/j.jaci.2003.12.312
Kaminska M., Foley S., Maghni K., Storness-Bliss C., Coxson H., Ghezzo H., et al. (2009). Airway remodeling in subjects with severe asthma with or without chronic persistent airflow obstruction. J. Allergy Clin. Immunol. 124 (1), 45e1–514. doi:10.1016/j.jaci.2009.03.049
Kanazawa H., Hirata K., Yoshikawa J. (2002). Involvement of vascular endothelial growth factor in exercise induced bronchoconstriction in asthmatic patients. Thorax 57 (10), 885–888. doi:10.1136/thorax.57.10.885
Kanazawa H., Asai K., Hirata K., Yoshikawa J. (2002). Vascular involvement in exercise-induced airway narrowing in patients with bronchial asthma. Chest 122 (1), 166–170. doi:10.1378/chest.122.1.166
Kang J. Y., Jo M. R., Kang H. H., Kim S. K., Kim M. S., Kim Y. H., et al. (2016). Long-term azithromycin ameliorates not only airway inflammation but also remodeling in a murine model of chronic asthma. Pulm. Pharmacol. Ther. 36, 37–45. doi:10.1016/j.pupt.2015.12.002
Kawasaki S., Takizawa H., Ohtoshi T., TakeuchiN. , Kohyama T., Nakamura H., et al. (1998). Roxithromycin inhibits cytokine production by and neutrophil attachment to human bronchial epithelial cells in vitro. Antimicrob. Agents Chemother. 42 (6), 1499–1502. doi:10.1128/AAC.42.6.1499
Kern A. L., Vogel-Claussen J. (2018). Hyperpolarized gas MRI in pulmonology. Br. J. Radiol. 91 (1084), 20170647. doi:10.1259/bjr.20170647
Khalajzeyqami Z., Grandi A., Ferrini E., Ravanetti F., Mambrini M., Leo L., et al. (2022). Pivotal role of micro-CT technology in setting up an optimized lung fibrosis mouse model for drug screening. Plos One 17 (6), e0270005. doi:10.1371/journal.pone.0270005
King G. G., Carroll J. D., Müller N. L., Whittall K. P., Gao M., Nakano Y., et al. (2004). Heterogeneity of narrowing in normal and asthmatic airways measured by HRCT. Eur. Respir. J. 24 (2), 211–218. doi:10.1183/09031936.04.00047503
Kirby M., Tanabe N., Tan W. C., Zhou G., Obeidat M., Hague C. J., et al. (2018). Total airway count on computed tomography and the risk of chronic obstructive pulmonary disease progression. Findings from a population-based study. Am. J. Respir. Crit. Care Med. 197 (1), 56–65. doi:10.1164/rccm.201704-0692OC
Knight D. A., Stick S. M., Hackett T. L. (2011). Defective function at the epithelial junction: A novel therapeutic frontier in asthma? J. Allergy Clin. Immunol. 128 (3), 557–558. doi:10.1016/j.jaci.2011.07.031
Koo H. K., Vasilescu D. M., Booth S., Hsieh A., Katsamenis O. L., Fishbane N., et al. (2018). Small airways disease in mild and moderate chronic obstructive pulmonary disease: A cross-sectional study. Lancet Respir. Med. 6 (8), 591–602. doi:10.1016/S2213-2600(18)30196-6
Koppelman G. H., Meyers D. A., Howard T. D., Zheng S. L., Hawkins G. A., Ampleford E. J., et al. (2009). Identification of PCDH1 as a novel susceptibility gene for bronchial hyperresponsiveness. Am. J. Respir. Crit. Care Med. 180 (10), 929–935. doi:10.1164/rccm.200810-1621OC
Kumar S. D., Emery M. J., Atkins N. D., Danta I., Wanner A. (1998). Airway mucosal blood flow in bronchial asthma. Am. J. Respir. Crit. Care Med. 158 (1), 153–156. doi:10.1164/ajrccm.158.1.9712141
Kuo C., Lim S., King N. J. C., Johnston S. L., Burgess J. K., Black J. L., et al. (2011). Rhinovirus infection induces extracellular matrix protein deposition in asthmatic and nonasthmatic airway smooth muscle cells. Am. J. Physiol. Lung Cell Mol. Physiol. 300 (6), L951–L957. doi:10.1152/ajplung.00411.2010
Kurashima K., Hoshi T., Takaku Y., Kanauchi T., Nakamoto K., Ueda M., et al. (2013). Changes in the airway lumen and surrounding parenchyma in chronic obstructive pulmonary disease. Int. J. Chron. Obstruct Pulmon Dis. 8, 523–532. doi:10.2147/COPD.S52637
Kuruvilla M. E., Lee F. E. H., Lee G. B. (2019). Understanding asthma phenotypes, endotypes, and mechanisms of disease. Clin. Rev. Allergy Immunol. 56 (2), 219–233. doi:10.1007/s12016-018-8712-1
Kuwano K., Bosken C. H., Paré P. D., Bai T. R., Wiggs B. R., Hogg J. C. (1993). Small airways dimensions in asthma and in chronic obstructive pulmonary disease. Am. Rev. Respir. Dis. 148 (5), 1220–1225. doi:10.1164/ajrccm/148.5.1220
Kuyper L. M., Paré P. D., Hogg J. C., Lambert R. K., Ionescu D., Woods R., et al. (2003). Characterization of airway plugging in fatal asthma. Am. J. Med. 115 (1), 6–11. doi:10.1016/s0002-9343(03)00241-9
Laitinen L. A., Heino M., Laitinen A., Kava T., Haahtela T. (1985). Damage of the airway epithelium and bronchial reactivity in patients with asthma. Am. Rev. Respir. Dis. 131 (4), 599–606. doi:10.1164/arrd.1985.131.4.599
Laitinen A., Altraja A., Kämpe M., Linden M., Virtanen I., Laitinen L. A. (1997). Tenascin is increased in airway basement membrane of asthmatics and decreased by an inhaled steroid. Am. J. Respir. Crit. Care Med. 156 (3), 951–958. doi:10.1164/ajrccm.156.3.9610084
Laitinen T., Polvi A., Rydman P., Vendelin J., Pulkkinen V., Salmikangas P., et al. (2004). Characterization of a common susceptibility locus for asthma-related traits. Science 304 (5668), 300–304. doi:10.1126/science.1090010
Lambert R. K., Codd S. L., Alley M. R., Pack R. J. (1994). Physical determinants of bronchial mucosal folding. J. Appl. Physiol. 77 (3), 1206–1216. doi:10.1152/jappl.1994.77.3.1206
Lambert R. K. (1991). Role of bronchial basement membrane in airway collapse. J. Appl. Physiol. 71 (2), 666–673. doi:10.1152/jappl.1991.71.2.666
Lambrecht B. N., Hammad H. (2012). The airway epithelium in asthma. Nat. Med. 18 (5), 684–692. doi:10.1038/nm.2737
Langton D., Wang W., Thien F., Plummer V. (2018). The acute effects of bronchial thermoplasty on FEV1. Respir. Med. 137, 147–151. doi:10.1016/j.rmed.2018.03.003
Langton D., Bennetts K., Noble P., Plummer V., Thien F. (2020). Bronchial thermoplasty reduces airway resistance. Respir. Res. 21 (1), 76. doi:10.1186/s12931-020-1330-5
Langton D., Noble P. B., Donovan G. M. (2021). Response of individual airways in vivo to bronchial thermoplasty. J. Appl. Physiol. 130 (4), 1205–1213. doi:10.1152/japplphysiol.00959.2020
Le Gros G., Ben-Sasson S. Z., Seder R., Finkelman F. D., Paul W. E. (1990). Generation of interleukin 4 (IL-4)-producing cells in vivo and in vitro: IL-2 and IL-4 are required for in vitro generation of IL-4-producing cells. J. Exp. Med. 172 (3), 921–929. doi:10.1084/jem.172.3.921
Lederlin M., Ozier A., Dournes G., Ousova O., Girodet P. O., Begueret H., et al. (2012). In vivo micro-CT assessment of airway remodeling in a flexible OVA-sensitized murine model of asthma. Plos One 7 (10), e48493. doi:10.1371/journal.pone.0048493
Lee P. H., Hong J., Jang A. S. (2020). N-acetylcysteine decreases airway inflammation and responsiveness in asthma by modulating claudin 18 expression. Korean J. Intern Med. 35 (5), 1229–1237. doi:10.3904/kjim.2019.105
Li X., Wilson J. W. (1997). Increased vascularity of the bronchial mucosa in mild asthma. Am. J. Respir. Crit. Care Med. 156 (1), 229–233. doi:10.1164/AJRCCM.156.1.9607066
Li X., Hastie A. T., Hawkins G. A., Moore W. C., Ampleford E. J., Milosevic J., et al. (2015). eQTL of bronchial epithelial cells and bronchial alveolar lavage deciphers GWAS-identified asthma genes. Allergy 70 (10), 1309–1318. doi:10.1111/all.12683
Looi K., Troy N. M., Garratt L. W., Iosifidis T., Bosco A., Buckley A. G., et al. (2016). Effect of human rhinovirus infection on airway epithelium tight junction protein disassembly and transepithelial permeability. Exp. Lung Res. 42 (7), 380–395. doi:10.1080/01902148.2016.1235237
Looi K., Buckley A. G., Rigby P. J., Garratt L. W., Iosifidis T., Zosky G. R., et al. (2018). Effects of human rhinovirus on epithelial barrier integrity and function in children with asthma. Clin. Exp. Allergy 48 (5), 513–524. doi:10.1111/cea.13097
Ludwig M. S., Dreshaj I., Solway J., Munoz A., Ingram R. H. (1987). Partitioning of pulmonary resistance during constriction in the dog: Effects of volume history. J. Appl. Physiol. Bethesda Md 1985 62 (2), 807–815. doi:10.1152/jappl.1987.62.2.807
Lundgren R., Soderberg M., Horstedt P., Stenling R. (1988). Morphological studies of bronchial mucosal biopsies from asthmatics before and after ten years of treatment with inhaled steroids. Eur. Respir. J. 1 (10), 883–889. doi:10.1183/09031936.93.01100883
Malmström K., Lohi J., Sajantila A., Jahnsen F. L., Kajosaari M., Sarna S., et al. (2017). Immunohistology and remodeling in fatal pediatric and adolescent asthma. Respir. Res. 18 (1), 94. doi:10.1186/s12931-017-0575-0
Mattos W., Lim S., Russell R., Jatakanon A., Chung K. F., Barnes P. J. (2002). Matrix metalloproteinase-9 expression in asthma: Effect of asthma severity, allergen challenge, and inhaled corticosteroids. Chest 122 (5), 1543–1552. doi:10.1378/chest.122.5.1543
McDonough J. E., Yuan R., Suzuki M., Seyednejad N., Elliott W. M., Sanchez P. G., et al. (2011). Small-airway obstruction and emphysema in chronic obstructive pulmonary disease. N. Engl. J. Med. 365 (17), 1567–1575. doi:10.1056/NEJMoa1106955
McDonough J. E. (2012). Use of CT and MicroCT to quantify structures in human lungs. Columbia: University of British Columbia. doi:10.14288/1.0072712
Mendes E. S., Campos M. A., Hurtado A., Wanner A. (2004). Effect of montelukast and fluticasone propionate on airway mucosal blood flow in asthma. Am. J. Respir. Crit. Care Med. 169 (10), 1131–1134. doi:10.1164/rccm.200311-1544OC
Metcalfe D. D., Baram D., Mekori Y. A. (1997). Mast cells. Physiol. Rev. 77 (4), 1033–1079. doi:10.1152/physrev.1997.77.4.1033
Michalik M., Wójcik-Pszczoła K., Paw M., Wnuk D., Koczurkiewicz P., Sanak M., et al. (2018). Fibroblast-to-myofibroblast transition in bronchial asthma. Cell Mol. Life Sci. 75 (21), 3943–3961. doi:10.1007/s00018-018-2899-4
Millman M., Millman F. M., Goldstein I. M., Mercandetti A. J. (1985). Use of acetylcysteine in bronchial asthma-another look. Ann. Allergy 54 (4), 294–296.
Minshall E., Wang C. G., Dandurand R., Eidelman D. (1997). Heterogeneity of responsiveness of individual airways in cultured lung explants. Can. J. Physiol. Pharmacol. 75 (7), 911–916. doi:10.1139/y97-107
Moffatt M. F., Kabesch M., Liang L., Dixon A. L., Strachan D., Heath S., et al. (2007). Genetic variants regulating ORMDL3 expression contribute to the risk of childhood asthma. Nature 448 (7152), 470–473. doi:10.1038/nature06014
Moffatt M. F., Gut I. G., Demenais F., Strachan D. P., Bouzigon E., Heath S., et al. (2010). A large-scale, consortium-based genomewide association study of asthma. N. Engl. J. Med. 363 (13), 1211–1221. doi:10.1056/NEJMoa0906312
Moffatt M. F. (2008). Genes in asthma: New genes and new ways. Curr. Opin. Allergy Clin. Immunol. 8 (5), 411–417. doi:10.1097/ACI.0b013e32830f1dc1
Moheimani F., Hsu A. C. Y., Reid A. T., Williams T., Kicic A., Stick S. M., et al. (2016). The genetic and epigenetic landscapes of the epithelium in asthma. Respir. Res. 17 (1), 119. doi:10.1186/s12931-016-0434-4
Montuschi P. (2010). Role of leukotrienes and leukotriene modifiers in asthma. Pharmaceuticals 3 (6), 1792–1811. doi:10.3390/ph3061792
Moore P. E., Church T. L., Chism D. D., Panettieri R. A., Shore S. A. (2002). IL-13 and IL-4 cause eotaxin release in human airway smooth muscle cells: A role for ERK. Am. J. Physiol. Lung Cell Mol. Physiol. 282 (4), L847–L853. doi:10.1152/ajplung.00245.2001
Morgan L. E., Jaramillo A. M., Shenoy S. K., Raclawska D., Emezienna N. A., Richardson V. L., et al. (2021). Disulfide disruption reverses mucus dysfunction in allergic airway disease. Nat. Commun. 12 (1), 249. doi:10.1038/s41467-020-20499-0
Mosmann T. R., Cherwinski H., Bond M. W., Giedlin M. A., Coffman R. L. (1986). Two types of murine helper T cell clone. I. Definition according to profiles of lymphokine activities and secreted proteins. J. Immunol. Balt. Md 136 (7), 2348–2357. doi:10.4049/jimmunol.136.7.2348
Mostaco-Guidolin L., Hajimohammadi S., Vasilescu D. M., Hackett T. L. (2017). Application of Euclidean distance mapping for assessment of basement membrane thickness distribution in asthma. J. Appl. Physiol. 123 (2), 473–481. doi:10.1152/japplphysiol.00171.2017
Mostaço-Guidolin L. B., Osei E. T., Ullah J., Hajimohammadi S., Fouadi M., Li X., et al. (2019). Defective fibrillar collagen organization by fibroblasts contributes to airway remodeling in asthma. Am. J. Respir. Crit. Care Med. 200 (4), 431–443. doi:10.1164/rccm.201810-1855OC
Mostaço-Guidolin L. B., Yang C. X., Hackett T. L. (2021). Pulmonary vascular remodeling is an early feature of fatal and nonfatal asthma. Am. J. Respir. Cell Mol. Biol. 65 (1), 114–118. doi:10.1165/rcmb.2020-0339LE
Moynihan B. J., Tolloczko B., El Bassam S., Ferraro P., Michoud M. C., Martin J. G., et al. (2008). IFN-gamma, IL-4 and IL-13 modulate responsiveness of human airway smooth muscle cells to IL-13. Respir. Res. 9, 84. doi:10.1186/1465-9921-9-84
Mummy D. G., Dunican E. M., Carey K. J., Evans M. D., Elicker B. M., Newell J. D., et al. (2022). Mucus plugs in asthma at CT associated with regional ventilation defects at 3He MRI. Radiology 303 (1), 184–190. doi:10.1148/radiol.2021204616
Neelakantan S., Xin Y., Gaver D. P., Cereda M., Rizi R., Smith B. J., et al. (2022). Computational lung modelling in respiratory medicine. J. R. Soc. Interface 19 (191), 20220062. doi:10.1098/rsif.2022.0062
Newman K. B., Lynch D. A., Newman L. S., Ellegood D., Newell J. D. (1994). Quantitative computed tomography detects air trapping due to asthma. Chest 106 (1), 105–109. doi:10.1378/chest.106.1.105
Niimi A., Matsumoto H., Amitani R., MishiMa M., Minakuchi M., Izumi T., et al. (2000). Airway wall thickness in asthma assessed by computed tomography. Relation to clinical indices. Am. J. Respir. Crit. Care Med. 162, 1518–1523. doi:10.1164/ajrccm.162.4.9909044
Noble P. B., Turner D. J., Mitchell H. W. (2002). Relationship of airway narrowing, compliance, and cartilage in isolated bronchial segments. J. Appl. Physiol. 92 (3), 1119–1124. doi:10.1152/japplphysiol.00662.2001
Noble P. B., Pascoe C. D., Lan B., Demoulin B., Chalon B., Ruckebusch O., et al. (2014). Airway smooth muscle in asthma: Linking contraction and mechanotransduction to disease pathogenesis and remodelling. Pulm. Pharmacol. Ther. 29 (2), 96–101. doi:10.1016/j.pupt.2013.07.005
Okuda K., Chen G., Subramani D. B., Wolf M., Gilmore R. C., Kato T., et al. (2019). Localization of secretory mucins MUC5AC and MUC5B in normal/healthy human airways. Am. J. Respir. Crit. Care Med. 199 (6), 715–727. doi:10.1164/rccm.201804-0734OC
Orsida B. E., Li X., Hickey B., Thien F., Wilson J. W., Walters E. H. (1999). Vascularity in asthmatic airways: Relation to inhaled steroid dose. Thorax 54 (4), 289–295. doi:10.1136/thx.54.4.289
Osei E. T., B Mostaço-Guidolin L., Hsieh A., Warner S. M., Al-Fouadi M., Wang M., et al. (2020). Epithelial-interleukin-1 inhibits collagen formation by airway fibroblasts: Implications for asthma. Sci. Rep. 10 (1), 8721. doi:10.1038/s41598-020-65567-z
Otis A. B., McKerrow C. B., Bartlett R. A., Mead J., Mcilroy M. B., Selver-Stone N. J., et al. (1956). Mechanical factors in distribution of pulmonary ventilation. J. Appl. Physiol. 8 (4), 427–443.
Paik S. H., Kim W. K., Park J. S., Park C. S., Jin G. Y. (2014). A quantitative study of airway changes on micro-CT in a mouse asthma model: Comparison with histopathological findings. Allergy Asthma Immunol. Res. 6 (1), 75–82. doi:10.4168/aair.2014.6.1.75
Panettieri R. A., Goldie R. G., Rigby P. J., Eszterhas A. J., Hay D. W. (1996). Endothelin-1-induced potentiation of human airway smooth muscle proliferation: An ETA receptor-mediated phenomenon. Br. J. Pharmacol. 118 (1), 191–197. doi:10.1111/j.1476-5381.1996.tb15385.x
Paplińska-Goryca M., Grabczak E. M., Dąbrowska M., Hermanowicz-Salamon J., Proboszcz M., Nejman-Gryz P., et al. (2018). Sputum interleukin-25 correlates with asthma severity: A preliminary study. Adv. Dermatol Allergol. Dermatol Alergol. 35 (5), 462–469. doi:10.5114/ada.2017.71428
Park K. S., Korfhagen T. R., Bruno M. D., Kitzmiller J. A., Wan H., Wert S. E., et al. (2007). SPDEF regulates goblet cell hyperplasia in the airway epithelium. J. Clin. Invest. 117 (4), 978–988. doi:10.1172/JCI29176
Pascoe C. D., Seow C. Y., Hackett T. L., Paré P. D., Donovan G. M. (2017). Heterogeneity of airway wall dimensions in humans: A critical determinant of lung function in asthmatics and nonasthmatics. Am. J. Physiol. Lung Cell Mol. Physiol. 312 (3), L425–L431. doi:10.1152/AJPLUNG.00421.2016
Pascoe C. D., Green F. H. Y., Elliot J. G., James A. L., Noble P. B., Donovan G. M. (2020). Airway remodelling with spatial correlations: Implications for asthma pathogenesis. Respir. Physiol. Neurobiol. 279, 103469. doi:10.1016/j.resp.2020.103469
Payne D. N. R., Rogers A. V., Adelroth E., Bandi V., Guntupalli K. K., Bush A., et al. (2003). Early thickening of the reticular basement membrane in children with difficult asthma. Am. J. Respir. Crit. Care Med. 167 (1), 78–82. doi:10.1164/rccm.200205-414OC
Pei Q. M., Jiang P., Yang M., Qian X. J., Liu J. B., Kim S. H. (2016). Roxithromycin inhibits VEGF-induced human airway smooth muscle cell proliferation: Opportunities for the treatment of asthma. Exp. Cell Res. 347 (2), 378–384. doi:10.1016/j.yexcr.2016.08.024
Pelaia C., Calabrese C., Vatrella A., Busceti M. T., Garofalo E., Lombardo N., et al. (2018). Benralizumab: From the basic mechanism of action to the potential use in the biological therapy of severe eosinophilic asthma. Biomed. Res. Int. 2018, 4839230. doi:10.1155/2018/4839230
Phelan P. D., Robertson C. F., Olinsky A. (2002). The melbourne asthma study: 1964-1999. J. Allergy Clin. Immunol. 109 (2), 189–194. doi:10.1067/mai.2002.120951
Ramakrishnan R. K., Al Heialy S., Hamid Q. (2019). Role of IL-17 in asthma pathogenesis and its implications for the clinic. Expert Rev. Respir. Med. 13 (11), 1057–1068. doi:10.1080/17476348.2019.1666002
Ramos-Barbón D., Fraga-Iriso R., Brienza N. S., Montero-Martinez C., Verea-Hernando H., Olivenstein R., et al. (2010). T cells localize with proliferating smooth muscle α-actin+ cell compartments in asthma. Am. J. Respir. Crit. Care Med. 182 (3), 317–324. doi:10.1164/rccm.200905-0745OC
Raundhal M., Morse C., Khare A., Oriss T. B., Milosevic J., Trudeau J., et al. (2015). High IFN-γ and low SLPI mark severe asthma in mice and humans. J. Clin. Invest. 125 (8), 3037–3050. doi:10.1172/JCI80911
Rezaee F., DeSando S. A., Ivanov A. I., Chapman T. J., Knowlden S. A., Beck L. A., et al. (2013). Sustained protein kinase D activation mediates respiratory syncytial virus-induced airway barrier disruption. J. Virol. 87 (20), 11088–11095. doi:10.1128/JVI.01573-13
Riccio A. M., Dal Negro R. W., Micheletto C., De Ferrari L., Folli C., ChiAppori A., et al. (2012). Omalizumab modulates bronchial reticular basement membrane thickness and eosinophil infiltration in severe persistent allergic asthma patients. Int. J. Immunopathol. Pharmacol. 25 (2), 475–484. doi:10.1177/039463201202500217
Richards D. B., Bareille P., Lindo E. L., Quinn D., Farrow S. N. (2010). Treatment with a peroxisomal proliferator activated receptor gamma agonist has a modest effect in the allergen challenge model in asthma: A randomised controlled trial. Respir. Med. 104 (5), 668–674. doi:10.1016/j.rmed.2009.11.006
Roberts C. R. (1995). Is asthma a fibrotic disease? CHEST 107 (3), 111S–117S. doi:10.1378/chest.107.3_Supplement.111S
Roche W. R., Williams J. H., Beasley R., Holgate S. T. (1989). Subepithelial fibrosis in the bronchi of asthmatics. Lancet Lond Engl. 1 (8637), 520–524. doi:10.1016/S0140-6736(89)90067-6
Rogers D. F. (2002). Mucoactive drugs for asthma and COPD: Any place in therapy? Expert Opin. Investig. Drugs 11 (1), 15–35. doi:10.1517/13543784.11.1.15
Roth M., Johnson P. R. A., Borger P., Bihl M. P., Rudiger J. J., King G. G., et al. (2004). Dysfunctional interaction of C/EBPalpha and the glucocorticoid receptor in asthmatic bronchial smooth-muscle cells. N. Engl. J. Med. 351 (6), 560–574. doi:10.1056/NEJMoa021660
Rousseau K., Byrne C., Griesinger G., Leung A., Chung A., Hill A. S., et al. (2007). Allelic association and recombination hotspots in the mucin gene (MUC) complex on chromosome 11p15.5. Ann. Hum. Genet. 71 (5), 561–569. doi:10.1111/j.1469-1809.2007.00374.x
Saldiva P. H., Zin W. A., Santos R. L., Eidelman D. H., Milic-Emili J. (1992). Alveolar pressure measurement in open-chest rats. J. Appl. Physiol. Bethesda Md 1985 72 (1), 302–306. doi:10.1152/jappl.1992.72.1.302
Sandhu M. S., Dimov V., Sandhu A. K., Walters R. W., Wichman T., Casale T. (2012). The use of the peroxisome proliferator-activated receptors γ agonist rosiglitazone to treat airway hyperreactivity. Ann. Allergy Asthma Immunol. 109 (1), 75–77. doi:10.1016/j.anai.2012.05.001
Saunders R., Kaul H., Berair R., Gonem S., Singapuri A., Sutcliffe A. J., et al. (2019). DP2 antagonism reduces airway smooth muscle mass in asthma by decreasing eosinophilia and myofibroblast recruitment. Sci. Transl. Med. 11 (479), eaao6451. doi:10.1126/scitranslmed.aao6451
Sears M. R., Greene J. M., Willan A. R., Wiecek E. M., Taylor D. R., Flannery E. M., et al. (2003). A longitudinal, population-based, cohort study of childhood asthma followed to adulthood. N. Engl. J. Med. 349 (15), 1414–1422. doi:10.1056/NEJMOA022363
Seow C. Y., Schellenberg R. R., Paré P. D. (1998). Structural and functional changes in the airway smooth muscle of asthmatic subjects. Am. J. Respir. Crit. Care Med. 158, S179–S186. doi:10.1164/ajrccm.158.supplement_2.13tac160
Shebani E., Shahana S., Janson C., Roomans G. M.BHR group (2005). Attachment of columnar airway epithelial cells in asthma. Tissue Cell 37 (2), 145–152. doi:10.1016/j.tice.2004.12.002
Shimizu T., Kato M., Mochizuki H., Tokuyama K., Morikawa A., Kuroume T. (1994). Roxithromycin reduces the degree of bronchial hyperresponsiveness in children with asthma. CHEST 106 (2), 458–461. doi:10.1378/chest.106.2.458
Shofer S., Badea C., Auerbach S., Schwartz D. A., Johnson G. A. (2007). A micro-computed tomography-based method for the measurement of pulmonary compliance in healthy and bleomycin-exposed mice. Exp. Lung Res. 33 (3-4), 169–183. doi:10.1080/01902140701364458
Shrine N., Portelli M. A., John C., Soler Artigas M., Bennett N., Hall R., et al. (2019). Moderate-to-severe asthma in individuals of European ancestry: A genome-wide association study. Lancet Respir. Med. 7 (1), 20–34. doi:10.1016/S2213-2600(18)30389-8
Snelgrove R. J., Patel D. F. (2019). Zooming into the matrix: Using nonlinear optical microscopy to visualize collagen remodeling in asthmatic airways. Am. J. Respir. Crit. Care Med. 200 (4), 403–405. doi:10.1164/rccm.201904-0722ED
Stamatiou R., Paraskeva E., Boukas K., Gourgoulianis K. I., Molyvdas P. A., Hatziefthimiou A. A. (2009). Azithromycin has an antiproliferative and autophagic effect on airway smooth muscle cells. Eur. Respir. J. 34 (3), 721–730. doi:10.1183/09031936.00089407
Stamatiou R., Boukas K., Paraskeva E., Molyvdas P. A., Hatziefthimiou A. (2010). Azithromycin reduces the viability of human bronchial smooth muscle cells. J. Antibiot. (Tokyo) 63 (2), 71–75. doi:10.1038/ja.2009.125
Stern E. J., Frank M. S. (1994). Small-airway diseases of the lungs: Findings at expiratory CT. Am. J. Roentgenol. 163 (1), 37–41. doi:10.2214/ajr.163.1.8010242
Strachan D. P., Butland B. K., Anderson H. R. (1996). Incidence and prognosis of asthma and wheezing illness from early childhood to age 33 in a national British cohort. BMJ 312 (7040), 1195–1199. doi:10.1136/bmj.312.7040.1195
Symmes B. A., Stefanski A. L., Magin C. M., Evans C. M. (2018). Role of mucins in lung homeostasis: Regulated expression and biosynthesis in health and disease. Biochem. Soc. Trans. 46 (3), 707–719. doi:10.1042/BST20170455
Tanaka H., Yamada G., Saikai T., Hashimoto M., Tanaka S., Suzuki K., et al. (2003). Increased airway vascularity in newly diagnosed asthma using a high-magnification bronchovideoscope. Am. J. Respir. Crit. Care Med. 168 (12), 1495–1499. doi:10.1164/RCCM.200306-727OC
Tang M., Elicker B. M., Henry T., Gierada D. S., Schiebler M. L., Huang B. K., et al. (2022). Mucus plugs persist in asthma, and changes in mucus plugs associate with changes in airflow over time. Am. J. Respir. Crit. Care Med. 205 (9), 1036–1045. doi:10.1164/rccm.202110-2265OC
ten Brinke A., Zwinderman A. H., Sterk P. J., Rabe K. F., Bel E. H. (2004). "Refractory" eosinophilic airway inflammation in severe asthma: Effect of parenteral corticosteroids. Am. J. Respir. Crit. Care Med. 170 (6), 601–605. doi:10.1164/rccm.200404-440OC
Thorpe C. W., Bates J. H. T. (1997). Effect of stochastic heterogeneity on lung impedance during acute bronchoconstriction: A model analysis. J. Appl. Physiol. 82 (5), 1616–1625. doi:10.1152/JAPPL.1997.82.5.1616/ASSET/IMAGES/LARGE/JAPP0553106.JPEG
Trautmann A., Kruger K., Akdis M., Muller-Wening D., Akkaya A., Brocker E. B., et al. (2005). Apoptosis and loss of adhesion of bronchial epithelial cells in asthma. Int. Arch. Allergy Immunol. 138 (2), 142–150. doi:10.1159/000088436
Trejo Bittar H. E., Doberer D., Mehrad M., Strollo D. C., Leader J. K., Wenzel S., et al. (2017). Histologic findings of severe/therapy-resistant asthma from video-assisted thoracoscopic surgery biopsies. Am. J. Surg. Pathol. 41 (2), 182–188. doi:10.1097/PAS.0000000000000777
Tsartsali L., Hislop A. A., McKay K., James A. L., Elliot J., Zhu J., et al. (2011). Development of the bronchial epithelial reticular basement membrane: Relationship to epithelial height and age. Thorax 66 (4), 280–285. doi:10.1136/thx.2010.149799
Tsurikisawa N., Oshikata C., Tsuburai T., Saito H., Sekiya K., Tanimoto H., et al. (2010). Bronchial hyperresponsiveness to histamine correlates with airway remodelling in adults with asthma. Respir. Med. 104 (9), 1271–1277. doi:10.1016/j.rmed.2010.03.026
Tunon-de-Lara J. M., Laurent F., Giraud V., Perez T., Aguilaniu B., Meziane H., et al. (2007). Air trapping in mild and moderate asthma: Effect of inhaled corticosteroids. J. Allergy Clin. Immunol. 119 (3), 583–590. doi:10.1016/j.jaci.2006.11.005
Uhlík J., Vajner L., Adášková J., Konrádová V. (2007). Effect of inhalation of single dose of beclomethasone on airway epithelium. Ultrastruct. Pathol. 31 (3), 221–232. doi:10.1080/01913120701425951
Vock C., Hauber H. P., Wegmann M. (2010). The other T helper cells in asthma pathogenesis. J. Allergy 2010, 519298. doi:10.1155/2010/519298
Vos T., Lim S. S., Abbafati C. (2020). Global burden of 369 diseases and injuries in 204 countries and territories, 1990–2019: A systematic analysis for the global burden of disease study 2019. Lancet 396 (10258), 1204–1222. doi:10.1016/S0140-6736(20)30925-9
Vrugt B., Wilson S., Bron A., Holgate S. T., Djukanovic R., Aalbers R. (2000). Bronchial angiogenesis in severe glucocorticoid-dependent asthma. Eur. Respir. J. 15 (6), 1014–1021. doi:10.1034/j.1399-3003.2000.01507.x
Wan H., Kaestner K. H., Ang S. L., Ikegami M., Finkelman F. D., Stahlman M. T., et al. (2004). Foxa2 regulates alveolarization and goblet cell hyperplasia. Development 131 (4), 953–964. doi:10.1242/dev.00966
Wang K. C. W., Donovan G. M., James A. L., Noble P. B. (2020). Asthma: Pharmacological degradation of the airway smooth muscle layer. Int. J. Biochem. Cell Biol. 126, 105818. doi:10.1016/j.biocel.2020.105818
Wanner A., Chediak A. D., Csete M. E. (1990). Airway mucosal blood flow: Response to autonomic and inflammatory stimuli. Eur. Respir. J. Suppl. 12, 618s–623s.
Ward C., Pais M., Bish R., ReiD D., Feltis B., Johns D., et al. (2002). Airway inflammation, basement membrane thickening and bronchial hyperresponsiveness in asthma. Thorax 57 (4), 309–316. doi:10.1136/thorax.57.4.309
Ward J. E., Gould H., Harris T., Bonacci J. V., Stewart A. G. (2004). PPAR gamma ligands, 15-deoxy-delta12, 14-prostaglandin J2 and rosiglitazone regulate human cultured airway smooth muscle proliferation through different mechanisms. Br. J. Pharmacol. 141 (3), 517–525. doi:10.1038/sj.bjp.0705630
Wark P. A. B., Johnston S. L., Bucchieri F., Powell R., Puddicombe S., Laza-Stanca V., et al. (2005). Asthmatic bronchial epithelial cells have a deficient innate immune response to infection with rhinovirus. J. Exp. Med. 201 (6), 937–947. doi:10.1084/jem.20041901
Wenzel S. E., Vitari C. A., Shende M., Strollo D. C., Larkin A., Yousem S. A. (2012). Asthmatic granulomatosis: A novel disease with asthmatic and granulomatous features. Am. J. Respir. Crit. Care Med. 186 (6), 501–507. doi:10.1164/rccm.201203-0476OC
West E. E., Kashyap M., Leonard W. J. (2012). TSLP: A key regulator of asthma pathogenesis. Drug Discov. Today Dis. Mech. 9 (3-4), e83–e88. doi:10.1016/j.ddmec.2012.09.00310.1016/j.ddmec.2012.09.003
Whiting P. J. (2003). The GABAA receptor gene family: New opportunities for drug development. Curr. Opin. Drug Discov. Devel 6 (5), 648–657.
Wielpütz M. O., Eichinger M., Zhou Z., Leotta K., Hirtz S., Bartling S. H., et al. (2011). In vivo monitoring of cystic fibrosis-like lung disease in mice by volumetric computed tomography. Eur. Respir. J. 38 (5), 1060–1070. doi:10.1183/09031936.00149810
Wiggs B. R., Hrousis C. A., Drazen J. M., Kamm R. D. (1997). On the mechanism of mucosal folding in normal and asthmatic airways. J. Appl. Physiol. 83 (6), 1814–1821. doi:10.1152/jappl.1997.83.6.1814
Wilson J. W., Li X. (1997). The measurement of reticular basement membrane and submucosal collagen in the asthmatic airway. Clin. Exp. Allergy 27 (4), 363–371. doi:10.1111/j.1365-2222.1997.tb00720.x
Wilson J. W., Robertson C. F. (2002). Angiogenesis in paediatric airway disease. Paediatr. Respir. Rev. 3 (3), 219–229. doi:10.1016/s1526-0542(02)00200-2
Witt C. A., Sheshadri A., Carlstrom L., Tarsi J., Kozlowski J., Wilson B., et al. (2014). Longitudinal changes in airway remodeling and air trapping in severe asthma. Acad. Radiol. 21 (8), 986–993. doi:10.1016/j.acra.2014.05.001
Wurnig M. C., Tsushima Y., Weiger M., Jungraithmayr W., Boss A. (2014). Assessing lung transplantation ischemia-reperfusion injury by microcomputed tomography and ultrashort echo-time magnetic resonance imaging in a mouse model. Invest. Radiol. 49 (1), 23–28. doi:10.1097/RLI.0b013e3182a53111
Xiang Y. Y., Wang S., Liu M., Hirota J. A., Li J., Ju W., et al. (2007). A GABAergic system in airway epithelium is essential for mucus overproduction in asthma. Nat. Med. 13 (7), 862–867. doi:10.1038/nm1604
Xiao C., Puddicombe S. M., Field S., Haywood J., Broughton-Head V., Puxeddu I., et al. (2011). Defective epithelial barrier function in asthma. J. Allergy Clin. Immunol. 128 (3), 549–556. doi:10.1016/j.jaci.2011.05.038
Yoshida Y., Takaku Y., Nakamoto Y., Takayanagi N., Yanagisawa T., Takizawa H., et al. (2020). Changes in airway diameter and mucus plugs in patients with asthma exacerbation. PLoS ONE 15 (2), e0229238. doi:10.1371/JOURNAL.PONE.0229238
Zastrzeżyńska W., Przybyszowski M., Bazan-Socha S., Gawlewicz-Mroczka A., Sadowski P., Okon K., et al. (2020). Omalizumab may decrease the thickness of the reticular basement membrane and fibronectin deposit in the bronchial mucosa of severe allergic asthmatics. J. Asthma Off. J. Assoc. Care Asthma 57 (5), 468–477. doi:10.1080/02770903.2019.1585872
Keywords: asthma, airway remodeling, airway heterogeneity, computed tomgraphy (CT), magnetic resonance imaging (MRI), mucus plug, asthma therapeutics
Citation: Hsieh A, Assadinia N and Hackett T-L (2023) Airway remodeling heterogeneity in asthma and its relationship to disease outcomes. Front. Physiol. 14:1113100. doi: 10.3389/fphys.2023.1113100
Received: 01 December 2022; Accepted: 05 January 2023;
Published: 19 January 2023.
Edited by:
Giuseppe Guida, University of Turin, ItalyReviewed by:
Thomas Trian, Université de Bordeaux, FranceChristopher D. Pascoe, University of Manitoba, Canada
Copyright © 2023 Hsieh, Assadinia and Hackett. This is an open-access article distributed under the terms of the Creative Commons Attribution License (CC BY). The use, distribution or reproduction in other forums is permitted, provided the original author(s) and the copyright owner(s) are credited and that the original publication in this journal is cited, in accordance with accepted academic practice. No use, distribution or reproduction is permitted which does not comply with these terms.
*Correspondence: Tillie-Louise Hackett, dGlsbGllLmhhY2tldHRAaGxpLnViYy5jYQ==