- Department of Poultry Science, University of Georgia, Athens, GA, United States
Commercial laying hens can produce one egg approximately every 24 h. During this process, regulatory systems that control vitamin D3 metabolism, calcium and phosphorus homeostasis, and intestinal uptake of these minerals work in concert to deliver components required for eggshell calcification and bone mineralization. Commercial production cycles have been extended in recent years to last through 100 weeks of age, and older hens often exhibit an increased prevalence of skeletal fractures and poor eggshell quality. Issues such as these arise, in part, through imbalances that occur in calcium and phosphorus utilization as hens age. As a result, an in-depth understanding of the mechanisms that drive calcium and phosphorus uptake and utilization is required to develop solutions to these welfare and economic challenges. This paper reviews factors that influence calcium and phosphorus homeostasis in laying hens, including eggshell formation and development and roles of cortical and medullary bone. Metabolism and actions of vitamin D3 and physiological regulation of calcium and phosphorus homeostasis in key tissues are also discussed. Areas that require further research in avian species, such as the role of fibroblast growth factor 23 in these processes and the metabolism and action of bioactive vitamin D3, are highlighted and the importance of using emerging technologies and establishing in vitro systems to perform functional and mechanistic studies is emphasized.
1 Introduction
As the global population grows, there is increased demand for affordable, high-quality, and sustainable protein sources like table eggs. Commercial laying hens have been selected to increase eggs produced per hen lifetime, with production cycles now lasting past 100 weeks of age. Economic and sustainability benefits of extended lay persistency include decreased cost and environmental impact on a per-egg basis (Bain et al., 2016), but there are challenges associated with egg quality and bird welfare as hens age.
Older hens often produce larger, weak-shelled eggs (Al-Batshan et al., 1994) and exhibit compromised skeletal structure. Efficiency of intestinal calcium absorption decreases with age (Diana et al., 2021), leading to increased reliance on bone-derived calcium contributing to fractures (Gregory and Wilkins, 1989). Elucidating physiological mechanisms responsible for the uptake and utilization of calcium and phosphorus throughout the hen’s productive lifecycle will provide insights that can be used to develop strategies limiting economic losses to producers and improving animal welfare.
2 Egg formation
Commercial laying hens produce an egg approximately every 24 h (Nys and Guyot, 2011) and must efficiently regulate calcium and phosphorus utilization for eggshell calcification and cuticle formation, respectively (Cusack et al., 2003). Ovulation occurs 15–75 min after oviposition, or egg-laying (Sturkie and Mueller, 1976), and the follicle resides in the infundibulum for under 30 min (Sah and Mishra, 2018). It continues into the magnum where albumen is added over the next 3.25–3.5 h (Nys and Guyot, 2011) and enters the isthmus where inner and outer shell membranes are deposited around the albumen (Warren and Scott, 1935). Organic eggshell matrix proteins (e.g. ovalbumins, osteopontins, ovocleidins, ovocalyxins) and calcium carbonate are deposited onto the outer shell membrane (Hincke et al., 2010) in the shell gland, and the eggshell forms over the final 19–20 h (Nys and Guyot, 2011; Gautron et al., 2021).
As previously described (Nys and Guyot, 2011; Gautron et al., 2021), the eggshell develops as distinct mamillary, palisade, and cuticle layers deposited from interior to exterior. During mineralization of the mammillary and palisade layers, deposition of amorphous calcium carbonate is followed by its transformation into calcite crystals (Rodriguez-Navarro et al., 2015). Initially, the mamillary layer forms at nucleation sites laid on the outer shell membrane between 5–6 h post-oviposition (HPOP) and the mamillary core develops between 7–10 HPOP. Large calcite crystal units form the columnar palisade layer between 10–22 HPOP, and the cuticle forms an organic film preventing bacterial penetration of the egg about 2 h before oviposition. A calcium and phosphorus-rich hydroxyapatite [Ca10(PO4)6(OH)2] crystal layer lies just internal to the cuticle (Wedral et al., 1974; Cusack et al., 2003). Since phosphorus is a potent inhibitor of calcite formation (Bachra et al., 1963; Simkiss, 1964), some authors speculate that these crystals (Dennis et al., 1996) or the secretion of phosphate-containing organic eggshell constituents towards the end of shell formation (Nys et al., 1991) may be involved in terminating calcification.
3 Bone development and remodeling
Since most eggshell calcification takes place in the dark when dietary calcium is largely unavailable, hens mobilize approximately 20%–40% of calcium required for eggshell formation from bone (Comar and Driggers, 1949). Structural cortical and trabecular bone with highly organized hydroxyapatite crystals is formed during embryonic and juvenile development. After structural bone deposition subsides (Hudson et al., 1993), increased circulating estrogen at the onset of sexual maturity around 18 weeks of age leads to development of medullary bone in pneumatic and long bones (Whitehead, 2004). Medullary bone is highly vascularized with randomly orientated hydroxyapatite crystals (Dacke et al., 1993), allowing for rapid anabolism and catabolism of hydroxyapatite during egg formation. Since hydroxyapatite is composed of calcium and phosphorus, bone resorption releases both minerals into circulation as ionized calcium (iCa2+) and inorganic phosphate [PO43− (Pi)] that must be utilized for shell formation or excreted.
Medullary bone undergoes remineralization when eggshell calcification is not occurring (Wilson and Duff, 1990; Kerschnitzki et al., 2014) and is resorbed during eggshell calcification (Van de Velde et al., 1984b) through increased osteoclast activity driven by parathyroid hormone (PTH) and the bioactive form of vitamin D3, 1,25-dihydroxycholecalciferol [1,25(OH)2D3] (Taylor and Belanger, 1969). When PTH binds PTH receptor 1 (PTH1R) on osteocytes (Silve et al., 1982; Zhao et al., 2002), receptor activator of nuclear factor-kappa B ligand (RANKL) is secreted and interacts with receptor activator of nuclear factor-kappa B (RANK) on osteoclasts, stimulating bone resorption. Additionally, PTH increases osteoclast vacuolar-type adenosine triphosphatase (V-ATPase) activity, causing intracellular acidification required for bone breakdown (Liu et al., 2016). Osteoclast activity increases nine-fold during shell calcification (Van de Velde et al., 1984b), and osteoporosis can develop when osteoclasts resorb structural bone once medullary bone is depleted. Dysregulation of medullary bone remodeling may contribute to development of osteoporosis in aged hens, which exhibit increased medullary bone expression of the resorption marker carbonic anhydrase 2 (CA2) and vitamin D3 receptor (VDR), as well as reduced expression of accretion proteins like collagen type 1 alpha 1 (COL1A1), relative to younger hens (Gloux et al., 2020b).
4 Vitamin D3 metabolism and mechanism of action
Skeletal integrity and eggshell quality depend on 1,25(OH)2D3 because of its role in regulating calcium and phosphorus homeostasis. Dietary vitamin D3 is constitutively hydroxylated in the liver by a 25-hydroxylase enzyme encoded by the CYP2R1 gene (Watanabe et al., 2013), with >90% converted into 25(OH)D3 (Heaney et al., 2008; San Martin Diaz, 2018). A second, more tightly regulated hydroxylation occurs in the kidney at the 1α-carbon to form 1,25(OH)2D3 (Jones et al., 1998). In mammals and fish, this is carried out by an enzyme encoded by CYP27B1 (Monkawa et al., 1997; Shinki et al., 1997; Chun et al., 2014); however, this gene has not been identified in chickens and the enzyme responsible is currently unknown despite recent publications that have reported measuring expression of CYP27B1 mRNA or an equivalent (Shanmugasundaram and Selvaraj, 2012; Gloux et al., 2020a; Gloux et al., 2020b; Yan et al., 2022). Investigation into transcripts amplified reveals these are an enzyme involved in retinoic acid metabolism (CYP27C1) or one identified as vitamin D3 hydroxylase-associated protein (Ettinger et al., 1994; Ettinger and DeLuca, 1995), neither of which have demonstrable 1α-hydroxylase activity. PTH stimulates 1α-hydroxylation of vitamin D3 when circulating iCa2+ and 1,25(OH)2D3 are low; however, the efficiency of this may decrease with age (Abe et al., 1982; Gloux et al., 2020b). During periods of elevated circulating 1,25(OH)2D3, 1α-hydroxylase is inhibited and 24-hydroxylase, encoded for by CYP24A1, is upregulated. The 24-hydroxylase enzyme inactivates 25(OH)D3 by producing biologically inert 24,25(OH)2D3 or 1,24,25(OH)3D3 (Holick et al., 1973; Omdahl et al., 2002), thereby preventing excessive bone resorption and intestinal calcium absorption. Hydroxylation of 25(OH)D3 into either active or inactive metabolites provides an additional level of control by fine-tuning the availability of this hormone.
Vitamin D3 affects calcium and phosphorus homeostasis through its influence on expression and activity of transport and chaperone molecules for these minerals. When bound by 1,25(OH)2D3, VDR acts as a ligand-activated transcription factor that enters the nucleus to form a heterodimeric complex with retinoid-X-receptor alpha (RXRA) or gamma (RXRG) and binds vitamin D3 response elements (VDRE) in regulatory regions of vitamin D3-responsive genes (Bikle, 2014). Not all tissues respond to 1,25(OH)2D3 in the same way. For example, shell gland calbindin D-28k (CALB1) expression does not appear to be influenced by 1,25(OH)2D3 (Bar et al., 1977), unlike that in the kidney and small intestine (Taylor and Wasserman, 1972). It may be under the control of estrogen (Nys et al., 1992; Corradino et al., 1993), driven by half-palindromic estrogen response elements in the CALB1 promoter as has been shown in mice (Gill and Christakos, 1995), and intracellular calcium levels (Corradino, 1993). Since CALB1 in shell gland, intestine, and kidney share the same electrophoretic mobility, amino acid composition, and immunoreactivity, it is likely the same protein (Fullmer et al., 1976); however, estrogen receptor rather than VDR could be a key regulatory protein driving its expression in the shell gland.
5 Calcium homeostasis and transport
Regulation of calcium homeostasis is required to maintain the daily flux of this mineral in laying hens. The highest demand occurs when the eggshell is actively calcifying during the nocturnal fast, and hens must rely on reduced intestinal pH to solubilize coarse limestone retained in the gizzard (Scanes et al., 1987). This occurs through stimulation of H+/K+-ATPase activity in the proventriculus (Guinotte et al., 1995) and subsequent secretion of hydrochloric acid (Guinotte et al., 1993).
During eggshell calcification, decreased circulating iCa2+ due to high demand by the shell gland (Parsons and Combs, 1980) is detected by calcium-sensing receptor (CASR) (Hofer and Brown, 2003) and leads to PTH secretion from the parathyroid gland (Van de Velde et al., 1984a; Singh et al., 1986). PTH rectifies circulating iCa2+ back to its homeostatic range by stimulating bone resorption (Taylor and Belanger, 1969) and increasing 1,25(OH)D3 production in the kidney (Brenza and DeLuca, 2000); 1,25(OH)D3 works to increase calcium absorption from the small intestine (Spencer et al., 1978; Chandra et al., 1990) and reabsorption in the kidney (Jande et al., 1981).
Calcitonin (CALC), produced within ultimobranchial bodies near the thyroid gland (Copp et al., 1967; Kraintz and Intscher, 1969), may reduce iCa2+ in chickens (Luck et al., 1979), and expression of CALC receptor (CALCR) in shell gland, kidney, and bone of laying hens (Yasuoka et al., 1998; Ieda et al., 2001) suggests it could play a role in regulating calcium homeostasis. However, unlike in mammals, CALC does not influence avian osteoclast activity under normal physiological conditions (Nicholson et al., 1987; Eliam et al., 1988), nor does it appear to affect renal cyclic adenosine monophosphate formation in chickens or pigeons (Dousa, 1974). This implies that avian CALCR could use alternative intracellular signaling pathways or that CALC does not have the same effect on bone as it does in mammals. At present, there is limited evidence that CALC strongly influences calcium homeostasis in birds, suggesting it may not be an important regulator of calcium availability for egg production.
Calcium absorption from the small intestine appears to fluctuate throughout the daily egg formation cycle (Hurwitz and Bar, 1969; Hurwitz et al., 1973) and is thought to occur primarily in the duodenum and jejunum, with smaller amounts absorbed in the ileum (Hurwitz and Bar, 1965; Hurwitz and Bar, 1968). Intestinal calcium uptake occurs through active transcellular and passive paracellular pathways. Active transcellular absorption accounts for most calcium uptake and involves ATPase plasma membrane calcium transporting 1 (ATP2B1), 2 (ATP2B2), and 4 (ATP2B4), sodium-calcium exchanger 1 (NCX1), transient receptor potential cation channel subfamilies C member 1 (TRPC1), M member 7 (TRPM7), and V member 2 (TRPV2), and CALB1 (Bar, 2009; Gloux et al., 2019). Passive paracellular calcium absorption likely takes place via tight junction proteins 1 (TJP1), 2 (TJP2), and 3 (TJP3), claudin 2 (CLDN2) and 12 (CLDN12), and occludin (OCLN) (Gloux et al., 2019; Gloux et al., 2020b). Findings suggest that intestinal capacity for calcium absorption could change with age, as expression of some transcellular (ATP2B4, TRPV2) and paracellular (TJP3, CLDN2, OCLN) transporters decreased in older hens (Gloux et al., 2020b). Calcium transport in the shell gland (Brionne et al., 2014) and kidney occurs through many of these same proteins, with the addition of transient receptor potential cation channel subfamily V member 6 (TRPV6) in the kidney (Proszkowiec-Weglarz and Angel, 2013; Gautron et al., 2021; Wang et al., 2022). This has been shown to decrease with age in hens (Gloux et al., 2020b), indicating that the calcium-handling capacity of the kidney is perturbed in older layers. In addition to the above-listed transporters, recent findings suggest vesicular transport systems may export calcium into the shell gland lumen (Stapane et al., 2020).
6 Phosphorus homeostasis and transport
Approximately 80% of phosphorus is stored in the skeleton as hydroxyapatite. It is released when bone is resorbed during eggshell calcification, and this excess Pi (Nys et al., 1986; Frost and Roland, 1990) must be excreted to negate toxic effects. Maintenance of circulating Pi occurs in the kidney, small intestine, and bone (Michigami et al., 2018) and is primarily regulated by fibroblast growth factor 23 (FGF23); however, PTH and 1,25(OH)2D3 also influence it through their actions on calcium homeostasis (Ren et al., 2020).
In mice (Perwad et al., 2005) and laying hens (Ren et al., 2017; Wang et al., 2018; Gloux et al., 2020a; Ren et al., 2020), hyperphosphatemia increases FGF23 production in bone. It has been shown to bind to one of four FGF receptors (FGFR1-4) along with the co-receptor klotho (KL) in mammals (Razzaque, 2009), and this complex induces expression of Pi transport proteins that mediate FGF23’s phosphaturic effects. Laying hens express FGF23 mRNA in both medullary and structural bone (Hadley et al., 2016; Wang et al., 2018), and increases in its expression occur as they age (Gloux et al., 2020b). Furthermore, hens exhibit FGFR1-4 and KL mRNA expression in the kidney, intestine, and bones (Ren et al., 2020). Immunoneutralization of FGF23 in laying hens led to increased plasma Pi and bone ash under phosphorus-deficient conditions (Bobeck et al., 2012; Ren et al., 2017), and limiting dietary Pi in laying hens reduced circulating Pi, suppressed bone FGF23 mRNA, circulating FGF23, and renal sodium-dependent Pi transporter IIa (NaPiIIa) expression, and induced duodenal sodium-dependent Pi transporter IIb (NaPiIIb) expression (Ren et al., 2020). These changes corresponded with reduced phosphorus excretion and increased calcium excretion. Studies conducted in mammals have found that FGF23 directly inhibited PTH secretion (Ben-Dov et al., 2007), decreased renal Pi transporter 2 (PiT-2) expression (Tomoe et al., 2009), and limited 1,25(OH)2D3 production in the kidney, in part through upregulation of 24-hydroxylase (Perwad et al., 2007). In hens, similarities exist whereby elevated medullary FGF23 mRNA during eggshell calcification was followed by increased renal mRNA for CYP24A1 after oviposition, which may have led to observed reductions in 1,25(OH)2D3 (Gloux et al., 2020a).
In birds, 1,25(OH)2D3 appears to directly stimulate renal Pi reabsorption in the short-term and inhibit it in the long-term (Liang et al., 1982; Liang et al., 1984). Renal Pi reabsorption was decreased, and therefore Pi excretion increased, by PTH (Wideman and Braun, 1981). The capacity of the kidney to regulate Pi balance could change with age, as expression of NaPiIIa and Pi transporter 1 (PiT-1) in kidney decreased in older hens (Gloux et al., 2020b). Since PTH stimulates production of 1,25(OH)2D3, it indirectly increases Pi absorption from the intestine (Liao et al., 2017). Intestinal Pi uptake in chickens is thought to be mediated by PiT-1, PiT-2, NaPiIIa, and NaPiIIb (Yan et al., 2007; Huber et al., 2015; Li et al., 2018), with NaPiIIb as the primary transporter in the duodenum and jejunum and PiT-1 as the primary transporter in the ileum (Gloux et al., 2019).
7 Discussion
This review investigates physiological mechanisms influencing calcium and phosphorus utilization in laying hens during egg production (Figure 1). Age-dependent changes in levels of FGF23, 1,25(OH)2D3, and several calcium and phosphorus transporters in the intestine and kidney suggest that the ability of hens to maintain adequate mineral balance for optimal shell strength and bone health is compromised during extended lay. This leads to deterioration of structural bone when the rate of medullary bone resorption required for eggshell calcification exceeds that of remineralization during periods outside eggshell development, predisposing hens to fractures that negatively impact their welfare and reduce egg production in an age-dependent fashion (Rufener et al., 2019). To maintain healthy, high-producing hens throughout extended production, skeletal development should be prioritized during rearing to ensure adequate deposition of structural bone prior to initiation of medullary bone accretion.
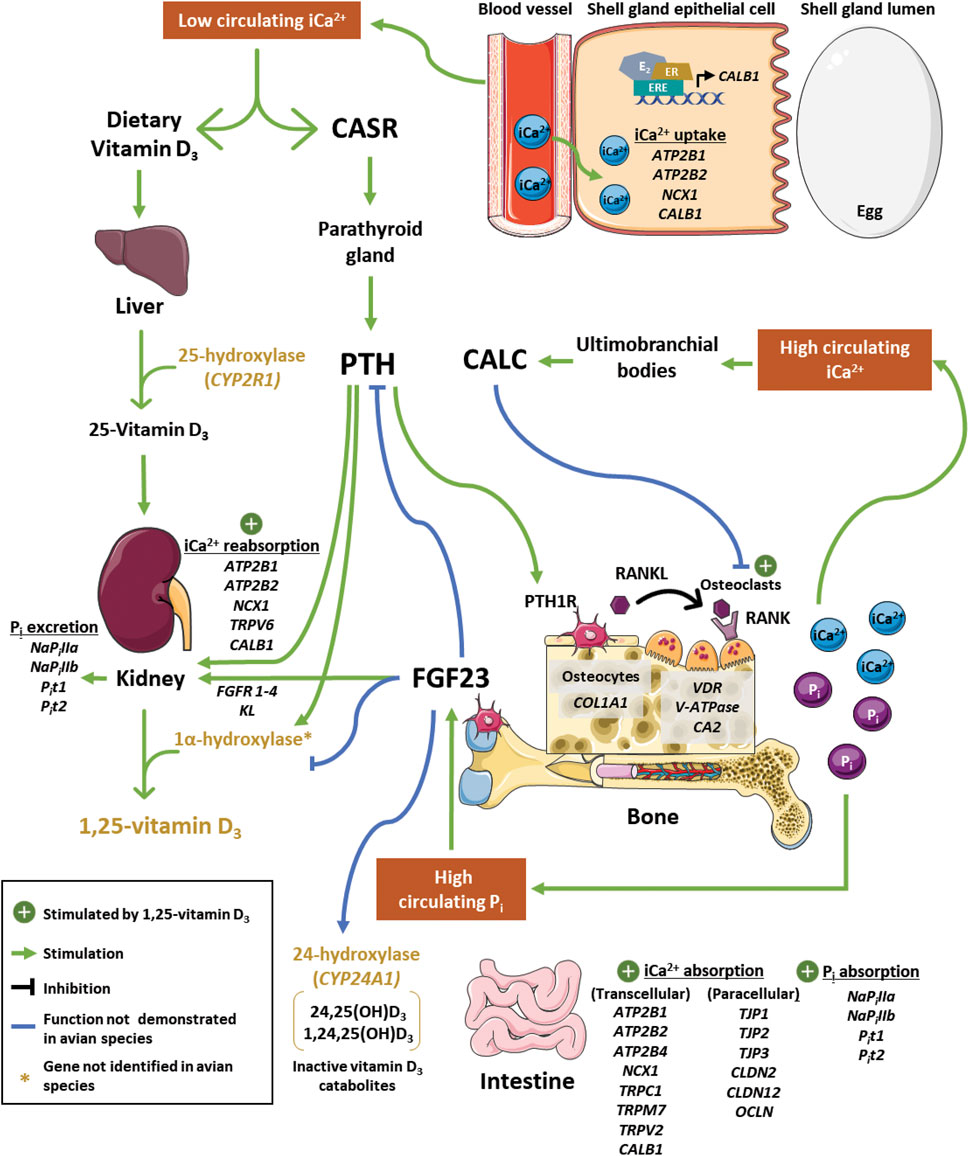
FIGURE 1. Regulation of calcium and phosphorus homeostasis during eggshell mineralization in laying hens. During eggshell calcification, high demand for calcium decreases circulating ionized calcium (iCa2+). Low iCa2+ is detected by calcium-sensing receptor (CASR), which stimulates parathyroid hormone (PTH) secretion from the parathyroid gland. Secreted PTH binds to PTH receptor 1 (PTH1R) on osteocytes to promote interaction between receptor activator of nuclear factor-kappa B (RANK) and RANK ligand (RANKL) on the osteoclast surface. This induces vacuolar-type adenosine triphosphatase (V-ATPase) production to facilitate bone resorption alongside carbonic anhydrase 2 (CA2). In contrast, bone accretion is facilitated by deposition of matrix proteins such as collagen type 1 alpha 1 (COL1A1). In the kidney, PTH stimulates inorganic phosphate (Pi) excretion and upregulates production of 1,25(OH)2D3. Bioactive 1,25(OH)2D3, which binds to vitamin D3 receptor (VDR), stimulates osteoclast activity, calcium transport in the kidney, and calcium and phosphorus uptake in the intestine. Impacts of 1,25(OH)2D3 in the shell gland and on paracellular intestinal calcium uptake still need to be elucidated. Transcellular transport of calcium in these tissues is thought to occur through ATPase plasma membrane calcium transporting 1, 2, and 4 (ATP2B1, ATB2B2, ATP2B4; intestine only), sodium-calcium exchanger 1 (NCX1), calbindin-28K (CALB1), transient receptor potential cation channels subfamily C member 1 (TRPC1; intestine only), transient receptor potential cation channels subfamily M member 7 (TRPM7; intestine only), and transient receptor potential cation channel subfamily V member two and six (TRPV2, intestine only; TRPV6, kidney only). Paracellular transport in the intestine is achieved by tight junction proteins 1, 2, and 3 (TJP1, TJP2, TJP3), claudin 2 and 12 (CLDN2, CLDN12) and occludin (OCLN). Transport of phosphorus in these tissues is thought to occur by sodium-dependent phosphorus transporters IIa and IIb (NaPiIIa and NaPiIIb) and sodium-dependent inorganic phosphorus transporters 1 and 2 (Pit1 and Pit2). Shell gland calcium transport by CALB1 may be under the control of estradiol (E2) through estrogen receptor (ER) interaction with estrogen-response elements (EREs) in its promoter region. Bone breakdown releases Pi into circulation, which induces production of fibroblast growth factor 23 (FGF23). In chickens and mammals, this peptide stimulates renal phosphorus excretion, which has been shown to be mediated through its binding to FGF23 receptors (FGFR1, FGFR2, FGFR3, FGFR4) and co-receptor klotho (KL) in mammals. In mice, FGF23 has also been shown to exhibit negative feedback on PTH and 1α-hydroxylase activity, as well as stimulate 24-hydroxylase activity. During periods of elevated iCa2+, calcitonin (CALC) is secreted from cells in ultimobranchial bodies to inhibit osteoclast activity in mammals, but its effects in birds are unclear. Further investigation into several of these processes and how transporters function in a tissue-specific manner is required to determine their role in calcium and phosphorus homeostasis in chickens. Parts of the figure were drawn by using pictures from servier medical art, licensed under a creative commons attribution 3.0 unported license (https://creativecommons.org/licenses/by/3.0/).
Improvements in laying hen skeletal health require an in-depth understanding of regulatory systems driving calcium and phosphorus utilization and how they change with age. Further research on how FGF23 influences PTH secretion, vitamin D3 metabolism, and other aspects of calcium and phosphorus homeostasis in birds is necessary. Though a role for FGF23 in regulating Pi homeostasis in layers has been supported by the findings described above, functional and mechanistic studies demonstrating its direct involvement are limited. As there are differences in medullary bone expression of FGF23 mRNA with age (Gloux et al., 2020b), and FGF23 appears to influence phosphorus and calcium balance (Bobeck et al., 2012; Ren et al., 2017; Ren et al., 2020), understanding effects of FGF23 on mineral homeostasis and how to manage changes across the production cycle is crucial for maintaining skeletal health and egg production throughout extended lay.
A second area needing further elucidation is the metabolism and action of vitamin D3. The gene encoding 1-α hydroxylase has not been identified in avian species, hindering mechanistic studies of its activity. Characterization of CYP27B1 or a functional equivalent would provide valuable insights into ways that vitamin D3 metabolism could be harnessed to improve eggshell integrity and skeletal welfare in layers, including using selection strategies for hens that exhibit stronger bones and eggshells. Furthermore, the influence of 1,25(OH)2D3 on shell gland calcium transport has been questioned due to unresponsiveness of typical 1,25(OH)2D3-dependent proteins (Bar et al., 1977; Bar, 2008); additional studies are needed to confirm if this applies to other aspects of shell gland calcium transport. This is especially important, as regulation of ionic calcium transfer into the shell gland lumen is poorly understood (Nys et al., 2022) despite it being a limiting factor in calcium supply to the eggshell (Cohen et al., 1978), so alterations in this process with age likely contribute to decreased shell quality in older hens.
Though a better picture of laying hen calcium, phosphorus, and vitamin D3 metabolism has emerged in recent years, critical knowledge gaps exist and much of our understanding of these homeostatic mechanisms is derived from mammalian research. However, hens undergo additional biological processes such as development and maintenance of medullary bone and eggshell calcification, so direct inferences from mammals to birds may be flawed. Availability of the chicken genome in conjunction with “omics” approaches should help identify relevant gene networks across tissues that are involved in these processes, allowing development of testable hypotheses that can be used to discern functionality where it is lacking. Establishment of reliable in vitro models for bone, kidney, and shell gland and validated assays for functional proteins would greatly facilitate fundamental, mechanistic studies on these systems. This is essential for generating successful nutritional and genetic management strategies that prioritize skeletal welfare throughout the productive lifecycle of the hen.
Author contributions
MS-B drafted the manuscript; RG assisted with drafting the manuscript and prepared Figure 1; LE conceptualized the review, edited the manuscript and Figure 1, and obtained funding. All authors have read and approved the submitted version of the manuscript.
Funding
Graduate student support for MS-B and RG was provided to LE by H&N International (Cuxhaven, Germany) and Iluma Alliance (Durham, NC, United States).
Acknowledgments
The authors thank Brett Marshall, Lauren Vaccaro, Charles Meeks, Colin Barcelo, and Shailes Bhattrai for initial editing of this review.
Conflict of interest
The authors declare that the research was conducted in the absence of any commercial or financial relationships that could be construed as a potential conflict of interest.
Publisher’s note
All claims expressed in this article are solely those of the authors and do not necessarily represent those of their affiliated organizations, or those of the publisher, the editors and the reviewers. Any product that may be evaluated in this article, or claim that may be made by its manufacturer, is not guaranteed or endorsed by the publisher.
References
Abe E., Horikawa H., Masumura T., Sugahara M., Kubota M., Suda T. (1982). Disorders of cholecalciferol metabolism in old egg-laying hens. J. Nutr. 112 (3), 436–446. doi:10.1093/jn/112.3.436
Al-Batshan H. A., Scheideler S. E., Black B. L., Garlich J. D., Anderson K. E. (1994). Duodenal calcium uptake, femur ash, and eggshell quality decline with age and increase following molt. Poult. Sci. 73 (10), 1590–1596. doi:10.3382/ps.0731590
Bachra B. N., Trautz O. R., Lawrence Simon S. (1963). Precipitation of calcium carbonates and phosphates. I. Spontaneous precipitation of calcium carbonates and phosphates under physiological conditions. Biochem. Bioph. 103, 124–138. doi:10.1016/0003-9861(63)90018-3
Bain M. M., Nys Y., Dunn I. C. (2016). Increasing persistency in lay and stabilizing egg quality in longer laying cycles. What are the challenges? Br. Poul. Sci. 57 (3), 330–338. doi:10.1080/00071668.2016.1161727
Bar A. (2008). Calcium homeostasis and vitamin D metabolism and expression in strongly calcifying laying birds. Comp. Biochem. Physiol. 151 (4), 477–490. doi:10.1016/j.cbpa.2008.07.006
Bar A. (2009). Calcium transport in strongly calcifying laying birds: Mechanisms and regulation. Comp. Biochem. Physiol. 152 (4), 447–469. doi:10.1016/j.cbpa.2008.11.020
Bar A., Cohen A., Eisner U., Riesenfeld G., Hurwitz S. (1977). Differential response of calcium transport systems in laying hens to exogenous and endogenous changes in vitamin D status. J. Nutr. 108 (8), 1322–1328. doi:10.1093/jn/108.8.1322
Ben-Dov I. Z., Galitzer H., Lavi-Moshayoff V., Goetz R., Kuro-o M., Mohammadi M., et al. (2007). The parathyroid is a target organ for FGF23 in rats. J. Clin. Invest. 117 (12), 4003–4008. doi:10.1172/Jci32409
Bikle D. D. (2014). Vitamin D metabolism, mechanism of action and clinical applications. Chem. Biol. 21 (3), 319–329. doi:10.1016/j.chembiol.2013.12.016
Bobeck E. A., Burgess K. S., Jarmes T. R., Piccione M. L., Cook M. E. (2012). Maternally-derived antibody to fibroblast growth factor-23 reduced dietary phosphate requirements in growing chicks. Biochem. Bioph. Res. Co. 420 (3), 666–670. doi:10.1016/j.bbrc.2012.03.063
Brenza H. L., DeLuca H. F. (2000). Regulation of 25-Hydroxyvitamin D3 1α-hydroxylase gene expression by parathyroid hormone and 1,25-dihydroxyvitamin D3. Biochem. Bioph. 381 (1), 143–152. doi:10.1006/abbi.2000.1970
Brionne A., Nys Y., Hennequet-Antier C., Gautron J. (2014). Hen uterine gene expression profiling during eggshell formation reveals putative proteins involved in the supply of minerals or in the shell mineralization process. BMC Genomics 15 (220), 220–317. doi:10.1186/1471-2164-15-220
Chandra S., Fullmer C. S., Smith C. A., Wasserman R. H., Morrison G. H. (1990). Ion microscopic imaging of calcium transport in the intestinal tissue of vitamin D deficient and vitamin D replete chickens: A 44Ca stable isotope study. P. Natl. Acad. Sci. U. S. A. 87 (15), 5715–5719. doi:10.1073/pnas.87.15.5715
Chun R. F., Blatter E., Elliott S., Fitz-Gibbon S., Rieger S., Sagasti A., et al. (2014). Cloning of a functional 25-hydroxyvitamin D-1α-hydroxylase in zebrafish (Danio rerio). Cell biochem. Funct. 32 (8), 675–682. doi:10.1002/cbf.3071
Cohen A., Bar A., Eisner U., Hurwitz S. (1978). Calcium absorption, calcium binding protein, and eggshell quality in laying hens fed hydroxylated vitamin D derivatives. Poult. Sci. 57 (6), 1646–1651. doi:10.3382/ps.0571646
Comar C. L., Driggers J. C. (1949). Secretion of radioactive calcium in the hen's egg. Science 109 (2829), 282. doi:10.1126/science.109.2829.282
Copp D. H., Cockcroft D. W., Kueh Y. (1967). Calcitonin from ultimobranchial glands of dogfish and chickens. Science 158 (3803), 924–925. doi:10.1126/science.158.3803.924
Corradino R. A. (1993). Calbindin D28k regulation in precociously matured chick egg shell gland in vitro. Gen. Comp. Endocr. 91 (2), 158–166. doi:10.1006/gcen.1993.1115
Corradino R. A., Smith C. A., Krook L. P., Fullmer C. S. (1993). Tissue-specific regulation of shell gland calbindin D28K biosynthesis by estradiol in precociously matured, vitamin D-depleted chicks. Endocrinology 132 (1), 193–198. doi:10.1210/endo.132.1.8419123
Cusack M., Fraser A. C., Stachel T. (2003). Magnesium and phosphorus distribution in the avian eggshell. Comp. Biochem. Physiol. 134 (1), 63–69. doi:10.1016/s1096-4959(02)00185-9
Dacke C. G., Arkle S., Cook D. J., Wormstone I. M., Jones S., Zaidi M., et al. (1993). Medullary bone and avian calcium regulation. J. Exp. Biol. 184, 63–88. doi:10.1242/jeb.184.1.63
Dennis J. E., Xiao S., Agarwal M., Fink D., Heuer A. H., Caplan A. (1996). Microstructure of matrix and mineral components of eggshells from White Leghorn chickens (Gallus gallus). J. Morph. 228 (3), 287–306. doi:10.1002/(SICI)1097-4687(199606)228:3<287::AID-JMOR2>3.0.CO;2-%23
Diana T. F., Calderano A. A., Tavernari F. d. C., Rostagno H. S., Teixeira A. d. O., Albino L. F. T. (2021). Age and calcium sources in laying hen feed affect calcium digestibility. J. Anim. Sci. 11, 501–513. doi:10.4236/ojas.2021.113034
Dousa T. P. (1974). Effects of hormones on cyclic AMP formation in kidneys of nonmammalian vertebrates. Am. J. Physiol. 226 (5), 1193–1197. doi:10.1152/ajplegacy.1974.226.5.1193
Eliam M. C., Basle M., Bouizar Z., Bielakoff J., Moukhtar M., De Vernejoul M. C. (1988). Influence of blood calcium on calcitonin receptors in isolated chick osteoclasts. J. Endocrinol. 199 (2), 243–248. doi:10.1677/joe.0.1190243
Ettinger R. A., DeLuca H. F. (1995). The vitamin D3 hydroxylase-associated protein is a propionamide-metabolizing amidase enzyme. Arch. Biochem. Biophys. 316 (1), 14–19. doi:10.1006/abbi.1995.1003
Ettinger R. A., Ismail R., DeLuca H. F. (1994). cDNA cloning and characterization of a vitamin D3 hydroxylase-associated protein. J. Biol. Chem. 269 (1), 176–182. doi:10.1016/S0021-9258(17)42331-3
Frost T. J., Roland D. A. (1990). The effects of various dietary phosphorus levels on the circadian patterns of plasma 1,25-dihydroxycholecalciferol, total calcium, ionized calcium and phosphorus in laying hens. Poult. Sci. 70, 1564–1570. doi:10.3382/ps.0701564
Fullmer C. S., Brindak M. E., Wasserman R. H., Bar A. (1976). The purification of calcium-binding protein from the uterus of the laying hen. P. Soc. Exp. Biol. Med. 152 (2), 237–241. doi:10.3181/00379727-152-39369
Gautron J., Le Roy N., Nys Y., Rodriguez-Navarro A. B., Hincke M. T. (2021). Avian eggshell biomineralization: An update on its structure, mineralogy, and protein tool kit. BMC Mol. Biol. 22 (11), 11–17. doi:10.1186/s12860-021-00350-0
Gill R. K., Christakos S. (1995). Regulation by estrogen through the 5' flanking region of the mouse calbindin-D28K gene. Mol. Endocrinol. 9 (3), 319–326. doi:10.1210/mend.9.3.7776978
Gloux A., Le Roy N., Brionne A., Bonin E., Juanchich A., Benzoni G., et al. (2019). Candidate genes of the transcellular and paracellular calcium absorption pathways in the small intestine of laying hens. Poult. Sci. 98 (11), 6005–6018. doi:10.3382/ps/pez407
Gloux A., Le Roy N., Ezagal J., Meme N., Hennequet-Antier C., Piketty M. L., et al. (2020a). Possible roles of parathyroid hormone, 1,25(OH)2D3, and fibroblast growth factor 23 on genes controlling calcium metabolism across different tissues of the laying hen. Dom. Anim. Endocrinol. 72 (72), 106407–106412. doi:10.1016/j.domaniend.2019.106407
Gloux A., Le Roy N., Meme N., Piketty M. L., Prie D., Benzoni G., et al. (2020b). Increased expression of fibroblast growth factor 23 is the signature of a deteriorated Ca/P balance in ageing laying hens. Sci. Rep. 10 (1), 21124. doi:10.1038/s41598-020-78106-7
Gregory N. G., Wilkins L. J. (1989). Broken bones in domestic fowl: Handling and processing damage in end-of-lay battery hens. Br. Poul. Sci. 30 (3), 555–562. doi:10.1080/00071668908417179
Guinotte F., Gautron J., Nys Y., Soumarmon A. (1995). Calcium solubilization and retention in the gastrointestinal tract in chicks (Gallus domesticus) as a function of gastric acid secretion inhibition and of calcium carbonate particle size. Brit. J. Nut. 73 (1), 125–139. doi:10.1079/Bjn19950014
Guinotte F., Gautron J., Soumarmon A., Robert J. C., Peranzi G., Nys Y. (1993). Gastric acid secretion in the chicken: Effect of histamine H2 antagonists and H+/K+-ATPase inhibitors on gastro-intestinal pH and of sexual maturity calcium carbonate level and particle size on proventricular H+/K+-ATPase activity. Comp. Biochem. Physiol. 106, 319–327. doi:10.1016/0300-9629(93)90520-E
Hadley J. A., Horvat-Gordon M., Kim W. K., Praul C. A., Burns D., Leach R. M. (2016). Bone sialoprotein keratan sulfate proteoglycan (BSP-KSPG) and FGF-23 are important physiological components of medullary bone. Comp. Biochem. Physiol. 194, 1–7. doi:10.1016/j.cbpa.2015.12.009
Heaney R. P., Armas L. A., Shary J. R., Bell N. H., Binkley N., Hollis B. W. (2008). 25-Hydroxylation of vitamin D3: Relation to circulating vitamin D3 under various input conditions. Am. J. Clin. Nutr. 87 (6), 1738–1742. doi:10.1093/ajcn/87.6.1738
Hincke M. T., Nys Y., Gautron J. (2010). The role of matrix proteins in eggshell formation. J. Poult. Sci. 47 (3), 208–219. doi:10.2141/jpsa.009122
Hofer A. M., Brown E. M. (2003). Extracellular calcium sensing and signalling. Nat. Rev. Mol. Cell Bio. 4, 530–538. doi:10.1038/nrm1154
Holick M. F., Kleiner-Bossallier A., Schnoes H. K., Kasten P. M., Boyle I. T., DeLuca H. F., et al. (1973). 1,24,25-Trihydroxyvitamin D3. J. Biol. Chem. 248 (19), 6691–6696. doi:10.1016/S0021-9258(19)43408-X
Huber K., Zeller E., Rodehutscord M. (2015). Modulation of small intestinal phosphate transporter by dietary supplements of mineral phosphorus and phytase in broilers. Poult. Sci. 94, 1009–1017. doi:10.3382/ps/pev065
Hudson H. A., Britton W. M., Rowland G. N., Buhr R. J. (1993). Histomorphometric bone properties of sexually immature and mature white leghorn hens with evaluation of fluorochrome injection on egg production traits. Poult. Sci. 72 (8), 1537–1547. doi:10.3382/ps.0721537
Hurwitz S., Bar A. (1965). Absorption of calcium and phosphorus along the gastrointestinal tract of the laying fowl as influenced by dietary calcium and eggshell formation. J. Nutr. 86, 433–438. doi:10.1093/jn/86.4.433
Hurwitz S., Bar A. (1968). Activity, concentration, and lumen-blood electrochemical protential difference of calcium in the intestine of the laying hen. J. Nutr. 95, 647–654. doi:10.1093/jn/95.4.647
Hurwitz S., Bar A., Cohen I. (1973). Regulation of calcium absorption by fowl intestine. Am. J. Physiol. 225 (1), 150–154. doi:10.1152/ajplegacy.1973.225.1.150
Hurwitz S., Bar A. (1969). Intestinal calcium absorption in the laying fowl and its importance in calcium homeostasis. Am. J. Clin. Nutr. 22 (4), 391–395. doi:10.1093/ajcn/22.4.391
Ieda T., Takahasi T., Saito N., Yasuoka T., Kawashima M., Izumi T., et al. (2001). Changes in calcitonin receptor bonding in the shell gland of laying hens (Gallus domesticus) during the oviposition cycle. Poult. Sci. 38, 203–212. doi:10.2141/jpsa.38.203
Jande S. S., Tolnai S., Lawson D. E. M. (1981). Immunohistochemical localization of vitamin D-dependent calcium binding protein in duodenum, kidney, uterus, and cerebellum of chickens. Histochemistry 71, 99–116. doi:10.1007/BF00592574
Jones G., Strungnell S. A., DeLuca H. F. (1998). Current understanding of the molecular actions of vitamin D. Phys. Rev. 78 (4), 1193–1231. doi:10.1152/physrev.1998.78.4.1193
Kerschnitzki M., Zander T., Zaslansky P., Fratzl P., Shahar R., Wagermaier W. (2014). Rapid alterations of avian medullary bone material during the daily egg-laying cycle. Bone 69, 109–117. doi:10.1016/j.bone.2014.08.019
Kraintz L., Intscher K. (1969). Effect of calcitonin on the domestic fowl. Can. J. Physiol. Pharm. 47 (3), 313–315. doi:10.1139/y69-057
Li P., Wang R. M., Jiao H. C., Wang X. J., Zhao J. P., Lin H. (2018). Effects of dietary phosphorus level on the expression of calcium and phosphorus transporters in laying hens. Front. Physiol. 9, 627–712. doi:10.3389/fphys.2018.00627
Liang C. T., Balakir R., Barnes J., Sacktor B. (1984). Responses of chick renal cell to parathyroid hormone: Effect of vitamin D. Am. J. Physiol. 246 (5), 401–406. doi:10.1152/ajpcell.1984.246.5.C401
Liang C. T., Barnes J., Cheng L., Balakir R., Sacktor B. (1982). Effects of 1,25-(OH)2D3 administered in vivo on phosphate uptake by isolated chick renal cells. Am. J. Physiol. 242 (5), 312–318. doi:10.1152/ajpcell.1982.242.5.C312
Liao X. D., Suo H. Q., Lu L., Hu Y. X., Zhang L. Y., Luo X. G. (2017). Effects of sodium, 1,25-dihydroxyvitamin D3 and parathyroid hormone fragment on inorganic P absorption and Type IIb sodium-phosphate cotransporter expression in ligated duodenal loops of broilers. Poult. Sci. 96 (7), 2344–2350. doi:10.3382/ps/pex033
Liu S., Zhu W., Ma J., Zhang H., Li Z., Zhang L., et al. (2016). Bovine parathyroid hormone enhances osteoclast bone resorption by modulating V-ATPase through PTH1R. Int. J. Mol. Med. 37 (2), 284–292. doi:10.3892/ijmm.2015.2423
Luck M. R., Sommerville B. A., Scanes C. G. (1979). The effect of egg-shell calcification on the response of plasma calcium activity to parathyroid hormone and calcitonin in the domestic fowl (Gallus domesticus). Comp. Biochem. Physiol. 65, 151–154. doi:10.1016/0300-9629(80)90397-7
Michigami T., Kawai M., Yamazaki M., Ozono K. (2018). Phosphate as a signaling molecule and its sensing mechanism. Am. J. Physiol. 98 (4), 2317–2348. doi:10.1152/physrev.00022.2017
Monkawa T., Yoshida T., Wakino S., Shinki T., Anazawa H., DeLuca H. F., et al. (1997). Molecular cloning of cDNA and genomic DNA for human 25-hydroxyvitamin D3 1α-hydroxylase. Biochem. Bioph. Res. Co. 239 (2), 527–533. doi:10.1006/bbrc.1997.7508
Nicholson G. C., Moseley J. M., Sexton P. M., Martin T. J. (1987). Chicken osteoclasts do not possess calcitonin receptors. J. Bone Min. Res. 2 (1), 53–59. doi:10.1002/jbmr.5650020109
Nys Y., Baker K., Lawson D. E. M. (1992). Estrogen and a calcium flux dependent factor modulate the calbindin gene expression in the uterus of laying hens. Gen. Comp. Endocr. 87 (1), 87–94. doi:10.1016/0016-6480(92)90153-b
Nys Y., Gautron J., Rodriguez-Navarro A. B., Hincke M. T. (2022). “Mechanisms and hormonal regulation of shell formation: Supply of ionic and organic precursors, shell mineralization,” in Sturkie's avian Physiology. Editors C. G. Scanes, and S. Dridi 7 ed (Academic Press), 833–879.
Nys Y., Guyot N. (2011). “Egg formation and chemistry,” in Improving the safety and quality of eggs and egg products (SawstonCambridge: Woodhead Publishing), 83–132.
Nys Y., N'Guyen T. M., Williams J., Etches J. R. (1986). Blood levels of ionized calcium, inorganic phosphorus, 1,25-dihydroxycholecalciferol and gonadal hormones in hens laying hard-shelled or shell-less eggs. J. Endocrinol. 111 (1), 151–157. doi:10.1677/joe.0.1110151
Nys Y., Zawadzki J., Gautron J., Mills A. D. (1991). Whitening of Brown-shelled eggs: Mineral composition of uterine fluid and rate of protoporphyrin deposition. Poult. Sci. 70 (5), 1236–1245. doi:10.3382/ps.0701236
Omdahl J. L., Morris H. A., May B. K. (2002). Hydroxylase enzymes of the vitamin D pathway: Expression, function, and regulation. Annu. Rev. Nutr. 22, 139–166. doi:10.1146/annurev.nutr.22.120501.150216
Parsons A. H., Combs G. F. (1980). Blood ionized calcium cycles in the chicken. Poult. Sci. 60, 1520–1524. doi:10.3382/ps.0601520
Perwad F., Azam N., Zhang M. Y. H., Yamashita T., Tenenhouse H. S., Portale A. A. (2005). Dietary and serum phosphorus regulate fibroblast growth factor 23 expression and 1,25-dihydroxyvitamin D metabolism in mice. Endocrinology 146 (12), 5358–5364. doi:10.1210/en.2005-0777
Perwad F., Zhang M. Y. H., Tenenhouse H. S., Portale A. A. (2007). Fibroblast growth factor 23 impairs phosphorus and vitamin D metabolism in vivo and suppresses 25-hydroxyvitamin D-1alpha-hydroxylase expression in vitro. Am. J. Physiol. 293 (5), 1577–1583. doi:10.1152/ajprenal.00463.2006
Proszkowiec-Weglarz M., Angel R. (2013). Calcium and phosphorus metabolism in broilers: Effect of homeostatic mechanism on calcium and phosphorus digestibility. J. Appl. Poult. Res. 22 (3), 609–627. doi:10.3382/japr.2012-00743
Razzaque M. S. (2009). The FGF23-klotho axis: Endocrine regulation of phosphate homeostasis. Nat. Rev. Endocrinol. 5 (11), 611–619. doi:10.1038/nrendo.2009.196
Ren Z., Ebrahimi M., Butz D. E., Sand J. M., Zhang K., Cook M. E. (2017). Antibody to fibroblast growth factor 23-peptide reduces excreta phosphorus of laying hens. Poult. Sci. 96 (1), 127–134. doi:10.3382/ps/pew189
Ren Z., Yan J., Hu Q., Liu X., Pan C., Liu Y., et al. (2020). Phosphorus restriction changes the expression of fibroblast growth factor 23 and its receptors in laying hens. Front. Physiol. 11, 85–12. doi:10.3389/fphys.2020.00085
Rodriguez-Navarro A. B., Marie P., Nys Y., Hincke M. T., Gautron J. (2015). Amorphous calcium carbonate controls avian eggshell mineralization: A new paradigm for understanding rapid eggshell calcification. J. Struct. Biol. 190 (3), 291–303. doi:10.1016/j.jsb.2015.04.014
Rufener C., Baur S., Stratmann A., Toscano M. J. (2019). Keel bone fractures affect egg laying performance but not egg quality in laying hens housed in a commercial aviary system. Poult. Sci. 98 (4), 1589–1600. doi:10.3382/ps/pey544
Sah N., Mishra B. (2018). Regulation of egg formation in the oviduct of laying hen. World Poult. Sci. J. 74 (3), 509–522. doi:10.1017/S0043933918000442
San Martin Diaz V. E. (2018). Effects of 1α-hydroxycholecalciferol and other vitamin D analogues on liver performance, bone development, meat yield and quality, and mineral digestibility in broilers. Master of Science. Raleigh, NC: North Carolina State University.
Scanes C. G., Campbell R., Griminger P. (1987). Control of energy balance during egg production in the laying hen. J. Nutr. 117 (3), 605–611. doi:10.1093/jn/117.3.605
Shanmugasundaram R., Selvaraj R. K. (2012). Vitamin D-1α-hydroxylase and vitamin D-24-hydroxylase mRNA studies in chickens. Poult. Sci. 91 (8), 1819–1824. doi:10.3382/ps.2011-02129
Shinki T., Shimada H., Wakino S., Anazawa H., Hayashi M., Saruta T., et al. (1997). Cloning and expression of rat 25-hydroxyvitamin D3-1a-hydroxylase cDNA. P. Natl. Acad. Sci. U. S. A. 94, 12920–12925. doi:10.1073/pnas.94.24.12920
Silve C. M., Hradek G. T., Jones A. L., Arnaud C. D. (1982). Parathyroid hormone receptor in intact embryonic chicken bone: Characterization and cellular localization. J. Cell. Biol. 94 (2), 379–386. doi:10.1083/jcb.94.2.379
Simkiss K. (1964). Phosphates as crystal poisons of calcification. Biol. Rev. 39 (4), 487–505. doi:10.1111/j.1469-185X.1964.tb01166.x
Singh R., Joyner C. J., Peddie M. J., Geoffrey Taylor T. (1986). Changes in the concentrations of parathyroid hormone and ionic calcium in the plasma of laying hens during the egg cycle in relation to dietary deficiencies of calcium and vitamin D. Gen. Comp. Endocr. 61 (1), 20–28. doi:10.1016/0016-6480(86)90245-5
Spencer R., Charman M., Wilson P. W., Lawson E. M. (1978). The relationship between vitamin D stimulated calcium transport and intestinal calcium binding protein in the chicken. Biochemistry 170, 93–101. doi:10.1042/bj1700093
Stapane L., Le Roy N., Ezagal J., Rodriguez-Navarro A. B., Labas V., Combes-Soia L., et al. (2020). Avian eggshell formation reveals a new paradigm for vertebrate mineralization via vesicular amorphous calcium carbonate. J. Biol. Chem. 295 (47), 15853–15869. doi:10.1074/jbc.RA120.014542
Sturkie P. D., Mueller w. J. (1976). “Reproduction in the female and egg production,” in Avian Physiology. Editor C. G. Scanes (Berlin, Heidelberg: Springer Springer Advanced Texts in Life Sciences), 302–330.
Taylor T. G., Belanger L. F. (1969). The mechanism of bone resorption in laying hens. Calc. Tiss. Res. 4 (2), 162–173. doi:10.1007/BF02279117
Taylor T. G., Wasserman R. H. (1972). Vitamin D-induced calcium-binding protein: Comparative aspects in kidney and intestine. Am. J. Physiol. 223, 110–114. doi:10.1152/ajplegacy.1972.223.1.110
Tomoe Y., Segawa H., Shiozawa K., Kaneko I., Tominaga R., Hanabusa E., et al. (2009). Phosphaturic action of fibroblast growth factor 23 in Npt2 null mice. Am. J. Physiol. Ren. Physiol. 298 (6), 1341–1350. doi:10.1152/ajprenal.00375.2009
Van de Velde J. P., Loveridge N., Vermieden J. P. W. (1984a). Parathyroid hormone responses to calcium stress during eggshell calcification. Endocrinology 115 (5), 1901–1904. doi:10.1210/endo-115-5-1901
Van de Velde J. P., Vermieden J. P. W., Touw J. J. A., Veldhuizen J. P. (1984b). Changes in activity of chicken medullary bone cell populations in relation to the egg laying cycle. Metab. Bone Dis. Relat. 5 (4), 191–193. doi:10.1016/0221-8747(84)90029-8
Wang R. M., Zhao J. P., Wang X. J., Jiao H. C., Wu J. M., Lin H. (2018). Fibroblast growth factor 23 mRNA expression profile in chickens and its response to dietary phosphorus. Poult. Sci. 97 (7), 2258–2266. doi:10.3382/ps/pey092
Wang X. J., Li P., Zhao J. P., Jiao H. C., Lin H. (2022). The temporal gene expression profiles of calcium and phosphorus transporters in Hy-Line Brown layers. Poult. Sci. 101 (4), 101736–101811. doi:10.1016/j.psj.2022.101736
Warren D. C., Scott H. M. (1935). Physiological factors influencing the rate of egg formation in the domestic hen. J. Agric. Res. 51 (6), 565–572.
Watanabe K. P., Kawai Y. K., Ikenaka Y., Kawata M., Ikushiro S., Sakaki T., et al. (2013). Avian cytochrome P450 (CYP) 1-3 family genes: Isoforms, evolutionary relationships, and mRNA expression in chicken liver. PLoS One 8 (9), 756899–e75711. doi:10.1371/journal.pone.0075689
Wedral E. M., Vadehra D. V., Baker R. C. (1974). Chemical composition of the cuticle, and the inner and outer shell membranes from eggs of gallus gallus. Comp. Biochem. Physiol. 47 (3), 631–640. doi:10.1016/0305-0491(74)90011-x
Whitehead C. C. (2004). Overview of bone biology in the egg-laying hen. Poult. Sci. 83 (2), 193–199. doi:10.1093/ps/83.2.193
Wideman R. F., Braun E. J. (1981). Stimulation of avian renal phosphate secretion by parathyroid hormone. Am. J. Physiol. 241 (3), 263–272. doi:10.1152/ajprenal.1981.241.3.F263
Wilson S., Duff S. R. (1990). Morphology of medullary bone during the egg formation cycle. Vet. Sci. 48 (2), 216–220. doi:10.1016/s0034-5288(18)30993-7
Yan F., Angel R., Ashwell C. M. (2007). Characterization of the chicken small intestine type IIb sodium phosphate cotransporter. Poult. Sci. 86, 67–76. doi:10.1093/ps/86.1.67
Yan J., Pan C., Liu Y., Liao X., Chen J., Zhu Y., et al. (2022). Dietary vitamin D3 deprivation suppresses fibroblast growth factor 23 signals by reducing serum phosphorus levels in laying hens. Anim. Nutr. 9, 23–30. doi:10.1016/j.aninu.2021.07.010
Yasuoka T., Kawashima M., Takahasi T., Tanaka K. (1998). Calcitonin receptor binding properties in bone and kidney of the chicken during the oviposition cycle. J. Bone Min. Res. 13 (9), 1412–1419. doi:10.1359/jbmr.1998.13.9.1412
Keywords: laying hen, calcium, phosphorus, vitamin D3, skeletal health, egg formation
Citation: Sinclair-Black M, Garcia RA and Ellestad LE (2023) Physiological regulation of calcium and phosphorus utilization in laying hens. Front. Physiol. 14:1112499. doi: 10.3389/fphys.2023.1112499
Received: 30 November 2022; Accepted: 27 January 2023;
Published: 07 February 2023.
Edited by:
Anthony Pokoo-Aikins, Agricultural Research Service (USDA), United StatesReviewed by:
Wu Shugeng, Feed Research Institute, Chinese Academy of Agricultural Sciences, ChinaMichel Duclos, UMR BOA INRAE University of Tours, France
Catarina Stefanello, Federal University of Santa Maria, Brazil
Matthew F. Warren, University of Wisconsin-Madison, United States
Yves Nys, INRAE centre de Tours, France
Copyright © 2023 Sinclair-Black, Garcia and Ellestad. This is an open-access article distributed under the terms of the Creative Commons Attribution License (CC BY). The use, distribution or reproduction in other forums is permitted, provided the original author(s) and the copyright owner(s) are credited and that the original publication in this journal is cited, in accordance with accepted academic practice. No use, distribution or reproduction is permitted which does not comply with these terms.
*Correspondence: Laura E. Ellestad, bGVsbGVzdGFkQHVnYS5lZHU=