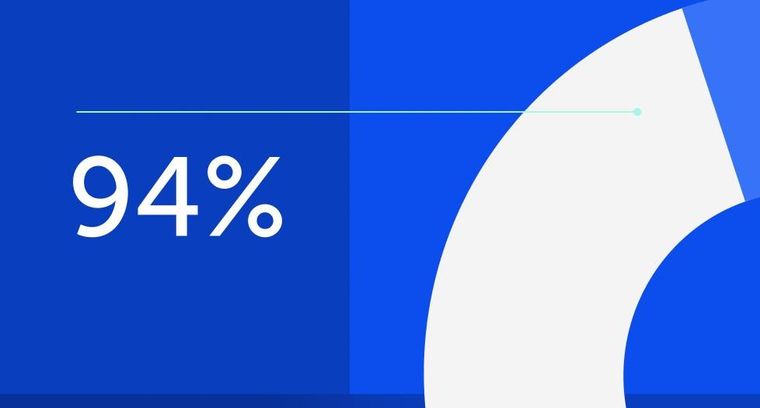
94% of researchers rate our articles as excellent or good
Learn more about the work of our research integrity team to safeguard the quality of each article we publish.
Find out more
ORIGINAL RESEARCH article
Front. Physiol., 25 April 2023
Sec. Invertebrate Physiology
Volume 14 - 2023 | https://doi.org/10.3389/fphys.2023.1099806
Pesticide residues have been reported in hive-stored products for long periods. Larvae of honey bees experience oral or contact exposure to these products during their normal growth and development inside the cells. We analyzed various toxicological, morphogenic, and immunological effects of residue-based concentrations of two fungicides, captan and difenoconazole, on the larvae of worker honey bees, Apis mellifera. Selected concentrations (0.08, 0.4, 2, 10, and 50 ppm) of both fungicides were applied topically at a volume of 1 µL/larva/cell as single and multiple exposures. Our results revealed a continuous, concentration-dependent decrease in brood survival after 24 h of treatment to the capping and emergence stages. Compared to larvae with a single exposure, the multiply exposed youngest larvae were most sensitive to fungicidal toxicity. The larvae that survived higher concentrations, especially multiple exposures, showed several morphological defects at the adult stage. Moreover, difenoconazole-treated larvae showed a significantly decreased number of granulocytes after 1 h of treatment followed by an increase after 24 h of treatment. Thus, fungicidal contamination poses a great risk as the tested concentrations showed adverse effects on the survival, morphology, and immunity of larval honey bees.
The economic and ecological importance of honey bees is high, as they provide valuable pollination services to crops, in addition to a variety of hive products (Rişcu and Bura, 2013). Biotic pollinators play an important role in food production and the maintenance of plant ecosystems; among these pollinators, bees are essential for good levels of pollination of most cultivated crops globally (Partap, 2011).
Since the 1990s, alarming declines in the populations of both wild and domesticated pollinators have been reported worldwide (Finley et al., 1996; Abrol, 2012). Interactions among different stressors like pests, diseases, pesticides, changing climate, and management practices are linked to these declines (Hristov et al., 2020). Moreover, race, comb age, cell size, and diet also affect the overall colony survival by inducing morphological or physiological variations (Abou-Shaara and Al-Ghamdi, 2012; Alfalah et al., 2012; Sauthier et al., 2017; Chole et al., 2019; Kaur et al., 2021). In addition, the geographic origin of a species and varying environmental conditions in a region also alter honey bee morphology (Al-Kahtani and Taha, 2014; Al-Kahtani and Taha, 2021; Shawer et al., 2021).
Among pesticides, insecticides cause maximum toxicity to bees, whereas other pesticides like fungicides, herbicides, and plant growth regulators are considered relatively safe (Mayer and Lunden, 1986; Devillers, 2002). However, recent studies have demonstrated the negative impact of fungicides on various hymenopterans (Heneberg et al., 2021; Thompson et al., 2023) including honey bees (Drummond, 2022; Serra et al., 2023; Xiong et al., 2023). The application of fungicides on blooming crops (Mayer and Lunden, 1986; Tamburini et al., 2021) leads to the direct contamination of forager bees, which, along with the collected pollen or nectar, transport fungicide residues to hives and, thus, contaminate the entire colony, including hive-stored products (Devillers, 2002; Bogdanov 2006). While the concentrations of fungicidal residues declined from treated flowers to forager bees (Drummond, 2022) to hive-stored honey and brood (Piechowicz et al., 2018), residues remain abundant in various hive products (Piechowicz et al., 2018; Rondeau and Raine, 2022; Xiao et al., 2022) and in some cases even exceeded the levels of concern for chronic risk to bees (Rondeau and Raine, 2022). The persistence of residues in hive-stored products may increase brood exposure to these residues. However, few studies have reported the role of residual fungicides on compromised bee health (Mullin et al., 2010; Rondeau and Raine, 2022). The toxicity criteria for field-recommended concentrations of captan toward honey bee brood and adults have been reported (Mussen et al., 2004; Ladurner et al., 2005) but are much higher than the residual forms of captan present in hive-stored products. Furthermore, fungicides like difenoconazole were studied only jointly with other pesticides or pests (Almasri et al., 2021; Pal et al., 2022), which masked their individual effects.
In the present scenario, the decline in the honey bee population is linked to weakened immune systems due to pesticides. These immuno-challenged bees are more prone to disease (Pamminger et al., 2018). Immunity in insects is generally comprised of cellular and humoral responses. At the cellular level, hemocytes (predominantly plasmatocytes and granulocytes) play an important role in immunity as they are involved in defense responses like phagocytosis, encapsulation, and nodulation, and show variations in their number in response to a foreign agent (Kwon et al., 2014; Negri et al., 2014; Barakat et al., 2016). The adverse effects of insecticides on the hemocytes of honey bees have been reported (Perveen and Ahmad, 2017; Sukkar et al., 2023) but similar studies for fungicides are lacking (Inoue et al., 2022). Until now no information has been reported regarding the adverse effects of residual forms of captan and difenoconazole on honey bee broods. Therefore, the present study assessed the individual effects of these fungicides (in both single and multiple exposures) on larvae survival and immunity and also on the morphology of adult bees developed from treated larvae.
Three well-populated hives (each with 8–10 frames) of A. mellifera were selected from the apiary of Khalsa College, Amritsar (India) for each fungicide. These colonies were well maintained throughout the experimental period.
Captan 50 WP (Captaf, Rallis India Ltd., Mumbai) and difenoconazole 25 EC (Score, Syngenta India Ltd., Pune) were selected for the study. The concentrations of both fungicides were selected based on reported residual amounts in various hive products. The maximum reported residues of difenoconazole in hive-stored honey, pollen, and bee bread varied from 0.0006–0.0009 mg/kg, 0.043–0.411 mg/kg, and 0.27–0.327 mg/kg, respectively. Similarly, the maximum reported residues of captan in hive-stored honey, pollen, bee wax, and bee bread varied from 0.009–0.019 mg/kg, 2.99–10.36 mg/kg, 0.069–0.4 mg/kg, and 6.39 mg/kg, respectively (Kubik et al., 2000; Bernal et al., 2010; Johnson et al., 2010; Mullin et al., 2010; Chauzat et al., 2011; Rennich et al., 2012; Stoner and Eitzer, 2013; McArt et al., 2017). An extensive literature review suggested that growing larvae may be exposed to a maximum of 10 ppm of captan and 0.4 ppm of difenoconazole either ectopically or via food. Therefore, the final concentrations (including the maximum reported residues and concentrations above and below these residues) of both fungicides were selected to be 0.08 ppm, 0.4 ppm, 2 ppm, 10 ppm, and 50 ppm. A negative control (NC) consisting of distilled water (solvent) and an untreated control (UC) were also included for comparison.
To obtain uniformly aged larvae of worker honey bees, a vertical queen excluder was introduced in each colony to cage the queen on one side along with bees and an empty drawn frame for egg laying. After every 24 h, a frame with newly laid eggs was replaced with another empty comb for continuous egg laying. Frames with eggs were marked with the date of egg laying and kept on the other side of the hive. For the measurement of age, newly hatched larvae were defined as 1 day old and so on up to 6 days old. Accordingly, the larvae were divided into three age groups: 1–2, 3–4, and 5–6 days old.
Different colored pins were used on a single frame to mark larvae receiving different concentrations and the controls. Depending upon the persistence of residues in hive products, risks related to honey bee health may increase due to increased exposure. The larval stage usually lasts for 5–6 days, and they are inspected and fed multiple times by multiple bees (Huang and Otis, 1991), which likely increases the possibility of larval exposure to contaminated food multiple times before the capping of their cells. Thus, in the present study, selected larvae were exposed both once and multiple times to selected concentrations of each fungicide. In one-time exposure (OTE), larvae belonging to the three different age groups were exposed once. In multiple exposures (ME), 1–2-day-old larvae were exposed four times, and 3–4-day-old larvae were exposed twice. From each tested concentration and solvent, 1 µL was delivered topically into cells containing larvae (Atkins and Kellum, 1986). A total of 90 larvae were selected (30 from each of three hives), for each concentration per age group per fungicide and control. After the treatments, the frames were returned to their respective hives, where they were fed by the nurse bees. Survival was checked 24 h after treatment (HAT), at capping, and at emergence.
After capping, the treated larvae were caged and the emerging adults were collected and checked for morphological variations in length and width of the head; length and breadth of the abdomen, forewing, and hindwing; and length of the hind leg (Figure 1). All body parts were measured with a Magnus trinocular stereomicroscope (MSZ-TR [LED]) using MagVision advanced image analysis software (MIPS-ver 3.7).
Similar treatments were administered to 4-day-old worker larvae. Differential hemocyte counts (DHCs) were estimated at 1 HAT and 24 HAT. Hemolymph was extracted by puncturing the larva from the lateral side with a sterilized needle. Smears were prepared on clean glass slides. Cells were stained using Giemsa’s staining solution. The prepared slides were observed using a binocular microscope (Olympus CX21i LED) to identify cells based on the shape and size of the cells and nuclei, vacuolization, and cytoplasmic staining. A total of 100 cells per slide were counted, and three slides per treatment were observed containing hemolymph of three different larvae administered the same treatment.
Statistical analyses were performed using IBM SPSS Statistics for Windows, version 21.0. One-way ANOVA followed by Tukey’s HSD (at a 0.05 level of significance) was used to analyze the effects of concentration on survival, morphology, and DHC. Two-way ANOVA was used to analyze the combined effect of concentration and time on DHC.
A single exposure to the 10 ppm concentration of captan significantly reduced the survival of 1–2-day-old larvae (at the emergence stage) compared to UC and NC. In addition, the 50 ppm concentration of captan significantly lowered the survival of 1–2-day-old larvae (at all stages of observation), 3–4-day-old larvae (capping and emergence stages), and 5–6-day-old larvae (emergence stage), with the lowest survival (65.7%) observed in 1–2-day-old larvae at the emergence stage (Figure 2). However, OTE to 10 and 50 ppm of difenoconazole significantly reduced the survival of 1–2-day-old larvae (at capping and emergence stage) and older 3–4- and 5–6-day-old larvae (at emergence stage), with the lowest survival (66.7%) observed in 1–2-day-old larvae at 50 ppm at the emergence stage (Figure 3). Moreover, 50 ppm of difenoconazole also lowered the survival of 1–2- and 3–4-day-old larvae after 24 h of treatment.
FIGURE 2. Survival of 1–2-, 3–4-, and 5–6-day-old honey bee larvae after OTE to captan. The different letters (a, b … ) indicate significant differences by Tukey’s HSD (p < 0.05).
FIGURE 3. Survival of 1–2-, 3–4-, and 5–6-day-old honey bee larvae after OTE to difenoconazole The different letters (a, b … ) indicate significant differences by Tukey’s HSD (p < 0.05).
ME had more pronounced effects, as 10 ppm and 50 ppm of both fungicides significantly lowered the survival of all tested age groups at all stages of observation. The lowest bee survival (44.3% for groups exposed to captan and 46.7% for groups exposed to difenoconazole) was observed at the emergence stage after exposing 1–2-day-old larvae to the 50 ppm concentration (Figures 4, 5). The lower tested concentrations had no significant effect on brood survival in both OTE and ME.
FIGURE 4. Survival of 1–2- and 3–4-day-old honey bee larvae after ME to captan. The different letters (a, b … ) indicate significant differences by Tukey’s HSD (p < 0.05).
FIGURE 5. Survival of 1–2- and 3–4-day-old honey bee larvae after ME to difenoconazole. The different letters (a, b … ) indicate significant differences by Tukey’s HSD (p < 0.05).
All tested larval age groups after OTE to 10 and 50 ppm of captan showed significant reductions in forewing length at the adult stage compared to UC and NC, with maximum reductions at 50 ppm. Additionally, the hind leg length was also significantly reduced in bees developed from 1–2- and 3–4-day-old larvae after OTE to 50 ppm of captan (Figure 6). However, OTE to 50 ppm of difenoconazole significantly reduced the forewing length and breadth only in bees developed from 1–2-day-old larvae (Figure 7).
FIGURE 6. Morphological variations in adult honey bees developed from larvae after OTE and ME to captan. UC, untreated control; NC, negative control; OTE, one-time exposure; ME, multiple exposures. The different small letters (a, b … ) within a column indicate significant differences by Tukey’s HSD (p < 0.05).
FIGURE 7. Morphological variations in adult honey bees developed from larvae after OTE and ME to difenoconazole. UC, untreated control; NC, negative control; OTE, one-time exposure; ME, multiple exposures. The different small letters (a, b … ) within a column indicate significant differences by Tukey’s HSD (p < 0.05).
More significant variations were observed in ME than in OTE (Supplementary Table S1). The highest (50 ppm) concentration of captan significantly reduced the lengths of the abdomen, forewing, and hind leg in bees developed from both tested age groups of larvae. Additionally, bees developed from 1–2-day-old larvae treated with 10 and 50 ppm showed significantly reduced head width and forewing size (Figure 6). Head width, abdomen breadth, forewing length and breadth, and hind leg length were significantly reduced in bees exposed as 1–2-day-old larvae to 50 ppm of difenoconazole. Only the forewing length was significantly reduced at 10 ppm. Bees developed from 3–4-day-old treated larvae showed significant reductions in similar body parts except for the abdomen at 50 ppm (Figure 7).
Four types of cells—granulocytes, permeabilized cells, spindle-shaped cells, and permeable nuclei—were identified from the hemolymph of 4-day-old worker larvae (Figure 8), with the dominance of granulocytes followed by permeabilized cells and other hemocyte communities (including spindle shaped-cells and permeable nuclei) in control larvae.
FIGURE 8. Hemocytes observed in worker honey bee larvae. (A) Granulocytes. (B) Permeabilized cell. (C) Spindle-shaped cell. (D) Permeable nucleus.
Two-way ANOVA analysis of captan-exposed larvae showed time-dependent but concentration-independent significant variations in hemocytes (Figure 9). However, two-way ANOVA analysis of difenoconazole-exposed larvae showed significant time- and concentration-dependent variations in hemocytes. Treated larvae at 1 HAT showed significant variations in the numbers of granulocytes and permeabilized cells at 10 and 50 ppm compared to UC and NC. The lowest granulocyte count, 26.3%, was observed at 50 ppm along with 65.7% permeabilized cells and 8% others. However, at 24 HAT, the counts were 61% granulocytes, 36.3% permeabilized cells, and 2.7% others, which was statistically non-significant compared to controls (Figure 10).
FIGURE 10. Effect of difenoconazole on the differential hemocyte counts (DHC) of honey bee larvae, where * indicates a significant difference by Tukey’s HSD (p < 0.05).
Our results indicated that both tested fungicides had concentration- and age-dependent effects, with maximum damage at 50 ppm in the youngest (1–2-day-old) larvae followed by 3–4- and 5–6-day-old larvae. This age-dependent variation in sensitivity could have occurred due to two reasons. The first possibility is the increased body mass of older larvae. The average body weight of the oldest worker larva is more than 50 times that of the youngest larva (Malone et al., 2002). Since the amount of pesticide applied to any individual is a function of its body mass (Malbert-Colas et al., 2020), the quantity of fungicide applied per unit body mass of older larvae was lower compared to younger larvae, thus making older larvae less sensitive. Second, the age-specific effects of fungicides could be attributed to age-dependent variations in detoxifying enzyme levels, which usually increase with age in bees (Smirle, 1993). Detoxifying activity is further enhanced by phytochemicals like abscisic acid and p-coumaric acid, which are naturally present in bee food, i.e., pollen and honey (Mao et al., 2013; Negri et al., 2015). Food consumption usually increases with the age of the growing larva and peaks on the 4–5th day of larval age (Rortais et al., 2005). Furthermore, the quality of food consumed also varies with age as young larvae are predominantly fed royal jelly, while older larvae receive pollen (Haydak, 1970). Thus, the higher survival of older larvae may be due to greater consumption of pollen, which naturally contains phytochemicals responsible for pesticide tolerance and detoxification.
In our study, residue-based and higher concentrations of captan significantly altered brood survival. Captan shows antifungal activity due to its negative interaction with glutathione (Roberts et al., 2007), which also play an important role in oxidative stress management in animals (Wu et al., 2004). Thus, captan presumably has the same mode of action in honey bees as in fungi. Our results on A. mellifera agree with those of Mussen et al. (2004) who reported complete mortality of worker larvae after oral exposure to captan at a test dose of 0.8 mg/10 g diet (80 ppm/larva) but contrast with those of Everich et al. (2009) who reported nonsignificant effects of available commercial formulations of captan 50 WP and captan 80 WDH on overall hive health including foragers and brood after their application on blooming crops at a rate of 5 kg a. i./ha. As reported by Mussen et al. (2004), foragers are contaminated with 1 µg of pesticide for each 1 pound of pesticide applied per acre; thus, the application of 5 kg a. i./ha (4.46 lb/acre) in the study of Everich et al. (2009) would lead to the contamination of a visiting forager with 4.46 µg of captan (44.6 ppm/bee). These variations in the toxicity behavior of captan could be attributed to its different formulations, concentrations, exposure routes, and age or stage of the test insect.
We also found that higher concentrations of difenoconazole significantly reduced brood survival. Azole fungicide shows fungus toxicity due to its interference with cytochrome P-450 (Yoshida, 1988) which also regulates detoxification process in insects (Haas et al., 2022). Therefore, difenoconazole toxicity in honey bees occurs due to oxidation stress induced by the fungicide by reducing or inhibiting the activity of enzymes involved in antioxidant defenses (Pal et al., 2022).
Broods that survived higher fungicide concentrations showed various amorphogenic alterations in the adult stages. Our results indicated that residue-based concentrations of captan significantly reduced the sizes of the forewing and head in bees developed from the youngest group of larvae. Moreover, higher concentrations had more severe effects on morphology. These results agree with those of Atkins and Kellum. (1986), who showed that the negative effect of captan was greatly enhanced when applied to younger stages than later stages and that the contaminated larvae that survived developed into deformed bees. Our results also demonstrated that residue-based concentrations of difenoconazole had no adverse effect on the morphology of adults developed from treated larvae. However, higher concentrations significantly altered the morphology.
Morphological variations in adult insects may occur due to the disturbance of the imaginal discs of larvae (Gibson and Schubiger, 2000) which form the basic body structure in the adult stage, including legs in honey bees (Santos and Hartfelder, 2015). The treatment of larvae with fungicides may also disturb their imaginal discs; however, more research is needed on this topic. Other possible explanations for the morphogenic effects could be the altered behavior of nurse bees, as they change nursing activity with the condition of developing larvae (Siefert et al., 2020) and show lesser brood care in case of contaminated broods. The resulting malformed adults may interfere with the future work efficiencies of bees by reducing their lifespan (Wu et al., 2011), foraging (Higginson and Barnard, 2004), and learning activities (Worden et al., 2005).
The results of the current study provided experimental evidence that exposure of 4-day-old A. mellifera larvae to fungicides led to significant variations in DHC. Under normal conditions, we found that granulocytes are the most dominant hemocyte type, followed by permeabilized cells and others. Richardson et al. (2018) also reported similar types of cells with a predominance of granulocytes in worker honey bee larvae. Granulocytes and plasmatocytes are the two main types of hemocytes, which provide cellular immunity in insects through defense responses like encapsulation, phagocytosis, and nodulation. The other hemocyte components participate by interacting with these two hemocytes (Kwon et al., 2014).
The results of the current experiments demonstrated that with higher concentrations of difenoconazole, granulocyte numbers were significantly decreased while the counts of permeabilized cells and others were increased at 1 HAT. The decrease in granulocytes may have occurred due to reduced mitotic division or increased cell death following pesticide application (Uckan and Sak, 2010). Another possible explanation for these variations in granulocyte counts could be fungicide-generated oxidative stress (Han et al., 2016), which can cause cell injury (Sies, 2000). While the field-recommended concentrations of difenoconazole have very low or no toxicity to bees (Mommaerts and Smagghe, 2011), it enhances the toxicity of other pesticides when used in combination (Pal et al., 2022). This enhanced toxicity could be linked to the weakening of bee immune systems by difenoconazole due to reduced granulocyte count.
Furthermore, at 24 HAT, our results showed increases in granulocyte counts and decreases in all other hemocyte types. The increase in granulocytes might be due to the initiation of defense responses against fungicides. Our results are consistent with those reported by Negri et al. (2014) and Barakat et al. (2016), who also reported increased granulocyte counts in honey bee larvae at 24 HAT following exposure to other stress factors like nylon implants and bacteria injections. James and Xu (2012) reported that the increased total hemocyte and granulocyte counts were closely associated with cellular defense responses and detoxification of pesticides.
Both fungicides adversely affected the brood survival in a concentration-dependent manner. The effects of fungicides applied to immature stages (larvae) were observed in the mature stages as they developed into malformed adults. More pronounced effects of both tested fungicides were observed in multiply exposed larvae as compared to singly exposed larvae. The application of fungicides further affected larval immunity by reducing the number of granulocytes at 1 HAT. Therefore, fungicides, a class of pesticides generally considered to be relatively non-toxic to bees, showed noteworthy effects on the survival, morphology, and immunity of honey bee larvae. Thus, the toxicity criteria of fungicides must be re-evaluated, particularly in the context of immature honey bee stages.
The original contributions presented in the study are included in the article/Supplementary Material. Further inquiries can be directed to the corresponding author.
The experiment was designed by GK, AS, and RS. The experiment was conducted by GK, ST, and Shyamli. Data analysis was performed by RS and AT. The manuscript was written by GK. The study was edited and supervised by RS.
The infrastructure provided by the PG Department of Agriculture of Khalsa College, Amritsar, to conduct this research is gratefully acknowledged.
The authors declare that the research was conducted in the absence of any commercial or financial relationships that could be construed as a potential conflict of interest.
All claims expressed in this article are solely those of the authors and do not necessarily represent those of their affiliated organizations, or those of the publisher, the editors and the reviewers. Any product that may be evaluated in this article, or claim that may be made by its manufacturer, is not guaranteed or endorsed by the publisher.
The Supplementary Material for this article can be found online at: https://www.frontiersin.org/articles/10.3389/fphys.2023.1099806/full#supplementary-material
ANOVA, analysis of variance; DHC, differential hemocyte count; Fig., figure; HAT, hours after treatment; HSD, honest significant difference; ME, multiple exposures; NC, negative control; OTE, one-time exposure; ppm, parts per million; mg/kg, milligrams per kilograms; lb/acre, pounds per acre; µg, microgram; µl, microliter; a.i./ha, active ingredient per hectare; %, percent, g, gram; mm, millimeter, UC, untreated control.
Abou-Shaara, H. F., and Al-Ghamdi, A. A. (2012). Studies on wings symmetry and honey bee races discrimination by using standard and geometric morphometrics. Biotechnol. Anim. Husb. 28, 575–584. doi:10.2298/BAH1203575A
Abrol, D. P. (2012). “Decline in pollinators,” in Pollination biology (Dordrecht: Springer), 545–601.
Al-Kahtani, S. N., and Taha, E. K. A. (2014). Morphometric studies on dwarf honey bee Apis florea F. workers in Saudi Arabia. J. Apic. Sci. 58, 127–134. doi:10.2478/jas-2014-0013
Al-Kahtani, S. N., and Taha, E. K. A. (2021). Morphometric study of Yemeni (Apis mellifera jemenitica) and Carniolan (A. m. carnica) honey bee workers in Saudi Arabia. Plos One 16, e0247262. doi:10.1371/journal.pone.0247262
Alfalah, H. A., Shaibi, T., Tawfiq, M. M., and Mogrby, A. (2012). The effects of wax comb age on some morphometrics characteristics of honey bee (Apis mellifera L.) workers. Persian Gulf Crop Prot. 1, 18–23.
Almasri, H., Tavares, D. A., Diogon, M., Pioz, M., Alamil, M., Sené, D., et al. (2021). Physiological effects of the interaction between Nosema ceranae and sequential and overlapping exposure to glyphosate and difenoconazole in the honey bee Apis mellifera. Ecotoxicol. Environ. Saf. 217, 112258. doi:10.1016/j.ecoenv.2021.112258
Atkins, E. L., and Kellum, D. (1986). Comparative morphogenic and toxicity studies on the effect of pesticides on honey bee brood. J. Apic. Res. 25, 242–255. doi:10.1080/00218839.1986.11100725
Barakat, E. M. S., AboKersh, M. O., and Gomaa, S. A. S. (2016). Haemocyte activity and cellular defense reactions in various larval instars of honey bee (Apis mellifera L.) following natural and experimental bacterial infections. Greener J. Biol. Sci. 6, 020–033. doi:10.15580/GJBS.2016.2.012016017
Bernal, J., Garrido-Bailon, E., Del Nozal, M. J., Gonzalez-Porto, A. V., Martin-Hernandez, R., Diego, J. C., et al. (2010). Overview of pesticide residues in stored pollen and their potential effect on bee colony (Apis mellifera) losses in SpainApis mellifera) losses in Spain. J. Econ. Entomol. 103, 1964–1971. doi:10.1603/EC10235
Chauzat, M. P., Martel, A. C., Cougoule, N., Porta, P., Lachaize, J., Zeggane, S., et al. (2011). An assessment of honey bee colony matrices, Apis mellifera (Hymenoptera: Apidae) to monitor pesticide presence in continental FranceApis mellifera (Hymenoptera: Apidae) to monitor pesticide presence in continental France. Environ. Toxicol. Chem. 30, 103–111. doi:10.1002/etc.361
Chole, H., Woodard, S. H., and Bloch, G. (2019). Body size variation in bees: Regulation, mechanisms, and relationship to social organization. Curr. Opin. Insect Sci. 35, 77–87. doi:10.1016/j.cois.2019.07.006
Devillers, J. (2002). “Acute toxicity of pesticides to honey bees,” in Honey bees: Estimating the environmental impact of chemicals. Editors J. Devillers, and M. Pham-Delegue (London: Taylor & Francis), 56–66.
Drummond, F. A. (2022). Honey bee exposure to the fungicide propiconazole in lowbush blueberry fields. Agronomy 12, 3081. doi:10.3390/agronomy12123081
Everich, R., Schiller, C., Whitehead, J., Beavers, M., and Barrett, K. (2009). Effects of captan on Apis mellifera brood development under field conditions in California almond orchards. J. Econ. Entomol. 102, 20–29. doi:10.1603/029.102.0104
Finley, J., Camazine, S., and Frazier, M. (1996). The epidemic of honey bee colony losses during the 1995-1996 season. Am. Bee J. 136, 805–808.
Gibson, M. C., and Schubiger, G. (2000). Peripodial cells regulate proliferation and patterning of Drosophila imaginal discs. Cell. 10, 343–350. doi:10.1016/S0092-8674(00)00125-2
Haas, J., Glaubitz, J., Koenig, U., and Nauen, R. (2022). A mechanism-based approach unveils metabolic routes potentially mediating chlorantraniliprole synergism in honey bees, Apis mellifera L., by azole fungicides. Pest Manag. Sci. 78, 965–973. doi:10.1002/ps.6706
Han, Y., Liu, T., Wang, J., Wang, J., Zhang, C., and Zhu, L. (2016). Genotoxicity and oxidative stress induced by the fungicide azoxystrobin in zebrafish (Danio rerio) livers. Pestic. Biochem. Physiol. 133, 13–19. doi:10.1016/j.pestbp.2016.03.011
Haydak, M. H. (1970). Honey bee nutrition. Annu. Rev. Entomol. 15, 143–156. doi:10.1146/annurev.en.15.010170.001043
Heneberg, P., Svoboda, J., and Pech, P. (2021). Claustral colony founding does not prevent sensitivity to the detrimental effects of azole fungicides on the fecundity of ants. J. Environ. Manage. 280, 111740. doi:10.1016/j.jenvman.2020.111740
Higginson, A. D., and Barnard, C. J. (2004). Accumulating wing damage affects foraging decisions in honey bees (Apis mellifera L.). Ecol. Entomol. 29, 52–59. doi:10.1111/j.0307-6946.2004.00573.x
Hristov, P., Shumkova, R., Palova, N., and Neov, B. (2020). Factors associated with honey bee colony losses: A mini-review. Vet. Sci. 7, 166. doi:10.3390/vetsci7040166
Huang, Z. Y., and Otis, G. W. (1991). Inspection and feeding of larvae by worker honey bees (hymenoptera: Apidae): Effect of starvation and food quantity. J. Insect Behav. 4, 305–317. doi:10.1007/BF01048280
Inoue, L. V., Domingues, C. E., Gregorc, A., Silva-Zacarin, E. C., and Malaspina, O. (2022). Harmful effects of pyraclostrobin on the fat body and pericardial cells of foragers of africanized honey bee. Toxics 10, 530. doi:10.3390/toxics10090530
James, R. R., and Xu, J. (2012). Mechanisms by which pesticides affect insect immunity. J. Invertebr. Pathol. 109, 175–182. doi:10.1016/j.jip.2011.12.005
Johnson, R. M., Ellis, M. D., Mullin, C. A., and Frazier, M. (2010). Pesticides and honey bee toxicity–USA. Apidologie 41, 312–331. doi:10.1051/apido/2010018
Kaur, G., Sharma, R., Chaudhary, A., and Singh, R. (2021). Factors affecting immune responses in honey bees: An insight. J. Apic. Sci. 65, 25–47. doi:10.2478/JAS-2021-0012
Kubik, M., Nowacki, J., Pidek, A., Warakomska, Z., Michalczuk, L., Goszczyñski, W., et al. (2000). Residues of captan (contact) and difenoconazole (systemic) fungicides in bee products from an apple orchard. Apidologie 31, 531–541. doi:10.1051/apido:2000144
Kwon, H., Bang, K., and Cho, S. (2014). Characterization of the hemocytes in larvae of Protaetia brevitarsis seulensis: Involvement of granulocyte-mediated phagocytosis. PLoS ONE 9, e103620. doi:10.1371/journal.pone.0103620
Ladurner, E., Bosch, J., Kemp, W. P., and Maini, S. (2005). Assessing delayed and acute toxicity of five formulated fungicides to Osmia lignaria Say and Apis mellifera. Apidologie 36, 449–460. doi:10.1051/apido:2005032
Malbert-Colas, A., Drozdz, T., Massot, M., Bagni, T., Chertemps, T., Maria, A., et al. (2020). Effects of low concentrations of deltamethrin are dependent on developmental stages and sexes in the pest moth Spodoptera littoralis. Environ. Sci. Pollut. Res. 27, 41893–41901. doi:10.1007/s11356-020-10181-9
Malone, L. A., Tregidga, E. L., Todd, J. H., Burgess, E. P., Philip, B. A., Markwick, N. P., et al. (2002). Effects of ingestion of a biotin-binding protein on adult and larval honey bees. Apidologie 3, 447–458. doi:10.1051/apido:2002030
Mao, W., Schuler, M. A., and Berenbaum, M. R. (2013). Honey constituents up-regulate detoxification and immunity genes in the Western honey bee Apis mellifera. PNAS 110, 8842–8846. doi:10.1073/pnas.1303884110
Mayer, D. F., and Lunden, J. D. (1986). Toxicity of fungicides and an acaricide to honey bees (Hymenoptera: Apidae) and their effects on bee foraging behavior and pollen viability on blooming apples and pears. Environ. Entomol. 15, 1047–1049. doi:10.1093/ee/15.5.1047
McArt, S. H., Fersch, A. A., Milano, N. J., Truitt, L. L., and Böröczky, K. (2017). High pesticide risk to honey bees despite low focal crop pollen collection during pollination of a mass blooming crop. Sci. Rep. 7, 46554. doi:10.1038/srep46554
Mommaerts, V., and Smagghe, G. (2011). “Side-effects of pesticides on the pollinator bombus: An overview,” in Pesticides of the modern world. Editor M. Stoytcheva (Rijeka: InTech), 507–552.
Mullin, C. A., Frazier, M., Frazier, J. L., Ashcraft, S., Simonds, R., Vanengelsdorp, D., et al. (2010). High levels of miticides and agrochemicals in north American apiaries: Implications for honey bee health. PloS ONE 5, e9754. doi:10.1371/journal.pone.0009754
Mussen, E. C., Lopez, J. E., and Peng, C. Y. (2004). Effects of selected fungicides on growth and development of larval honey bees, Apis mellifera L. (Hymenoptera: Apidae). Environ. Entomol. 33, 1151–1154. doi:10.1603/0046-225X-33.5.1151
Negri, P., Maggi, M. D., Ramirez, L., De Feudis, L., Szwarski, N., Quintana, S., et al. (2015). Abscisic acid enhances the immune response in Apis mellifera and contributes to the colony fitness. Apidologie 46, 542–557. doi:10.1007/s13592-014-0345-7
Negri, P., Quintana, S., Maggi, M., Szawarski, N., Lamattina, L., and Eguaras, M. (2014). Apis mellifera hemocytes generate increased amounts of nitric oxide in response to wounding/encapsulation. Apidologie 45, 610–617. doi:10.1007/s13592-014-0279-0
Pal, E., Almasri, H., Paris, L., Diogon, M., Pioz, M., Cousin, M., et al. (2022). Toxicity of the pesticides imidacloprid, difenoconazole and glyphosate alone and in binary and ternary mixtures to winter honey bees: Effects on survival and antioxidative defenses. Toxics 10, 104. doi:10.3390/toxics10030104
Pamminger, T., Botías, C., Goulson, D., and Hughes, W. O. (2018). A mechanistic framework to explain the immunosuppressive effects of neurotoxic pesticides on bees. Funct. Ecol. 32, 1921–1930. doi:10.1111/1365-2435.13119
Partap, U. (2011). “The pollination role of honey bees,” in Honey bees of asia. Editors H. Hepburn, and S. Radloff (Berlin, Heidelberg: Springer), 227–255. doi:10.1007/978-3-642-16422-4_11
Perveen, N., and Ahmad, M. (2017). Toxicity of some insecticides to the haemocytes of giant honey bee, Apis dorsata F. under laboratory conditions. Saudi J. Boil. Sci. 24, 1016–1022. doi:10.1016/j.sjbs.2016.12.011
Piechowicz, B., Woś, I., Podbielska, M., and Grodzicki, P. (2018). The transfer of active ingredients of insecticides and fungicides from an orchard to beehives. J. Environ. Sci. Health B 53, 18–24. doi:10.1080/03601234.2017.1369320
Rennich, K., Pettis, J., van Engelsdorp, D., Bozarth, R., Eversole, H., Roccasecca, K., et al. (2012). 2011–2012 National honey bee pests and diseases survey report. USDA. Available at: http://www.aphis.usda.gov/plant_health/plant_pest_info/honey_bees/downloads/2011_National_Survey_Report.pdf (Accessed September 20, 2022).
Richardson, R. T., Ballinger, M. N., Qian, F., Christman, J. W., and Johnson, R. M. (2018). Morphological and functional characterization of honey bee, Apis mellifera, hemocyte cell communities. Apidologie 49, 397–410. doi:10.1007/s13592-018-0566-2
Rişcu, A., and Bura, M. (2013). The impact of pesticides on honey bees and hence on humans. Sci. Pap. Animal Sci. Biotechnol. 46, 272–277.
Roberts, T. R., Hutson, D. H., Jewess, P. J., Lee, P., and Nicholls, P. H. (2007). “Metabolic pathways of agrochemicals,” in Insecticides and fungicides (Cambridge: Royal Society of Chemistry).
Rondeau, S., and Raine, N. E. (2022). Fungicides and bees: A review of exposure and risk. Environ. Int. 165, 107311. doi:10.1016/j.envint.2022.107311
Rortais, A., Arnold, G., Halm, M. P., and Touffet-Briens, F. (2005). Modes of honey bees exposure to systemic insecticides: Estimated amounts of contaminated pollen and nectar consumed by different categories of bees. Apidologie 36, 71–83. doi:10.1051/apido:2004071
Santos, C. G., and Hartfelder, K. (2015). Insights into the dynamics of hind leg development in honey bee (Apis mellifera L.) queen and worker larvae-A morphology/differential gene expression analysis. Genet. Mol. Biol. 38, 263–277. doi:10.1590/S1415-475738320140393
Sauthier, R., I’Anson Price, R., and Grüter, C. (2017). Worker size in honey bees and its relationship with season and foraging distance. Apidologie 48, 234–246. doi:10.1007/s13592-016-0468-0
Serra, R. S., Martínez, L. C., Cossolin, J. F. S., Resende, M. T. C. S. D., Carneiro, L. S., Fiaz, M., et al. (2023). The fungicide azoxystrobin causes histopathological and cytotoxic changes in the midgut of the honey bee Apis mellifera (Hymenoptera: Apidae). Ecotoxicology 32, 234–242. doi:10.1007/s10646-023-02633-y
Shawer, M. B., Taha, E. K. A., Mousa, K. M., Khan, K. A., Ibrahim, S., Hassan, S., et al. (2021). Seasonal variations of colony activities linked to morphometric and glands characterizations of hybrid Carniolan honey bee (Apis mellifera carnica Pollmann) workers. J. King Saud. Univ. Sci. 33, 101543. doi:10.1016/j.jksus.2021.101543
Siefert, P., Hota, R., Ramesh, V., and Grünewald, B. (2020). Chronic within-hive video recordings detect altered nursing behaviour and retarded larval development of neonicotinoid treated honey bees. Sci. Rep. 10 (1), 8727. doi:10.1038/s41598-020-65425-y
Sies, H. (2000). “What is oxidative stress?,” in Oxidative stress and vascular disease. Editor J. F. Keaney (Boston, MA: Springer), 1–8.
Smirle, M. J. (1993). The influence of colony population and brood rearing intensity on the activity of detoxifying enzymes in worker honey bees. Physiol. Entomol. 18, 420–424. doi:10.1111/j.1365-3032.1993.tb00616.x
Stoner, K. A., and Eitzer, B. D. (2013). Using a hazard quotient to evaluate pesticide residues detected in pollen trapped from honey bees (Apis mellifera) in ConnecticutApis mellifera) in Connecticut. PLoS ONE 8, e77550. doi:10.1371/journal.pone.0077550
Sukkar, D., Laval-Gilly, P., Bonnefoy, A., Malladi, S., Azoury, S., Kanso, A., et al. (2023). Differential production of nitric oxide and hydrogen peroxide among Drosophila melanogaster, Apis mellifera, and Mamestra brassicae immune-activated hemocytes after exposure to imidacloprid and amitraz. Insects 14, 174. doi:10.3390/insects14020174
Tamburini, G., Wintermantel, D., Allan, M. J., Dean, R. R., Knauer, A., Albrecht, M., et al. (2021). Sulfoxaflor insecticide and azoxystrobin fungicide have no major impact on honey bees in a realistic-exposure semi-field experiment. Sci. Total Environ. 778, 146084. doi:10.1016/j.scitotenv.2021.146084
Thompson, L. J., Stout, J. C., and Stanley, D. A. (2023). Contrasting effects of fungicide and herbicide active ingredients and their formulations on bumblebee learning and behaviour. J. Exp. Biol. 226, jeb245180. doi:10.1242/jeb.245180
Uckan, F., and Sak, O. (2010). Cytotoxic effect of cypermethrin on Pimpla turionellae (Hymenoptera: Ichneumonidae) larval hemocytes. Ekoloji 19, 20–26. doi:10.5053/ekoloji.2010.753
Worden, B. D., Skemp, A. K., and Papaj, D. R. (2005). Learning in two contexts: The effects of interference and body size in bumblebees. J. Exp. Biol. 208, 2045–2053. doi:10.1242/jeb.01582
Wu, G., Fang, Y. Z., Yang, S., Lupton, J. R., and Turner, N. D. (2004). Glutathione metabolism and its implications for health. J. Nutr. 134, 489–492. doi:10.1093/jn/134.3.489
Wu, J. Y., Anelli, C. M., and Sheppard, W. S. (2011). Sub-lethal effects of pesticide residues in brood comb on worker honey bee (Apis mellifera) development and longevity. PloS ONE 6, e14720. doi:10.1371/journal.pone.0014720
Xiao, J., He, Q., Liu, Q., Wang, Z., Yin, F., Chai, Y., et al. (2022). Analysis of honey bee exposure to multiple pesticide residues in the hive environment. Sci. Total Environ. 805, 150292. doi:10.1016/j.scitotenv.2021.150292
Xiong, M., Qin, G., Wang, L., Wang, R., Zhou, R., Luo, X., et al. (2023). Field recommended concentrations of pyraclostrobin exposure disturb the development and immune response of worker bees (Apis mellifera L.) larvae and pupae. Front. Physiol. 14, 1137264. doi:10.3389/fphys.2023.1137264
Keywords: honey bee, fungicide, granulocyte, larvae, morphology, survival
Citation: Kaur G, Singh A, Sharma R, Thakur A, Tuteja S, Shyamli and Singh R (2023) Effect of fungicidal contamination on survival, morphology, and cellular immunity of Apis mellifera (Hymenoptera: Apidae). Front. Physiol. 14:1099806. doi: 10.3389/fphys.2023.1099806
Received: 16 November 2022; Accepted: 10 April 2023;
Published: 25 April 2023.
Edited by:
Khalid Ali Khan, King Khalid University, Saudi ArabiaReviewed by:
El-Kazafy Abdou Taha, Kafrelsheikh University, EgyptCopyright © 2023 Kaur, Singh, Sharma, Thakur, Tuteja, Shyamli and Singh. This is an open-access article distributed under the terms of the Creative Commons Attribution License (CC BY). The use, distribution or reproduction in other forums is permitted, provided the original author(s) and the copyright owner(s) are credited and that the original publication in this journal is cited, in accordance with accepted academic practice. No use, distribution or reproduction is permitted which does not comply with these terms.
*Correspondence: Randeep Singh, c2luZ2hyYW5kZWVwODNAaG90bWFpbC5jb20=
Disclaimer: All claims expressed in this article are solely those of the authors and do not necessarily represent those of their affiliated organizations, or those of the publisher, the editors and the reviewers. Any product that may be evaluated in this article or claim that may be made by its manufacturer is not guaranteed or endorsed by the publisher.
Research integrity at Frontiers
Learn more about the work of our research integrity team to safeguard the quality of each article we publish.