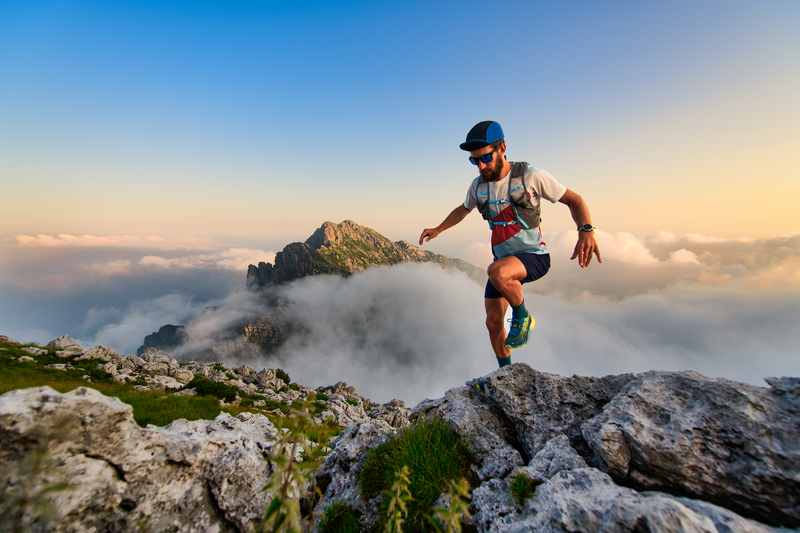
94% of researchers rate our articles as excellent or good
Learn more about the work of our research integrity team to safeguard the quality of each article we publish.
Find out more
ORIGINAL RESEARCH article
Front. Physiol. , 17 January 2023
Sec. Computational Physiology and Medicine
Volume 14 - 2023 | https://doi.org/10.3389/fphys.2023.1090696
This article is part of the Research Topic Advances in Basic and Applied Research in Photoplethysmography View all 17 articles
Pain affects every fifth adult worldwide and is a significant health problem. From a physiological perspective, pain is a protective reaction that restricts physical functions and causes responses in physiological systems. These responses are accessible for evaluation via recorded biosignals and can be favorably used as feedback in active pain therapy via auricular vagus nerve stimulation (aVNS). The aim of this study is to assess the significance of diverse parameters of biosignals with respect to their deflection from cold stressor to deep breathing and their suitability for use as biofeedback in aVNS stimulator. Seventy-eight volunteers participated in two cold pressors and one deep breathing test. Three targeted physiological parameters (RR interval of electrocardiogram, cardiac deflection magnitude ZAC of ear impedance signal, and cardiac deflection magnitude PPGAC of finger photoplethysmogram) and two reference parameters (systolic and diastolic blood pressures BPS and BPD) were derived and monitored. The results show that the cold water decreases the medians of targeted parameters (by 5.6, 9.3%, and 8.0% of RR, ZAC, and PPGAC, respectively) and increases the medians of reference parameters (by 7.1% and 6.1% of BPS and BPD, respectively), with opposite changes in deep breathing. Increasing pain level from relatively mild to moderate/strong with cold stressor varies the medians of targeted and reference parameters in the range from 0.5% to 6.0% (e.g., 2.9% for RR, ZAC and 6.0% for BPD). The physiological footprints of painful cold stressor and relaxing deep breathing were shown for auricular and non-auricular biosignals. The investigated targeted parameters can be used as biofeedback to close the loop in aVNS to personalize the pain therapy and increase its compliance.
Acute or chronic pain is one of the main complaints for seeking medical care. According to the International Association for the Study of Pain, pain is defined as “unpleasant sensory and emotional experience associated with, or resembling that associated with, actual or potential tissue damage” Raja et al. (2020). Pain is a protective reaction restricting physical functions with various physiological parameters, such as heart rate, respiratory rate, and arterial blood pressure, which are potential indicators of pain intensity Arbour et al. (2014); Cowen et al. (2015); Peters and Schmidt (1991). The specific changes can be observed by the reactivity and reflexivity of the autonomic nervous, cardiovascular, and respirator systems Kyle and McNeil (2014).
Adults and children suffer the pain associated with different medical conditions, undergo different painful procedures, or are referred with acute pain to the emergency department Dahlhamer et al. (2018); Keating and Smith (2011); Mura et al. (2017); Othow et al. (2022). Data suggest that 7 out of 10 patients come to the emergency department due to pain Todd et al. (2007). Meanwhile, chronic pain affects about 20.5% of adults in the United States Yong et al. (2022) and about 10%–30% in Europe Breivik et al. (2006); Reid et al. (2011). The meta-analysis showed that the prevalence of chronic pain ranges between 0% and 24% globally Mansfield et al. (2016).
Different medications, such as non-steroidal anti-inflammatory drugs, opioids, or others, are used daily against pain. Despite various pain medications and strategies, pain treatment faces many adversities Fishman (2007), such as severe side effects, the use of illicit drugs, opioid crisis St. Marie and Broglio (2020), peptic ulcers Tai and McAlindon (2021), and others. All the more, pain management is a fundamental human right Enright and Goucke (2016); Fishman (2007).
Vagal nerve stimulation (VNS)—as a pain neuromodulation technique, as reviewed in Kaniusas et al. (2019a)—has been investigated in humans and animals. VNS can affect the autonomic nervous system and is an approved treatment for pharmacoresistant depression and drug-resistant epilepsy Nemeroff et al. (2006); O’Reardon et al. (2006). Non-invasive transcutaneous modalities of VNS emerge Busch et al. (2013); Nesbitt et al. (2015), such as the electrical stimulation of the external surface of the ear innervated by the afferent auricular branch of the vagus nerve, known as auricular vagus nerve stimulation (aVNS). aVNS is performed using miniature electrodes tightly fixed inside the auricular concha. The current intensity is individually adjusted at the beginning of the aVNS session to a level without evoking pain. However, the initial personalization of the intensity of current alone does not ensure adequate vagus nerve stimulation for the relatively long treatment duration (from days to weeks). Here habituation effects, varying physiology, and deterioration of the electrode-tissue interface contribute to this uncertainty in the treatment Bouton (2017); Kaniusas (2019); Kaniusas et al. (2019b). Therefore, aVNS can be hypothesized to avoid under- or over-stimulation, reduce side effects, and save stimulation energy when based on individual physiological biofeedback.
Biofeedback can be assessed using data from internal (in-the-ear) and external (outside-the-ear) sensors, i.e., auricular and non-auricular biosignals. However, it is not known which biosignals and extracted parameters help estimate the balance between the stimulated parasympathetic system and the complementary sympathetic system, which is generally predominant in chronic ailments such as pain. In the ideal case, this balance should be provided to the aVNS stimulator to avoid the disadvantages of the non-personalized aVNS. Thus, easy-to-access biosignals are of high interest which could estimate this balance in favor of the efficiency of aVNS therapy.
The present study proposes a cold stressor as a sympathetically driven stimulus (usually accompanied by acute pain) and deep breathing as a mainly parasympathetically driven stimulus (with relaxing effects) to manipulate the sympathovagal balance from sympathetic to parasympathetic dominance while recording a set of auricular and non-auricular biosignals. The aim of this study is to assess the significance of diverse parameters of biosignals with respect to their deflection from cold stressor to deep breathing and their suitability for use as biofeedback in aVNS stimulator.
Seventy-eight healthy volunteers (36 women), 32.6 ± 10.7 years old (range 20–64 years, with 23 men and 19 women
Data collection took place indoors at the Biomedical Engineering Institute (Kaunas, Lithuania) in a quiet and temperature-controlled (24.0°C ± 1.0°C) laboratory at the same time of the day (08:00–13:00) to minimize the circadian influence. Four synchronous biosignals were recorded in the study, as illustrated in Figure 1: 1) a modified bipolar three-lead electrocardiogram (ECG) signal (sampling rate 2 kHz); 2) a red wavelength finger photoplethysmogram (PPG) signal (sampling rate 1 kHz) using a proprietary multimodal signals recording system Nautilus II (Biomedical Engineering Institute, Kaunas, Lithuania); 3) an ear impedance signal (at the frequency of 12.5 kHz, sampling rate 1 kHz) using the data acquisition system Biopac MP150 (Biopac Systems Inc., Aero Camino, Goleta, CA, United States); and 4) arterial blood pressure signal (sampling rate 100 Hz) using the non-invasive arterial blood pressure monitoring system CNAP Monitor 500 (CNSystems, Graz, Austria). The subjective/perceived pain was recorded by a volunteer self-report (announced verbally and aloud to an experimenter) using the numerical rating scale NRS (range 0–100, with 0 for no pain and 100 for unbearable pain) at least every 30 s (or even more often based on a volunteer’s initiative).
FIGURE 1. Placement of all sensors and electrodes for the recording of electrocardiogram ECG(t), pulse plethysmogram PPG(t), auricular impedance Z(t), and blood pressure BP(t) signals.
Well-known and effective pain-causing (the cold pressor test) and relaxation (deep breathing) tests were used in the study. Namely, the study protocol consisted of eight phases, as depicted in Figure 2A: 1) the first rest phase (Rest #1) lasting 10 min in the sitting position; 2) the warm water for 1 min (equalization phase), in which the participant immersed his left hand into warm water (32.0°C ± 0.1°C); 3) the first cold water phase (CPT #1), in which the participant immersed his left hand into cold water (7.0°C ± 0.1°C) for 2 min or even shorter if the volunteer felt very uncomfortable and voluntarily resumed; 4) the second rest phase (Rest #2) for 5 min where the participant took his left hand out from cold water and rested in the sitting position; 5) the second cold water phase (CPT #2), in which the participant immersed his left hand into a little less cold water (10.0°C ± 0.1°C) for 2 min or even shorter if the volunteer felt very uncomfortable and voluntarily resumed; 6) the third rest phase (Rest #3) for 10 min in analogy with Rest #2; 7) the deep breathing phase (DB) for 1 min with the paced breathing rate 6 1/min (paced via a monitor and a bar rising/falling periodically every 10 s); 8) the fourth rest phase (Rest #4) for 5 min in analogy with Rest #2. Participants were verbally instructed to immerse their left hand (up to the middle of the forearm) in warm or cold water, indicate their subjective pain level (in cold water), and take out their hand after 1 min in warm and 2 min in cold water.
The study was conducted following the ethical principles of the Declaration of Helsinki and with ethics approval from the Kaunas Region Biomedical Research Ethics Committee (No. BE-2-24), including informed consent and voluntary participation. Personal information was removed from the collected data to ensure participants’ anonymity.
ECG was filtered using zero-phase Butterworth high-pass and low-pass filters (cut-off frequencies 0.5 and 35 Hz, respectively), R waves were detected using the modified Tompkins algorithm Hamilton and Tompkins (1986), and RR was estimated as the time interval between the successive R peaks. The ear impedance signal reflects local changes in the blood perfusion and blood vessel size, accounting for the local changes in capacitance and resistance. The impedance signal is morphologically similar to PPG so that both PPG and Z were filtered using high-pass and low-pass zero-phase Butterworth filters (cut-off frequencies 0.5 and 10 Hz, respectively). The associated peak and valley fiducial points in PPG, Z, and BP signals were detected in line with the detected R waves of ECG. Five parameters were extracted out of the four recorded biosignals (Figure 3): 1) time interval RR between R peaks of ECG; 2) cardiac deflection magnitude PPGAC of PPG; 3) cardiac deflection magnitude ZAC of ear impedance signal; 4) systolic blood pressure BPS; and 5) diastolic blood pressure BPD. Please note that the analyzed PPGAC is mainly related to the pulsatile arterial blood, proportional to the local systolic-diastolic deflection of the blood pressure and the arterial compliance of the vascular wall Kaniusas (2015).
FIGURE 3. Instructive qualitative changes of the interbeat period RR, the pulse plethysmography PPG, bioimpedance Z, and blood pressure BP from the first rest phase (Rest #1), the first cold water phase (CPT #1), and the deep breathing phase (DB) of a single participant. The maximum reported NRS value of the CPT #1 was 80.
The entire periods of stimulation phases CPT #1, CPT #2, and DB were included in the analysis, only the last 4 min of rest phases Rest #1, Rest #2, and Rest #3 were included to avoid the transient influence of the preceding phase (Figure 2B). The medians of evaluated parameters from CPT #1, CPT #2, and DB phases were compared with the medians of the respective Rest #1, Rest #2, and Rest #3 phases, without any averaging. The analysis was performed using different pain levels, ages, and gender. The pain level threshold was chosen at 40 points, corresponding to mild pain Karcioglu et al. (2018). The age threshold was selected at 30 years in order to end up with comparably populated groups of men and women.
The Shapiro-Wilk test was used to assess data normality. Because of the non-normal distribution, the results are summarized using boxplots with medians and quartiles. The Wilcoxon signed-rank test with the Bonferroni’s adjustment for dependent samples was used to compute the p-value, and statistical significance was set at p
Out of 78 recorded data sets, two ECG, thirteen PPG, twenty-four Z, and six BP traces were eliminated from the analysis due to poor quality. Eight participants retreated earlier and did not finish the CPT #1 phase, i.e., two women (age ≤30 years), two women (age
Figure 3 illustrates the temporal courses of RR, PPG, Z, and BP during phases Rest #1, CPT #1, and DB. Compared to Rest #1, the cold stimulus CPT #1 shows reduced both RR and its variability, as well as reduced cardiac deflection magnitude PPGAC of PPG and reduced cardiac deflection ZAC of Z. The associated mean BP is larger during CPT #1 than during Rest #1. The subsequent DB phase contrasts CPT #1 in that PPGAC and ZAC increase in DB. The respiration-related variability of all four RR, PPGAC, ZAC, and BP dominates in DB, with the indicated respiration rate fR (Figure 3).
As shown in Figure 4, the first cold water stimulation CPT #1 decreases the median of RR (−5.5%) and ZAC (−9.8%) while increasing that of BPS (+12.6%) and BPD (+13.4%) of BP, as compared with the first rest phase Rest #1. Here the associated PPGAC remains almost constant (+0.9%). The second cold water stimulation CPT #2 decreases the median of RR (−5.6%), PPGAC (−8.0%), and ZAC (−9.3%) while increasing that of BPS (+7.1%) and BPD (+6.1%), as compared with the second rest phase Rest #2. The subsequent deep breathing DB produces opposite effects: the median of RR (+1.8%), PPGAC (+5.1%), and ZAC (+5.4%) increase, while that of BPS (−0.9%) and BPD (−5.6%) decrease, as compared with the third rest phase Rest #3. The observed changes in DB are significantly different compared to CPT #2 in all five parameters.
FIGURE 4. Relative changes (A) ΔRR of RR, (B) ΔPPGAC of PPGAC, (C) ΔZAC of ZAC, (D) ΔBPS of systolic BP values, and (E) ΔBPD of diastolic BP values from CPT #1, CPT #2, and DB as related to the respective Rest #1, Rest #2, and Rest #3. The asterisk ”*” indicates significant changes (p
Figure 5 summarizes and contrasts the observed changes for all parameters in CPT #2 (Figure 5A) versus DB (Figure 5B), with the indicated interquartile range from 25% to 75%. In line with Figure 4, CPT #2 reduces RR, PPGAC, and ZAC and increases BPS and BPD, whereas DB causes physiological processes with reversed tendencies, i.e., RR, PPGAC, and ZAC increase while BPS and BPD decrease.
FIGURE 5. Medians and interquartile ranges of ΔRR, ΔPPGAC, ΔZAC, ΔBPS, and ΔBPD (compare Figure 4) during (A) CPT #2 and (B) DB.
Figure 6 illustrates the relative changes in the parameters in CPT #2 for relatively mild pain with the associated NRS ≤40 (Figure 6A) in comparison with moderate to strong pain with NRS
FIGURE 6. Median and interquartile ranges of ΔRR, ΔPPGAC, ΔZAC, ΔBPS, and ΔBPD (compare Figure 4) during CPT #2 for (A) mild pain with NRS ≤40 and (B) moderate to severe pain with NRS
The influence of gender and age is depicted in Figure 7 considering CPT #2 and DB (compare Figure 5). In CPT #2, the relative values of ΔRR decrease by 1.5%–8.4%, with a minor decrease for young men (
FIGURE 7. Medians of ΔRR, ΔPPGAC, ΔZAC, ΔBPS, and ΔBPD (compare Figure 4) differentiated by age and gender during (A) CPT #2 and (B) DB.
During DB, in line with Figure 5, the observed changes in all gender and age groups mainly follow the opposite behavior. ΔRR increases by 2.1%–4.5% except for adult men (≥30 years); ΔPPGAC increases by 4.5%–12.1% but also except for adult men; ΔZAC increases by 2.5%–9.6% with a minor increase for young men (
The present study investigates the physiological footprints of auricular and non-auricular biosignals in response to a cold stressor and deep breathing. While a cold stressor is a sympathetically driven stimulus (accompanied by acute pain), deep breathing is a mainly parasympathetically driven stimulus (with relaxing effects). Thus, it was investigated how the opposing sympathetic and parasympathetic stimuli are reflected by the auricular biosignals, namely, its parameter ZAC, and by parameters accessible from the auricular biosignals such as RR and PPGAC. All these three parameters can be used as biofeedback to close the loop in aVNS, i.e., in a targeted stimulation of the parasympathetic system. The closed-loop set-up personalizes aVNS with an expected tendency to avoid over and under-stimulation of the vagus nerve/parasympathetic system. Thus, the closed-loop aVNS may minimize both the energy consumption of the aVNS stimulator and potential side effects (no over-stimulation) while optimizing and personalizing the aVNS therapy (no recruitment of pain fibers), e.g., in chronic pain. Here, the non-auricular biosignals with their parameters BPS and BPD serve as a necessary reference to monitor stimuli-related vital functions of the body and as an instructive substrate for their comparison with stimuli-related changes in auricular biosignals.
The auricular biosignal Z, namely its parameter ZAC (Figure 1), decreases significantly during the sympathetic stimulus (CPT #2) as compared with the parasympathetic one (DB) (Figures 3–5), as well as decreases tendentially with increasing pain perception (Figure 6). This behavior indicates the potential suitability of ZAC in assessing changes in the balance of the parasympathetic and sympathetic stimuli, or, more generally, in the balance of the parasympathetic and sympathetic systems of the human body (sympathovagal balance). On the other hand, this balance, especially its normalization from a derailed state, is usually a therapeutic target in aVNS when applied to different chronic ailments Kaniusas et al. (2019a). Thus ZAC can be hypothesized to be reasonable auricular biofeedback for the closed-loop aVNS without using any sensors external to the ear, which may obstruct the patient.
The parameters RR and PPGAC also reflect sympathovagal balance. RR and PPGAC decrease significantly during the sympathetic CPT #2 compared to the parasympathetic DB (Figures 3–5). While RR tends to decrease with increasing pain, the level of PPGAC does not (Figure 6). Therefore, RR and PPGAC, the former to a larger extent, can also be hypothesized to be reasonable auricular biofeedback for the closed-loop aVNS targeting a derailed sympathovagal balance. Please note that RR could be estimated from the period of the cardiac oscillation of the auricular Z (Figure 3), whereas PPGAC from the cardiac deflection of PPG from the earlobe Allen (2007). However, limitations in the precision of the estimated RR may apply in the former case due to a rather smooth waveform of Z in contrast to the spiky R peak of ECG. Likewise, limitations in PPGAC may apply in the latter case due to a rather central connection of the ear perfusion in contrast to the peripheral perfusion of the finger (Figure 1).
The non-auricular parameters BPS and BPD reflect the sympathovagal balance as well. Both increase significantly during the sympathetic CPT #2 as compared with the parasympathetic DB (Figures 3–5), while this increase in CPT #2 tends to be larger for stronger pain (Figure 6). The level of BPD appears to depend even stronger on the stimuli-induced sympathovagal balance with the observed changes of 11.7% (from CPT #2 to DB) in contrast to the associated changes in BPS of 8.0% (Figure 4). Likewise, the sympathetically-governed vasoconstriction (governing BPD) may be more dominant than stroke volume changes (governing BPS) Kaniusas (2012). This leads to a hypothesis that BPS and BPD could be used as non-auricular biofeedback for the closed-loop aVNS when external sensors are used outside the ear.
In terms of gender and age, the largest changes from CPT #2 to DB were shown in RR for adult women (≥30 years), PPGAC for young women (
A limitation of the present study is the relatively small database of recordings representing the elder part of the population which has tendentially a larger prevalence of suffering pain. Collecting and analyzing a more representative database is planned as a future research direction in the research of the aVNS stimulator. Since the warm water phase immediately preceding CPT #1 strongly affected the results in CPT #1, we focused our investigations on the comparison of CPR #2 and DB, both preceded by rest phases. Another limitation is that the order of the different phases of the protocol were not randomized, especially the order of CPT and DB. Therefore, the results may have been influenced by other factors such as expectation, adaptation, prolonged exposure.
Lastly, it should be noted that the recorded pain level, in contrast to nociception with its physiological encoding and processing of nociceptive stimuli, is a subjective feeling connected with the emotional experience to impeding or actual harm Loeser and Treede (2008) but also altering autonomic nervous system Woo et al. (2017); Adamczyk et al. (2020); Abdallah and Geha (2017). Thus, the investigated objective characteristics of the autonomic system may be useful for a continuous and objective personalization of aVNS in chronic ailments such as pain.
The three parameters RR, PPGAC, and ZAC accessible from auricular biosignals reflect the artificially-induced stimuli with sympathetic or parasympathetic dominance and thus the sympathovagal balance derailed in pain and other chronic conditions. Therefore, auricular biosignals can be used as biofeedback to close the loop in auricular vagus nerve stimulation to personalize the strength and timing of the stimulation in favor of therapy, patient compliance, and resourceful energy use.
The raw data supporting the conclusions of this article will be made available by the authors, without undue reservation.
The studies involving human participants were reviewed and approved by Kaunas Region Biomedical Research Ethics Committee (No. BE-2-24). The patients/participants provided their written informed consent to participate in this study.
AR contributed to the study design, collected data, developed algorithms, conducted data analysis, interpreted the results, and wrote and revised the manuscript. PP collected data, contributed to algorithm development, and revised the manuscript. LJ interpreted the results and revised the manuscript. VM contributed to the study design and revised the manuscript. EK initiated and designed the study, interpreted the results, and designed, wrote, and revised the manuscript. All authors have read the submitted manuscript and approved the final version.
This research was funded by the Research and Innovation Fund of Kaunas University of Technology (project grant No. PP2021/5) and the Research Fund of Lithuanian University of Health Sciences (2021-JV-00006). This study received funding from the European Union’s Horizon 2020 research and innovation program under grant agreement No. 880603 (SzeleSTIM GmbH). The funder SzeleSTIM GmbH had the following involvement with the study: the study design and collection of data. All authors declare no other competing interests.
The authors acknowledge TU Wien Bibliothek for financial support through its Open Access Funding Programme. We thank all the volunteers for their participation in the study.
The authors declare that the research was conducted in the absence of any commercial or financial relationships that could be construed as a potential conflict of interest.
The handling editor JA declared a shared research group VascAgeNet WG3 with the author VM at the time of review.
All claims expressed in this article are solely those of the authors and do not necessarily represent those of their affiliated organizations, or those of the publisher, the editors and the reviewers. Any product that may be evaluated in this article, or claim that may be made by its manufacturer, is not guaranteed or endorsed by the publisher.
Abdallah C. G., Geha P. (2017). Chronic pain and chronic stress: Two sides of the same coin? Chronic Stress 1, 2470547017704763. doi:10.1177/2470547017704763
Adamczyk A. K., Ligeza T. S., Wyczesany M. (2020). The dynamics of pain reappraisal: The joint contribution of cognitive change and mental load. Cognitive, Affect. Behav. Neurosci. 20, 276–293. doi:10.3758/s13415-020-00768-7
Allen J. (2007). Photoplethysmography and its application in clinical physiological measurement. Physiol. Meas. 28, R1–R39. doi:10.1088/0967-3334/28/3/R01
Arbour C., Choinière M., Topolovec-Vranic J., Loiselle C. G., Gélinas C. (2014). Can fluctuations in vital signs be used for pain assessment in critically ill patients with a traumatic brain injury? Pain Res. Treat. 2014, 175794. doi:10.1155/2014/175794
Bouton C. (2017). Cracking the neural code, treating paralysis and the future of bioelectronic medicine. J. Intern. Med. 282, 37–45. doi:10.1111/joim.12610
Breivik H., Collett B., Ventafridda V., Cohen R., Gallacher D. (2006). Survey of chronic pain in Europe: Prevalence, impact on daily life, and treatment. Eur. J. Pain 10, 287–333. doi:10.1016/j.ejpain.2005.06.009
Busch V., Zeman F., Heckel A., Menne F., Ellrich J., Eichhammer P. (2013). The effect of transcutaneous vagus nerve stimulation on pain perception-an experimental study. Brain Stimul. 6, 202–209. doi:10.1016/j.brs.2012.04.006
Cowen R., Stasiowska M. K., Laycock H., Bantel C. (2015). Assessing pain objectively: The use of physiological markers. Anaesthesia 70, 828–847. doi:10.1111/anae.13018
Dahlhamer J., Lucas J., Zelaya C., Nahin R., Mackey S., DeBar L., et al. (2018). Prevalence of chronic pain and high-impact chronic pain among adults — United States, 2016. MMWR. Morb. Mortal. Wkly. Rep. 67, 1001–1006. doi:10.15585/mmwr.mm6736a2
Edwards R. R., Fillingim R. B., Ness T. J. (2003). Age-related differences in endogenous pain modulation: A comparison of diffuse noxious inhibitory controls in healthy older and younger adults. Pain 101, 155–165. doi:10.1016/S0304-3959(02)00324-X
Enright A., Goucke R. (2016). The global burden of pain: The tip of the iceberg? Anesth. Analgesia 123, 529–530. doi:10.1213/ANE.0000000000001519
Fillingim R. B., King C. D., Ribeiro-Dasilva M. C., Rahim-Williams B., Riley J. L. (2009). Sex, gender, and pain: A review of recent clinical and experimental findings. J. Pain 10, 447–485. doi:10.1016/j.jpain.2008.12.001
Fishman S. M. (2007). Recognizing pain management as a human right: A first step. Anesth. Analgesia 105, 8–9. doi:10.1213/01.ane.0000267526.37663.41
Hamilton P. S., Tompkins W. J. (1986). Quantitative investigation of qrs detection rules using the mit/bih arrhythmia database. IEEE Trans. Biomed. Eng. BME- 33, 1157–1165. doi:10.1109/TBME.1986.325695
Kaniusas E. (2012). Biomedical signals and sensors I. Springer Berlin, Heidelberg. doi:10.1007/978-3-642-24843-6
Kaniusas E. (2015). Biomedical signals and sensors II. Springer Berlin, Heidelberg. doi:10.1007/978-3-662-45106-9
Kaniusas E. (2019). Biomedical signals and sensors III. Springer Cham. doi:10.1007/978-3-319-74917-4
Kaniusas E., Kampusch S., Tittgemeyer M., Panetsos F., Gines R. F., Papa M., et al. (2019a). Current directions in the auricular vagus nerve stimulation i – A physiological perspective. Front. Neurosci. 13, 854–923. doi:10.3389/fnins.2019.00854
Kaniusas E., Kampusch S., Tittgemeyer M., Panetsos F., Gines R. F., Papa M., et al. (2019b). Current directions in the auricular vagus nerve stimulation ii - an engineering perspective. Front. Neurosci. 13, 772. doi:10.3389/fnins.2019.00772
Karcioglu O., Topacoglu H., Dikme O., Dikme O. (2018). A systematic review of the pain scales in adults: Which to use? Am. J. Emerg. Med. 36, 707–714. doi:10.1016/j.ajem.2018.01.008
Keating L., Smith S. (2011). Acute pain in the emergency department: The challenges. Rev. Pain 5, 13–17. doi:10.1177/204946371100500304
Kyle B. N., McNeil D. W. (2014). Autonomic arousal and experimentally induced pain: A critical review of the literature. Pain Res. Manag. 19, 159–167. doi:10.1155/2014/536859
Loeser J. D., Treede R. D. (2008). The kyoto protocol of iasp basic pain terminology. Pain 137, 473–477. doi:10.1016/j.pain.2008.04.025
Mansfield K. E., Sim J., Jordan J. L., Jordan K. P. (2016). A systematic review and meta-analysis of the prevalence of chronic widespread pain in the general population. Pain 157, 55–64. doi:10.1097/j.pain.0000000000000314
Mogil J. S. (2012). Sex differences in pain and pain inhibition: Multiple explanations of a controversial phenomenon. Nat. Rev. Neurosci. 13, 859–866. doi:10.1038/nrn3360
Mura P., Serra E., Marinangeli F., Patti S., Musu M., Piras I., et al. (2017). Prospective study on prevalence, intensity, type, and therapy of acute pain in a second-level urban emergency department. J. Pain Res. 10, 2781–2788. doi:10.2147/JPR.S137992
Nemeroff C. B., Mayberg H. S., Krahl S. E., McNamara J., Frazer A., Henry T. R., et al. (2006). Vns therapy in treatment-resistant depression: Clinical evidence and putative neurobiological mechanisms. Neuropsychopharmacology 31, 1345–1355. doi:10.1038/sj.npp.1301082
Nesbitt A. D., Marin J. C., Tompkins E., Ruttledge M. H., Goadsby P. J. (2015). Initial use of a novel noninvasive vagus nerve stimulator for cluster headache treatment. Neurology 84, 1249–1253. doi:10.1212/WNL.0000000000001394
O’Reardon J. P., Cristancho P., Peshek A. D. (2006). Vagus nerve stimulation (vns) and treatment of depression: To the brainstem and beyond. Psychiatry (Edgmont (Pa, Townsh. 3, 54–63.
Othow C. O., Ferede Y. A., Tawuye H. Y., Aytolign H. A. (2022). The magnitude and associated factors of post-operative pain among adult patients. Ann. Med. Surg. 81, 104406. doi:10.1016/j.amsu.2022.104406
Peters M. L., Schmidt A. J. (1991). Psychophysiological responses to repeated acute pain stimulation in chronic low back pain patients. J. Psychosomatic Res. 35, 59–74. doi:10.1016/0022-3999(91)90007-B
Popescu A., Leresche L., Truelove E. L., Drangsholt M. T. (2010). Gender differences in pain modulation by diffuse noxious inhibitory controls: A systematic review. Pain 150, 309–318. doi:10.1016/j.pain.2010.05.013
Raja S. N., Carr D. B., Cohen M., Finnerup N. B., Flor H., Gibson S., et al. (2020). The revised international association for the study of pain definition of pain: Concepts, challenges, and compromises. Pain 161, 1976–1982. doi:10.1097/j.pain.0000000000001939
Reid K. J., Harker J., Bala M. M., Truyers C., Kellen E., Bekkering G. E., et al. (2011). Epidemiology of chronic non-cancer pain in Europe: Narrative review of prevalence, pain treatments and pain impact. Curr. Med. Res. Opin. 27, 449–462. doi:10.1185/03007995.2010.545813
Riley J. L., King C. D., Wong F., Fillingim R. B., Mauderli A. P. (2010). Lack of endogenous modulation and reduced decay of prolonged heat pain in older adults. Pain 150, 153–160. doi:10.1016/j.pain.2010.04.020
Rittger H., Rieber J., Breithardt O. A., Dücker M., Schmidt M., Abbara S., et al. (2011). Influence of age on pain perception in acute myocardial ischemia: A possible cause for delayed treatment in elderly patients. Int. J. Cardiol. 149, 63–67. doi:10.1016/j.ijcard.2009.11.046
St. Marie B., Broglio K. (2020). Managing pain in the setting of opioid use disorder. Pain Manag. Nurs. 21, 26–34. doi:10.1016/j.pmn.2019.08.003
Tai F. W. D., McAlindon M. E. (2021). Non-steroidal anti-inflammatory drugs and the gastrointestinal tract. Clin. Med. J. R. Coll. Physicians Lond. 21, 131–134. doi:10.7861/CLINMED.2021-0039
Todd K. H., Ducharme J., Choiniere M., Crandall C. S., Fosnocht D. E., Homel P., et al. (2007). Pain in the emergency department: Results of the pain and emergency medicine initiative (pemi) multicenter study. J. Pain 8, 460–466. doi:10.1016/j.jpain.2006.12.005
Woo C. W., Schmidt L., Krishnan A., Jepma M., Roy M., Lindquist M. A., et al. (2017). Quantifying cerebral contributions to pain beyond nociception. Nat. Commun. 8, 14211. doi:10.1038/ncomms14211
Keywords: auricular bioimpedance, auricular vagus nerve stimulation, blood pressure, cold pressor test, deep breathing, physiological biofeedback, photoplethysmography
Citation: Rapalis A, Piartli P, Jankauskaitė L, Marozas V and Kaniusas E (2023) Induced pain affects auricular and body biosignals: From cold stressor to deep breathing. Front. Physiol. 14:1090696. doi: 10.3389/fphys.2023.1090696
Received: 05 November 2022; Accepted: 06 January 2023;
Published: 17 January 2023.
Edited by:
John Allen, Coventry University, United KingdomReviewed by:
Youngsun Kong, University of Connecticut, United StatesCopyright © 2023 Rapalis, Piartli, Jankauskaitė, Marozas and Kaniusas. This is an open-access article distributed under the terms of the Creative Commons Attribution License (CC BY). The use, distribution or reproduction in other forums is permitted, provided the original author(s) and the copyright owner(s) are credited and that the original publication in this journal is cited, in accordance with accepted academic practice. No use, distribution or reproduction is permitted which does not comply with these terms.
*Correspondence: Eugenijus Kaniusas, ZXVnZW5panVzLmthbml1c2FzQHR1d2llbi5hYy5hdA==
Disclaimer: All claims expressed in this article are solely those of the authors and do not necessarily represent those of their affiliated organizations, or those of the publisher, the editors and the reviewers. Any product that may be evaluated in this article or claim that may be made by its manufacturer is not guaranteed or endorsed by the publisher.
Research integrity at Frontiers
Learn more about the work of our research integrity team to safeguard the quality of each article we publish.