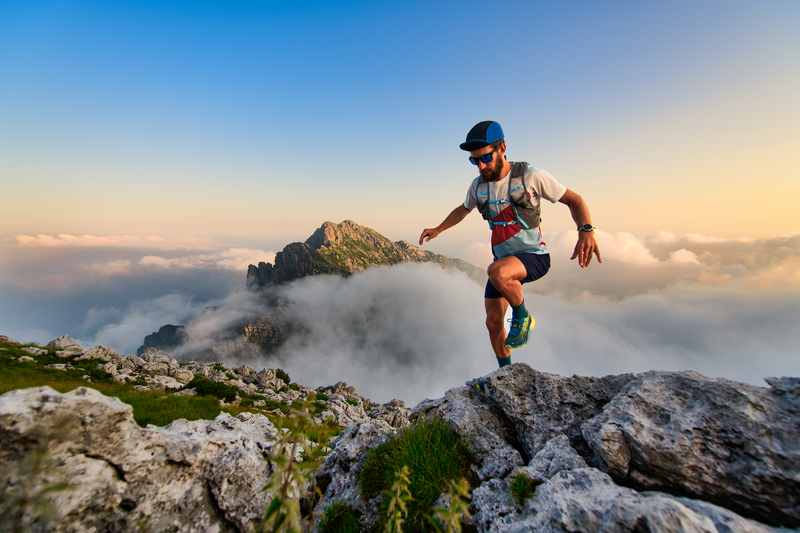
95% of researchers rate our articles as excellent or good
Learn more about the work of our research integrity team to safeguard the quality of each article we publish.
Find out more
ORIGINAL RESEARCH article
Front. Physiol. , 24 January 2023
Sec. Avian Physiology
Volume 14 - 2023 | https://doi.org/10.3389/fphys.2023.1079638
This article is part of the Research Topic Women in Avian Physiology: 2023 View all 13 articles
Optimal embryonic development and growth of meat-type chickens (broilers) rely on incubation conditions (oxygen, heat, and humidity), on nutrients and on energy resources within the egg. Throughout incubation and according to the embryo’s energy balance, the main energy storage molecules (creatine and glycogen) are continuously utilized and synthesized, mainly in the embryonic liver, breast muscle, and the extraembryonic yolk sac (YS) tissue. During the last phase of incubation, as the embryo nears hatching, dynamic changes in energy metabolism occur. These changes may affect embryonic survival, hatchlings’ uniformity, quality and post hatch performance of broilers, hence, being of great importance to poultry production. Here, we followed the dynamics of creatine and glycogen from embryonic day (E) 11 until hatch and up to chick placement at the farm. We showed that creatine is stored mainly in the breast muscle while glycogen is stored mainly in the YS tissue. Analysis of creatine synthesis genes revealed their expression in the liver, kidney, YS tissue and in the breast muscle, suggesting a full synthesis capacity in these tissues. Expression analysis of genes involved in gluconeogenesis, glycogenesis, and glycogenolysis, revealed that glycogen metabolism is most active in the liver. Nevertheless, due to the relatively large size of the breast muscle and YS tissue, their contribution to glycogen metabolism in embryos is valuable. Towards hatch, post E19, creatine levels in all tissues increased while glycogen levels dramatically decreased and reached low levels at hatch and at chick placement. This proves the utmost importance of creatine in energy supply to late-term embryos and hatchlings.
The phase of embryonic development has great importance to the production chain of meat-type chickens (broilers) as the 21-day of incubation constitutes more than a third of the broiler lifespan, starting with incubation and ending with marketing at 35–42 days (Havenstein et al., 2003a; Havenstein et al., 2003b; Zuidhof et al., 2014; Givisiez et al., 2020). During the period of incubation, in order to ensure optimal development and growth, avian embryos depend on levels of oxygen, heat and humidity together with the supply of nutrients and energy (Decuypere et al., 2001; Moran, 2007; De Oliveira et al., 2008). Embryos utilize the nutrients within the egg to form high energy-value compounds such as glycogen and creatine with the final aim of ATP production (Christensen et al., 1999; 2000; 2001; Moran, 2007).
Glycogen is the main storage form of glucose in tissues, its high-energetic value is derived from the potential to release glucose and the production of ATP by aerobic respiration or by anaerobic glycogen breakdown (glycolysis). Two key enzymes are involved in glycogen metabolism; glycogen synthase (GYS), a rate limiting enzyme in glycogen synthesis, and glycogen phosphorylase L (PYGL), which catalyzes the release of glucose-1-phosphate from glycogen (Blaxter, 1989; Scanes, 2022). During most of incubation period, up to embryonic day (E) 19, gas exchange is achieved through chorioallantoic respiration and oxygen is sufficient to support aerobic processes of energy metabolism and lipid oxidation (Tazawa et al., 1983; Decuypere et al., 2001; Moran, 2007). Along this period, sufficient glucose is produced, and the unutilized glucose is funneled to storage, i.e., glycogen synthesis in the embryonic liver, muscles, and the extraembryonic yolk sac (YS) tissue (Romanoff, 1960; Romanoff, 1967; Noble and Cocchi, 1990; Speake et al., 1998; Yadgary and Uni, 2012; Wong and Uni, 2021). Post E19, prior to the initiation of the hatching process, oxygen levels become limiting. At this phase, energy production in the embryo switches from lipid oxidation to anaerobic catabolism, by the breakdown of glycogen to glucose and by gluconeogenesis with the breakdown of amino acids for glucose and ATP production. The latter is apparent with the activation of two key gluconeogenic enzymes; fructose-1,6-bisphosphatase 1 (FBP1), which catalyzes the hydrolysis of fructose 1,6-bisphosphate to fructose 6-phosphate, and glucose-6-phosphatase 2 (G6PC2), which catalyzes the hydrolysis of glucose-6-phosphate to glucose and inorganic phosphate, leading to the release of glucose into the bloodstream. By the time the embryo hatches, almost all glycogen is exploited, and its stores are depleted (Uni and Ferket, 2004; De Oliveira et al., 2008; Yadgary and Uni, 2012).
Creatine, another high-energy value molecule, is deposited by the hen in the egg compartments (yolk and albumen) where it is available to the embryo (Reicher et al., 2020). However, as only small amounts are deposited, the embryo relies also on de novo synthesis of creatine during incubation (Walker, 1979). Creatine synthesis involves two main steps driven by two enzymes. The first enzyme, L-arginine: glycine amidinotransferase (AGAT), catalyzes the reaction between glycine and arginine to create ornithine and guanidinoacetic acid (GAA). The second enzyme is guanidinoacetate N-methyltransferase (GAMT), which is responsible for GAA methylation via S-adenosylmethionine (SAM) to form creatine. Once inside the cells, as shown in rats and mice, creatine is phosphorylated into phosphocreatine, initiating the conversion of ADP into ATP during its dephosphorylation. Thus, creatine functions as an energy storage molecule, which can generate ATP on demand (Bessman and Carpenter, 1985; Wyss, 2000; Da Silva et al., 2009). Additionally, unlike glycogen or fat, no metabolic breakdown is needed for ATP production from the creatine/phosphocreatine system, making it the fastest way of ATP provision. In chicken embryos and hatchlings, creatine was shown to play a major role in energy metabolism, with the potential to promote their energetic status and the post-hatch performance (Murakami et al., 2014; Zhang et al., 2016). However, little is known about the dynamics of creatine during chicken embryogenesis and its relation to glycogen stores, as well as to the energetic status of late-term embryos and hatchlings. Moreover, previous studies have not shown an inclusive comparison of glycogen and creatine dynamics simultaneously in the liver, YS tissue, and breast muscle.
This study examined creatine and glycogen dynamics from E11 until hatch and up to chick placement at the farm. Embryos and hatchlings were sampled and examined for; (1) creatine and glycogen levels in the liver, YS tissue, and breast muscle, (2) levels of blood glucose and ketones, and (3) the expression levels of genes encoding for enzymes involved in creatine synthesis in the liver, kidney, YS tissue and breast muscle (AGAT and GAMT), along with genes that are encoding for gluconeogenic enzymes (FBP1 and G6PC2), and enzymes responsible for glycogen synthase (GYS), and glycogenolysis (PYGL).
Fertile eggs (n = 100; mean weight = 70.04 g, SD = 2.19 g) from 40-week-old broiler hens (Cobb 500) were purchased from a commercial breeder farm (Y. Brown and Sons Ltd., Hod Hasharon, Israel). Eggs were incubated in a Petersime hatchery at the Faculty of Agriculture of the Hebrew University under standard conditions (37.8°C and 56% relative humidity). On embryonic day (E) 10, eggs were candled, unfertilized eggs and dead embryo eggs were removed. Tissue sampling was performed at E11, E13, E15, E17, E19, at hatch (actual time of hatch at the hatchery, 480–483 h in incubation) and at chick placement (31–34 h post hatch, prior to the exposure to feed at the farm). At each sampling day, six embryos/hatchlings were randomly selected, euthanized by cervical dislocation and the following parameters were recorded: embryo yolk free body mass (YFBM), yolk content weight, YS tissue weight (according to Yadgary et al., 2010), liver weight and breast muscle weight. The relative weights of liver, breast muscle and YS tissue were calculated as percent of YFBM. Blood glucose and ketone levels were also recorded. In addition, liver, YS tissue and breast muscle samples were collected for creatine and glycogen content determination and for the evaluation of expression levels of genes involved in creatine synthesis, gluconeogenesis, glycogenesis, and glycogenolysis. Kidney samples were collected for the evaluation of expression of creatine synthesis. Immediately after collection, tissue samples were placed in liquid nitrogen and kept under −80oc until further processing.
Liver, YS tissue and breast muscle samples were lyophilized and later examined for their creatine and glycogen content by Swiss-BioQuant-AG (Reinach, Switzerland). The concentrations of creatine and glycogen (mg/g dry weight tissue) were determined, and the total amounts (mg) in each tissue were calculated. Due to insufficient material load, breast muscle samples were analyzed only at E15, E17, E19, hatch and chick placement.
Blood glucose and ketones levels were obtained directly from the jugular vein of embryos and determined using FreeStyle Optimum Gluco/Keto meter and test strips (Abbott Diabetes Care Ltd., Witney Oxon, United Kingdom) according to the manufacturer’s instructions.
Total RNA was isolated from 100 mg of tissue (from YS tissue, liver, kidney and breast muscle) using TRI-Reagent (Sigma-Aldrich, St. Louis, MO) according to the manufacturer’s protocol. RNA concentration was determined using a Nano Drop ND-1000 instrument (Thermo Fisher Scientific, Wilmington, DE). Total RNA was treated with DNase using a Turbo DNA-free Kit according to the manufacturer’s protocol (Ambion; Thermo Fisher Scientific, Wilmington, DE). cDNA was created from 1 µg of DNA-free RNA using the qPCRBIO cDNA synthesis kit according to the manufacturer’s protocol (PCRBIOSYSTEMS, London, United Kingdom). Relative mRNA expression was evaluated using gene-specific primers (Table 1) of genes involved in creatine synthesis [arginine-glycine amidinotransferase (AGAT), guanidinoacetate N-methyltransferase (GAMT)], gluconeogenesis [fructose-1,6-bisphosphatase 1 (FBP1), glucose-6-phosphatase 2 (G6PC2)], glycogenesis [glycogen synthase (GYS)], and glycogenolysis [glycogen phosphorylase L (PYGL)] and the housekeeping genes; cytoskeletal protein (β-actin) and hypoxanthine phosphoribosyl transferase 1 (HPRT). Primer sequences were designed using Primer-BLAST software (Ye et al., 2012) based on published cDNA sequences where available and purchased from Sigma-Aldrich (Rehovot, Israel). PCR products were validated by gel electrophoresis in 1.5% agarose gel. Real-time qPCR reactions were conducted in triplicate in a Roche Light cycler 96 (Roche Molecular Systems, Inc., Pleasanton, CA). Each reaction (20 µL) included 3 µL of cDNA sample diluted 1:20 in ultrapure water (UPW, Biological Industries, Beit HaEmek, Israel), 4 µM of each primer, and Platinum SYBR Green qPCR super mix-UDG (Thermo Fisher Scientific, Wilmington, DE). Reaction conditions: preincubation at 95°C for 60°s, followed by 40 cycles of a 2-step amplification cycle of 95°C for 10°s and 60°C for 30°s, ended with a melting curve generated by the following conditions: 95°C for 60°s, 65°C for 60°s, and 97°C for 1 s. Relative mRNA expression was calculated by subtracting the geometric mean of cycle threshold (Ct) values of β-Actin and HPRT reference genes from sample Ct (Pfaffl, 2007).
Data of creatine and glycogen concentrations in tissues and data of blood glucose and ketone levels were subjected to one-way ANOVA analysis with the embryonic day (age) as main effect. Data of creatine and glycogen amount and of gene expression in tissues were subjected to two-way ANOVA analysis with the embryonic day (age), tissue and their interaction as the main effects. Differences between means were tested by using Tukey’s HSD test and considered significantly different with p-value lower than or equal to 0.05 (p ≤ 0.05). Values are presented as mean ± standard error mean (SEM). All statistical analyses were carried out using JMP-pro 16 software (SAS Institute Inc., Cary, NC).
Measurements of creatine concentration (mg/g of dry tissue) in the breast muscle showed a significant increase by 47% (p-value, p = 0.0009) from embryonic day (E) 15 to E17 (Figure 1A, and Supplementary Table S1). Then, throughout E19, hatch and chick placement, creatine levels did not differ significantly between consecutive days. The liver and yolk sac (YS) tissue exhibited similar patterns (Figures 1B, C, and Supplementary Table S1), as creatine concentration increased by 3.8-fold and 3.4-fold respectively from E13 up to the highest value at hatch (p < 0.0001). Different from the breast muscle, creatine concentration decreased in the liver and YS tissue at chick placement by 2-fold and 1.5-fold, respectively. The total amount of creatine in each organ (Figure 1D and Supplementary Table S2) was calculated by multiplying the total dry tissue weight (Suppl. Table 6) by the concentration of creatine (Figures 1A–C and Supplementary Table S1). A two-way ANOVA test revealed a significant interaction between the effects of age and tissue (p < 0.0001). This was mainly due to the breast muscle tissue, as its creatine amount showed a constant elevation pattern unlike the liver and YS tissue. Results also show that creatine is stored mainly in the breast muscle, as its amount was 3-fold higher at hatch and 15-fold higher at chick placement compared with combined amounts of the liver and YS tissue. It should be noted that compared to the liver, the YS tissue had higher amounts of creatine throughout incubation, significantly higher on E17 and at hatch (Figure 1D and Supplementary Table S2).
FIGURE 1. Creatine levels from E11 until hatch and at chick placement (A–C) Dry weight concentration (mg/g) and (D) total tissue amount (mg) of Yolk sac (YS) tissue, liver, and breast muscle (A–C) The lower-case letters denote for means significantly different between days within each organ, as derived from a one-way ANOVA Tukey’s HSD test (D) The capital letters denote for means significantly different between organs within each day as derived from a two-way ANOVA followed by Tukey’s HSD test. p ≤ 0.05 n = 6 per day.
As for glycogen, its concentration (mg/g of dry tissue) exhibited similar patterns in the breast muscle, liver, and YS tissue (Figures 2A–C, and Supplementary Table S1). Towards E17 an increase in glycogen levels was observed in all tissues, then towards hatch, glycogen levels were significantly decreased reaching the lowest levels at chick placement (p < 0.0001). The total amount of glycogen in each organ (Figure 2D and Supplementary Table S1) was also calculated by multiplying the total dry tissue weight (Supplementary Table S6) by the concentration in tissues (Figures 2A–C and Supplementary Table S1). A two-way ANOVA test revealed a significant interaction between the effects of age and tissue (p < 0.0001). The interaction was mainly due to the YS tissue, as its glycogen amount showed the most dynamic pattern, with a rapid increase up to E17 followed by a sharp decrease. In addition, throughout incubation the YS tissue had the highest amount of glycogen, as it was 27-fold higher on E11 compared to the liver and 3.3-fold higher at hatch and at chick placement, compared to the combined amount in the liver and breast muscle (Figure 2D and Supplementary Table S2).
FIGURE 2. Glycogen levels from E11 until hatch and at chick placement (A–C) Dry weight concentration (mg/g) and (D) total tissue amount (mg) of YS tissue, liver, and breast muscle (A–C) The lower-case letters denote for means significantly different between days within each organ, as derived from a one-way ANOVA Tukey’s HSD test (D) The capital letters denote for means significantly different between organs within each day as derived from a two-way ANOVA followed by Tukey’s HSD test. (p ≤ 0.05), n = 6 per day.
Glucose (mg/dL) and ketones (β-HBA; mmol/L) levels were measured from E11 to chick placement and exhibited altered patterns. From E11 to E17, glucose levels were relatively constant ranging between values of 123.5–143.6 mg/dL (Figure 3A). From E19 and until hatch and chick placement, blood glucose levels increased significantly by 38% (p < 0.0001), reaching values of 191.9–208.3 mg/dL. Ketone levels (Figure 3B) showed a dynamic pattern, starting on E11 with the lowest level (2.09 mmol), increasing gradually up to the highest value on E17 (4.33 mmol/L; p < 0.0001). On E19, ketones level decreased to 3.24 mmol/L while at hatch levels increased again to 4.06 mmol/L.
FIGURE 3. Glucose and ketones levels from E11 until hatch and at chick placement. (A)-Glucose (mg/di) and (B)-ketones ((3-HBA; mmol) were measured by FreeStyle Optimum Gluco/Keto meter. The lower-case letters denote for means significantly different between days, as derived from a one-way ANOVA Tukey’s HSD test (p < 0.05), n = 6 per day.
The potential of creatine de novo synthesis in the tissues was evaluated by the examination of arginine-glycine amidinotransferase (AGAT), and guanidinoacetate N-methyltransferase (GAMT) (Figure 4 and Supplementary Table S3). Expression analysis of AGAT and GAMT by a two-way ANOVA test revealed significant interactions between the effects of age and tissue (p < 0.0001). The interaction was mainly associated with the varying expression patterns in tissues. In the breast muscle, AGAT expression showed a dynamic pattern, as it was increased from E11 to E19, followed by a decrease at hatch, then, sharply increased again reaching the highest level at chick placement. GAMT expression in the breast muscle also showed a dynamic pattern, increasing from E11 to E19 followed by a decrease at hatch. In the kidney, expression of AGAT remained stable and was not significantly different between consecutive embryonic days, however, GAMT expression increased gradually from E13 along the second half of incubation, reaching highest values at hatch and at placement. The liver and YS tissue showed alternating expression patterns as liver AGAT expression increased towards hatch and chick placement, and YS tissue AGAT expression decreased. As for GAMT expression, in the liver significantly different values were found on E13 as levels were decreased, while at chick placement levels were significantly increased. In the YS tissue GAMT expression increased up to E17, followed by a decrease up to chick placement. Altogether, results demonstrate a shift in expression levels of AGAT and GAMT, decreasing in the extraembryonic YS tissue towards hatch and placement, and increasing in the embryonic liver, breast muscle and kidney.
FIGURE 4. Dynamic expression of genes involved in creatine synthesis (A) Arginine-glycine amidinotransferase (AGAT), catalyzes the reaction between glycine and arginine to create GAA (B) Guanidinoacetate N-methyltransferase (GAMT), responsible for the methylation of GAA to create creatine. Expression analysis of AGAT and GAMT by a two-way ANOVA followed by Tukey’s HSD test revealed significant interactions between the effects of age and tissue. (p < 0.05), n = 6 per day.
Gluconeogenesis potential was evaluated by the examination of two genes: fructose-1,6-bisphosphatase 1 (FBP1) and glucose-6-phosphatase 2 (G6PC2) (Figure 5 and Supplementary Table S4). Expression analysis of FBP1 and G6PC2 by a two-way ANOVA test revealed significant interactions between the effects of age and tissue (p < 0.0001). Also here, the interaction is associated with the altering expression patterns in tissues. Results showed daily fluctuation in liver FBP1 expression. In the YS tissue, FBP1 expression was elevated on E13 and significantly decreased on E15, then remained constant throughout incubation up to chick placement. In the breast muscle, FBP1 expression level was relatively low, decreasing between E15 and E17, and increasing again at placement. The variation in expression pattern of G6PC2 was similar to that of FBP1 expression in the liver, breast muscle and YS tissue. In general, when comparing the expression of gluconeogenic genes between the liver, YS tissue and breast muscle within days, the liver showed the highest values throughout incubation.
FIGURE 5. Dynamic expression of genes involved in gluconeogenesis (A) Fructose-1,6-bisphosphatase 1 (FBP1), regulatory enzyme, catalyzes the hydrolysis of fructose 1,6-bisphosphate to fructose 6-phosphate (B) Glucose-6- phosphatase 2 (G6PC2), catalyzes the hydrolysis of glucose-6-phosphate, allowing the release of glucose into the bloodstream. Expression analysis of FBP1 and G6PC2 by a two-way ANOVA followed by Tukey’s HSD test revealed significant interactions between the effects of age and tissue. (p < 0.05), n = 6 per day.
The potential of glycogen synthesis and breakdown was evaluated by two genes: glycogen synthase (GYS) and glycogen phosphorylase L (PYGL) (Figure 6 and Supplementary Table S5). Glycogen synthase (GYS) has two isoforms/variants: glycogen synthase 1 (GYS1) and GYS2 (Roach et al., 1998). GYS1 is expressed at lower levels in muscle, whereas GYS2 is primarily responsible for glycogen synthesis in the liver (Browner et al., 1989; Nuttall et al., 1994). Accordingly, in the breast muscle, the muscle variant GYS1 was used for expression analysis of glycogen synthase, while in the YS tissue and liver, GYS2 was used. As for, glycogen phosphorylase, the variant PYGL was used for the breast muscle, YS tissue and liver. PYGL was shown to be expressed at the protein level also in the breast muscle (Zambonelli et al., 2016). A two-way ANOVA test revealed that there was no significant interaction between the effects of age and tissue in GYS and PYGL expression. The tissue factor had statistically significant effect on GYS expression (p < 0.0001) with the highest expression levels in the liver, medium values in the YS tissue and lowest in the breast muscle. Similarly, the age factor was also found to be significant (p = 0.00035), with a peak in overall GYS expression at E19, significantly different from all sampling points apart from E17. As for PYGL, the tissue factor had a significant effect (p < 0.0001) with the highest expression levels in the liver, medium values in the YS tissue and lowest in the breast muscle. The age factor was also found to be significant (p = 0.049), with the highest PYGL expression at E17. As in the gluconeogenic genes, expression analysis of glycogen synthesis and breakdown genes between tissues within each day, showed the highest expression levels in the liver, with medium values in the YS tissue and lowest in the breast muscle.
FIGURE 6. Dynamic expression of genes involved in glycogen synthesis and breakdown. (A, B) Glycogen synthase (GYS*) catalyzes the rate-limiting step in the synthesis of glycogen; (C) Glycogen phosphorylase L (PYGL), catalyzes the release of glucose- 1 -phosphate from glycogen. As derived from a two-way ANOVA followed by Tukey’s HSD test, the factor of tissue was found to be significantly different in GYS and PYGL (highest in the liver, mean in the YS tissue and lowest in the breast muscle). The factor age was also significant, as E 1 9 was highest in GYS and E17 was highest in PYGL (p < 0.05), n = 6 per day. *Two variants were used for glycogen synthase gene; (A) GYS2 in the liver and YS tissue and (B) GYS one in the breast muscle.
The fertile egg contains trace amounts of available energy molecules in the form of carbohydrates (Yadgary and Uni, 2012; Réhault-Godbert et al., 2019) and creatine (Reicher et al., 2020). Accordingly, chicken embryos depend on the supply of high energy-value molecules that are synthesized, stored, and utilized by the embryonic and extraembryonic tissues. The current study investigated the dynamics of the two major energy-value molecules, creatine and glycogen, in key tissues for energy metabolism, from mid incubation (E11) until hatch and placement at the farm. Based on our findings, creatine is stored mainly in the breast muscle, while glycogen is stored mainly in the YS tissue. We demonstrate, for the first time, that creatine synthesis genes (AGAT, GAMT) are expressed in the chicken breast muscle as early as mid-incubation. The expression analysis of genes related to gluconeogenesis (FBP1, G6PC2), glycogenesis (GYS), and glycogenolysis (PYGL), reveals that the liver constitutes the main site of glycogen metabolism activity. However, due to the relatively large size and high storage capacity of the breast muscle and YS tissue, their contribution to glycogen metabolism is substantial. Towards hatch, creatine levels in all tissues increased, while glycogen levels decreased post E19 reaching low levels at hatch and at chick placement. Together, the findings suggest that when glycogen stores are depleted, creatine serves as an available energy source for late-term embryos and hatchlings.
In accordance with the traditional description of spatial separation between the supply and demand organs of endogenous creatine (Wyss, 2000), our results show that in chicken embryos creatine synthesis genes (AGAT, GAMT) are expressed in the liver and kidney. Here we show, that AGAT and GAMT are also expressed in the extraembryonic YS tissue. This fits the concept of the YS tissue as a multifunctional metabolic organ, which supports embryonic development (Yadgary et al., 2010; Yadgary et al., 2011; Yadgary and Uni, 2012; Yadgary et al., 2013; Yadgary et al., 2014; Dayan et al., 2020; Wong and Uni, 2021). To the best of our knowledge, we are the first to show AGAT and GAMT expression in the breast muscle of chicken embryos; the expression of these genes was previously found in the skeletal muscle of fish, mice, cattle, and pigs (Borchel et al., 2019). Ostojic (2021) points towards a metabolic advantage of de novo creatine synthesis in myocytes to maintain muscle creatine levels and cellular energy. The levels of creatine in the liver, YS tissue and breast muscle show similar pattern of increase up to hatch, where creatine is stored mainly in the breast muscle. Nevertheless, considering the relative size of organs, the significance of the YS tissue, the largest organ during incubation is highlighted as a superior creatine source vs the liver, with higher total creatine amount (19-fold higher on E17 and 7-fold higher at hatch). Together, we conclude that during incubation the supply of creatine depends on its de novo synthesis by the embryonic and extraembryonic tissues. As creatine levels increase towards hatch, it serves as an essential energy source, especially to late-term embryos and hatchlings.
Comparable patterns of glycogen levels were exhibited in the breast muscle, liver and YS tissue where all tissues reached peak values on E17-E19, followed by a sharp decrease towards chick placement. Previous studies showed a similar pattern corresponding with the changes in energy metabolism towards hatch and decreased glycogen levels in tissues (Christensen et al., 1999; Christensen et al., 2000; Christensen et al., 2001; Uni and Ferket, 2004; Uni et al., 2005; Foye et al., 2006; Moran 2007; De Oliveira et al., 2008; Yadgary and Uni, 2012). This study shows that throughout incubation, the majority of glycogen is stored in the YS tissue. This agrees with a previously published study by Yadgary and Uni (2012), which showed the importance of the YS tissue as the major glycogen storage organ. Our results suggest a pivotal role of the YS tissue, not only in creatine supply, but also in the supply of glycogen during mid-term incubation up to the initiation of the energy-demanding hatching process. Expression analysis of genes involved in glycogen synthesis and breakdown (GYS and PYGL, respectively), showed the highest expression levels in the liver compared with mid values in the YS tissue and the lowest values in the breast muscle. According to these findings, E19 marks a critical time point, showing a shift in balance between glycogen synthesis and breakdown. The decrease in GYS expression but not in PYGL expression fits the observed decrease in glycogen levels. On this day (E19), as oxygen becomes limited (Tazawa et al., 1983), other metabolic pathways are involved in glucose and ATP production, shifting towards anaerobic catabolism of glucose, with the breakdown of glycogen and gluconeogenesis (Uni and Ferket, 2004; Moran, 2007; De Oliveira et al., 2008; Yadgary and Uni 2012). Indeed, the increase in ketones levels up to E17 correspond with β-oxidation of yolk-derived fatty acids, and the rise in blood glucose levels (starting on E19) correspond with the metabolic shift and the decrease in tissue glycogen levels from E17 onwards. At hatch, when oxygen supply was restored, ketones levels increase again. The expression of the two key genes FBP1 and G6PC2 was the highest in the liver along the incubation period with mid values in the YS tissue and lowest in the breast muscle. Together, our results lead to the conclusion that during the second half of incubation the gluconeogenic pathway as well as glycogen synthesis and breakdown is most active in the liver. However, due to the relatively large size and high storage capacity of the breast muscle and YS tissue, their contribution to energy supply for the chicken embryo should not be neglected.
In conclusion, this study evaluated the dynamics of creatine, glycogen, and their synthesis during the second half of the broiler embryonic development period. We demonstrate that during incubation, creatine is stored mainly in the breast muscle while glycogen is stored mainly in the YS tissue. The exhibited decrease in glycogen levels towards hatch in all tissues, starting on E19, opposes the increase in creatine levels, suggesting the significance of creatine in energy supply for late-term embryos and hatchlings (summarized in Figure 7). Moreover, in breast muscle, as glycogen levels are depleted, high creatine levels are maintained until chick placement, emphasizing the utmost importance of creatine involvement in supplying available energy for the hatching chick before placement at the farm. The fact that creatine is involved in maintaining available energy supply for the hatching broiler chick could have a great impact when considering hatchling quality, uniformity, chick survival and post-hatch performance.
FIGURE 7. Graphical illustration demonstrating the available energy resources (creatine and glycogen) in the chicken embryo during the pre-post hatch period. Towards hatch, creatine levels in the YS tissue, liver and breast muscle increase while glycogen levels dramatically decrease, reaching low levels at hatch and at chick placement. In the breast muscle, as glycogen levels are depleted, creatine levels are elevated. Thus, highlighting the importance of creatine as an available energy source for the late-term embryo and hatchling.
The original contributions presented in the study are included in the article/Supplementary Materials, further inquiries can be directed to the corresponding author.
The animal study was reviewed and approved by IACUC: AG-20-16298.
JD: experimental design and execution, data analysis, manuscript writing. TM-Z: experimental methodologies, data analysis, manuscript review. NR: experimental methodologies. OH and ZU: supervision, manuscript review. SM: experimental methodologies, manuscript review. UB and VI: funding. All authors contributed to the article and approved the submitted version.
This study received partial funding from AlzChem Trostberg GmbH. The funder was not involved in the study design, analysis, interpretation of data, the writing of this article or the decision to submit it for publication.
UB and VI were employed by AlzChem Trostberg GmbH.
The remaining authors declare that the research was conducted in the absence of any commercial or financial relationships that could be construed as a potential conflict of interest.
All claims expressed in this article are solely those of the authors and do not necessarily represent those of their affiliated organizations, or those of the publisher, the editors and the reviewers. Any product that may be evaluated in this article, or claim that may be made by its manufacturer, is not guaranteed or endorsed by the publisher.
The Supplementary Material for this article can be found online at: https://www.frontiersin.org/articles/10.3389/fphys.2023.1079638/full#supplementary-material.
Bessman S. P., Carpenter C. L. (1985). The creatine-creatine phosphate energy shuttle. Annu. Rev. Biochem. 54 (1), 831–862. doi:10.1146/annurev.bi.54.070185.004151
Blaxter K. (1989). Energy metabolism in animals and man. Cambridge, United Kingdom: Cambridge University Press.
Boo S. Y., Tan S. W., Alitheen N. B., Ho C. L., Omar A. R., Yeap S. K. (2020). Identification of reference genes in chicken intraepithelial lymphocyte natural killer cells infected with very-virulent infectious bursal disease virus. Sci. Rep. 10 (1), 8561–8569. doi:10.1038/s41598-020-65474-3
Borchel A., Verleih M., Kühn C., Rebl A., Goldammer T. (2019). Evolutionary expression differences of creatine synthesis-related genes: Implications for skeletal muscle metabolism in fish. Sci. Rep. 9 (1), 5429–5438. doi:10.1038/s41598-019-41907-6
Browner M. F., Nakano K., Bang A. G., Fletterick R. J. (1989). Human muscle glycogen synthase cDNA sequence: A negatively charged protein with an asymmetric charge distribution. Proc. Natl. Acad. Sci. 86 (5), 1443–1447. doi:10.1073/pnas.86.5.1443
Christensen V. L., Donaldson W. E., Nestor K. E., McMurtry J. P. (1999). Effects of genetics and maternal dietary iodide supplementation on glycogen content of organs within embryonic turkeys. Poult. Sci. 78 (6), 890–898. doi:10.1093/ps/78.6.890
Christensen V. L., Grimes J. L., Donaldson W. E., Lerner S. (2000). Correlation of body weight with hatchling blood glucose concentration and its relationship to embryonic survival. Poult. Sci. 79 (12), 1817–1822. doi:10.1093/ps/79.12.1817
Christensen V. L., Wineland M. J., Fasenko G. M., Donaldson W. E. (2001). Egg storage effects on plasma glucose and supply and demand tissue glycogen concentrations of broiler embryos. Poult. Sci. 80 (12), 1729–1735. doi:10.1093/ps/80.12.1729
Cogburn L. A., Trakooljul N., Chen C., Huang H., Wu C. H., Carré W., et al. (2018). Transcriptional profiling of liver during the critical embryo-to-hatchling transition period in the chicken (Gallus gallus). BMC genomics 19 (1), 695. doi:10.1186/s12864-018-5080-4
Collin A., Berri C., Tesseraud S., Rodon F. R., Skiba-Cassy S., Crochet S., et al. (2007). Effects of thermal manipulation during early and late embryogenesis on thermotolerance and breast muscle characteristics in broiler chickens. Poult. Sci. 86 (5), 795–800. doi:10.1093/ps/86.5.795
Da Silva R. P., Nissim I., Brosnan M. E., Brosnan J. T. (2009). Creatine synthesis: Hepatic metabolism of guanidinoacetate and creatine in the rat in vitro and in vivo. Am. J. Physiology-Endocrinology Metabolism 296 (2), E256–E261. doi:10.1152/ajpendo.90547.2008
Dayan J., Reicher N., Melkman-Zehavi T., Uni Z. (2020). Incubation temperature affects yolk utilization through changes in expression of yolk sac tissue functional genes. Poult. Sci. 99 (11), 6128–6138. doi:10.1016/j.psj.2020.07.037
De Oliveira J. E., Uni Z., Ferket P. R. (2008). Important metabolic pathways in poultry embryos prior to hatch. World's Poult. Sci. J. 64 (4), 488–499. doi:10.1017/s0043933908000160
Decuypere E., Tona K., Bruggeman V., Bamelis F. (2001). The day-old chick: A crucial hinge between breeders and broilers. World's Poult. Sci. J. 57 (2), 127–138. doi:10.1079/wps20010010
Foye O. T., Uni Z., Ferket P. R. (2006). Effect of in ovo feeding egg white protein, β-hydroxy-β methylbutyrate, and carbohydrates on glycogen status and neonatal growth of turkeys. Poult. Sci. 85 (7), 1185–1192. doi:10.1093/ps/85.7.1185
Givisiez P. E., Moreira Filho A. L., Santos M. R., Oliveira H. B., Ferket P. R., Oliveira C. J., et al. (2020). Chicken embryo development: Metabolic and morphological basis for in ovo feeding technology. Poult. Sci. 99 (12), 6774–6782. doi:10.1016/j.psj.2020.09.074
Havenstein G. B., Ferket P. R., Qureshi M. A. (2003a). Carcass composition and yield of 1957 versus 2001 broilers when fed representative 1957 and 2001 broiler diets. Poult. Sci. 82 (10), 1509–1518. doi:10.1093/ps/82.10.1509
Havenstein G. B., Ferket P. R., Qureshi M. A. (2003b). Growth, livability, and feed conversion of 1957 versus 2001 broilers when fed representative 1957 and 2001 broiler diets. Poult. Sci. 82 (10), 1500–1508. doi:10.1093/ps/82.10.1500
Leksrisompong N., Romero-Sanchez H., Plumstead P. W., Brannan K. E., Brake J. (2007). Broiler incubation. 1. Effect of elevated temperature during late incubation on body weight and organs of chicks. Poult. Sci. 86 (12), 2685–2691. doi:10.3382/ps.2007-00170
Moran E. T. (2007). Nutrition of the developing embryo and hatchling. Poult. Sci. 86 (5), 1043–1049. doi:10.1093/ps/86.5.1043
Murakami A. E., Rodrigueiro R. J. B., Santos T. C., Ospina-Rojas I. C., Rademacher M. (2014). Effects of dietary supplementation of meat-type quail breeders with guanidinoacetic acid on their reproductive parameters and progeny performance. Poult. Sci. 93 (9), 2237–2244. doi:10.3382/ps.2014-03894
Noble R. C., Cocchi M. (1990). Lipid metabolism and the neonatal chicken. Prog. lipid Res. 29 (2), 107–140. doi:10.1016/0163-7827(90)90014-c
Nuttall F. Q., Gannon M. C., Bai G., Lee E. Y. (1994). Primary structure of human liver glycogen synthase deduced by cDNA cloning. Archives Biochem. biophysics 311 (2), 443–449. doi:10.1006/abbi.1994.1260
Ostojic S. M. (2021). Creatine synthesis in the skeletal muscle: The times they are a-changin’. Am. J. Physiology-Endocrinology Metabolism 320 (2), E390–E391. doi:10.1152/ajpendo.00645.2020
Pfaffl M. W. (2007). “Relative quantification,” in Real-time PCR (Abingdon, UK: Taylor & Francis), 89–108.
Réhault-Godbert S., Guyot N., Nys Y. (2019). The golden egg: Nutritional value, bioactivities, and emerging benefits for human health. Nutrients, 11(3), 684, doi:10.3390/nu11030684
Reicher N., Epstein T., Gravitz D., Cahaner A., Rademacher M., Braun U., et al. (2020). From broiler breeder hen feed to the egg and embryo: The molecular effects of guanidinoacetate supplementation on creatine transport and synthesis. Poult. Sci. 99 (7), 3574–3582. doi:10.1016/j.psj.2020.03.052
Roach P. J., Cheng C., Huang D., Lin A., Mu J., Skurat A. V., et al. (1998). Novel aspects of the regulation of glycogen storage. J. basic Clin. physiology Pharmacol. 9 (2-4), 139–151. doi:10.1515/jbcpp.1998.9.2-4.139
Romanoff A. L. (1960). “The extraembryonic membranes,” in The avian embryo: Structural and functional development (New York, NY: The Macmillan Company), 1041–1140.
Scanes C. G. (2022). “Carbohydrate metabolism,” in Sturkie's avian physiology (Massachusetts, United States: Academic Press), 593–625.
Speake B. K., Murray A. M., Noble R. C. (1998). Transport and transformations of yolk lipids during development of the avian embryo. Prog. Lipid Res. 37, 1–32. doi:10.1016/s0163-7827(97)00012-x
Tazawa H., Visschedijk A. H. J., Wittmann J., Piiper J. (1983). Gas exchange, blood gases and acid-base status in the chick before, during and after hatching. Respir. Physiol. 53 (2), 173–185. doi:10.1016/0034-5687(83)90065-8
Uni Z., Ferket P. R., Tako E., Kedar O. (2005). In ovo feeding improves energy status of late-term chicken embryos. Poult. Sci. 84 (5), 764–770. doi:10.1093/ps/84.5.764
Uni Z., Ferket R. P. (2004). Methods for early nutrition and their potential. World's Poult. Sci. J. 60 (1), 101–111. doi:10.1079/WPS20038
Walker J. B. (1979). Creatine: Biosynthesis, regulation, and function. Adv. Enzym. Relat. Areas Mol. Biol., 50(177), 177–242. doi:10.1002/9780470122952.ch4
Wong E. A., Uni Z. (2021). Centennial Review: The chicken yolk sac is a multifunctional organ. Poult. Sci., 100(3), 100821. doi:10.1016/j.psj.2020.11.004
Wyss M. (2000). Creatine and creatinine metabolism. Physiol. Rev. 80, 1107–1213. doi:10.1152/physrev.2000.80.3.1107
Yadgary L., Cahaner A., Kedar O., Uni Z. (2010). Yolk sac nutrient composition and fat uptake in late-term embryos in eggs from young and old broiler breeder hens. Poult. Sci. 89 (11), 2441–2452. doi:10.3382/ps.2010-00681
Yadgary L., Kedar O., Adepeju O., Uni Z. (2013). Changes in yolk sac membrane absorptive area and fat digestion during chick embryonic development. Poult. Sci. 92 (6), 1634–1640. doi:10.3382/ps.2012-02886
Yadgary L., Uni Z. (2012). Yolk sac carbohydrate levels and gene expression of key gluconeogenic and glycogenic enzymes during chick embryonic development. Poult. Sci. 91 (2), 444–453. doi:10.3382/ps.2011-01669
Yadgary L., Wong E. A., Uni Z. (2014). Temporal transcriptome analysis of the chicken embryo yolk sac. BMC genomics 15 (1), 690–715. doi:10.1186/1471-2164-15-690
Yadgary L., Yair R., Uni Z. (2011). The chick embryo yolk sac membrane expresses nutrient transporter and digestive enzyme genes. Poult. Sci. 90 (2), 410–416. doi:10.3382/ps.2010-01075
Ye J., Coulouris G., Zaretskaya I., Cutcutache I., Rozen S., Madden T. L. (2012). Primer BLAST: A tool to design target-specific primers for polymerase chain reaction. BMC Bioinforma. 13 (1), 134–211. doi:10.1186/1471-2105-13-134
Zambonelli P., Zappaterra M., Soglia F., Petracci M., Sirri F., Cavani C., et al. (2016). Detection of differentially expressed genes in broiler pectoralis major muscle affected by White Striping–Wooden Breast myopathies. Poult. Sci. 95 (12), 2771–2785. doi:10.3382/ps/pew268
Zhang L., Zhu X. D., Wang X. F., Li J. L., Gao F., Zhou G. H. (2016). Individual and combined effects of in-ovo injection of creatine monohydrate and glucose on somatic characteristics, energy status, and post hatch performance of broiler embryos and hatchlings. Poult. Sci. 95 (10), 2352–2359. doi:10.3382/ps/pew130
Keywords: incubation, chicken, embryo, creatine, glycogen
Citation: Dayan J, Melkman-Zehavi T, Reicher N, Braun U, Inhuber V, Mabjeesh SJ, Halevy O and Uni Z (2023) Supply and demand of creatine and glycogen in broiler chicken embryos. Front. Physiol. 14:1079638. doi: 10.3389/fphys.2023.1079638
Received: 25 October 2022; Accepted: 11 January 2023;
Published: 24 January 2023.
Edited by:
Xiaofei Wang, Tennessee State University, United StatesReviewed by:
Monika Proszkowiec-Weglarz, Agricultural Research Service (USDA), United StatesCopyright © 2023 Dayan, Melkman-Zehavi, Reicher, Braun, Inhuber, Mabjeesh, Halevy and Uni. This is an open-access article distributed under the terms of the Creative Commons Attribution License (CC BY). The use, distribution or reproduction in other forums is permitted, provided the original author(s) and the copyright owner(s) are credited and that the original publication in this journal is cited, in accordance with accepted academic practice. No use, distribution or reproduction is permitted which does not comply with these terms.
*Correspondence: Zehava Uni, emVoYXZhLnVuaUBtYWlsLmh1amkuYWMuaWw=
Disclaimer: All claims expressed in this article are solely those of the authors and do not necessarily represent those of their affiliated organizations, or those of the publisher, the editors and the reviewers. Any product that may be evaluated in this article or claim that may be made by its manufacturer is not guaranteed or endorsed by the publisher.
Research integrity at Frontiers
Learn more about the work of our research integrity team to safeguard the quality of each article we publish.