- 1The Hospital for Sick Children, Division of Neonatology, Department of Pediatrics and Translational Medicine Program, Toronto, ON, Canada
- 2Department of Laboratory Medicine and Pathobiology, University of Toronto, Toronto, ON, Canada
Extremely low gestational age neonates (ELGANs) are born in a relatively hyperoxic environment with weak antioxidant defenses, placing them at high risk for mitochondrial dysfunction affecting multiple organ systems including the nervous, respiratory, ocular, and gastrointestinal systems. The brain and lungs are highly affected by mitochondrial dysfunction and dysregulation in the neonate, causing white matter injury (WMI) and bronchopulmonary dysplasia (BPD), respectively. Adequate mitochondrial function is important in providing sufficient energy for organ development as it relates to alveolarization and axonal myelination and decreasing oxidative stress via reactive oxygen species (ROS) and reactive nitrogen species (RNS) detoxification. Peroxisome proliferator-activated receptor gamma coactivator-1 alpha (PGC-1α) is a master regulator of mitochondrial biogenesis and function. Since mitochondrial dysfunction is at the root of WMI and BPD pathobiology, exploring therapies that can regulate PGC-1α activity may be beneficial. This review article describes several promising therapeutic agents that can mitigate mitochondrial dysfunction through direct and indirect activation and upregulation of the PGC-1α pathway. Metformin, resveratrol, omega 3 fatty acids, montelukast, L-citrulline, and adiponectin are promising candidates that require further pre-clinical and clinical studies to understand their efficacy in decreasing the burden of disease from WMI and BPD in preterm infants.
1 Introduction
Extremely low gestational age neonates (ELGANs) born less than 28 weeks of gestation are at increased risk for a myriad of comorbidities including white matter injury (WMI) in the brain, bronchopulmonary dysplasia (BPD), necrotizing enterocolitis (NEC), and retinopathy of prematurity (Kelly, 2006; Thébaud et al., 2019). Apnea of prematurity is also common in these infants wherein periods of intermittent hypoxia are frequent (Kelly, 2006). Intermittent hypoxia increases reactive oxygen species (ROS) and reactive nitrogen species (RNS), leading to oxidative stress and mitochondrial dysfunction with adverse consequences to developing organs. In this review, we discuss the downstream impacts of oxidative stress and mitochondrial dysfunction leading to WMI and BPD, two major comorbidities in ELGANs. Moreover, we discuss the importance of peroxisome proliferator-activated receptor gamma coactivator-1 alpha (PGC-1α), master regulator of mitochondrial biogenesis and function, as it relates to the pathogenesis of WMI and BPD, and potential therapies that indirectly regulate PGC-1α activity to reduce the burden of disease in ELGANs.
2 Mitochondrial dysfunction in preterm infants and its downstream effects
Substantial cellular energy is required throughout gestation for organ development and growth. ELGANs have especially high energy needs for adequate postnatal organ development since they are born with structurally and functionally immature organs (Ten, 2017; Harvey, 2019; Rodríguez-Cano et al., 2020). Mitochondria are responsible for cellular energy generation in the form of adenosine triphosphate (ATP) by oxidative phosphorylation through the electron transport chain (ETC). The ETC is composed of five multi-subunit enzyme complexes (complex I-V) and two electron carriers (Coenzyme Q and cytochrome c) located in the inner mitochondrial membrane (Guo et al., 2013). The transfer of electrons through the ETC is coupled with the transport of protons across the inner membrane, establishing the electrochemical gradient which allows for the protonmotive force and ATP production (Guo et al., 2013). During this process, mitochondria continuously function to metabolize oxygen and generate ROS and RNS. Mitochondria are equipped with defense systems to detoxify ROS and RNS and protect the cell against oxidative damage. Some antioxidant enzymes are manganese superoxide dismutase (SOD2), catalase, thioredoxin reductase 2, peroxiredoxin (PRX)-5, PRX-3, uncoupling protein (UCP)-2, and thioredoxin (TRX)-2 (MatÉs et al., 1999; Mailloux, 2018; Napolitano et al., 2021).
Under normal physiological conditions, ROS and RNS levels are balanced by the enzymatic and non-enzymatic antioxidant detoxification systems (Douglas et al., 2010; Zhang et al., 2016). However, under pathologic conditions, ROS and RNS production exceeds detoxification, leading to oxidative and nitrosative stress (Mittal et al., 2014; Zhang et al., 2016; Cannavò et al., 2021). Elevated ROS and RNS levels cause injury via lipid peroxidation, cell death, and release of damage-associated molecular patterns (DAMPs), initiating an inflammatory response (Circu and Aw, 2010; Nakahira et al., 2015; Su et al., 2019; Falsaperla et al., 2020). ROS overproduction can cause pathophysiological changes in the mitochondria, known as mitochondrial dysfunction. It involves mitochondrial DNA mutations, ETC dysregulation, increased membrane permeability, and impaired antioxidant modulatory systems (Guo et al., 2013) (Figure 1). Damage to mitochondria can also lead to the release of cytotoxic mitochondrial DAMPs that can initiate innate and adaptive immune responses (Nakahira et al., 2015). Mitochondria have various mechanisms such as mitochondrial fusion and fission, biogenesis, and mitophagy to maintain homeostasis and prevent ROS-induced oxidative damage. Mitophagy is an autophagic response by which dysfunctional mitochondria are targeted for lysosomal degradation, preventing further ROS production and cytotoxicity (Wu et al., 2019). The accumulation of damaged mitochondria and a decrease in mitophagy are hallmarks of organ failure (Zhulong et al., 2021). Lastly, mitochondria continuously undergo fission and fusion to allow for the exchange or segregation of material between mitochondria to repair damaged components and to ensure energy needs are met (Thornton et al., 2018; Jones and Thornton, 2022).
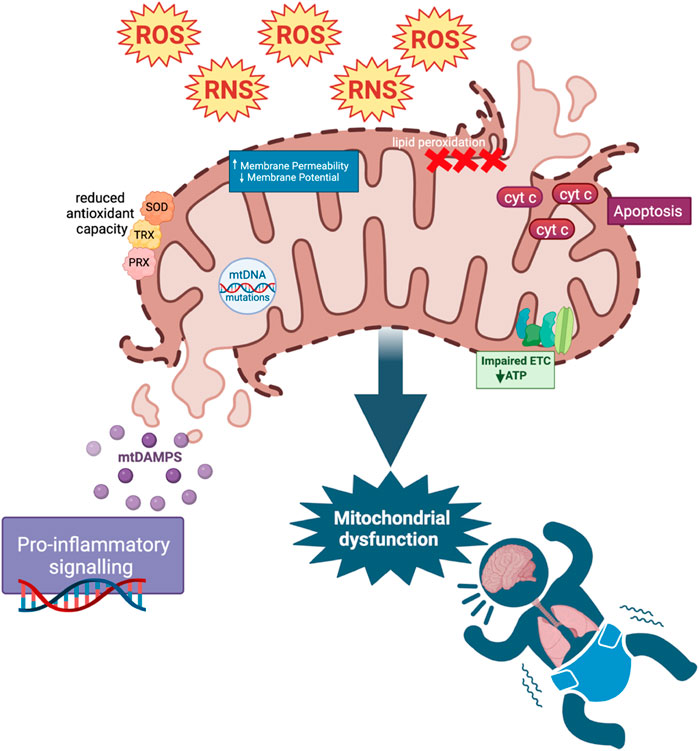
FIGURE 1. ROS and Mitochondrial dysfunction. Increased ROS and RNS cause mitochondrial dysfunction through mitochondrial DNA (mtDNA) mutations, defects in the electron transport chain (ETC) and antioxidant modulatory proteins, and lipid peroxidation in cell and mitochondrial membranes (affecting mitochondrial membrane potential). These changes in mitochondrial permeability could lead to the release of cytochrome C (cyt c), mediating apoptosis as well as mitochondrial DAMP (mtDAMP) release. MtDAMPs and increased ROS cause downstream pro-inflammatory signaling. Reduced antioxidant capacity resulting from mitochondrial dysfunction further adds to the pool of ROS in a positive feedback loop.
At birth, preterm infants with decreased antioxidant capacities are exposed to a high oxidative load, creating substantial risk for cellular and tissue injury because the intrauterine environment is hypoxic relative to the extrauterine environment (Mittal et al., 2014; Ozsurekci and Aykac, 2016; Falsaperla et al., 2020; Cannavò et al., 2021). In this review, we discuss the role of mitochondrial dysfunction in the pathobiology of WMI and BPD.
2.1 Mitochondrial dysfunction in the brain
Preterm infants have structurally and functionally immature brains because major components of brain development including oligodendrocyte maturation, myelination, and synapse formation occur in the latter stages of gestation (Jakovcevski et al., 2009; Stiles and Jernigan, 2010; Moura et al., 2022). Adequate brain development requires increased total mitochondrial capacity and higher ATP levels to meet the significantly increased rate of energy consumption (Erecinska et al., 2004; Ten, 2017). Mitochondrial dysfunction is operative in injury to the developing oligodendrocyte, cells responsible for myelination, leading to WMI (Bizzozero et al., 1999; Morató et al., 2014). WMI is characterized as abnormal axonal myelination in the brain (Ten, 2017). Oligodendrocytes are highly sensitive to changes in mitochondrial function. Inhibition of mitochondrial complexes I and IV results in arrested oligodendrocyte differentiation and degeneration of mature and precursor cells, respectively. Impaired mitochondrial ETC activity, particularly at complexes I and III, also leads to increased ROS production, and subsequent neuronal cell death (Douglas et al., 2010). ROS levels are further increased as a result of impaired antioxidant defenses by enzymes such as SOD and glutathione peroxidase which are low in infants born 28 weeks of gestation (Flint Beal, 1995; Neubauer, 2001; Prabhakar et al., 2006; Shan et al., 2007; Lee and Davis, 2011; Lembo et al., 2021). When brain tissue is injured, it resorbs. Depending on the stage of brain development when the injury occurs, these infants can have poor brain growth leading to microcephaly or hydrocephalus ex vacuo (enlargement of the ventricles due to resorbed brain tissue). Periventricular leukomalacia (PVL) which involves the death of brain tissue around the ventricles exists in two forms, non-cystic and cystic PVL. Non-cystic PVL affects ∼50% of ELGANs whereas, the more severe form, cystic PVL, affects ∼5% of these infants (Khwaja and Volpe, 2008; Ten, 2017). Abnormal axonal myelination from arrested oligodendrocyte maturation is associated with poor motor outcomes, while injury to migrating neurons from the subventricular region to the cortex is associated with cognitive, behavioral, attentional, and sensorimotor deficits (Lee, 2017; Ten, 2017).
Breathing instability is a hallmark of the preterm infant due to the immature central respiratory network along with the strong influence of peripheral arterial chemoreceptors on baseline breathing. The combination of low functional residual capacity, ventilation-perfusion mismatch, and hypoxic pulmonary vasoconstriction, increases the risk of intermittent hypoxic episodes which are common and significant in ELGANs. Notably, both hypoxia and hyperoxia lead to ROS production (Chandel et al., 2000; Guzy and Schumacker, 2006), and repetitive episodes of intermittent hypoxia in preterm infants are associated with increased markers of oxidative stress, ROS generation, and mitochondrial dysfunction (Schulz et al., 2000; Lavie, 2003; Douglas et al., 2010). Early postnatal intermittent hypoxia in newborn rats causes WMI, hypomyelination, apoptosis, and an increase in pro-inflammatory markers in the brain (Gozal et al., 2001a; Gozal et al., 2001b; Xu et al., 2004; Douglas et al., 2010; Cai et al., 2012; Juliano et al., 2015; Darnall et al., 2017; Ten, 2017). Intermittent hypoxia in adult rodents also causes apoptosis, mitochondrial dysfunction, oxidative stress, and ROS generation in the brain (Gozal et al., 2001a; Gozal et al., 2001b; Row et al., 2003; Xu et al., 2004). Transgenic mice overexpressing the antioxidant enzyme extracellular SOD (SOD3) have lower levels of ROS and reduced neuronal apoptosis in response to hypoxia suggesting that upregulation of SOD in preterm infants at risk for WMI may be protective (Zaghloul et al., 2014).
Systemic inflammation is another factor that markedly increases the risk of developing neuroinflammation and subsequent WMI in ELGANs (Volpe, 2008; Lee, 2017). For instance, preterm infants with inflammatory diseases such as BPD and NEC are at increased risk of developing WMI than infants without BPD or NEC (Shah et al., 2008; Volpe, 2008; Gagliardi et al., 2009; Biouss et al., 2019; Agut et al., 2020; Grelli et al., 2021). Circulating cytokines, chemokines, and DAMPs originating from inflamed tissue can damage the central nervous system through the bloodstream and a disrupted blood-brain barrier. Increased blood-brain barrier permeability directly disrupts brain homeostasis (Sankowski et al., 2015; Kempuraj et al., 2017; Varatharaj and Galea, 2017; Galea, 2021). The influx of inflammatory mediators can trigger neuroinflammation and activate microglia; microglia are specialized macrophages in the central nervous system. Activated microglia produce increased levels of ROS and RNS that can damage developing neurons and cells that lead to WMI in preterm infants (Kadhim et al., 2006; Deng et al., 2008; Niizuma et al., 2010; Chen et al., 2011; Franklin, 2011; Baburamani et al., 2014; Back, 2017; Kempuraj et al., 2017; Lee et al., 2019; McNamara and Miron, 2020).
2.2 Mitochondrial dysfunction in the lungs
BPD is a chronic lung disease initiated by inflammation and oxidative stress during the canalicular and saccular stages of lung development, disrupting alveolar and vascular growth (Thébaud et al., 2019). Mitochondrial dysfunction, impaired oxidative phosphorylation, and their downstream effects are all operative in BPD pathogenesis (Ratner et al., 2009; Yue and Yao, 2016; Kandasamy et al., 2017; Shah et al., 2018; Xuefei et al., 2021; Yue et al., 2021). Mitochondrial density continues to increase during the postnatal period to support normal lung development and growth but mitochondrial density and function are disrupted in infants with BPD (El-Merhie et al., 2017). Preterm infants with BPD have increased ROS production and mitochondrial dysfunction which lead to impaired tissue development (Berkelhamer et al., 2013a; Collins et al., 2017; Ten, 2017; Valencia et al., 2018; Wang and Dong, 2018). Hyperoxia-induced mitochondrial DNA damage negatively impacts branching morphogenesis and lung development (Ballinger et al., 1999; Gebb et al., 2013). Mitochondrial dysfunction and inhibition of Complex I in neonatal mice results in disrupted alveolarization, a key feature of BPD, suggesting an important role for mitochondrial regulation and function in lung development (Bizzozero et al., 1999; Ratner et al., 2009; Vohwinkel et al., 2011; Das, 2013; Ratner et al., 2013). Mitochondrial dysfunction also occurs in adult lung diseases such as pulmonary fibrosis and chronic obstructive pulmonary disorder (Ahmad et al., 2015; Yue and Yao, 2016; Rangarajan et al., 2017; Cloonan et al., 2020; Larson-Casey et al., 2020; Siekacz et al., 2021).
The clinical definition of BPD is a need for supplemental oxygen at 36 weeks corrected gestational age in a formerly preterm infant (Jensen et al., 2019; Sahni and Mowes, 2022). Although the concentration of inhaled supplemental oxygen may not lead to toxic levels of oxygen in the blood, it is directly toxic to the bronchial and alveolar epithelial cells that line the airway and internal lung surface area. Oxygen toxicity in alveolar epithelial cells is characterized by abnormal mitochondrial morphology, impaired mitochondrial biogenesis, increased mitochondrial ROS production, and mitochondrial DNA damage (O’Donovan and Fernandes, 2000; Ahmad et al., 2001; Dobson et al., 2002; Roper et al., 2004; Ruchko et al., 2005; Brueckl et al., 2006; Chan, 2006; Ratner et al., 2009; Afolayan et al., 2012; Das, 2013; Yue and Yao, 2016; Kandasamy et al., 2017; Shah et al., 2018). Alveolar Type 1 epithelial cells cover 95–98% of the internal lung surface area and are vulnerable to oxygen toxicity even at low levels of inhaled oxygen (Harris et al., 1991; McElroy and Kasper, 2004). Mitochondrial density varies with cell type and function. For instance, alveolar epithelial Type 2 cells have a high number of mitochondria to support surfactant production (Massaro et al., 1975). Notably, hyperoxia-associated mitochondrial dysfunction is not rapidly reversible; mitochondria dysregulation persists in alveolar epithelial cells exposed to hyperoxia even after recovery in normoxic conditions (Garcia et al., 2021). Alveolar epithelial cells, specifically Type 1 cells, are in close proximity to endothelial cells as they play an important role in facilitating gas exchange. Human umbilical vein endothelial cells (HUVECs) are often used to study endothelial function. HUVECs from preterm infants who later developed BPD or died had increased indicators of mitochondrial dysfunction when the cells harvested at birth were exposed to hyperoxia in vitro (Kandasamy et al., 2017). Specifically, these cells produced more mitochondrial ROS, had a lower capacity for oxidative phosphorylation, decreased oxygen consumption, and more mitochondrial DNA damage (Kandasamy et al., 2017). These effects were not seen in HUVECs harvested from infants without BPD. Another characteristic feature of BPD is chronic hypercapnia; elevated carbon dioxide levels cause mitochondrial dysfunction as well as inhibition of oxidative phosphorylation, which decreases cell proliferation in alveolar epithelial cells and lung fibroblasts (Vohwinkel et al., 2011; Thébaud et al., 2019).
Pulmonary hypertension (PH) is a common comorbidity in preterm infants with BPD, occurring in approximately 25% of infants with moderate-severe BPD (Berkelhamer et al., 2013b; Mourani et al., 2015; Al-Ghanem et al., 2017; Weismann et al., 2017; Hansmann et al., 2021) with mortality rates of 14–38% in infants with BPD-PH (Khemani et al., 2007; An et al., 2010; Ambalavanan and Mourani, 2014; Al-Ghanem et al., 2017; Arjaans et al., 2021). PH is characterized by pulmonary artery remodeling and increased pulmonary vascular resistance, leading to right ventricular dysfunction (Berkelhamer et al., 2013b; Hansmann et al., 2021). Another feature of PH pathophysiology is pulmonary artery smooth muscle cell (PASMC) and endothelial cell hyperproliferation and resistance to apoptosis, causing pulmonary artery wall thickening and lumen narrowing (Marshall et al., 2018; Humbert et al., 2019; Nesterova et al., 2020). Mitochondrial dysfunction and dysregulation are also operative in PH pathogenesis (Trenker et al., 2007; Angara and Bhandari, 2013; Dromparis et al., 2013; Pak et al., 2013; Adesina et al., 2015; Bruns et al., 2015; Diebold et al., 2015; Houten, 2015; Chan and Rubin, 2017; Hong et al., 2017; Culley and Chan, 2018; Marshall et al., 2018; Rafikova et al., 2018; Suliman and Nozik-Grayck, 2019). Neonatal mice who developed hyperoxia-mediated BPD-PH with right ventricular hypertrophy had reduced expression of antioxidant enzymes and increased expression of ROS-producing enzymes in lung homogenates, compared to normoxic controls (Datta et al., 2015). The mechanisms by which hyperoxia, hypoxia, and inflammation contribute to an imbalance in ROS production and detoxification, oxidative stress, mitochondrial dysfunction, and characteristic PH pathophysiology in PASMCs and endothelial cells, are summarized by Marshall and colleagues (Iwata et al., 2014; Adesina et al., 2015; Rafikov et al., 2015; Ghouleh et al., 2017; Marshall et al., 2018; Martinho et al., 2020). Ultimately, mitochondrial dysregulation leads to mitophagy and decreased mitochondrial biogenesis, as described in animal models and patients with PH (Haslip et al., 2015; Ryan et al., 2015; Aggarwal et al., 2016; Marshall et al., 2018). Administration of mitoTEMPO, a mitochondrial-specific antioxidant, and activation of nuclear respiratory factor 2 (NRF-2), a transcription factor that reduces ROS production, improves mitochondrial dysfunction, decreases ROS levels, and attenuates the pathophysiology associated with BPD-PH in mice (Eba et al., 2013; Datta et al., 2015).
Collectively, the published data strongly support an important role for mitochondrial dysfunction in the pathogenesis of BPD-PH and its associated mortality. Thus, strategies that can preserve mitochondria integrity during early development in ELGANs could potentially be useful in reducing the burden of disease from BPD-PH.
3 The role of PGC-1α in mitochondrial dysfunction
Peroxisome proliferator-activated receptor gamma coactivator-1 alpha (PGC-1α) is a major transcriptional coactivator of several critical downstream pathways that regulate mitochondrial biogenesis, ROS detoxification, and oxidative phosphorylation (Wu et al., 1999; Villena, 2015). PGC-1α is also critical in regulating cellular metabolism, thus its expression is enhanced in tissues and organs with high energy requirements including the liver, cardiac and skeletal muscle, kidney, brown adipose tissue, brain, and retina (Handschin and Spiegelman, 2006; Liang and Ward, 2006; Handschin, 2009). Importantly, PGC-1α expression and activity are very tissue specific and while studies have examined tissues that highly express PGC-1α, our knowledge of the isoforms expressed in the brain and lungs and how they are regulated is incomplete (Martínez-Redondo et al., 2015; Jannig et al., 2022).
PGC-1α co-activates transcription factors involved in mitochondrial biogenesis and function, including nuclear respiratory factors (NRF−1 and −2), peroxisome proliferator-activated receptors (PPARs), mitochondrial transcription factor A (TFAM), and estrogen-related receptor alpha (ERRα) (Bastin et al., 2008; Rebelo et al., 2011; Gureev et al., 2019). PGC-1α decreases mitochondrial ROS by modulating the expression and activity of mitochondrial antioxidant enzymes including SOD2, catalase, thioredoxin reductase 2, PRX-5, PRX-3, UCP-2, and TRX-2 (Valle et al., 2005; Rius-Pérez et al., 2020). These free radical scavenging enzymes protect cells from mitochondrial dysfunction and subsequent oxidative stress.
PGC-1α dysregulation is also closely tied with inflammation and the nuclear factor kappa-light-chain-enhancer of activated B cells (NF-κB) signaling pathway (Valle et al., 2005; Handschin and Spiegelman, 2006; Palomer et al., 2009; Remels et al., 2013; Eisele et al., 2015; Dinulovic et al., 2016; Kadlec et al., 2016). PGC-1α directly inhibits NF-κB by binding to the p65 subunit and preventing its translocation to the nucleus and transcription of genes encoding pro-inflammatory cytokines (Alvarez-Guardia et al., 2010; Eisele et al., 2013). Inflammation represses PGC-1α activity which subsequently downregulates the gene expression of mitochondrial antioxidants, increases ROS levels and oxidative injury, and further exacerbates the inflammatory response. The relationship between PGC-1α, inflammation, and oxidative stress is summarized by Rius-Pérez and colleagues (Rius-Pérez et al., 2020).
3.1 PGC-1α dysregulation in the brain
Normal brain development has high energy requirements hence, mitochondria biogenesis and effective detoxification of ROS and RNS are essential to the process (Schon and Manfredi, 2003; Li et al., 2017). Studies using brain tissue from adults with multiple sclerosis, a demyelinating disease triggered by inflammation, show that a low level of PGC-1α is associated with the process of demyelination (Nijland et al., 2014). PGC-1α overexpression in human astrocytes reduced pro-inflammatory mediators, ROS production, and cell death, and protected neuronal cells co-cultured with the astrocytes against oxidative injury, suggesting a protective role for PGC-1α in reducing neurodegeneration (Nijland et al., 2014). These findings support the need for future studies exploring the role of PGC-1α in normal and abnormal brain development, specifically as it relates to WMI and PVL in preterm infants.
Kuczynska and colleagues have written a comprehensive review on the role of PGC-1α in the central and peripheral nervous system and its involvement in various neurodegenerative and neuropsychiatric disorders in adults (Schon and Manfredi, 2003; Chen et al., 2011; Kuczynska et al., 2022). Notably, PGC-1α deficiency in adults leads to abnormal myelination, neurodegeneration, and structural abnormalities seen in amyotrophic lateral sclerosis (ALS), Parkinson’s, Huntington’s, and Alzheimer’s diseases in adult humans and animal models (Lin et al., 2004; Leone et al., 2005; Zheng et al., 2010; Thau et al., 2012; Tsunemi et al., 2012; Johri et al., 2013; Lucas et al., 2015; Sweeney and Song, 2016; Mota and Sastre, 2021; Piccinin et al., 2021). These disorders are also associated with oxidative stress, mitochondrial dysfunction, and neuroinflammation. Microglia in the central nervous system have important immune functions and can display pro-inflammatory (M1) or anti-inflammatory (M2) phenotypes depending on the surrounding microenvironment (Gagliardi et al., 2009). They also play a large role in brain development, particularly in myelination (McNamara and Miron, 2020). During inflammation, PGC-1α is necessary for promoting and maintaining the M2 anti-inflammatory microglia phenotype (Yang et al., 2017). PGC-1α overexpression in microglia significantly decreases ischemic injury-induced neurologic deficits by reducing NLRP3 inflammasome activation and pro-inflammatory cytokine production, and enhancing mitophagy in adult mice (Han et al., 2021).
Given the role of mitochondrial dysfunction and oxidative stress in adult disease pathophysiology and the significance of PGC-1α as a master regulator of mitochondrial biogenesis, it is likely that PGC-1α signaling also plays an important role in the pathogenesis of WMI and PVL. However, there are limited preclinical models in newborn animals examining the role and importance of PGC-1α activity and regulation in neonatal brain injury. Additionally, emerging evidence suggests that hypoxia may also regulate PGC-1α activity (Shoag and Arany, 2010). Neonatal hypoxic-ischemic brain injury in rats induces mitochondrial biogenesis, specifically increasing NRF-1 and TFAM gene and protein expression (Yin et al., 2008). Intermittent hypoxia also has a neuroprotective effect in adult rats with cerebral ischemia injury, by improving mitochondrial health and function as well as promoting mitochondrial biogenesis through AMPK-PGC-1α-SIRT3 activation (Su et al., 2022). Further studies are required to understand the complex relationship between hypoxia and PGC-1α signaling and its relevance to neonates who experience periods of intermittent hypoxia is particularly critical.
3.2 PGC-1α dysregulation in the lungs
BPD is characterized by arrested lung development, and although limited, published data support an essential role for PPARγ and PGC-1α in numerous processes involved in lung development. For instance it stimulates interstitial lipofibroblast maturation and differentiation, and the transdifferentiation of myofibroblasts into lipofibroblasts, essential for alveolarization (Torday et al., 2003; Simon et al., 2006a; Simon et al., 2006b; Rehan et al., 2006; Rehan and Torday, 2012). PGC-1α also activates TFAM which is key for normal lung development and postnatal survival; TFAM mutations in utero lead to lethal abnormalities in branching morphogenesis (Srivillibhuthur et al., 2018). The Wnt/β-catenin pathways involved in organogenesis is tightly regulated during lung development, injury, and repair. This pathway is persistently activated in infants with BPD (Lingappan and Savani, 2020). Overactivation of the Wnt/β-catenin canonical pathway is associated with disrupted lung development and maturation (Lecarpentier et al., 2019; Zhang et al., 2020). Increased activation of this pathway has an inhibitory effect on PPARγ. The relationship between the Wnt/β-catenin and PPARγ pathways are reviewed in detail by Lecarpentier and colleagues (Lecarpentier et al., 2019).
M2 macrophages in the lung are critical for branching morphogenesis and alveolarization during lung development (Jones et al., 2013). PGC-1α, similar to its role in the brain, regulates macrophage polarization to an anti-inflammatory M2 phenotype during inflammation (Eisele et al., 2013; Eisele et al., 2015; Fontecha-Barriuso et al., 2019). Moreover, aberrant PGC-1α regulation is found in tissue from patients with idiopathic pulmonary fibrosis, asthma, COPD, PH, and lung cancers (Li et al., 2010; Mata et al., 2012; Rao et al., 2012; Ye et al., 2016; Chan and Rubin, 2017; Caporarello et al., 2019; Aghapour et al., 2020; Bueno et al., 2020; Larson-Casey et al., 2020; Oh et al., 2021; Saito et al., 2021; Xu et al., 2022). Taken together these data from pre-clinical studies support an important and necessary role for PGC-1α in normal lung development and dysregulation. Fewer data are available describing the role of PGC-1α in BPD-PH. However, since PGC-1α regulates mitochondrial function, oxidative stress, and inflammation, all of which are essential processes involved in BPD-PH pathogenesis, it is highly likely that it could be an effective therapeutic target that could modify the development of BPD-PH (2, 71, 76). Thus, for the remainder of this review, we will discuss therapeutics that can upregulate PGC-1α expression and activity (Figure 2).
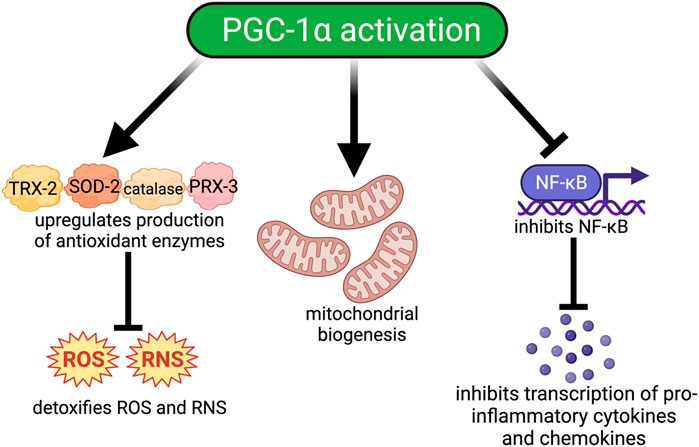
FIGURE 2. Downstream signaling pathways modulated by PGC-1α activation. Activation of PGC-1α. 1) improves oxidative injury by increasing the production of antioxidant enzymes such as catalase, thioredoxin-2 (TRX-2), peroxiredoxin-3 (PRX-3), and manganese superoxide dismutase (SOD-2) which detoxify ROS/RNS; 2) Increases mitochondrial biogenesis by upregulating the transcription of important transcription factors such as NRF-1, NRF-2, and TFAM; and 3) Reduces inflammation by inhibiting NF-κB and the transcription of proinflammatory cytokines and chemokines.
4 Therapeutic agents that can regulate PGC-1α activity
PGC-1α can be transcriptionally regulated through the cAMP response element-binding (CREB) pathway and activated by post-translational modifications including deacetylation by sirtuin 1 (SIRT1) and phosphorylation by adenosine monophosphate-activated kinase (AMPK) (Figure 3). AMPK can also indirectly activate PGC-1α through activation of SIRT1 by increasing nicotinamide adenine dinucleotide (NAD+) levels. Upon activation, PGC-1α translocates from the cytoplasm to the nucleus where it co-activates the transcription of multiple transcription factors including NRF-1 and NRF-2. NRF-1 and NRF-2 are transcription factors for TFAM, which regulates aspects of energy metabolism including synthesis of ETC subunits and mitochondrial biogenesis.
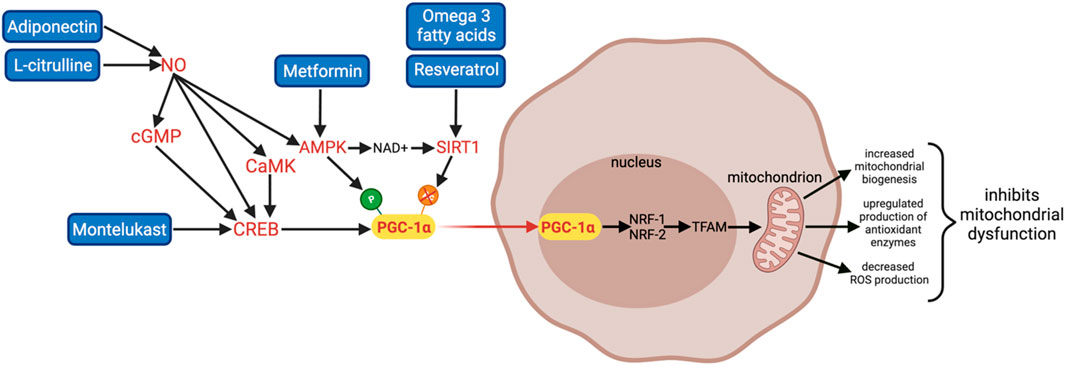
FIGURE 3. A summary of 6 promising therapies that can activate PGC-1α and its downstream pathways: Adiponectin, L-citrulline, Montelukast, Metformin, omega 3 fatty acids, and Resveratrol. Adiponectin and L-citrulline increase NO bioavailability; NO can indirectly upregulate PGC-1α through multiple pathways including the CREB, NO/cGMP/CREB, NO/CaMK pathways, and AMPK activation. Montelukast activates PGC-1α transcription directly via the CREB pathway. Metformin is an AMPK activator; it activates PGC-1α via phosphorylation. Omega 3 fatty acids and Resveratrol are SIRT1 activators; they activate PGC-1α via deacetylation. Therapies that can activate PGC-1α are beneficial in treating WMI and BPD by increasing mitochondrial biogenesis, detoxifying ROS to inhibit oxidative stress and mitochondrial dysfunction, and inhibiting inflammation.
Moreover, mitochondrial function and biogenesis are also regulated by complex mechanisms where nitric oxide (NO) is a key factor (Figure 3). NO modulates the expression of PGC-1α and its downstream effectors through four different pathways: increasing the second messenger cyclic guanosine monophosphate (cGMP) via activation of particulate guanylyl cyclase, activating AMPK, upregulating CREB, and via the calcium–calmodulin-dependent protein kinase (CaMK), an upstream activator of CREB (Riccio et al., 2006; Lira et al., 2010; Fernandez-Marcos and Auwerx, 2011; Rius-Pérez et al., 2020). Hence, therapies that increase NO bioavailability can also enhance PGC-1α activity.
PGC-1α expression and activation are complex and extensively controlled so while we discuss AMPK and SIRT1 activators, the CREB pathway, and NO donors, it is important to note that there are numerous transcriptional, post-transcriptional, and post-translational modifications to consider and explore (Martínez-Redondo et al., 2015; Miller et al., 2019; Jannig et al., 2022). Notably, while PGC-1α modulation may be one potential mechanism by which these therapeutics act, their efficacy is likely attributed to the modulation of numerous pathways. Furthermore, although increased PGC-1α gene and protein expression may be beneficial, it does not necessitate increased PGC-1α activity and further, an effect on the downstream pathways regulated by PGC-1α. Current studies show promising results however, further studies are critical in understanding how the discussed therapeutics have favorable effects.
4.1 AMPK activators
4.1.1 Metformin
Metformin is an ancient traditional herbal medicine naturally found in the French lilac flower now commonly used to treat diabetes. It can upregulate PGC-1α via AMPK phosphorylation. There is extensive evidence to support metformin as an AMPK activator in skeletal muscle, liver, heart, stomach, pancreas, and kidney (Miao and Scutt, 2002; Suwa et al., 2006; Kristensen et al., 2013; Aatsinki et al., 2014; Chan and Rubin, 2017; Izzo et al., 2017). Metformin prevents neurodegeneration and encourages neural repair in pre-clinical studies of childhood brain injury and neonatal stroke in rodents (Dadwal et al., 2015; Kang et al., 2017; Rotermund et al., 2018; Ruddy et al., 2019). It is also helpful in treating COVID-related lung inflammation and acute respiratory distress syndrome (ARDS) (Xian et al., 2021). Metformin attenuates inflammation and prevents the onset of ARDS by decreasing IL-1β and IL-6 mRNA and protein production, and NLRP3 inflammasome activation in mice (Xian et al., 2021). Additionally, it alleviates pulmonary fibrosis and prevents PH in pre-clinical studies (Agard et al., 2009; Chan and Rubin, 2017; Goncharov et al., 2018; Liu et al., 2019a; Mulkareddy and Simon, 2020; Cheng et al., 2021; Wu et al., 2021).
Metformin is currently one of few oral medications approved for use in children 10 years of age or older and in mothers with gestational diabetes (Wu et al., 1999; Handschin and Spiegelman, 2006; Villena, 2015). Metformin crosses the placenta; its reported effects on infants in utero are mixed. Some data report no adverse effects of in utero metformin exposure on the fetus and normal growth and development during infancy and childhood (Glueck et al., 2004; Given et al., 2018; Landi et al., 2019; Greger et al., 2020; Jorquera et al., 2020). Whereas others showed a strong association between in utero metformin exposure and decreased birth weight, excessive weight gain in infancy, and higher BMI during childhood (Ijäs et al., 2015; Tarry-Adkins et al., 2019; Estrella et al., 2021; Yu et al., 2021). These findings are concerning since low birth weight followed by rapid postnatal growth puts infants at a higher risk of developing cardiovascular disease and type 2 diabetes (Nguyen et al., 2018; Tarry-Adkins et al., 2019; Estrella et al., 2021). Thus, additional studies are necessary to determine whether metformin is a safe therapeutic to increase PGC-1α activity and target the pathological processes contributing to WMI and BPD in neonates.
4.2 SIRT1 activators
4.2.1 Resveratrol
Resveratrol is a polyphenol compound naturally found in red grapes, berries, peanuts, and cocoa. It has potent antioxidant, anti-inflammatory, and anti-apoptotic properties, and activates PGC-1α through the SIRT1 pathway (Tellone et al., 2015; Hoda et al., 2017; Gomes et al., 2018; Xuefei et al., 2021; Zhou et al., 2021). The preponderance of published data, reviewed by Zhu and colleagues, supports the therapeutic benefit of resveratrol in treating respiratory diseases such as BPD-PH, asthma, and pulmonary fibrosis in neonatal and adult models (Liu et al., 2015; Xu et al., 2015; Zhu et al., 2017). Resveratrol upregulates SIRT1, PGC-1α, NRF-1, and TFAM in human alveolar epithelial cells exposed to hyperoxia, improving mitochondrial dysfunction, reducing mitochondrial ROS, and apoptosis (Zhu et al., 2021). It inhibits lipopolysaccharide (LPS)-induced activation of the NF-κB inflammatory pathway, secretion of pro-inflammatory cytokines, and the NLRP3 inflammasome in rodents (Jiang et al., 2016; Wang et al., 2017). Both resveratrol and acetylresveratrol, a prodrug or inactive form of resveratrol, reduce mortality, prevent LPS-induced morphological changes in the lung, activation of NF-κB, secretion of pro-inflammatory cytokines, as well as LPS-induced inhibition of SIRT1 expression in adult mice (Özdemir et al., 2014; Ma et al., 2015). Furthermore, resveratrol inhibits injury-induced human pulmonary microvascular endothelial cell apoptosis and PASMCs proliferation which play a fundamental role in PH pathophysiology (Xia et al., 2011; Chen et al., 2014). Resveratrol also preserves myelination and protects against neurodegeneration in a variety of neurodegenerative diseases such as Alzheimer’s, Parkinson’s, and Huntington’s disease (Xiang et al., 2011; Tellone et al., 2015). It activates PGC-1α signaling, promoting mitochondrial biogenesis, improving oxidative stress, and decreasing ROS levels in a preclinical model of subarachnoid hemorrhage (Zhou et al., 2021). Lastly, resveratrol promotes M2 microglia polarization in a pro-inflammatory mileu (Nguyen et al., 2018). Despite its therapeutic potential, resveratrol has a low bioavailability, water solubility, and chemical stability. Suitable formulations, methods of administration, and storage conditions are needed due to its sensitivity to light, temperature, and pH, and further work is required to understand the effects of resveratrol on brain and lung disease in preterm infants (Zhu et al., 2017; Shaito et al., 2020).
4.2.2 Omega 3 fatty acids
Omega 3 fatty acid supplementation is associated with improved health outcomes related to brain and lung diseases across the lifespan (Newberry et al., 2016; Derbyshire, 2018; Derbyshire, 2019). The three key omega 3 fatty acids: eicosapentaenoic acid (EPA), docosahexaenoic acid (DHA), and alpha-linolenic acid (ALA), are naturally found in seafood, nuts, and different oils. Additionally, breast milk is an excellent source of omega 3 for newborn infants (Berenhauser et al., 2012; Samuel et al., 2020). The positive effect of omega 3 fatty acids is largely attributed to PGC-1α activation through the SIRT1/3 pathways (Son et al., 2021). Omega 3 fatty acids are essential for brain and retinal development during the postnatal period and decreased levels are associated with increased risk for neonatal morbidities such as respiratory disease, decline in cognitive and motor abilities, and decreased postnatal growth (Martin et al., 2011; Bernardi et al., 2012; Fink et al., 2016; Lapillonne and Moltu, 2016; Gould et al., 2018). Maternal omega 3 fatty acid supplementation protects the newborn rat brain against WMI; specifically, brain weight loss, apoptosis, and hypomyelination (Tuzun et al., 2012). However, the reported effects of omega 3 fatty acids on preterm infants with BPD are varied. Some studies report that a high dose of DHA supplementation effectively decreases the incidence of BPD (Manley et al., 2011; Zhang et al., 2014; Fink et al., 2016) whereas a meta-analysis investigating the effect of omega 3 fatty acids on BPD found no significant effect of supplementation on the incidence of BPD in preterm infants (Wang et al., 2019a). The dosage, frequency of administration, as well as pharmaceutical ingredients of the omega 3 fatty acid supplements given to infants in the different studies was not consistent, suggesting promise for future trials. Limited studies have investigated the mechanism for the protective effect of omega 3 supplementation. However, recent studies report that it rescues PGC-1α, SIRT1/3, NRF-1/2, and AMPK protein expression in nephrectomy rats, and significantly increases total mitochondrial content in rodent skeletal muscle tissue and human skeletal muscle cells (Vaughan et al., 2012; Liu et al., 2019b; Son et al., 2021). Omega 3 fatty acids are safe and well-tolerated orally in infants and children hence, further mechanistic studies exploring its effect on PGC-1α signaling in the brain and lung during development would enhance our understanding of the therapeutic potential in preterm infants.
4.3 CREB pathway
4.3.1 Montelukast
Montelukast is a leukotriene receptor antagonist commonly used to treat asthma. Data from some preliminary studies support a therapeutic benefit in preterm infants with severe BPD while others found no efficacy in reducing moderate or severe BPD (Kim et al., 2009; Rupprecht et al., 2014). It blocks hyperoxia-induced abnormal alveolarization and septation indicative of BPD injury in the lungs of newborn mice (Chen et al., 2019; Chen et al., 2020). It decreases the inflammatory response by inhibiting NF-κB, reduces markers of oxidative stress, and inhibits hyperoxia-induced apoptosis in rodent lungs and alveolar epithelial cells (Chen et al., 2019). Montelukast increases mitochondrial biogenesis in the lung via activation of the CREB pathway, consequently upregulating the expression of the PGC-1α-NRF-1-TFAM pathway (Wang et al., 2019b). CREB is an important transcription factor that can directly regulate PGC-1α expression (Wu et al., 1999; Fernandez-Marcos and Auwerx, 2011; Schmidt and Mandrup, 2011; Cheng et al., 2012; Miller et al., 2019; McMeekin et al., 2021). Montelukast treatment significantly increases mitochondrial mass, DNA, and function including respiration and ATP production in bronchial epithelial cells (Wang et al., 2019b). CREB inhibition in these cells via the H89 pharmacological inhibitor prevents montelukast-induced PGC-1α upregulation (Wang et al., 2019b). Moreover, montelukast is neuroprotective in young children and adults with brain tumors, traumatic brain injury, brain ischemia, and epilepsy but has not been tested in infants with WMI (Biber et al., 2009; Rupprecht et al., 2014; Eriksson et al., 2018; Fatima et al., 2019; Tesfaye et al., 2021). Overall, montelukast is a low-risk drug that has been used in infants and children of various ages with no significant adverse effects reported. These promising data support future trials to determine its efficacy in reducing WMI in preterm infants (Kim et al., 2009; Hon et al., 2014; Rupprecht et al., 2014).
4.4 Nitric oxide donors
4.4.1 L-citrulline
L-citrulline is a non-essential amino acid naturally found in watermelons. It is an intermediate in the urea cycle where L-arginine is metabolized by nitric oxide synthase (NOS) to produce L-citrulline and NO thus, serving as a NO donor (Rashid et al., 2020). Preterm infants have significantly lower L-citrulline plasma levels than neonates born at term and subsequently, decreased NO bioavailability (Contreras et al., 2017). Plasma L-citrulline levels are lower in infants with BPD-PH than those without PH, suggesting that L-citrulline may be used as a biomarker (Montgomery et al., 2016). Moreover, adults with severe sepsis who develop ARDS have lower plasma levels of L-citrulline than those who do not develop ARDS (Ware et al., 2013).
L-citrulline has been used to reduce the severity of injury in hyperoxic and inflammatory models of lung injury during development. Pre-treatment with L-citrulline prevents hyperoxia or inflammation-induced arrested alveolar and vascular growth in newborn rodent models of BPD-PH (Vadivel et al., 2010; Grisafi et al., 2012; Ma et al., 2017; Dedja et al., 2018; Lauterbach et al., 2018; Martinho et al., 2020). L-citrulline supplementation also has neuroprotective effects. It prevents neuronal death and cerebrovascular injury, and increases endothelial NOS expression in a mouse model of adult brain ischemia (Yabuki et al., 2013). The protective effects of L-citrulline in the brain and lung have largely been attributed to increased NO bioavailability as NO is critical for normal brain and lung development (Ergenekon and Gücüyener, 1998; Ricciardolo, 2003; Garthwaite, 2008; Paul and Ekambaram, 2011; Garry et al., 2015). While L-arginine supplementation has also been investigated, its efficacy in increasing NO levels is limited by the first pass effect hence, L-citrulline is a more potent NO donor (Agarwal et al., 2017; Khalaf et al., 2019; Scott et al., 2021). The importance of L-citrulline as a NO donor is undeniable however, NO treatment alone does not decrease BPD incidence in human infants and nearly 40% of infants are resistant or fail to respond to inhaled NO (Raffay et al., 2012; Martinho et al., 2020). Recently, Villareal and colleagues determined that L-citrulline increases PGC-1α mRNA and protein expression in mouse skeletal muscle via increased NO production and phosphorylation of CREB (Villareal et al., 2018). As outlined above, NO can activate PGC-1α to upregulate mitochondrial biogenesis via four different pathways: cGMP generation, AMPK activation, CREB pathway, and CaMK protein (Borniquel et al., 2006; Riccio et al., 2006; Lira et al., 2010; Fernandez-Marcos and Auwerx, 2011; Baldelli et al., 2014; Craige et al., 2016; Rius-Pérez et al., 2020). Since L-citrulline is safe, well-tolerated orally, and readily available, it is an ideal natural biologic for use in preterm neonates (Ma et al., 2017; Villareal et al., 2018; Roberts et al., 2021). Further studies are needed to determine its efficacy in preventing BPD-PH and improving neurodevelopmental outcomes directly or indirectly as infants with BPD-PH have a significantly increased risk for poor neurodevelopment outcomes (Choi et al., 2019; DeMauro, 2021; Grelli et al., 2021).
4.4.2 Adiponectin
Adiponectin, secreted by adipose tissue, binds to AdipoR1 and AdipoR2 receptors on many cells throughout the body with pleiotropic effects, including vasodilatory, angiogenic, anti-inflammatory, and antioxidant properties (Wang et al., 2008; Hansen-Pupp et al., 2015; Gauda and Master, 2018). It is well known for its effects on metabolism including improved glucose tolerance, increased fatty acid oxidation in liver and skeletal muscle, and decreased vascular inflammation. Serum adiponectin levels are indirectly correlated with fat mass in adults, whereas they are directly proportional in infants (Hansen-Pupp et al., 2015; Gauda and Master, 2018). Therefore, serum adiponectin is significantly lower in preterm infants than in full-term infants (Kamoda et al., 2004; Siahanidou et al., 2007; Saito et al., 2011; Oberthuer et al., 2012; Han et al., 2021). Adiponectin crosses the blood-brain barrier and binds to AdipoR1 and AdipoR2, expressed in brain endothelial cells (Thundyil et al., 2012). It protects the brain from cerebral ischemic injury, neurodegenerative diseases such as Alzheimer’s disease, as well as psychological disorders including post-traumatic stress disorder, anxiety, and depression (Hu et al., 2015; Na et al., 2017; Zhang et al., 2017; Bloemer et al., 2018; Wu, 2019; Polito et al., 2020; Li et al., 2021). Adiponectin deficiency is associated with increased white matter lesions, increased mortality post-ischemic stroke, and decreased cognitive function in adults (Efstathiou et al., 2005; Quan et al., 2022). A study in newborn rats shows that exogenous adiponectin can attenuate hypoxia-ischemia-induced brain injury by binding to its receptors and upregulating AMPK signaling (Xu et al., 2018). Adiponectin and its receptors are also expressed in various cell types in the lungs (Garcia and Sood, 2012). It has a protective role in inflammatory lung injuries such as COPD and asthma and pre-treatment with adiponectin also prevents LPS-induced BPD phenotype in newborn rats (Garcia and Sood, 2012; Ivanovska et al., 2020). Preclinical studies using adiponectin knockout mice corroborate findings in other rodent injury models showing increased lung injury and inflammation with adiponectin deficiency (Shah et al., 2022).
The preponderance of data suggest adiponectin signaling could protect the newborn brain and lung from oxidative injury via its antioxidant and anti-inflammatory properties. Interestingly, the different pharmacological agents outlined above, resveratrol, L-citrulline, metformin, and DHA (omega 3 fatty acids) all increase plasma adiponectin levels (Adamia et al., 2007; Gray et al., 2013; Joffin et al., 2015; Jimoh et al., 2018). Importantly, adiponectin may increase mitochondrial biogenesis through indirect PGC-1α upregulation. It activates PGC-1α via CaMK, AMPK, and SIRT1 activation by inducing calcium influx through the AdipoR1 receptor (Iwabu et al., 2010; Guo et al., 2020). AdipoR1 activation also effectively enhances NO production, a downstream regulator of various pathways which modulate PGC-1α expression and activity (Chen et al., 2003; Goldstein et al., 2009; Cohen et al., 2022). Despite its potential, high plasma adiponectin levels and AdipoR signaling have also been associated with chronic inflammation, including autoimmune diseases, chronic kidney disease, inflammatory bowel disease, and cystic fibrosis (Choi et al., 2020). Presently, small molecules that bind to adiponectin receptors are being explored since adiponectin is not available for use in humans. These agonists show promise in adult models of disease (Iwabu et al., 2010; Okada-Iwabu et al., 2013; Balasubramanian et al., 2022).
5 Conclusion
Mitochondrial dysregulation occurs in many developing cells and tissues as a result of oxidative stress and inflammation that are operative in the pathogenesis of WMI and BPD in premature infants. The transcriptional coactivator PGC-1α is a dynamic and potent transcriptional coactivator and master regulator of mitochondrial biogenesis and function therefore, therapeutics that can upregulate its expression and activity may be beneficial for use in diseases with underlying mitochondrial dysfunction. Upregulating PGC-1α activity, a key regulator of cellular metabolism, is also critical in addressing the large energy demands to support postnatal organ development. While therapies including metformin, resveratrol, omega 3 fatty acids, montelukast, L-citrulline, and adiponectin show great promise, there is much to be done to better understand and uncover how these therapeutics may affect PGC-1α activity. The precise mechanisms of action are complex and warrant further research. Most existing studies investigate PGC-1α signaling in highly metabolic tissues such as skeletal, cardiac, and adipose tissue so our understanding of PGC-1α such as the isoforms present, their functions, and how they are regulated in the brain and lungs are especially limited. Future studies in this field will allow us to better understand how the therapeutics discussed may beneficially regulate PGC-1α in the brain and lungs. Importantly, further studies are needed to understand their safety and efficacy in preterm infants at risk for brain and lung injury. It is also necessary to determine the optimal dose to maximize health benefits without toxicity, short and long-term side effects, and off-target effects that would be detrimental to the developing premature infant (Kaplowitz, 2017; Yang et al., 2018; Soliman et al., 2020; Raman and Foster, 2021).
Author contributions
AM wrote the manuscript and designed the figures. RH contributed to specific portions of the manuscript. EBG edited and revised the manuscript. All authors reviewed and approved the final version of the manuscript.
Funding
This work was supported by the Women’s Auxiliary Volunteers, The Hospital for Sick Children.
Acknowledgments
We thank Julijana Ivanovska, Harvard Tran, and Nikola Ivanovski for their insightful discussions and support. Figures created with BioRender.com.
Conflict of interest
The authors declare that the research was conducted in the absence of any commercial or financial relationships that could be construed as a potential conflict of interest.
Publisher’s note
All claims expressed in this article are solely those of the authors and do not necessarily represent those of their affiliated organizations, or those of the publisher, the editors and the reviewers. Any product that may be evaluated in this article, or claim that may be made by its manufacturer, is not guaranteed or endorsed by the publisher.
References
Aatsinki S. M., Buler M., Salomäki H., Koulu M., Pavek P., Hakkola J. (2014). Metformin induces PGC-1α expression and selectively affects hepatic PGC-1α functions. Br. J. Pharmacol. 171 (9), 2351–2363. doi:10.1111/bph.12585
Adamia N., Virsaladze D., Charkviani N., Skhirtladze M., Khutsishvili M. (2007). Effect of metformin therapy on plasma adiponectin and leptin levels in obese and insulin resistant postmenopausal females with type 2 diabetes. Georgian Med. News 2007 (145), 52–55.
Adesina S. E., Kang B. Y., Bijli K. M., Ma J., Cheng J., Murphy T., et al. (2015). Targeting mitochondrial reactive oxygen species to modulate hypoxia-induced pulmonary hypertension. Free Radic. Biol. Med. 87, 36–47. doi:10.1016/j.freeradbiomed.2015.05.042
Afolayan A. J., Eis A., Teng R. J., Bakhutashvili I., Kaul S., Davis J. M., et al. (2012). Decreases in manganese superoxide dismutase expression and activity contribute to oxidative stress in persistent pulmonary hypertension of the newborn. Am. J. Physiol. Lung Cell. Mol. Physiol. 303 (10), L870–L879. doi:10.1152/ajplung.00098.2012
Agard C., Rolli-Derkinderen M., Dumas-de-La-Roque E., Rio M., Sagan C., Savineau J., et al. (2009). Protective role of the antidiabetic drug metformin against chronic experimental pulmonary hypertension. Br. J. Pharmacol. 158 (5), 1285–1294. doi:10.1111/j.1476-5381.2009.00445.x
Agarwal U., Didelija I. C., Yuan Y., Wang X., Marini J. C. (2017). Supplemental citrulline is more efficient than arginine in increasing systemic arginine availability in mice. J. Nutr. 147 (4), 596–602. doi:10.3945/jn.116.240382
Aggarwal S., Mannam P., Zhang J. (2016). Differential regulation of autophagy and mitophagy in pulmonary diseases. Am. J. Physiol. Lung Cell. Mol. Physiol. 311 (2), L433–L452. doi:10.1152/ajplung.00128.2016
Aghapour M., Remels A. H. V., Pouwels S. D., Bruder D., Hiemstra P. S., Cloonan S. M., et al. (2020). Mitochondria: At the crossroads of regulating lung epithelial cell function in chronic obstructive pulmonary disease. Am. J. Physiol. Lung Cell. Mol. Physiol. 318 (1), L149–L164. doi:10.1152/ajplung.00329.2019
Agut T., Alarcon A., Cabañas F., Bartocci M., Martinez-Biarge M., Horsch S., et al. (2020). Preterm white matter injury: Ultrasound diagnosis and classification. Pediatr. Res. 87 (1), 37–49. doi:10.1038/s41390-020-0781-1
Ahmad S., White C. W., Chang L. Y., Schneider B. K., Allen C. B. (2001). Glutamine protects mitochondrial structure and function in oxygen toxicity. Am. J. Physiol. Lung Cell. Mol. Physiol. 280 (4), L779–L791. doi:10.1152/ajplung.2001.280.4.L779
Ahmad T., Sundar I. K., Lerner C. A., Gerloff J., Tormos A. M., Yao H., et al. (2015). Impaired mitophagy leads to cigarette smoke stress-induced cellular senescence: Implications for chronic obstructive pulmonary disease. FASEB J. Off. Publ. Fed. Am. Soc. Exp. Biol. 29 (7), 2912–2929. doi:10.1096/fj.14-268276
Al-Ghanem G., Shah P., Thomas S., Banfield L., El Helou S., Fusch C., et al. (2017). Bronchopulmonary dysplasia and pulmonary hypertension: A meta-analysis. J. Perinatol. 37 (4), 414–419. doi:10.1038/jp.2016.250
Alvarez-Guardia D., Palomer X., Coll T., Davidson M. M., Chan T. O., Feldman A. M., et al. (2010). The p65 subunit of NF-kappaB binds to PGC-1alpha, linking inflammation and metabolic disturbances in cardiac cells. Cardiovasc. Res. 87 (3), 449–458. doi:10.1093/cvr/cvq080
Ambalavanan N., Mourani P. (2014). Pulmonary hypertension in bronchopulmonary dysplasia. Birth Defects Res. A Clin. Mol. Teratol. 100 (3), 240–246. doi:10.1002/bdra.23241
An H. S., Bae E. J., Kim G. B., Kwon B. S., Beak J. S., Kim E. K., et al. (2010). Pulmonary hypertension in preterm infants with bronchopulmonary dysplasia. Korean Circ. J. 40 (3), 131–136. doi:10.4070/kcj.2010.40.3.131
Angara S., Bhandari V. (2013). Targeting mitochondrial dysfunction in lung diseases: Emphasis on mitophagy. Front. Physiol. 4, 384. doi:10.3389/fphys.2013.00384
Arjaans S., Haarman M. G., Roofthooft M. T. R., Fries M. W. F., Kooi E. M. W., Bos A. F., et al. (2021). Fate of pulmonary hypertension associated with bronchopulmonary dysplasia beyond 36 weeks postmenstrual age. Arch. Dis. Child. Fetal Neonatal Ed. 106 (1), 45–50. doi:10.1136/archdischild-2019-318531
Baburamani A. A., Supramaniam V. G., Hagberg H., Mallard C. (2014). Microglia toxicity in preterm brain injury. Reprod. Toxicol. 48, 106–112. doi:10.1016/j.reprotox.2014.04.002
Back S. A. (2017). White matter injury in the preterm infant: Pathology and mechanisms. Acta Neuropathol. Berl. 134 (3), 331–349. doi:10.1007/s00401-017-1718-6
Balasubramanian P., Schaar A. E., Gustafson G. E., Smith A. B., Howell P. R., Greenman A., et al. (2022). Adiponectin receptor agonist AdipoRon improves skeletal muscle function in aged mice. eLife 11, e71282. doi:10.7554/eLife.71282
Baldelli S., Lettieri Barbato D., Tatulli G., Aquilano K., Ciriolo M. R. (2014). The role of nNOS and PGC-1α in skeletal muscle cells. J. Cell Sci. 127, 4813–4820. doi:10.1242/jcs.154229
Ballinger S. W., Van Houten B., Jin G. F., Conklin C. A., Godley B. F. (1999). Hydrogen peroxide causes significant mitochondrial DNA damage in human RPE cells. Exp. Eye Res. 68 (6), 765–772. doi:10.1006/exer.1998.0661
Bastin J., Aubey F., Rötig A., Munnich A., Djouadi F. (2008). Activation of peroxisome proliferator-activated receptor pathway stimulates the mitochondrial respiratory chain and can correct deficiencies in patients’ cells lacking its components. J. Clin. Endocrinol. Metab. 93 (4), 1433–1441. doi:10.1210/jc.2007-1701
Berenhauser A. C., Pinheiro do Prado A. C., da Silva R. C., Gioielli L. A., Block J. M. (2012). Fatty acid composition in preterm and term breast milk. Int. J. Food Sci. Nutr. 63 (3), 318–325. doi:10.3109/09637486.2011.627843
Berkelhamer S. K., Kim G. A., Radder J. E., Wedgwood S., Czech L., Steinhorn R. H., et al. (2013). Developmental differences in hyperoxia-induced oxidative stress and cellular responses in the murine lung. Free Radic. Biol. Med. 61, 51–60. doi:10.1016/j.freeradbiomed.2013.03.003
Berkelhamer S. K., Mestan K. K., Steinhorn R. H. (2013). Pulmonary hypertension in bronchopulmonary dysplasia. Semin. Perinatol. 37 (2), 124–131. doi:10.1053/j.semperi.2013.01.009
Bernardi J. R., Escobar R. de S., Ferreira C. F., Silveira P. P. (2012). Fetal and neonatal levels of omega-3: Effects on neurodevelopment, nutrition, and growth. ScientificWorldJournal. 2012, 202473. doi:10.1100/2012/202473
Biber N., Toklu H. Z., Solakoglu S., Gultomruk M., Hakan T., Berkman Z., et al. (2009). Cysteinyl-leukotriene receptor antagonist montelukast decreases blood-brain barrier permeability but does not prevent oedema formation in traumatic brain injury. Brain Inj. 23 (6), 577–584. doi:10.1080/02699050902926317
Biouss G., Antounians L., Li B., O’Connell J. S., Seo S., Catania V. D., et al. (2019). Experimental necrotizing enterocolitis induces neuroinflammation in the neonatal brain. J. Neuroinflammation 16 (1), 97. doi:10.1186/s12974-019-1481-9
Bizzozero O. A., Sanchez P., Tetzloff S. U. (1999). Effect of ATP depletion on the palmitoylation of myelin proteolipid protein in young and adult rats. J. Neurochem. 72 (6), 2610–2616. doi:10.1046/j.1471-4159.1999.0722610.x
Bloemer J., Pinky P. D., Govindarajulu M., Hong H., Judd R., Amin R. H., et al. (2018). Role of adiponectin in central nervous system disorders. Neural Plast. 2018, e4593530. doi:10.1155/2018/4593530
Borniquel S., Valle I., Cadenas S., Lamas S., Monsalve M. (2006). Nitric oxide regulates mitochondrial oxidative stress protection via the transcriptional coactivator PGC-1alpha. FASEB J. Off. Publ. Fed. Am. Soc. Exp. Biol. 20 (11), 1889–1891. doi:10.1096/fj.05-5189fje
Brueckl C., Kaestle S., Kerem A., Habazettl H., Krombach F., Kuppe H., et al. (2006). Hyperoxia-induced reactive oxygen species formation in pulmonary capillary endothelial cells in situ. Am. J. Respir. Cell Mol. Biol. 34 (4), 453–463. doi:10.1165/rcmb.2005-0223OC
Bruns D. R., Brown R. D., Stenmark K. R., Buttrick P. M., Walker L. A. (2015). Mitochondrial integrity in a neonatal bovine model of right ventricular dysfunction. Am. J. Physiol. Lung Cell. Mol. Physiol. 308 (2), L158–L167. doi:10.1152/ajplung.00270.2014
Bueno M., Calyeca J., Rojas M., Mora A. L. (2020). Mitochondria dysfunction and metabolic reprogramming as drivers of idiopathic pulmonary fibrosis. Redox Biol. 33, 101509. doi:10.1016/j.redox.2020.101509
Cai J., Tuong C. M., Zhang Y., Shields C. B., Guo G., Fu H., et al. (2012). Mouse intermittent hypoxia mimicking apnoea of prematurity: Effects on myelinogenesis and axonal maturation. J. Pathol. 226 (3), 495–508. doi:10.1002/path.2980
Cannavò L., Perrone S., Viola V., Marseglia L., Di Rosa G., Gitto E. (2021). Oxidative stress and respiratory diseases in preterm newborns. Int. J. Mol. Sci. 22 (22), 12504. doi:10.3390/ijms222212504
Caporarello N., Meridew J. A., Jones D. L., Tan Q., Haak A. J., Choi K. M., et al. (2019). PGC1α repression in IPF fibroblasts drives a pathologic metabolic, secretory and fibrogenic state. Thorax 74 (8), 749–760. doi:10.1136/thoraxjnl-2019-213064
Chan D. C. (2006). Mitochondria: Dynamic organelles in disease, aging, and development. Cell 125 (7), 1241–1252. doi:10.1016/j.cell.2006.06.010
Chan S. Y., Rubin L. J. (2017). Metabolic dysfunction in pulmonary hypertension: From basic science to clinical practice. Eur. Respir. Rev. 26 (146), 170094. doi:10.1183/16000617.0094-2017
Chandel N. S., McClintock D. S., Feliciano C. E., Wood T. M., Melendez J. A., Rodriguez A. M., et al. (2000). Reactive oxygen species generated at mitochondrial complex III stabilize hypoxia-inducible factor-1alpha during hypoxia: A mechanism of O2 sensing. J. Biol. Chem. 275 (33), 25130–25138. doi:10.1074/jbc.M001914200
Chen B., Xue J., Meng X., Slutzky J. L., Calvert A. E., Chicoine L. G. (2014). Resveratrol prevents hypoxia-induced arginase II expression and proliferation of human pulmonary artery smooth muscle cells via Akt-dependent signaling. Am. J. Physiol. Lung Cell. Mol. Physiol. 307 (4), L317–L325. doi:10.1152/ajplung.00285.2013
Chen H., Montagnani M., Funahashi T., Shimomura I., Quon M. J. (2003). Adiponectin stimulates production of nitric oxide in vascular endothelial cells. J. Biol. Chem. 278 (45), 45021–45026. doi:10.1074/jbc.M307878200
Chen S. D., Yang D. I., Lin T. K., Shaw F. Z., Liou C. W., Chuang Y. C. (2011). Roles of oxidative stress, apoptosis, PGC-1α and mitochondrial biogenesis in cerebral ischemia. Int. J. Mol. Sci. 12 (10), 7199–7215. doi:10.3390/ijms12107199
Chen X., Peng W., Zhou R., Zhang Z., Xu J. (2020). Montelukast improves bronchopulmonary dysplasia by inhibiting epithelial-mesenchymal transition via inactivating the TGF-β1/Smads signaling pathway. Mol. Med. Rep. 22 (3), 2564–2572. doi:10.3892/mmr.2020.11306
Chen X., Zhang X., Pan J. (2019). Effect of montelukast on bronchopulmonary dysplasia (BPD) and related mechanisms. Med. Sci. Monit. 25, 1886–1893. doi:10.12659/MSM.912774
Cheng A., Wan R., Yang J. L., Kamimura N., Son T. G., Ouyang X., et al. (2012). Involvement of PGC-1α in the formation and maintenance of neuronal dendritic spines. Nat. Commun. 3, 1250. doi:10.1038/ncomms2238
Cheng D., Xu Q., Wang Y., Li G., Sun W., Ma D., et al. (2021). Metformin attenuates silica-induced pulmonary fibrosis via AMPK signaling. J. Transl. Med. 19 (1), 349. doi:10.1186/s12967-021-03036-5
Choi E. K., Shin S. H., Kim E. K., Kim H. S. (2019). Developmental outcomes of preterm infants with bronchopulmonary dysplasia-associated pulmonary hypertension at 18–24 months of corrected age. BMC Pediatr. 19 (1), 26. doi:10.1186/s12887-019-1400-3
Choi H. M., Doss H. M., Kim K. S. (2020). Multifaceted physiological roles of adiponectin in inflammation and diseases. Int. J. Mol. Sci. 21 (4), 1219. doi:10.3390/ijms21041219
Circu M. L., Aw T. Y. (2010). Reactive oxygen species, cellular redox systems, and apoptosis. Free Radic. Biol. Med. 48 (6), 749–762. doi:10.1016/j.freeradbiomed.2009.12.022
Cloonan S. M., Kim K., Esteves P., Trian T., Barnes P. J. (2020). Mitochondrial dysfunction in lung ageing and disease. Eur. Respir. Rev. 29 (157), 200165. doi:10.1183/16000617.0165-2020
Cohen K. E., Katunaric B., Schulz M. E., SenthilKumar G., Young M. S., Mace J. E., et al. (2022). Role of adiponectin receptor 1 in promoting nitric oxide-mediated flow-induced dilation in the human microvasculature. Front. Pharmacol. 13, 875900. doi:10.3389/fphar.2022.875900
Collins J. J. P., Tibboel D., de Kleer I. M., Reiss I. K. M., Rottier R. J. (2017). The future of bronchopulmonary dysplasia: Emerging pathophysiological concepts and potential new avenues of treatment. Front. Med. 4, 61. doi:10.3389/fmed.2017.00061
Contreras M. T., Gallardo M. J., Betancourt L. R., Rada P. V., Ceballos G. A., Hernandez L. E., et al. (2017). Correlation between plasma levels of arginine and citrulline in preterm and full-term neonates: Therapeutical implications. J. Clin. Lab. Anal. 31 (6), e22134. doi:10.1002/jcla.22134
Craige S. M., Kröller-Schön S., Li C., Kant S., Cai S., Chen K., et al. (2016). PGC-1α dictates endothelial function through regulation of eNOS expression. Sci. Rep. 6 (1), 38210. doi:10.1038/srep38210
Culley M. K., Chan S. Y. (2018). Mitochondrial metabolism in pulmonary hypertension: Beyond mountains there are mountains. J. Clin. Invest. 128 (9), 3704–3715. doi:10.1172/JCI120847
Dadwal P., Mahmud N., Sinai L., Azimi A., Fatt M., Wondisford F. E., et al. (2015). Activating endogenous neural precursor cells using metformin leads to neural repair and functional recovery in a model of childhood brain injury. Stem Cell Rep. 5 (2), 166–173. doi:10.1016/j.stemcr.2015.06.011
Darnall R. A., Chen X., Nemani K. V., Sirieix C. M., Gimi B., Knoblach S., et al. (2017). Early postnatal exposure to intermittent hypoxia in rodents is proinflammatory, impairs white matter integrity, and alters brain metabolism. Pediatr. Res. 82 (1), 164–172. doi:10.1038/pr.2017.102
Das K. C. (2013). Hyperoxia decreases glycolytic capacity, glycolytic reserve and oxidative phosphorylation in MLE-12 cells and inhibits complex I and II function, but not complex IV in isolated mouse lung mitochondria. PloS One 8 (9), e73358. doi:10.1371/journal.pone.0073358
Datta A., Kim G. A., Taylor J. M., Gugino S. F., Farrow K. N., Schumacker P. T., et al. (2015). Mouse lung development and NOX1 induction during hyperoxia are developmentally regulated and mitochondrial ROS dependent. Am. J. Physiol. Lung Cell. Mol. Physiol. 309 (4), L369–L377. doi:10.1152/ajplung.00176.2014
Dedja A., Gucciardi A., Giordano G., Gangi I. M. D., Porzionato A., Navaglia F., et al. (2018). Lipopolysaccharide-induced chorioamnionitis and postnatal lung injury: The beneficial effects of L-citrulline in newborn rats. Exp. Lung Res. 44 (4–5), 226–240. doi:10.1080/01902148.2018.1497730
DeMauro S. B. (2021). Neurodevelopmental outcomes of infants with bronchopulmonary dysplasia. Pediatr. Pulmonol. 56 (11), 3509–3517. doi:10.1002/ppul.25381
Deng W., Pleasure J., Pleasure D. (2008). Progress in periventricular leukomalacia. Arch. Neurol. 65 (10), 1291–1295. doi:10.1001/archneur.65.10.1291
Derbyshire E. (2018). Brain health across the lifespan: A systematic review on the role of omega-3 fatty acid supplements. Nutrients 10 (8), E1094. doi:10.3390/nu10081094
Derbyshire E. (2019). Oily fish and omega-3s across the life stages: A focus on intakes and future directions. Front. Nutr. 6, 165. doi:10.3389/fnut.2019.00165
Diebold I., Hennigs J. K., Miyagawa K., Li C. G., Nickel N. P., Kaschwich M., et al. (2015). BMPR2 preserves mitochondrial function and DNA during reoxygenation to promote endothelial cell survival and reverse pulmonary hypertension. Cell Metab. 21 (4), 596–608. doi:10.1016/j.cmet.2015.03.010
Dinulovic I., Furrer R., Di Fulvio S., Ferry A., Beer M., Handschin C. (2016). PGC-1α modulates necrosis, inflammatory response, and fibrotic tissue formation in injured skeletal muscle. Skelet. Muscle 6 (1), 38. doi:10.1186/s13395-016-0110-x
Dobson A. W., Grishko V., LeDoux S. P., Kelley M. R., Wilson G. L., Gillespie M. N. (2002). Enhanced mtDNA repair capacity protects pulmonary artery endothelial cells from oxidant-mediated death. Am. J. Physiol. Lung Cell. Mol. Physiol. 283 (1), L205–L210. doi:10.1152/ajplung.00443.2001
Douglas R. M., Ryu J., Kanaan A., del Carmen Rivero M., Dugan L. L., Haddad G. G., et al. (2010). Neuronal death during combined intermittent hypoxia/hypercapnia is due to mitochondrial dysfunction. Am. J. Physiol. Cell Physiol. 298 (6), C1594–C1602. doi:10.1152/ajpcell.00298.2009
Dromparis P., Paulin R., Sutendra G., Qi A. C., Bonnet S., Michelakis E. D. (2013). Uncoupling protein 2 deficiency mimics the effects of hypoxia and endoplasmic reticulum stress on mitochondria and triggers pseudohypoxic pulmonary vascular remodeling and pulmonary hypertension. Circ. Res. 113 (2), 126–136. doi:10.1161/CIRCRESAHA.112.300699
Eba S., Hoshikawa Y., Moriguchi T., Mitsuishi Y., Satoh H., Ishida K., et al. (2013). The nuclear factor erythroid 2-related factor 2 activator oltipraz attenuates chronic hypoxia-induced cardiopulmonary alterations in mice. Am. J. Respir. Cell Mol. Biol. 49 (2), 324–333. doi:10.1165/rcmb.2011-0396OC
Efstathiou S. P., Tsioulos D. I., Tsiakou A. G., Gratsias Y. E., Pefanis A. V., Mountokalakis T. D. (2005). Plasma adiponectin levels and five-year survival after first-ever ischemic stroke. Stroke 36 (9), 1915–1919. doi:10.1161/01.STR.0000177874.29849.f0
Eisele P. S., Furrer R., Beer M., Handschin C. (2015). The PGC-1 coactivators promote an anti-inflammatory environment in skeletal muscle in vivo. Biochem. Biophys. Res. Commun. 464 (3), 692–697. doi:10.1016/j.bbrc.2015.06.166
Eisele P. S., Salatino S., Sobek J., Hottiger M. O., Handschin C. (2013). The peroxisome proliferator-activated receptor γ coactivator 1α/β (PGC-1) coactivators repress the transcriptional activity of NF-κB in skeletal muscle cells. J. Biol. Chem. 288 (4), 2246–2260. doi:10.1074/jbc.M112.375253
El-Merhie N., Baumgart-Vogt E., Pilatz A., Pfreimer S., Pfeiffer B., Pak O., et al. (2017). Differential alterations of the mitochondrial morphology and respiratory chain complexes during postnatal development of the mouse lung. Oxid. Med. Cell. Longev. 2017, e9169146. doi:10.1155/2017/9169146
Erecinska M., Cherian S., Silver I. A. (2004). Energy metabolism in mammalian brain during development. Prog. Neurobiol. 73 (6), 397–445. doi:10.1016/j.pneurobio.2004.06.003
Ergenekon E., Gücüyener K. (1998). Nitric oxide in developing brain. Eur. J. Paediatr. Neurol. 2 (6), 297–301. doi:10.1016/s1090-3798(98)80004-4
Eriksson Y., Boström M., Sandelius A., Blennow K., Zetterberg H., Kuhn G., et al. (2018). The anti-asthmatic drug, montelukast, modifies the neurogenic potential in the young healthy and irradiated brain. Cell Death Dis. 9 (7), 775. doi:10.1038/s41419-018-0783-7
Estrella J., Wiley V., Simmons D., Hng T. M., McLean M. (2021). Effect of maternal metformin treatment in pregnancy on neonatal metabolism: Evidence from newborn metabolic screening. Diabetes Care 44 (11), 2536–2541. doi:10.2337/dc21-0327
Falsaperla R., Lombardo F., Filosco F., Romano C., Saporito M. A. N., Puglisi F., et al. (2020). Oxidative stress in preterm infants: Overview of current evidence and future prospects. Pharmaceuticals 13 (7), 145. doi:10.3390/ph13070145
Fatima N., Shuaib A., Saqqur M. (2019). The role of montelukast in traumatic brain injury and brain ischemia. Open Access J. Neurol. Neurosurg. 11 (1), 1–6.
Fernandez-Marcos P. J., Auwerx J. (2011). Regulation of PGC-1α, a nodal regulator of mitochondrial biogenesis. Am. J. Clin. Nutr. 93 (4), 884S–890S. doi:10.3945/ajcn.110.001917
Fink N. H., Collins C. T., Gibson R. A., Makrides M., Penttila I. A. (2016). Targeting inflammation in the preterm infant: The role of the omega-3 fatty acid docosahexaenoic acid. J. Nutr. Intermed. Metab. 5, 55–60. doi:10.1016/j.jnim.2016.03.004
Flint Beal M. (1995). Aging, energy, and oxidative stress in neurodegenerative diseases. Ann. Neurology 38, 357–366. doi:10.1002/ana.410380304
Fontecha-Barriuso M., Martín-Sánchez D., Martinez-Moreno J. M., Carrasco S., Ruiz-Andrés O., Monsalve M., et al. (2019). PGC-1α deficiency causes spontaneous kidney inflammation and increases the severity of nephrotoxic AKI. J. Pathol. 249 (1), 65–78. doi:10.1002/path.5282
Franklin J. L. (2011). Redox regulation of the intrinsic pathway in neuronal apoptosis. Antioxid. Redox Signal. 14 (8), 1437–1448. doi:10.1089/ars.2010.3596
Gagliardi L., Bellù R., Zanini R., Dammann O. (2009). Bronchopulmonary dysplasia and brain white matter damage in the preterm infant: A complex relationship. Paediatr. Perinat. Epidemiol. 23 (6), 582–590. doi:10.1111/j.1365-3016.2009.01069.x
Galea I. (2021). The blood–brain barrier in systemic infection and inflammation. Cell. Mol. Immunol. 18 (11), 2489–2501. doi:10.1038/s41423-021-00757-x
Garcia D., Carr J. F., Chan F., Peterson A. L., Ellis K. A., Scaffa A., et al. (2021). Short exposure to hyperoxia causes cultured lung epithelial cell mitochondrial dysregulation and alveolar simplification in mice. Pediatr. Res. 90 (1), 58–65. doi:10.1038/s41390-020-01224-5
Garcia P., Sood A. (2012). Adiponectin in pulmonary disease and critically ill patients. Curr. Med. Chem. 19 (32), 5493–5500. doi:10.2174/092986712803833263
Garry P. S., Ezra M., Rowland M. J., Westbrook J., Pattinson K. T. S. (2015). The role of the nitric oxide pathway in brain injury and its treatment — from bench to bedside. Exp. Neurol. 263, 235–243. doi:10.1016/j.expneurol.2014.10.017
Garthwaite J. (2008). Concepts of neural nitric oxide-mediated transmission. Eur. J. Neurosci. 27 (11), 2783–2802. doi:10.1111/j.1460-9568.2008.06285.x
Gauda E. B., Master Z. (2018). Contribution of relative leptin and adiponectin deficiencies in premature infants to chronic intermittent hypoxia: Exploring a new hypothesis. Respir. Physiol. Neurobiol. 256, 119–127. doi:10.1016/j.resp.2017.12.003
Gebb S. A., Decoux A., Waggoner A., Wilson G. L., Gillespie M. N. (2013). Mitochondrial DNA damage mediates hyperoxic dysmorphogenesis in rat fetal lung explants. Neonatology 103 (2), 91–97. doi:10.1159/000342632
Ghouleh I. A., Sahoo S., Meijles D. N., Amaral J. H., de Jesus D. S., Sembrat J., et al. (2017). Endothelial Nox1 oxidase assembly in human pulmonary arterial hypertension; driver of Gremlin1-mediated proliferation. Clin. Sci. 131 (15), 2019–2035. doi:10.1042/CS20160812
Given J. E., Loane M., Garne E., Addor M. C., Bakker M., Bertaut-Nativel B., et al. (2018). Metformin exposure in first trimester of pregnancy and risk of all or specific congenital anomalies: Exploratory case-control study. BMJ 361, k2477. doi:10.1136/bmj.k2477
Glueck C. J., GoldeNbergN. , Pranikoff J., LoftspringM. , Sieve L., Wang P. (2004). Height, weight, and motor-social development during the first 18 months of life in 126 infants born to 109 mothers with polycystic ovary syndrome who conceived on and continued metformin through pregnancy. Hum. Reprod. 19 (6), 1323–1330. doi:10.1093/humrep/deh263
Goldstein B. J., Scalia R. G., Ma X. L. (2009). Protective vascular and myocardial effects of adiponectin. Nat. Clin. Pract. Cardiovasc. Med. 6 (1), 27–35. doi:10.1038/ncpcardio1398
Gomes B. A. Q., Silva J. P. B., Romeiro C. F. R., dos Santos S. M., Rodrigues C. A., Gonçalves P. R., et al. (2018). Neuroprotective mechanisms of resveratrol in Alzheimer’s disease: Role of SIRT1. Oxid. Med. Cell. Longev. 2018, e8152373. doi:10.1155/2018/8152373
Goncharov D. A., Goncharova E. A., Tofovic S. P., Hu J., Baust J. J., Pena A. Z., et al. (2018). Metformin therapy for pulmonary hypertension associated with heart failure with preserved ejection fraction versus pulmonary arterial hypertension. Am. J. Respir. Crit. Care Med. 198 (5), 681–684. doi:10.1164/rccm.201801-0022LE
Gould J. F., Colombo J., Collins C. T., Makrides M., Hewawasam E., Smithers L. G. (2018). Assessing whether early attention of very preterm infants can be improved by an omega-3 long-chain polyunsaturated fatty acid intervention: A follow-up of a randomised controlled trial. BMJ Open 8 (5), e020043. doi:10.1136/bmjopen-2017-020043
Gozal D., Daniel J. M., Dohanich G. P. (2001). Behavioral and anatomical correlates of chronic episodic hypoxia during sleep in the rat. J. Neurosci. 21 (7), 2442–2450. doi:10.1523/jneurosci.21-07-02442.2001
Gozal E., Row B. W., Schurr A., Gozal D. (2001). Developmental differences in cortical and hippocampal vulnerability to intermittent hypoxia in the rat. Neurosci. Lett. 305 (3), 197–201. doi:10.1016/s0304-3940(01)01853-5
Gray B., Steyn F., Davies P. S. W., Vitetta L. (2013). Omega-3 fatty acids: A review of the effects on adiponectin and leptin and potential implications for obesity management. Eur. J. Clin. Nutr. 67 (12), 1234–1242. doi:10.1038/ejcn.2013.197
Greger H. K., Hanem L. G. E., Østgård H. F., Vanky E. (2020). Cognitive function in metformin exposed children, born to mothers with PCOS – follow-up of an RCT. BMC Pediatr. 20 (1), 60. doi:10.1186/s12887-020-1960-2
Grelli K. N., Keller R. L., Rogers E. E., Partridge J. C., Xu D., Barkovich A. J., et al. (2021). Bronchopulmonary dysplasia precursors influence risk of white matter injury and adverse neurodevelopmental outcome in preterm infants. Pediatr. Res. 90 (2), 359–365. doi:10.1038/s41390-020-01162-2
Grisafi D., Tassone E., Dedja A., Oselladore B., Masola V., Guzzardo V., et al. (2012). L-citrulline prevents alveolar and vascular derangement in a rat model of moderate hyperoxia-induced lung injury. Lung 190 (4), 419–430. doi:10.1007/s00408-012-9382-z
Guo C., Sun L., Chen X., Zhang D. (2013). Oxidative stress, mitochondrial damage and neurodegenerative diseases. Neural Regen. Res. 8 (21), 2003–2014. doi:10.3969/j.issn.1673-5374.2013.21.009
Guo Q., Chang B., li Yu Q., Xutong S., Yi X. J., cheng Cao S. (2020). Adiponectin treatment improves insulin resistance in mice by regulating the expression of the mitochondrial-derived peptide MOTS-c and its response to exercise via APPL1–SIRT1–PGC-1α. Diabetologia 63 (12), 2675–2688. doi:10.1007/s00125-020-05269-3
Gureev A. P., Shaforostova E. A., Popov V. N. (2019). Regulation of mitochondrial biogenesis as a way for active longevity: Interaction between the Nrf2 and PGC-1α signaling pathways. Front. Genet. 10, 435. doi:10.3389/fgene.2019.00435
Guzy R. D., Schumacker P. T. (2006). Oxygen sensing by mitochondria at complex III: The paradox of increased reactive oxygen species during hypoxia. Exp. Physiol. 91 (5), 807–819. doi:10.1113/expphysiol.2006.033506
Han B., Jiang W., Cui P., Zheng K., Dang C., Wang J., et al. (2021). Microglial PGC-1α protects against ischemic brain injury by suppressing neuroinflammation. Genome Med. 13 (1), 47. doi:10.1186/s13073-021-00863-5
Handschin C., Spiegelman B. M. (2006). Peroxisome proliferator-activated receptor γ coactivator 1 coactivators, energy homeostasis, and metabolism. Endocr. Rev. 27 (7), 728–735. doi:10.1210/er.2006-0037
Handschin C. (2009). The biology of PGC-1α and its therapeutic potential. Trends Pharmacol. Sci. 30 (6), 322–329. doi:10.1016/j.tips.2009.03.006
Hansen-Pupp I., Hellgren G., Hård A. L., Smith L., Hellström A., Löfqvist C. (2015). Early surge in circulatory adiponectin is associated with improved growth at near term in very preterm infants. J. Clin. Endocrinol. Metab. 100 (6), 2380–2387. doi:10.1210/jc.2015-1081
Hansmann G., Sallmon H., Roehr C. C., Kourembanas S., Austin E. D., Koestenberger M. (2021). Pulmonary hypertension in bronchopulmonary dysplasia. Pediatr. Res. 89 (3), 446–455. doi:10.1038/s41390-020-0993-4
Harris J. B., Chang L. Y., Crapo J. D. (1991). Rat lung alveolar type I epithelial cell injury and response to hyperoxia. Am. J. Respir. Cell Mol. Biol. 4 (2), 115–125. doi:10.1165/ajrcmb/4.2.115
Harvey A. J. (2019). Mitochondria in early development: Linking the microenvironment, metabolism and the epigenome. Reproduction 157 (5), R159–R179. doi:10.1530/REP-18-0431
Haslip M., Dostanic I., Huang Y., Zhang Y., Russell K. S., Jurczak M. J., et al. (2015). Endothelial uncoupling protein 2 regulates mitophagy and pulmonary hypertension during intermittent hypoxia. Arterioscler. Thromb. Vasc. Biol. 35 (5), 1166–1178. doi:10.1161/ATVBAHA.114.304865
Hoda U., Agarwal N. B., Vohora D., Parvez S., Raisuddin S. (2017). Resveratrol suppressed seizures by attenuating IL-1β, IL1-Ra, IL-6, and TNF-α in the hippocampus and cortex of kindled mice. Nutr. Neurosci. 20 (9), 497–504. doi:10.1080/1028415X.2016.1189057
Hon K. L. E., Leung T. F., Leung A. K. (2014). Clinical effectiveness and safety of montelukast in asthma. What are the conclusions from clinical trials and meta-analyses? Drug Des. devel. Ther. 8, 839–850. doi:10.2147/DDDT.S39100
Hong Z., Chen K. H., DasGupta A., Potus F., Dunham-Snary K., Bonnet S., et al. (2017). MicroRNA-138 and MicroRNA-25 down-regulate mitochondrial calcium uniporter, causing the pulmonary arterial hypertension cancer phenotype. Am. J. Respir. Crit. Care Med. 195 (4), 515–529. doi:10.1164/rccm.201604-0814OC
Houten B. V. (2015). Pulmonary arterial hypertension is associated with oxidative stress–induced genome instability. Am. J. Respir. Crit. Care Med. 192 (2), 129–130. doi:10.1164/rccm.201505-0904ED
Hu Y., Dong X., Chen J. (2015). Adiponectin and depression: A meta-analysis. Biomed. Rep. 3 (1), 38–42. doi:10.3892/br.2014.372
Humbert M., Guignabert C., Bonnet S., Dorfmüller P., Klinger J. R., Nicolls M. R., et al. (2019). Pathology and pathobiology of pulmonary hypertension: State of the art and research perspectives. Eur. Respir. J. 53 (1), 1801887. doi:10.1183/13993003.01887-2018
Ijäs H., Vääräsmäki M., Saarela T., Keravuo R., Raudaskoski T. (2015). A follow-up of a randomised study of metformin and insulin in gestational diabetes mellitus: Growth and development of the children at the age of 18 months. BJOG Int. J. Obstet. Gynaecol. 122 (7), 994–1000. doi:10.1111/1471-0528.12964
Ivanovska J., Kang N. Y. C., Ivanovski N., Nagy A., Belik J., Gauda E. B. (2020). Recombinant adiponectin protects the newborn rat lung from lipopolysaccharide-induced inflammatory injury. Physiol. Rep. 8 (17), e14553. doi:10.14814/phy2.14553
Iwabu M., Yamauchi T., Okada-Iwabu M., Sato K., Nakagawa T., Funata M., et al. (2010). Adiponectin and AdipoR1 regulate PGC-1alpha and mitochondria by Ca(2+) and AMPK/SIRT1. Nature 464 (7293), 1313–1319. doi:10.1038/nature08991
Iwata K., Ikami K., Matsuno K., Yamashita T., Shiba D., Ibi M., et al. (2014). Deficiency of NOX1/nicotinamide adenine dinucleotide phosphate, reduced form oxidase leads to pulmonary vascular remodeling. Arterioscler. Thromb. Vasc. Biol. 34 (1), 110–119. doi:10.1161/ATVBAHA.113.302107
Izzo A., Nitti M., Mollo N., Paladino S., Procaccini C., Faicchia D., et al. (2017). Metformin restores the mitochondrial network and reverses mitochondrial dysfunction in Down syndrome cells. Hum. Mol. Genet. 26 (6), 1056–1069. doi:10.1093/hmg/ddx016
Jakovcevski I., Filipovic R., Mo Z., Rakic S., Zecevic N. (2009). Oligodendrocyte development and the onset of myelination in the human fetal brain. Front. Neuroanat. 3, 5. doi:10.3389/neuro.05.005.2009
Jannig P. R., Dumesic P. A., Spiegelman B. M., Ruas J. L. (2022). SnapShot: Regulation and biology of PGC-1α. Cell 185 (8), 1444–1444.e1. doi:10.1016/j.cell.2022.03.027
Jensen E. A., Dysart K., Gantz M. G., McDonald S., Bamat N. A., Keszler M., et al. (2019). The diagnosis of bronchopulmonary dysplasia in very preterm infants. An evidence-based approach. Am. J. Respir. Crit. Care Med. 200 (6), 751–759. doi:10.1164/rccm.201812-2348OC
Jiang L., Zhang L., Kang K., Fei D., Gong R., Cao Y., et al. (2016). Resveratrol ameliorates LPS-induced acute lung injury via NLRP3 inflammasome modulation. Biomed. Pharmacother. Biomedecine Pharmacother. 84, 130–138. doi:10.1016/j.biopha.2016.09.020
Jimoh A., Tanko Y., Ayo J. O., Ahmed A., Mohammed A. (2018). Resveratrol increases serum adiponectin level and decreases leptin and insulin level in an experimental model of hypercholesterolemia. Pathophysiology 25 (4), 411–417. doi:10.1016/j.pathophys.2018.08.005
Joffin N., Jaubert A. M., Durant S., Barouki R., Forest C., Noirez P. (2015). Citrulline counteracts overweight- and aging-related effects on adiponectin and leptin gene expression in rat white adipose tissue. Biochim. Open 1, 1–5. doi:10.1016/j.biopen.2015.05.001
Johri A., Chandra A., Beal M. F. (2013). PGC-1α, mitochondrial dysfunction and Huntington’s disease. Free Radic. Biol. Med. 62, 37–46. doi:10.1016/j.freeradbiomed.2013.04.016
Jones A., Thornton C. (2022). Mitochondrial dynamics in the neonatal brain – A potential target following injury? Biosci. Rep. 42 (3), BSR20211696. doi:10.1042/bsr20211696
Jones C. V., Williams T. M., Walker K. A., Dickinson H., Sakkal S., Rumballe B. A., et al. (2013). M2 macrophage polarisation is associated with alveolar formation during postnatal lung development. Respir. Res. 14 (1), 41. doi:10.1186/1465-9921-14-41
Jorquera G., Echiburú B., Crisosto N., Sotomayor-Zárate R., Maliqueo M., Cruz G. (2020). Metformin during pregnancy: Effects on offspring development and metabolic function. Front. Pharmacol. 11, 653. doi:10.3389/fphar.2020.00653
Juliano C., Sosunov S., Niatsetskaya Z., Isler J. A., Utkina-Sosunova I., Jang I., et al. (2015). Mild intermittent hypoxemia in neonatal mice causes permanent neurofunctional deficit and white matter hypomyelination. Exp. Neurol. 264, 33–42. doi:10.1016/j.expneurol.2014.11.010
Kadhim H., Khalifa M., Deltenre P., Casimir G., Sebire G. (2006). Molecular mechanisms of cell death in periventricular leukomalacia. Neurology 67 (2), 293–299. doi:10.1212/01.wnl.0000224754.63593.c4
Kadlec A. O., Chabowski D. S., Ait-Aissa K., Gutterman D. D. (2016). Role of PGC-1α in vascular regulation: Implications for atherosclerosis. Arterioscler. Thromb. Vasc. Biol. 36 (8), 1467–1474. doi:10.1161/ATVBAHA.116.307123
Kamoda T., Saitoh H., Saito M., Sugiura M., Matsui A. (2004). Serum adiponectin concentrations in newborn infants in early postnatal life. Pediatr. Res. 56 (5), 690–693. doi:10.1203/01.PDR.0000142711.24999.8A
Kandasamy J., Olave N., Ballinger S. W., Ambalavanan N. (2017). Vascular endothelial mitochondrial function predicts death or pulmonary outcomes in preterm infants. Am. J. Respir. Crit. Care Med. 196 (8), 1040–1049. doi:10.1164/rccm.201702-0353OC
Kang H., Khang R., Ham S., Jeong G. R., Kim H., Jo M., et al. (2017). Activation of the ATF2/CREB-PGC-1α pathway by metformin leads to dopaminergic neuroprotection. Oncotarget 8 (30), 48603–48618. doi:10.18632/oncotarget.18122
Kaplowitz P. (2017). Is there a role for metformin in the treatment of childhood obesity? Pediatrics 140 (1), e20171205. doi:10.1542/peds.2017-1205
Kelly M. M. (2006). The basics of prematurity. J. Pediatr. Health Care 20 (4), 238–244. doi:10.1016/j.pedhc.2006.01.001
Kempuraj D., Thangavel R., Selvakumar G. P., Zaheer S., Ahmed M. E., Raikwar S. P., et al. (2017). Brain and peripheral atypical inflammatory mediators potentiate neuroinflammation and neurodegeneration. Front. Cell Neurosci. 11, 216. doi:10.3389/fncel.2017.00216
Khalaf D., Krüger M., Wehland M., Infanger M., Grimm D. (2019). The effects of oral l-arginine and l-citrulline supplementation on blood pressure. Nutrients 11 (7), 1679. doi:10.3390/nu11071679
Khemani E., McElhinney D. B., Rhein L., Andrade O., Lacro R. V., Thomas K. C., et al. (2007). Pulmonary artery hypertension in formerly premature infants with bronchopulmonary dysplasia: Clinical features and outcomes in the surfactant era. Pediatrics 120 (6), 1260–1269. doi:10.1542/peds.2007-0971
Khwaja O., Volpe J. J. (2008). Pathogenesis of cerebral white matter injury of prematurity. Arch. Dis. Child. Fetal Neonatal Ed. 93 (2), F153–F161. doi:10.1136/adc.2006.108837
Kim H. M., Song J. E., Lee S. M., Park M. S., Park K. I., Namgung R., et al. (2009). Montelukast as an add-on therapy in bronchopulmonary dysplasia. Korean J. Pediatr. 52 (2), 181–186. doi:10.3345/kjp.2009.52.2.181
Kristensen J. M., Larsen S., Helge J. W., Dela F., Wojtaszewski J. F. P. (2013). Two weeks of metformin treatment enhances mitochondrial respiration in skeletal muscle of AMPK kinase dead but not wild type mice. PLOS ONE 8 (1), e53533. doi:10.1371/journal.pone.0053533
Kuczynska Z., Metin E., Liput M., Buzanska L. (2022). Covering the role of PGC-1α in the nervous system. Cells 11 (1), 111. doi:10.3390/cells11010111
Landi S. N., Radke S., Engel S. M., Boggess K., Stürmer T., Howe A. S., et al. (2019). Association of long-term child growth and developmental outcomes with metformin vs insulin treatment for gestational diabetes. JAMA Pediatr. 173 (2), 160–168. doi:10.1001/jamapediatrics.2018.4214
Lapillonne A., Moltu S. J. (2016). Long-chain polyunsaturated fatty acids and clinical outcomes of preterm infants. Ann. Nutr. Metab. 69 (1), 35–44. doi:10.1159/000448265
Larson-Casey J. L., He C., Carter A. B. (2020). Mitochondrial quality control in pulmonary fibrosis. Redox Biol. 33, 101426. doi:10.1016/j.redox.2020.101426
Lauterbach R., Pawlik D., Lauterbach J. P. (2018). L-Citrulline supplementation in the treatment of pulmonary hypertension associated with bronchopulmonary dysplasia in preterm infant: A case report. SAGE Open Med. Case Rep. 6, 2050313X18778730. doi:10.1177/2050313X18778730
Lavie L. (2003). Obstructive sleep apnoea syndrome--an oxidative stress disorder. Sleep. Med. Rev. 7 (1), 35–51. doi:10.1053/smrv.2002.0261
Lecarpentier Y., Gourrier E., Gobert V., Vallée A. (2019). Bronchopulmonary dysplasia: Crosstalk between PPARγ, WNT/β-Catenin and TGF-β pathways; the potential therapeutic role of PPARγ agonists. Front. Pediatr. 7, 176. doi:10.3389/fped.2019.00176
Lee J., Hamanaka G., Lo E. H., Arai K. (2019). Heterogeneity of microglia and their differential roles in white matter pathology. CNS Neurosci. Ther. 25 (12), 1290–1298. doi:10.1111/cns.13266
Lee J. W., Davis J. M. (2011). Future applications of antioxidants in premature infants. Curr. Opin. Pediatr. 23 (2), 161–166. doi:10.1097/MOP.0b013e3283423e51
Lee Y. A. (2017). White matter injury of prematurity: Its mechanisms and clinical features. J. Pathol. Transl. Med. 51 (5), 449–455. doi:10.4132/jptm.2017.07.25
Lembo C., Buonocore G., Perrone S. (2021). Oxidative stress in preterm newborns. Antioxidants 10 (11), 1672. doi:10.3390/antiox10111672
Leone T. C., Lehman J. J., Finck B. N., Schaeffer P. J., Wende A. R., Boudina S., et al. (2005). PGC-1alpha deficiency causes multi-system energy metabolic derangements: Muscle dysfunction, abnormal weight control and hepatic steatosis. PLoS Biol. 3 (4), e101. doi:10.1371/journal.pbio.0030101
Li C., Meng F., Garza J. C., Liu J., Lei Y., Kirov S. A., et al. (2021). Modulation of depression-related behaviors by adiponectin AdipoR1 receptors in 5-HT neurons. Mol. Psychiatry 26 (8), 4205–4220. doi:10.1038/s41380-020-0649-0
Li J., Dai A., Hu R., Zhu L., Tan S. (2010). Positive correlation between PPARgamma/PGC-1alpha and gamma-GCS in lungs of rats and patients with chronic obstructive pulmonary disease. Acta Biochim. Biophys. Sin. 42 (9), 603–614. doi:10.1093/abbs/gmq071
Li P. A., Hou X., Hao S. (2017). Mitochondrial biogenesis in neurodegeneration. J. Neurosci. Res. 95 (10), 2025–2029. doi:10.1002/jnr.24042
Liang H., Ward W. F. (2006). PGC-1alpha: A key regulator of energy metabolism. Adv. Physiol. Educ. 30 (4), 145–151. doi:10.1152/advan.00052.2006
Lin J., Wu P. H., Tarr P. T., Lindenberg K. S., St-Pierre J., Zhang C. Y., et al. (2004). Defects in adaptive energy metabolism with CNS-linked hyperactivity in PGC-1alpha null mice. Cell 119 (1), 121–135. doi:10.1016/j.cell.2004.09.013
Lingappan K., Savani R. C. (2020). The Wnt signaling pathway and the development of bronchopulmonary dysplasia. Am. J. Respir. Crit. Care Med. 201 (10), 1174–1176. doi:10.1164/rccm.202002-0277ED
Lira V. A., Brown D. L., Lira A. K., Kavazis A. N., Soltow Q. A., Zeanah E. H., et al. (2010). Nitric oxide and AMPK cooperatively regulate PGC-1 in skeletal muscle cells. J. Physiol. 588 (18), 3551–3566. doi:10.1113/jphysiol.2010.194035
Liu P. L., Chong I. W., Lee Y. C., Tsai J. R., Wang H. M., Hsieh C. C., et al. (2015). Anti-inflammatory effects of resveratrol on hypoxia/reoxygenation-induced alveolar epithelial cell dysfunction. J. Agric. Food Chem. 63 (43), 9480–9487. doi:10.1021/acs.jafc.5b01168
Liu S. H., Chiu C. Y., Wang L. P., Chiang M. T. (2019). Omega-3 fatty acids-enriched fish oil activates AMPK/PGC-1α signaling and prevents obesity-related skeletal muscle wasting. Mar. Drugs 17 (6), 380. doi:10.3390/md17060380
Liu Y., Xu Y., Zhu J., Li H., Zhang J., Yang G., et al. (2019). Metformin prevents progression of experimental pulmonary hypertension via inhibition of autophagy and activation of adenosine monophosphate-activated protein kinase. J. Vasc. Res. 56 (3), 117–128. doi:10.1159/000498894
Lucas E. K., Reid C. S., McMeekin L. J., Dougherty S. E., Floyd C. L., Cowell R. M. (2015). Cerebellar transcriptional alterations with Purkinje cell dysfunction and loss in mice lacking PGC-1α. Front. Cell Neurosci. 8, 441. doi:10.3389/fncel.2014.00441
Ma L., Zhao Y., Wang R., Chen T., Li W., Nan Y., et al. (2015). 3, 5, 4′-tri-O-acetylresveratrol attenuates lipopolysaccharide-induced acute respiratory distress syndrome via MAPK/SIRT1 pathway. Mediat. Inflamm. 2015, 143074. doi:10.1155/2015/143074
Ma L., Zhou P., Neu J., Lin H. C. (2017). Potential nutrients for preventing or treating bronchopulmonary dysplasia. Paediatr. Respir. Rev. 22, 83–88. doi:10.1016/j.prrv.2016.08.013
Mailloux R. J. (2018). Mitochondrial antioxidants and the maintenance of cellular hydrogen peroxide levels. Oxid. Med. Cell. Longev. 2018, e7857251. doi:10.1155/2018/7857251
Manley B. J., Makrides M., Collins C. T., McPhee A. J., Gibson R. A., Ryan P., et al. (2011). High-dose docosahexaenoic acid supplementation of preterm infants: Respiratory and allergy outcomes. Pediatrics 128 (1), e71–e77. doi:10.1542/peds.2010-2405
Marshall J. D., Bazan I., Zhang Y., Fares W. H., Lee P. J. (2018). Mitochondrial dysfunction and pulmonary hypertension: Cause, effect, or both. Am. J. Physiol. Lung Cell. Mol. Physiol. 314 (5), L782–L796. doi:10.1152/ajplung.00331.2017
Martin C. R., Dasilva D. A., Cluette-Brown J. E., Dimonda C., Hamill A., Bhutta A. Q., et al. (2011). Decreased postnatal docosahexaenoic and arachidonic acid blood levels in premature infants are associated with neonatal morbidities. J. Pediatr. 159 (5), 743–749. e1-2. doi:10.1016/j.jpeds.2011.04.039
Martínez-Redondo V., Pettersson A. T., Ruas J. L. (2015). The hitchhiker’s guide to PGC-1α isoform structure and biological functions. Diabetologia 58 (9), 1969–1977. doi:10.1007/s00125-015-3671-z
Martinho S., Adão R., Leite-Moreira A. F., Brás-Silva C. (2020). Persistent pulmonary hypertension of the newborn: Pathophysiological mechanisms and novel therapeutic approaches. Front. Pediatr. 8, 342. doi:10.3389/fped.2020.00342
Massaro G. D., Gail D. B., Massaro D. (1975). Lung oxygen consumption and mitochondria of alveolar epithelial and endothelial cells. J. Appl. Physiol. 38 (4), 588–592. doi:10.1152/jappl.1975.38.4.588
Mata M., Sarrion I., Milian L., Juan G., Ramon M., Naufal D., et al. (2012). PGC-1α induction in pulmonary arterial hypertension. Oxid. Med. Cell. Longev. 2012, e236572. doi:10.1155/2012/236572
MatÉs J. M., Pérez-Gómez C., De Castro I. N. (1999). Antioxidant enzymes and human diseases. Clin. Biochem. 32 (8), 595–603. doi:10.1016/s0009-9120(99)00075-2
McElroy M. C., Kasper M. (2004). The use of alveolar epithelial type I cell-selective markers to investigate lung injury and repair. Eur. Respir. J. 24 (4), 664–673. doi:10.1183/09031936.04.00096003
McMeekin L. J., Fox S. N., Boas S. M., Cowell R. M. (2021). Dysregulation of PGC-1α-dependent transcriptional programs in neurological and developmental disorders: Therapeutic challenges and opportunities. Cells 10 (2), 352. doi:10.3390/cells10020352
McNamara N. B., Miron V. E. (2020). Microglia in developing white matter and perinatal brain injury. Neurosci. Lett. 714, 134539. doi:10.1016/j.neulet.2019.134539
Miao D., Scutt A. (2002). Histochemical localization of alkaline phosphatase activity in decalcified bone and cartilage. J. Histochem. Cytochem. 50 (3), 333–340. doi:10.1177/002215540205000305
Miller K. N., Clark J. P., Anderson R. M. (2019). Mitochondrial regulator PGC-1a—modulating the modulator. Curr. Opin. Endocr. Metab. Res. 5, 37–44. doi:10.1016/j.coemr.2019.02.002
Mittal M., Siddiqui M. R., Tran K., Reddy S. P., Malik A. B. (2014). Reactive oxygen species in inflammation and tissue injury. Antioxid. Redox Signal. 20 (7), 1126–1167. doi:10.1089/ars.2012.5149
Montgomery A. M., Bazzy-Asaad A., Asnes J. D., Bizzarro M. J., Ehrenkranz R. A., Weismann C. G. (2016). Biochemical screening for pulmonary hypertension in preterm infants with bronchopulmonary dysplasia. Neonatology 109 (3), 190–194. doi:10.1159/000442043
Morató L., Bertini E., Verrigni D., Ardissone A., Ruiz M., Ferrer I., et al. (2014). Mitochondrial dysfunction in central nervous system white matter disorders. Glia 62 (11), 1878–1894. doi:10.1002/glia.22670
Mota B. C., Sastre M. (2021). The role of PGC1α in Alzheimer’s disease and therapeutic interventions. Int. J. Mol. Sci. 22 (11), 5769. doi:10.3390/ijms22115769
Moura D. M. S., Brennan E. J., Brock R., Cocas L. A. (2022). Neuron to oligodendrocyte precursor cell synapses: Protagonists in oligodendrocyte development and myelination, and targets for therapeutics. Front. Neurosci. 15, 779125. doi:10.3389/fnins.2021.779125
Mourani P. M., Sontag M. K., Younoszai A., Miller J. I., Kinsella J. P., Baker C. D., et al. (2015). Early pulmonary vascular disease in preterm infants at risk for bronchopulmonary dysplasia. Am. J. Respir. Crit. Care Med. 191 (1), 87–95. doi:10.1164/rccm.201409-1594oc
Mulkareddy V., Simon M. A. (2020). Metformin in pulmonary hypertension in left heart disease. Front. Med. 7, 425. doi:10.3389/fmed.2020.00425
Na K. S., Kim E. K., Park J. T. (2017). Decreased plasma adiponectin among male firefighters with symptoms of post-traumatic stress disorder. J. Affect. Disord. 221, 254–258. doi:10.1016/j.jad.2017.06.015
Nakahira K., Hisata S., Choi A. M. K. (2015). The roles of mitochondrial damage-associated molecular patterns in diseases. Antioxid. Redox Signal. 23 (17), 1329–1350. doi:10.1089/ars.2015.6407
Napolitano G., Fasciolo G., Venditti P. (2021). Mitochondrial management of reactive oxygen species. Antioxidants 10 (11), 1824. doi:10.3390/antiox10111824
Nesterova A. P., Klimov E. A., Zharkova M., Sozin S., Sobolev V., Ivanikova N. V., et al. (2020). “Chapter 8 - diseases of the circulatory system,” in Disease pathways. Editors A. P. Nesterova, E. A. Klimov, M. Zharkova, S. Sozin, V. Sobolev, N. V. Ivanikovaet al. (Elsevier), 327–390.
Neubauer J. A. (2001). Invited Review: Physiological and pathophysiological responses to intermittent hypoxia. J. Appl. Physiol. 90 (4), 1593–1599. doi:10.1152/jappl.2001.90.4.1593
Newberry S. J., Chung M., Booth M., Maglione M. A., Tang A. M., O’Hanlon C. E., et al. (2016). Omega-3 fatty acids and maternal and child health: An updated systematic review. Evid. Rep. Technol. Assess. 2016 (224), 1–826. doi:10.23970/AHRQEPCERTA224
Nguyen L., Chan S. Y., Teo A. K. K. (2018). Metformin from mother to unborn child – are there unwarranted effects? EBioMedicine 35, 394–404. doi:10.1016/j.ebiom.2018.08.047
Niizuma K., Yoshioka H., Chen H., Kim G. S., Jung J. E., Katsu M., et al. (2010). Mitochondrial and apoptotic neuronal death signaling pathways in cerebral ischemia. Biochim. Biophys. Acta 1802 (1), 92–99. doi:10.1016/j.bbadis.2009.09.002
Nijland P. G., Witte M. E., van het Hof B., van der Pol S., Bauer J., Lassmann H., et al. (2014). Astroglial PGC-1alpha increases mitochondrial antioxidant capacity and suppresses inflammation: Implications for multiple sclerosis. Acta Neuropathol. Commun. 2 (1), 170. doi:10.1186/s40478-014-0170-2
Oberthuer A., Dönmez F., Oberhäuser F., Hahn M., Hoppenz M., Hoehn T., et al. (2012). Hypoadiponectinemia in extremely low gestational age newborns with severe hyperglycemia – a matched-paired analysis. PLoS ONE 7 (6), e38481. doi:10.1371/journal.pone.0038481
O’Donovan D. J., Fernandes C. J. (2000). Mitochondrial glutathione and oxidative stress: Implications for pulmonary oxygen toxicity in premature infants. Mol. Genet. Metab. 71 (1–2), 352–358. doi:10.1006/mgme.2000.3063
Oh T. I., Lee M., Lee Y. M., Kim G. H., Lee D., You J. S., et al. (2021). PGC1α loss promotes lung cancer metastasis through epithelial-mesenchymal transition. Cancers 13 (8), 1772. doi:10.3390/cancers13081772
Okada-Iwabu M., Yamauchi T., Iwabu M., Honma T., Hamagami K. I., Matsuda K., et al. (2013). A small-molecule AdipoR agonist for type 2 diabetes and short life in obesity. Nature 503 (7477), 493–499. doi:10.1038/nature12656
Özdemir Ö. M. A., Gözkeser E., Bir F., Yenisey Ç. (2014). The effects of resveratrol on hyperoxia-induced lung injury in neonatal rats. Pediatr. Neonatol. 55 (5), 352–357. doi:10.1016/j.pedneo.2013.11.004
Ozsurekci Y., Aykac K. (2016). Oxidative stress related diseases in newborns. Oxid. Med. Cell. Longev. 2016, e2768365. doi:10.1155/2016/2768365
Pak O., Sommer N., Hoeres T., Bakr A., Waisbrod S., Sydykov A., et al. (2013). Mitochondrial hyperpolarization in pulmonary vascular remodeling. Mitochondrial uncoupling protein deficiency as disease model. Am. J. Respir. Cell Mol. Biol. 49 (3), 358–367. doi:10.1165/rcmb.2012-0361OC
Palomer X., Álvarez-Guardia D., Rodríguez-Calvo R., Coll T., Laguna J. C., Davidson M. M., et al. (2009). TNF-alpha reduces PGC-1alpha expression through NF-kappaB and p38 MAPK leading to increased glucose oxidation in a human cardiac cell model. Cardiovasc. Res. 81 (4), 703–712. doi:10.1093/cvr/cvn327
Paul V., Ekambaram P. (2011). Involvement of nitric oxide in learning & memory processes. Indian J. Med. Res. 133 (5), 471–478.
Piccinin E., Sardanelli A. M., Seibel P., Moschetta A., Cocco T., Villani G. (2021). PGC-1s in the spotlight with Parkinson’s disease. Int. J. Mol. Sci. 22 (7), 3487. doi:10.3390/ijms22073487
Polito R., Di Meo I., Barbieri M., Daniele A., Paolisso G., Rizzo M. R. (2020). Adiponectin role in neurodegenerative diseases: Focus on nutrition review. Int. J. Mol. Sci. 21 (23), 9255. doi:10.3390/ijms21239255
Prabhakar N. R., Peng Y. J., Yuan G., Kumar G. K. (2006). Reactive oxygen species facilitate oxygen sensing. Novartis Found. Symp. 272, 95–99.
Quan H., Yu T., Lin Y., Pan J., Mao B., Wang X., et al. (2022). Adiponectin levels are associated with white matter lesions (WMLs) and cognitive impairment. Biomed. Res. Int. 2022, 9943250. doi:10.1155/2022/9943250
Raffay T. M., Martin R. J., Reynolds J. D. (2012). Can nitric oxide based therapy prevent bronchopulmonary dysplasia? Clin. Perinatol. 39 (3), 613–638. doi:10.1016/j.clp.2012.06.011
Rafikov R., Sun X., Rafikova O., Louise Meadows M., Desai A. A., Khalpey Z., et al. (2015). Complex I dysfunction underlies the glycolytic switch in pulmonary hypertensive smooth muscle cells. Redox Biol. 6, 278–286. doi:10.1016/j.redox.2015.07.016
Rafikova O., Srivastava A., Desai A. A., Rafikov R., Tofovic S. P. (2018). Recurrent inhibition of mitochondrial complex III induces chronic pulmonary vasoconstriction and glycolytic switch in the rat lung. Respir. Res. 19 (1), 69. doi:10.1186/s12931-018-0776-1
Raman V., Foster C. M. (2021). Metformin treatment of pediatric obesity. Pediatrics 147 (3), e2020044982. doi:10.1542/peds.2020-044982
Rangarajan S., Bernard K., Thannickal V. J. (2017). Mitochondrial dysfunction in pulmonary fibrosis. Ann. Am. Thorac. Soc. 14 (5), S383–S388. doi:10.1513/AnnalsATS.201705-370AW
Rao J., Li J., Liu Y., Lu P., Sun X., Sugumaran P. K., et al. (2012). The key role of PGC-1α in mitochondrial biogenesis and the proliferation of pulmonary artery vascular smooth muscle cells at an early stage of hypoxic exposure. Mol. Cell. Biochem. 367 (1), 9–18. doi:10.1007/s11010-012-1313-z
Rashid J., Kumar S. S., Job K. M., Liu X., Fike C. D., Sherwin C. M. (2020). Therapeutic potential of citrulline as an arginine supplement: A clinical pharmacology review. Paediatr. Drugs 22 (3), 279–293. doi:10.1007/s40272-020-00384-5
Ratner V., Slinko S., Utkina-Sosunova I., Starkov A., Polin R. A., Ten V. S. (2009). Hypoxic stress exacerbates hyperoxia-induced lung injury in a neonatal mouse model of bronchopulmonary dysplasia. Neonatology 95 (4), 299–305. doi:10.1159/000178798
Ratner V., Sosunov S. A., Niatsetskaya Z. V., Utkina-Sosunova I. V., Ten V. S. (2013). Mechanical ventilation causes pulmonary mitochondrial dysfunction and delayed alveolarization in neonatal mice. Am. J. Respir. Cell Mol. Biol. 49 (6), 943–950. doi:10.1165/rcmb.2012-0172OC
Rebelo A. P., Dillon L. M., Moraes C. T. (2011). Mitochondrial DNA transcription regulation and nucleoid organization. J. Inherit. Metab. Dis. 34 (4), 941–951. doi:10.1007/s10545-011-9330-8
Rehan V. K., Torday J. S. (2012). PPARγ signaling mediates the evolution, development, homeostasis, and repair of the lung. PPAR Res. 2012, 289867. doi:10.1155/2012/289867
Rehan V. K., Wang Y., Patel S., Santos J., Torday J. S. (2006). Rosiglitazone, a peroxisome proliferator-activated receptor-gamma agonist, prevents hyperoxia-induced neonatal rat lung injury in vivo. Pediatr. Pulmonol. 41 (6), 558–569. doi:10.1002/ppul.20407
Remels A. H. V., Gosker H. R., Bakker J., Guttridge D. C., Schols A. M. W. J., Langen R. C. J. (2013). Regulation of skeletal muscle oxidative phenotype by classical NF-κB signalling. Biochim. Biophys. Acta 1832 (8), 1313–1325. doi:10.1016/j.bbadis.2013.03.018
Ricciardolo F. L. M. (2003). Multiple roles of nitric oxide in the airways. Thorax 58 (2), 175–182. doi:10.1136/thorax.58.2.175
Riccio A., Alvania R. S., Lonze B. E., Ramanan N., Kim T., Huang Y., et al. (2006). A nitric oxide signaling pathway controls CREB-mediated gene expression in neurons. Mol. Cell 21 (2), 283–294. doi:10.1016/j.molcel.2005.12.006
Rius-Pérez S., Torres-Cuevas I., Millán I., Ortega Á. L., Pérez S. (2020). PGC-1α, inflammation, and oxidative stress: An integrative view in metabolism. Oxidative Med. Cell. Longev. 2020, e1452696. doi:10.1155/2020/1452696
Roberts K., Stepanovich G., Bhatt-Mehta V., Donn S. M. (2021). p New pharmacologic approaches to bronchopulmonary dysplasia p>. J. Exp. Pharmacol. 13, 377–396.
Rodríguez-Cano A. M., Calzada-Mendoza C. C., Estrada-Gutierrez G., Mendoza-Ortega J. A., Nutrients Perichart-Perera O. (2020). Nutrients, mitochondrial function, and perinatal health. Nutrients 12 (7), 21666–E2219. doi:10.3390/nu12072166
Roper J. M., Mazzatti D. J., Watkins R. H., Maniscalco W. M., Keng P. C., O’Reilly M. A. (2004). In vivo exposure to hyperoxia induces DNA damage in a population of alveolar type II epithelial cells. Am. J. Physiol. Lung Cell. Mol. Physiol. 286 (5), L1045–L1054. doi:10.1152/ajplung.00376.2003
Rotermund C., Machetanz G., Fitzgerald J. C. (2018). The therapeutic potential of metformin in neurodegenerative diseases. Front. Endocrinol. 9, 400. doi:10.3389/fendo.2018.00400
Row B. W., Liu R., Xu W., Kheirandish L., Gozal D. (2003). Intermittent hypoxia is associated with oxidative stress and spatial learning deficits in the rat. Am. J. Respir. Crit. Care Med. 167 (11), 1548–1553. doi:10.1164/rccm.200209-1050OC
Ruchko M., Gorodnya O., LeDoux S. P., Alexeyev M. F., Al-Mehdi A. B., Gillespie M. N. (2005). Mitochondrial DNA damage triggers mitochondrial dysfunction and apoptosis in oxidant-challenged lung endothelial cells. Am. J. Physiol. Lung Cell. Mol. Physiol. 288 (3), L530–L535. doi:10.1152/ajplung.00255.2004
Ruddy R. M., Adams K. V., Morshead C. M. (2019). Age- and sex-dependent effects of metformin on neural precursor cells and cognitive recovery in a model of neonatal stroke. Sci. Adv. 5 (9), eaax1912. doi:10.1126/sciadv.aax1912
Rupprecht T., Rupprecht C., Harms D., Sterlacci W., Vieth M., Seybold K. (2014). Leukotriene receptor blockade as a life-saving treatment in severe bronchopulmonary dysplasia. Respiration. 88 (4), 285–290. doi:10.1159/000365488
Ryan J., Dasgupta A., Huston J., Chen K. H., Archer S. L. (2015). Mitochondrial dynamics in pulmonary arterial hypertension. J. Mol. Med. 93 (3), 229–242. doi:10.1007/s00109-015-1263-5
Sahni M., Mowes A. K. (2022). “Bronchopulmonary dysplasia,” in StatPearls (Treasure Island (FL): StatPearls Publishing).
Saito M., Nishimura K., Nozue H., Miyazono Y., Kamoda T. (2011). Changes in serum adiponectin levels from birth to term-equivalent age are associated with postnatal weight gain in preterm infants. Neonatology 100 (1), 93–98. doi:10.1159/000322654
Saito T., Ichikawa T., Numakura T., Yamada M., Koarai A., Fujino N., et al. (2021). PGC-1α regulates airway epithelial barrier dysfunction induced by house dust mite. Respir. Res. 22 (1), 63. doi:10.1186/s12931-021-01663-6
Samuel T. M., Zhou Q., Giuffrida F., Munblit D., Verhasselt V., Thakkar S. K. (2020). Nutritional and non-nutritional composition of human milk is modulated by maternal, infant, and methodological factors. Front. Nutr. 7, 576133. doi:10.3389/fnut.2020.576133
Sankowski R., Mader S., Valdés-Ferrer S. I. (2015). Systemic inflammation and the brain: Novel roles of genetic, molecular, and environmental cues as drivers of neurodegeneration. Front. Cell. Neurosci. 9, 28. doi:10.3389/fncel.2015.00028
Schmidt S. F., Mandrup S. (2011). Gene program-specific regulation of PGC-1{alpha} activity. Genes Dev. 25 (14), 1453–1458. doi:10.1101/gad.2076411
Schon E. A., Manfredi G. (2003). Neuronal degeneration and mitochondrial dysfunction. J. Clin. Invest. 111 (3), 303–312. doi:10.1172/JCI17741
Schulz R., Mahmoudi S., Hattar K., Sibelius U., Olschewski H., Mayer K., et al. (2000). Enhanced release of superoxide from polymorphonuclear neutrophils in obstructive sleep apnea. Impact of continuous positive airway pressure therapy. Am. J. Respir. Crit. Care Med. 162 (2), 566–570. doi:10.1164/ajrccm.162.2.9908091
Scott J. A., Maarsingh H., Holguin F., Grasemann H. (2021). Arginine therapy for lung diseases. Front. Pharmacol. 12, 627503. doi:10.3389/fphar.2021.627503
Shah D., Das P., Bhandari V. (2018). Mitochondrial dysfunction in bronchopulmonary dysplasia. Am. J. Respir. Crit. Care Med. 197 (10), 1363. doi:10.1164/rccm.201711-2197LE
Shah D., Sandhu K., Das P., Bhandari V. (2022). Adiponectin ameliorates hyperoxia-induced lung endothelial dysfunction and promotes angiogenesis in neonatal mice. Pediatr. Res. 91 (3), 545–555. doi:10.1038/s41390-021-01442-5
Shah D. K., Doyle L. W., Anderson P. J., Bear M., Daley A. J., Hunt R. W., et al. (2008). Adverse neurodevelopment in preterm infants with postnatal sepsis or necrotizing enterocolitis is mediated by white matter abnormalities on magnetic resonance imaging at term. J. Pediatr. 153 (2), 170175–175e1. doi:10.1016/j.jpeds.2008.02.033
Shaito A., Posadino A. M., Younes N., Hasan H., Halabi S., Alhababi D., et al. (2020). Potential adverse effects of resveratrol: A literature review. Int. J. Mol. Sci. 21 (6), 2084. doi:10.3390/ijms21062084
Shan X., Chi L., Ke Y., Luo C., Qian S., Gozal D., et al. (2007). Manganese superoxide dismutase protects mouse cortical neurons from chronic intermittent hypoxia-mediated oxidative damage. Neurobiol. Dis. 28 (2), 206–215. doi:10.1016/j.nbd.2007.07.013
Shoag J., Arany Z. (2010). Regulation of hypoxia-inducible genes by PGC-1 alpha. Arterioscler. Thromb. Vasc. Biol. 30 (4), 662–666. doi:10.1161/ATVBAHA.108.181636
Siahanidou T., Mandyla H., Papassotiriou G., Papassotiriou I., Chrousos G. (2007). Circulating levels of adiponectin in preterm infants. Arch. Dis. Child. Fetal Neonatal Ed. 92 (4), F286–F290. doi:10.1136/adc.2006.106112
Siekacz K., Piotrowski W. J., Iwański M. A., Górski P., Białas A. J. (2021). The role of interaction between mitochondria and the extracellular matrix in the development of idiopathic pulmonary fibrosis. Oxid. Med. Cell. Longev. 2021, e9932442. doi:10.1155/2021/9932442
Simon D. M., Arikan M. C., Srisuma S., Bhattacharya S., Andalcio T., Shapiro S. D., et al. (2006). Epithelial cell PPARgamma is an endogenous regulator of normal lung maturation and maintenance. Proc. Am. Thorac. Soc. 3 (6), 510–511. doi:10.1513/pats.200603-034MS
Simon D. M., Arikan M. C., Srisuma S., Bhattacharya S., Tsai L. W., Ingenito E. P., et al. (2006). Epithelial cell PPAR[gamma] contributes to normal lung maturation. FASEB J. Off. Publ. Fed. Am. Soc. Exp. Biol. 20 (9), 1507–1509. doi:10.1096/fj.05-5410fje
Soliman A., De Sanctis V., Alaaraj N., Hamed N. (2020). The clinical application of metformin in children and adolescents: A short update. Acta Biomed. 91 (3), e2020086. doi:10.23750/abm.v91i3.10127
Son S. H., Lee S. M., Lee M. H., Son Y. K., Kim S. E., An W. S. (2021). Omega-3 fatty acids upregulate SIRT1/3, activate PGC-1α via deacetylation, and induce Nrf1 production in 5/6 nephrectomy rat model. Mar. Drugs 19 (4), 182. doi:10.3390/md19040182
Srivillibhuthur M., Warder B. N., Toke N. H., Shah P. P., Feng Q., Gao N., et al. (2018). TFAM is required for maturation of the fetal and adult intestinal epithelium. Dev. Biol. 439 (2), 92–101. doi:10.1016/j.ydbio.2018.04.015
Stiles J., Jernigan T. L. (2010). The basics of brain development. Neuropsychol. Rev. 20 (4), 327–348. doi:10.1007/s11065-010-9148-4
Su L. J., Zhang J. H., Gomez H., Murugan R., Hong X., Xu D., et al. (2019). Reactive oxygen species-induced lipid peroxidation in apoptosis, autophagy, and ferroptosis. Oxid. Med. Cell. Longev. 2019, e5080843. doi:10.1155/2019/5080843
Su Y., Ke C., Li C., Huang C., Wan C. (2022). Intermittent hypoxia promotes the recovery of motor function in rats with cerebral ischemia by regulating mitochondrial function. Exp. Biol. Med. 247, 1364–1378. doi:10.1177/15353702221098962
Suliman H. B., Nozik-Grayck E. (2019). Mitochondrial dysfunction: Metabolic drivers of pulmonary hypertension. Antioxid. Redox Signal. 31 (12), 843–857. doi:10.1089/ars.2018.7705
Suwa M., Egashira T., Nakano H., Sasaki H., Kumagai S. (2006). Metformin increases the PGC-1alpha protein and oxidative enzyme activities possibly via AMPK phosphorylation in skeletal muscle in vivo. J. Appl. Physiol. 101 (6), 1685–1692. doi:10.1152/japplphysiol.00255.2006
Sweeney G., Song J. (2016). The association between PGC-1α and Alzheimer’s disease. Anat. Cell Biol. 49 (1), 1–6. doi:10.5115/acb.2016.49.1.1
Tarry-Adkins J. L., Aiken C. E., Ozanne S. E. (2019). Neonatal, infant, and childhood growth following metformin versus insulin treatment for gestational diabetes: A systematic review and meta-analysis. PLoS Med. 16 (8), e1002848. doi:10.1371/journal.pmed.1002848
Tellone E., Galtieri A., Russo A., Giardina B., Ficarra S. (2015). Resveratrol: A focus on several neurodegenerative diseases. Oxid. Med. Cell. Longev. 2015, e392169. doi:10.1155/2015/392169
Ten V. S. (2017). Mitochondrial dysfunction in alveolar and white matter developmental failure in premature infants. Pediatr. Res. 81 (2), 286–292. doi:10.1038/pr.2016.216
Tesfaye B. A., Hailu H. G., Zewdie K. A., Ayza M. A., Berhe D. F. (2021). Montelukast: The new therapeutic option for the treatment of epilepsy. J. Exp. Pharmacol. 13, 23–31. doi:10.2147/jep.s277720
Thau N., Knippenberg S., Körner S., Rath K. J., Dengler R., Petri S. (2012). Decreased mRNA expression of PGC-1α and PGC-1α-regulated factors in the SOD1G93A ALS mouse model and in human sporadic ALS. J. Neuropathol. Exp. Neurol. 71 (12), 1064–1074. doi:10.1097/NEN.0b013e318275df4b
Thébaud B., Goss K. N., Laughon M., Whitsett J. A., Abman S. H., Steinhorn R. H., et al. (2019). Bronchopulmonary dysplasia. Nat. Rev. Dis. Prim. 5 (1), 78. doi:10.1038/s41572-019-0127-7
Thornton C., Jones A., Nair S., Aabdien A., Mallard C., Hagberg H. (2018). Mitochondrial dynamics, mitophagy and biogenesis in neonatal hypoxic-ischaemic brain injury. FEBS Lett. 592 (5), 812–830. doi:10.1002/1873-3468.12943
Thundyil J., Pavlovski D., Sobey C. G., Arumugam T. V. (2012). Adiponectin receptor signalling in the brain. Br. J. Pharmacol. 165 (2), 313–327. doi:10.1111/j.1476-5381.2011.01560.x
Torday J. S., Torres E., Rehan V. K. (2003). The role of fibroblast transdifferentiation in lung epithelial cell proliferation, differentiation, and repair in vitro. Pediatr. Pathol. Mol. Med. 22 (3), 189–207. doi:10.1080/pdp.22.3.189.207
Trenker M., Malli R., Fertschai I., Levak-Frank S., Graier W. F. (2007). Uncoupling proteins 2 and 3 are fundamental for mitochondrial Ca2+ uniport. Nat. Cell Biol. 9 (4), 445–452. doi:10.1038/ncb1556
Tsunemi T., Ashe T. D., Morrison B. E., Soriano K. R., Au J., Roque R. A. V., et al. (2012). PGC-1α rescues Huntington’s disease proteotoxicity by preventing oxidative stress and promoting TFEB function. Sci. Transl. Med. 4 (142), 142ra97. doi:10.1126/scitranslmed.3003799
Tuzun F., Kumral A., Dilek M., Ozbal S., Ergur B., Yesilirmak D. C., et al. (2012). Maternal omega-3 fatty acid supplementation protects against lipopolysaccharide-induced white matter injury in the neonatal rat brain. J. Matern. Fetal. Neonatal Med. 25 (6), 849–854. doi:10.3109/14767058.2011.587917
Vadivel A., Aschner J. L., Rey-Parra G. J., Magarik J., Zeng H., Summar M., et al. (2010). L-Citrulline attenuates arrested alveolar growth and pulmonary hypertension in oxygen-induced lung injury in newborn rats. Pediatr. Res. 68 (6), 519–525. doi:10.1203/PDR.0b013e3181f90278
Valencia A. M., Abrantes M. A., Hasan J., Aranda J. V., Beharry K. D. (2018). Reactive oxygen species, biomarkers of microvascular maturation and alveolarization, and antioxidants in oxidative lung injury. React. Oxyg. Species 6 (18), 373–388. doi:10.20455/ros.2018.867
Valle I., Álvarez-Barrientos A., Arza E., Lamas S., Monsalve M. (2005). PGC-1alpha regulates the mitochondrial antioxidant defense system in vascular endothelial cells. Cardiovasc. Res. 66 (3), 562–573. doi:10.1016/j.cardiores.2005.01.026
Varatharaj A., Galea I. (2017). The blood-brain barrier in systemic inflammation. Brain Behav. Immun. 60, 1–12. doi:10.1016/j.bbi.2016.03.010
Vaughan R. A., Garcia-Smith R., Bisoffi M., Conn C. A., Trujillo K. A. (2012). Conjugated linoleic acid or omega 3 fatty acids increase mitochondrial biosynthesis and metabolism in skeletal muscle cells. Lipids Health Dis. 11 (1), 142. doi:10.1186/1476-511X-11-142
Villareal M. O., Matsukawa T., Isoda H. (2018). l-Citrulline supplementation-increased skeletal muscle PGC-1α expression is associated with exercise performance and increased skeletal muscle weight. Mol. Nutr. Food Res. 62 (14), 1701043. doi:10.1002/mnfr.201701043
Villena J. A. (2015). New insights into PGC-1 coactivators: Redefining their role in the regulation of mitochondrial function and beyond. FEBS J. 282 (4), 647–672. doi:10.1111/febs.13175
Vohwinkel C. U., Lecuona E., Sun H., Sommer N., Vadász I., Chandel N. S., et al. (2011). Elevated CO(2) levels cause mitochondrial dysfunction and impair cell proliferation. J. Biol. Chem. 286 (43), 37067–37076. doi:10.1074/jbc.M111.290056
Volpe J. J. (2008). Postnatal sepsis, necrotizing entercolitis, and the critical role of systemic inflammation in white matter injury in premature infants. J. Pediatr. 153 (2), 160–163. doi:10.1016/j.jpeds.2008.04.057
Wang G., Hu Z., Fu Q., Song X., Cui Q., Jia R., et al. (2017). Resveratrol mitigates lipopolysaccharide-mediated acute inflammation in rats by inhibiting the TLR4/NF-κBp65/MAPKs signaling cascade. Sci. Rep. 7, 45006. doi:10.1038/srep45006
Wang H., Cheng Y., Liu Y., Shi J., Cheng Z. (2019). Montelukast promotes mitochondrial biogenesis via CREB/PGC-1α in human bronchial epithelial cells. Artif. Cells Nanomed. Biotechnol. 47 (1), 4234–4239. doi:10.1080/21691401.2019.1687502
Wang J., Dong W. (2018). Oxidative stress and bronchopulmonary dysplasia. Gene 678, 177–183. doi:10.1016/j.gene.2018.08.031
Wang Q., Zhou B., Cui Q., Chen C. (2019). Omega-3 long-chain polyunsaturated fatty acids for bronchopulmonary dysplasia: A meta-analysis. Pediatrics 144 (1), e20190181. doi:10.1542/peds.2019-0181
Wang Y., Lam K. S. L., hon Yau M., Xu A. (2008). Post-translational modifications of adiponectin: Mechanisms and functional implications. Biochem. J. 409 (3), 623–633. doi:10.1042/BJ20071492
Ware L. B., Magarik J. A., Wickersham N., Cunningham G., Rice T. W., Christman B. W., et al. (2013). Low plasma citrulline levels are associated with acute respiratory distress syndrome in patients with severe sepsis. Crit. Care 17 (1), R10. doi:10.1186/cc11934
Weismann C. G., Asnes J. D., Bazzy-Asaad A., Tolomeo C., Ehrenkranz R. A., Bizzarro M. J. (2017). Pulmonary hypertension in preterm infants: Results of a prospective screening program. J. Perinatol. 37 (5), 572–577. doi:10.1038/jp.2016.255
Wu J., Zhou Y., Wang G. (2021). Metformin use and survival in patients with advanced extrahepatic cholangiocarcinoma: A single-center cohort study in fuyang, China. Gastroenterol. Res. Pract. 2021, 9468227. doi:10.1155/2021/9468227
Wu M. H. (2019). Adiponectin: A pivotal role in the protection against cerebral ischemic injury. Neuroimmunol. Neuroinflammation 6, 4.
Wu N. N., Zhang Y., Ren J. (2019). Mitophagy, mitochondrial dynamics, and homeostasis in cardiovascular aging. Oxid. Med. Cell. Longev. 2019, 9825061. doi:10.1155/2019/9825061
Wu Z., Puigserver P., Andersson U., Zhang C., Adelmant G., Mootha V., et al. (1999). Mechanisms controlling mitochondrial biogenesis and respiration through the thermogenic coactivator PGC-1. Cell 98 (1), 115–124. doi:10.1016/S0092-8674(00)80611-X
Xia L., Ding F., Zhu J. H., Fu G. S. (2011). Resveratrol attenuates apoptosis of pulmonary microvascular endothelial cells induced by high shear stress and proinflammatory factors. Hum. Cell 24 (3), 127–133. doi:10.1007/s13577-011-0031-2
Xian H., Liu Y., Nilsson A. R., Gatchalian R., Crother T. R., Tourtellotte W. G., et al. (2021). Metformin inhibition of mitochondrial ATP and DNA synthesis abrogates NLRP3 inflammasome activation and pulmonary inflammation. Immunity 54 (7), 1463–1477. e11. doi:10.1016/j.immuni.2021.05.004
Xiang Z., Valenza M., Cui L., Leoni V., Jeong H. K., Brilli E., et al. (2011). Peroxisome-proliferator-activated receptor gamma coactivator 1 α contributes to dysmyelination in experimental models of Huntington’s disease. J. Neurosci. 31 (26), 9544–9553. doi:10.1523/JNEUROSCI.1291-11.2011
Xu N., Zhang Y., Doycheva D. M., Ding Y., Zhang Y., Tang J., et al. (2018). Adiponectin attenuates neuronal apoptosis induced by hypoxia-ischemia via the activation of AdipoR1/APPL1/LKB1/AMPK pathway in neonatal rats. Neuropharmacology 133, 415–428. doi:10.1016/j.neuropharm.2018.02.024
Xu R., Luo X., Ye X., Li H., Liu H., Du Q., et al. (2022). SIRT1/PGC-1α/PPAR-γ correlate with hypoxia-induced chemoresistance in non-small cell lung cancer. Front. Oncol. 11, 682762. doi:10.3389/fonc.2021.682762
Xu W., Chi L., Row B. W., Xu R., Ke Y., Xu B., et al. (2004). Increased oxidative stress is associated with chronic intermittent hypoxia-mediated brain cortical neuronal cell apoptosis in a mouse model of sleep apnea. Neuroscience 126 (2), 313–323. doi:10.1016/j.neuroscience.2004.03.055
Xu W., Zhao Y., Zhang B., Xu B., Yang Y., Wang Y., et al. (2015). Resveratrol attenuates hyperoxia-induced oxidative stress, inflammation and fibrosis and suppresses Wnt/β-catenin signalling in lungs of neonatal rats. Clin. Exp. Pharmacol. Physiol. 42 (10), 1075–1083. doi:10.1111/1440-1681.12459
Xuefei Y., Xinyi Z., Qing C., Dan Z., Ziyun L., Hejuan Z., et al. (2021). Effects of hyperoxia on mitochondrial homeostasis: Are mitochondria the hub for bronchopulmonary dysplasia? Front. Cell Dev. Biol. 9, 642717. doi:10.3389/fcell.2021.642717
Yabuki Y., Shioda N., Yamamoto Y., Shigano M., Kumagai K., Morita M., et al. (2013). Oral L-citrulline administration improves memory deficits following transient brain ischemia through cerebrovascular protection. Brain Res. 1520, 157–167. doi:10.1016/j.brainres.2013.05.011
Yang X., Dong W. B., Lei X. P., Li Q. P., Zhang L. Y., Zhang L. P. (2018). Resveratrol suppresses hyperoxia-induced nucleocytoplasmic shuttling of SIRT1 and ROS production in PBMC from preterm infants in vitro. J. Matern. Fetal. Neonatal Med. 31 (9), 1142–1150. doi:10.1080/14767058.2017.1311310
Yang X., Xu S., Qian Y., Xiao Q. (2017). Resveratrol regulates microglia M1/M2 polarization via PGC-1α in conditions of neuroinflammatory injury. Brain Behav. Immun. 64, 162–172. doi:10.1016/j.bbi.2017.03.003
Ye J. X., Wang S. S., Ge M., Wang D. J. (2016). Suppression of endothelial PGC-1α is associated with hypoxia-induced endothelial dysfunction and provides a new therapeutic target in pulmonary arterial hypertension. Am. J. Physiol. Lung Cell. Mol. Physiol. 310 (11), L1233–L1242. doi:10.1152/ajplung.00356.2015
Yin W., Signore A. P., Iwai M., Cao G., Gao Y., Chen J. (2008). Rapidly increased neuronal mitochondrial biogenesis after hypoxic-ischemic brain injury. Stroke 39 (11), 3057–3063. doi:10.1161/STROKEAHA.108.520114
Yu D. Q., Xu G. X., Teng X. Y., Xu J. W., Tang L. F., Feng C., et al. (2021). Glycemic control and neonatal outcomes in women with gestational diabetes mellitus treated using glyburide, metformin, or insulin: A pairwise and network meta-analysis. BMC Endocr. Disord. 21 (1), 199. doi:10.1186/s12902-021-00865-9
Yue L., Lu X., Dennery P. A., Yao H. (2021). Metabolic dysregulation in bronchopulmonary dysplasia: Implications for identification of biomarkers and therapeutic approaches. Redox Biol. 48, 102104. doi:10.1016/j.redox.2021.102104
Yue L., Yao H. (2016). Mitochondrial dysfunction in inflammatory responses and cellular senescence: Pathogenesis and pharmacological targets for chronic lung diseases. Br. J. Pharmacol. 173 (15), 2305–2318. doi:10.1111/bph.13518
Zaghloul N., Patel H., Codipilly C., Marambaud P., Dewey S., Frattini S., et al. (2014). Overexpression of extracellular superoxide dismutase protects against brain injury induced by chronic hypoxia. PLOS ONE 9 (9), e108168. doi:10.1371/journal.pone.0108168
Zhang D., Wang X., Wang B., Garza J. C., Fang X., Wang J., et al. (2017). Adiponectin regulates contextual fear extinction and intrinsic excitability of dentate gyrus granule neurons through AdipoR2 receptors. Mol. Psychiatry 22 (7), 1044–1055. doi:10.1038/mp.2016.58
Zhang J., Wang X., Vikash V., Ye Q., Wu D., Liu Y., et al. (2016). ROS and ROS-mediated cellular signaling. Oxid. Med. Cell. Longev. 2016, e4350965. doi:10.1155/2016/4350965
Zhang K., Yao E., Lin C., Chou Y. T., Wong J., Li J., et al. (2020). A mammalian Wnt5a-Ror2-Vangl2 axis controls the cytoskeleton and confers cellular properties required for alveologenesis. eLife 9, e53688. doi:10.7554/eLife.53688
Zhang P., Lavoie P. M., Lacaze-Masmonteil T., Rhainds M., Marc I. (2014). Omega-3 long-chain polyunsaturated fatty acids for extremely preterm infants: A systematic review. Pediatrics 134 (1), 120–134. doi:10.1542/peds.2014-0459
Zheng B., Liao Z., Locascio J. J., Lesniak K. A., Roderick S. S., Watt M. L., et al. (2010). PGC-1α, a potential therapeutic target for early intervention in Parkinson’s disease. Sci. Transl. Med. 2 (52), 52ra73. doi:10.1126/scitranslmed.3001059
Zhou J., Yang Z., Shen R., Zhong W., Zheng H., Chen Z., et al. (2021). Resveratrol improves mitochondrial biogenesis function and activates PGC-1α pathway in a preclinical model of early brain injury following subarachnoid hemorrhage. Front. Mol. Biosci. 8, 620683. doi:10.3389/fmolb.2021.620683
Zhu X. dan, Lei X. ping, Dong W. bin (2017). Resveratrol as a potential therapeutic drug for respiratory system diseases. Drug Des. devel. Ther. 11, 3591–3598. doi:10.2147/DDDT.S148868
Zhu X., Wang F., Lei X., Dong W. (2021). Resveratrol alleviates alveolar epithelial cell injury induced by hyperoxia by reducing apoptosis and mitochondrial dysfunction. Exp. Biol. Med. 246 (5), 596–606. doi:10.1177/1535370220975106
Keywords: mitochondrial dysfunction, oxidative stress, reactive oxygen species, PGC-1α, white matter injury, bronchopulmonary dysplasia
Citation: Mohammadi A, Higazy R and Gauda EB (2022) PGC-1α activity and mitochondrial dysfunction in preterm infants. Front. Physiol. 13:997619. doi: 10.3389/fphys.2022.997619
Received: 19 July 2022; Accepted: 09 September 2022;
Published: 26 September 2022.
Edited by:
Nicholas Jendzjowsky, Lundquist Institute for Biomedical Innovation, United StatesReviewed by:
Arlin Blood, Loma Linda University, United StatesAubrey Michi, Harvard University, United States
Copyright © 2022 Mohammadi, Higazy and Gauda. This is an open-access article distributed under the terms of the Creative Commons Attribution License (CC BY). The use, distribution or reproduction in other forums is permitted, provided the original author(s) and the copyright owner(s) are credited and that the original publication in this journal is cited, in accordance with accepted academic practice. No use, distribution or reproduction is permitted which does not comply with these terms.
*Correspondence: Estelle B. Gauda, ZXN0ZWxsZS5nYXVkYUBzaWNra2lkcy5jYQ==