- 1Department of Andrology, The First Hospital of Jilin University, Changchun, China
- 2Department of OB/GYN, University of Louisville School of Medicine, Louisville, KY, United States
- 3Department of Comparative Biosciences, University of Illinois at Urbana-Champaign, Urbana, IL, United States
- 4Birth Defects Center, University of Louisville School of Dentistry, Louisville, KY, United States
KRAS plays critical roles in regulating a range of normal cellular events as well as pathological processes in many tissues mediated through a variety of signaling pathways, including ERK1/2 and AKT signaling, in a cell-, context- and development-dependent manner. The in vivo function of KRAS and its downstream targets in gonadal steroidogenic cells for the development and homeostasis of reproductive functions remain to be determined. To understand the functions of KRAS signaling in gonadal theca and interstitial cells, we generated a Kras mutant (tKrasMT) mouse line that selectively expressed a constitutively active KrasG12D in these cells. KrasG12D expression in ovarian theca cells did not block follicle development to the preovulatory stage. However, tKrasMT females failed to ovulate and thus were infertile. The phosphorylated ERK1/2 and forkhead box O1 (FOXO1) and total FOXO1 protein levels were markedly reduced in tKrasMT theca cells. KrasG12D expression in theca cells also curtailed the phosphorylation of ERK1/2 and altered the expression of several ovulation-related genes in gonadotropin-primed granulosa cells. To uncover downstream targets of KRAS/FOXO1 signaling in theca cells, we found that the expression of bone morphogenic protein 7 (Bmp7), a theca-specific factor involved in ovulation, was significantly elevated in tKrasMT theca cells. Chromosome immunoprecipitation assays demonstrated that FOXO1 interacted with the Bmp7 promoter containing forkhead response elements and that the binding activity was attenuated in tKrasMT theca cells. Moreover, Foxo1 knockdown caused an elevation, whereas Foxo1 overexpression resulted in an inhibition of Bmp7 expression, suggesting that KRAS signaling regulates FOXO1 protein levels to control Bmp7 expression in theca cells. Thus, the anovulation phenotype observed in tKrasMT mice may be attributed to aberrant KRAS/FOXO1/BMP7 signaling in theca cells. Our work provides the first in vivo evidence that maintaining normal KRAS activity in ovarian theca cells is crucial for ovulation and female fertility.
Introduction
The Kirsten rat sarcoma viral oncogene homolog (Kras) is a member of three Ras (Hras, Kras, and Nras) family genes in mammals that encode a monomeric cytoplasmic protein with GTPase activity (Barbacid, 1987). It acts as a central component of the cellular networks communicating with extracellular stimuli through a variety of signaling pathways, including extracellular signal-regulated kinases 1 and 2 (ERK1/2) and phosphatidylinositol 3-kinase (PI3K)/protein kinase B (AKT) signaling, which modulate the growth, proliferation, survival, differentiation, adhesion, and motility of a cell (Malumbres and Barbacid, 2003; Han et al., 2021; Kim et al., 2021). Although Kras expression appears to be ubiquitous (Barbacid, 1987; Omerovic et al., 2007), its effects are cell-, context- and development-specific (Rocks et al., 2006; Sarkisian et al., 2007; Han et al., 2021). Studies of RAS-deficient mice reveal that Kras is the only member of the Ras gene family indispensable for embryonic development (Koera et al., 1997). Kras is the most frequently mutated in this family, and mutations are more likely at residue 12, resulting in a persistently GTP-bound form of KRAS and being inherently active independent of upstream input for activation (Bourne et al., 1990; Johnson et al., 2001). Constitutive activation of KRAS signaling is often associated with malignant cell transformation (Johnson et al., 2001; Murugan et al., 2019; Kim et al., 2021). Kras is expressed in various cell types of mammalian gonads (Fan and Richards, 2010; Tai and Ascoli, 2011; Fan et al., 2012; Richards et al., 2012). Amplified KRAS is observed in 11% of ovarian cancers and is much less common in testicular cancer cases (Cancer Genome Atlas Research, 2011; de Vries et al., 2020).
Mammalian gonads consist of three major cell populations, i.e., germ cells and steroidogenic and supporting somatic cells (Rotgers et al., 2018). The supporting cells, Sertoli cells in the testis and granulosa cells in the ovary, share a common precursor originating from the coelomic epithelium and play a vital role in nurturing the development of germ cells in the adult gonads. Sertoli cells support the progression of spermatogonia through meiosis to the production of motile spermatozoa. Granulosa cells, meanwhile, are a crucial component regulating the growth and maturation of ovarian follicles and the release of a fertilizable oocyte to produce an embryo. KRAS is an important physiological mediator of luteinizing hormone (LH) and follicle-stimulating hormone (FSH) signaling in granulosa cells (Fan and Richards, 2010; Fan et al., 2012). Abnormal activation of KRAS signaling in granulosa cells dysregulates the activity of ERK1/2 and AKT signaling and results in differentiation arrest of granulosa cells. The supporting cell-specific Kras mutant female mice are subfertile and display a phenotype resembling premature ovarian failure (Fan et al., 2008b). In contrast, mutant male mice are phenotypically healthy with grossly normal spermatogenesis and fertility (Richards et al., 2012).
Steroidogenic cells, interstitial cells in the testis and theca cells in the ovary, are crucial cell types in the gonadal interstitium that engage steroidogenesis and provide key hormones, androgens, for reproductive functions. They originate from the coelomic epithelium of the gonadal primordium and the neighboring mesonephros. Unlike testicular interstitial cells, ovarian theca cells do not differentiate during fetal gonadal development until perinatal stages when follicular development begins (Potter et al., 2016). Testicular androgen synthesis is obligatory for spermatogenesis and maintenance of the masculine phenotype. Androgens and many other growth regulatory factors produced by ovarian theca cells are important for normal follicle development, estrogen biosynthesis in granulosa cells, ovulation, and female fertility (Magoffin, 2005; Young and McNeilly, 2010; Richards et al., 2018). It is well characterized that androgenesis in theca cells is primarily regulated by LH signaling. While much has been learned about the factors that modulate steroidogenesis in theca cells, relatively less information is available about how the production of the factors in theca cells is regulated and what the roles are of these theca-produced factors, in addition to testosterone, in follicle development and ovulation. KRAS, a molecular switch in the transduction of multiple cellular signals, is known to be expressed in gonadal theca and interstitial cells (Shiraishi and Ascoli, 2006; Fan and Richards, 2010; Tai and Ascoli, 2011). However, the in vivo function of KRAS and its downstream targets in gonadal steroidogenic cells for the development and homeostasis of reproductive functions remain to be determined.
By knocking-in a constitutively active Kras specifically in mouse steroidogenic cells, the present study provides the first in vivo evidence that maintaining normal KRAS activity in ovarian theca cells is essential for ovulation and female fertility. Molecular analyses reveal that KRAS signaling in theca cells regulates forkhead box O1 (FOXO1) protein levels to control the expression of theca cell-specific growth factor bone morphogenic protein 7 (BMP7). Our results suggest that aberrant KRAS/FOXO1/BMP7 signaling in theca cells is likely an important cause of anovulation and female infertility.
Materials and methods
Animals and genotyping
Mice carrying a lox-stop-lox (Lsl) sequence followed by the KrasG12D point mutation allele (LslKrasG12D) (Johnson et al., 2001) were purchased from the Jackson Laboratories (Bar Harbor, ME, USA). Cyp17iCre mice (Bridges et al., 2008) were imported from the University of Kentucky (Lexington, KY, USA). LslKrasG12D mice were crossed with Cyp17iCre mice to generate mice with theca-interstitial cell specific knocking-in of the KrasG12D mutant allele, hereafter referred to as tKrasMT mice. Mouse tail genomic DNA was extracted using ZR genomic DNA-tissue mini prep kits according to the procedure recommended by the manufacturer (Lan et al., 2017) (Zymo Research Corp, Irvine, CA, USA) and genotyped by PCR using the primers synthesized by Eurofins Genomics LLC (Louisville, KY, USA) as follows: 5′-tgtctttccccagcacagt-3’ (wild-type Kras allele forward), 5′-gcaggtcgagggacctaata-3’ (KrasG12D mutant allele forward) and 5′-ctgcatagtacgctataccctgt-3’ (common reverse for wild-type and mutant alleles).
All mice were on the C57BL/6 background and were housed on a 14-h light:10-h dark cycle with free access to food and water. The studies were approved by the Animal Care and Use Committee of the University of Louisville. All mice were sacrificed under ketamine anesthesia, and efforts were made to minimize their discomfort.
Fertility, superovulation, and oocyte maturation studies
Six 7- to 8-week-old tKrasMT and control (LslKrasG12D) female mice were bred with proven fertile wild-type C57BL/6 males (1 male/1 female per cage) for 2 months. The total number of pups produced from each female was recorded as described previously (Lan et al., 2003).
Superovulation studies were performed on 21- to 25-day-old female littermates by intraperitoneal injections of 5 IU equine chorionic gonadotropin (eCG, Sigma, St Louis, MO, USA) for 48 h followed by 5 IU human chorionic gonadotropin (hCG, Sigma) for 22 h as described previously (Lan et al., 2003; Fan et al., 2009b). The total number of oocytes collected from both sides of the oviducts of each animal was recorded.
For the spontaneous oocyte maturation assay, immature oocytes were retrieved from 21- to 25-day-old eCG-primed (5 IU for 48 h) tKrasMT and control (LslKrasG12D) female mice according to previously described methods (Anderiesz et al., 2000; Lan et al., 2003). The oocytes were denuded by incubation with hyaluronidase solution immediately after removal from the ovarian follicle and cultured in 20 μL drops of Eagle’s Minimal Essential Medium–alpha modification (α-EMEM, Sigma) medium supplemented with 10% fetal bovine serum (FBS) under light mineral oil at 37°C for 24 h. The oocytes were examined with phase contrast microscopy for meiotic progression. The oocytes containing a polar body were considered in vitro matured.
Histological and immunohistochemical staining
Ovaries and testes were fixed in 4% paraformaldehyde (Sigma) in phosphate-buffered saline (PBS) overnight and embedded in paraffin. Seven-micrometer-thick cross-sections were cut and used for hematoxylin and eosin (H&E) and immunohistochemical staining.
Immunohistochemistry was performed by an avidin-biotin immunoperoxidase method as described previously (Cao et al., 2022). Briefly, deparaffinized sections were rehydrated and then incubated with 1% H2O2 for 30 min. After rinsing with PBS and incubating with normal serum at room temperature (RT), the sections were incubated with rabbit anti-KRAS or anti-HSD3B1 (3-β-hydroxysteroid dehydrogenase 1) antibody (1:100, ABclonal, Woburn, MA, USA) overnight at 4°C and then incubated with biotinylated anti-rabbit IgG secondary antibody (1:100, Vector Laboratories, Burlingame, CA, USA) for 1 h at RT. After rinsing with PBS, sections were incubated with avidin-biotin-horseradish peroxidase complex using a Vectastain ABC kit (Vector Labs, Burlingame, CA, USA) for 1 h and rinsed with PBS. Immunostaining was detected by incubation of the sections with the substrate 3′3-diaminobenzidine at RT. All sections were counterstained with hematoxylin. Replacement of the primary antibody with irrelevant rabbit IgG was used as a procedure control.
Isolation of granulosa, theca and interstitial cells
Ovaries isolated from eCG-primed 21- to 25-day-old female mice with the bursa removed were placed in RPMI 1640 medium (Sigma). Granulosa cells were collected by multiple follicle punctures using 30-gauge needles followed by filtration through 40-µm cell strainers (BD Falcon, Bedford, MA, USA) to remove oocytes. Theca cells were isolated as reported with minor modifications (Magoffin and Erickson, 1988; Li and Hearn, 2000). In brief, ovaries were punctured using 30-gauge needles to completely release antral granulosa/oocyte complexes. Punctured ovaries were incubated in 0.1°ml/ovary of 0.1% collagenase and 0.01% DNase I (Sigma) solution at 37°C to release theca cells. The incubation was terminated when theca layers from most preantral follicles were released into the solution, while most preantral granulosa/oocyte complexes remained intact (Fan et al., 2009a). Dispersed theca cells were obtained by filtration of the enzyme-digested solutions through 40-µm cell strainers (BD Falcon) to remove preantral granulosa/oocyte complexes and undigested ovarian tissues. The isolated theca cells were purified by a Percoll gradient centrifugation procedure. Briefly, dispersed cells were layered on top of a discontinuous Percoll density gradient (a specific gravity of 1.055 and 44% Percoll solution, Sigma) and then centrifuged for 20 min at 400 X g. The cells within the gravity of 1.055 Percoll layer were collected (Magoffin and Erickson, 1988; Li and Hearn, 2000). RT-PCR tests showed that isolated cells expressed high levels of theca cell marker genes (Lhcgr, Hsd3b1 and Cyp17b1) but not granulosa cell marker genes (Fshr and Cyp19a1).
Interstitial cells from the testes of adult tKrasMT mice were prepared as described by Yang et al (Yang et al., 2016). In brief, the testes were decapsulated, cut into small pieces, and then subjected to a gentle mechanical dissociation followed by a 15-min digestion at 34°C with 0.05% collagenase (Sigma). The crude testicular cell suspension obtained was then filtered through 70-µm cell strainers (BD Falcon), and the cells were layered on top of discontinuous Percoll gradients (5–70%, Sigma) and centrifuged.
Reverse transcription-polymerase chain reaction and quantitative RT-PCR
Total RNA from testes, interstitial cells, ovaries, adrenal glands, uteri, pituitaries, theca cells, granulosa cells and oocyte-granulosa mixtures was isolated using TRIzol reagent (Invitrogen, Carlsbad, CA, USA) as described previously (Yuan et al., 2010). Briefly, total RNA was adjusted to a concentration of approximately 1.0 μg/μL. Two microgram of total RNA was reverse transcribed into cDNA with random primers (Invitrogen) and avian myeloblastosis virus (AMV) reverse transcriptase (Promega Corp, Madison, WI, USA). KrasG12D expression in the ovary, theca cells, granulosa cells, interstitial cells and testes was detected by RT-PCR using the primer set (5′-aggcctgctgaaaatgactg-3′ and 5′-ccctccccagttctcatgta-3′, Eurofins Genomics LLC) followed by digestion of amplified PCR products with Hind III (Promega Corp) as described (Aguirre et al., 2003). The digested products were separated by electrophoresis in agarose gels and stained with ethidium bromide. The KrasG12D allele, but not the wild-type Kras allele, contained a Hind III restriction site engineered in exon 1. Digestion of the 243°bp PCR products with Hind III yielded 213 and 30 base pair bands from the mutant products only.
qRT-PCR analyses were performed as described previously (Lan et al., 2003). In brief, first strand cDNA was synthesized using TaqMan RNA reverse transcription reagents (Thermo Fisher Scientific, Waltham, MA, USA). Predesigned TaqMan probes and primers for Dusp6 (Duel-specific phosphatase 6, Mm00518185_m1), Foxo1 (Forkhead box O1, Mm00490672_m1), Bmp7 (Bone morphogenetic protein 7, Mm00432102_m1), Fshr (FSH receptor, Mm00442819_m1), Hsd17b1 (Hydroxysteroid 17-Beta-dehydrogenase 1, Mm00501692_g1), Cyp19a1 (Aromatase, Mm00484049_m1), Cyp11a1 (Cytochrome P450 cholesterol side-chain cleavage enzyme, Mm00490735_m1), Nrip1 (Nuclear receptor interacting protein 1, Mm00476537_s1), Pten (Phosphatase and tensin homolog, Mm00477208_m1), Npr2 (Natriuretic peptide receptor 2, Mm00612889_m1), Areg (Amphiregulin, Mm01354389_m1) and Btc (Betacellulin, Mm00432137_m1) were purchased from Applied Biosystem/Thermo Fisher Scientific. Our previously reported TaqMan probes and primers of Star (Steroidogenic acute regulatory protein), Cyp17a1 (Cytochrome P450 family 17 subfamily A member 1), Lhcgr (LH/hCG receptor), Hsd3b1 (Hydroxy-delta-5-steroid dehydrogenase) and Actb (Actin beta) were used to determine their expression in the samples (Lan et al., 2003). Relative mRNA levels were obtained after normalization to the mRNA levels of the housekeeping gene Actb in each sample. At least three individual RNA samples per group were examined.
Hormone assays
Eleven-to 12-week-old male mice and female mice at diestrous were anesthetized and exsanguinated by cardiac puncture. Sera were separated and stored at −80°C until assayed. FSH, estradiol and testosterone levels were determined by the Ligand Assay and Analysis Core Center for Research in Reproduction, the University of Virginia (Charlottesville, VA, USA). Mouse FSH, estradiol and testosterone levels were measured by radioimmunoassay (RIA). The limits of sensitivities of these assays were 2 ng/ml for FSH, 10 pg/ml for estradiol and 5 ng/dl for testosterone.
Theca cell culture, Foxo1 knockdown and pCMV-Foxo1 transfection
The isolated theca cells were maintained in 12-well plates with RPMI-1640 medium containing 25 mM HEPES, 2 mM l-glutamine, 10% heat-inactivated FBS and 1% Pen-Strep (Invitrogen). Cells were then cultured at 37°C in an incubator with 95% air and 5% CO2. For Foxo1 knockdown, theca cells were isolated from 60-day-old wild-type ovaries and transfected with Foxo1 siRNA using a procedure described previously (Yuan et al., 2010). The Foxo1 siRNAs, purchased from Santa Cruz Biotech (Dallas, TX, USA) consisted of a pool of 3–5 target-specific siRNAs against mouse Foxo1. Typically, a mixture of 0.25 μg siRNA, 100 μL RPMI-1640 and 4 μL Lipofectamine 2000 (Invitrogen) was added to the cells in a 12-well plate and incubated overnight. The transfection medium was replaced with 0.5 ml RPMI-1640 containing 10% FBS (Sigma) for an additional 24°h, and these cells were used for subsequent experiments. A control siRNA (Santa Cruz Biotech) consisting of a scrambled RNA sequence was transfected in parallel with Foxo1 siRNA as a specificity control for the knockdown. Western blotting was used to determine the effectiveness of Foxo1 siRNAs in suppressing FOXO1 protein levels in theca cells. A transient DNA transfection procedure described previously was used to overexpress mouse Foxo1 in tKrasMT theca cells (Cao et al., 2022). Briefly, the cells were cultured to approximately 80% confluence in 12-well plates and were transiently transfected with 1 µg of pCMV-Foxo1 plasmid DNA (Nakae et al., 2001) (Addgene, Watertown, MA, USA) with 2 µL of X-TremeGene 360 reagent (Roche, Mannheim, Germany) per well for 72 h. The cells were then cultured in RPMI-1640 containing 10% FBS. The cells transfected with empty vector pCMV served as the control. FOXO1 protein levels in transfected cells were checked by Western blot.
Western blotting
Granulosa and theca cells were homogenized by sonication with a sonic dismembrator Model 150 (Thermo Fisher Scientific) in ice-cold lysis buffer containing complete proteinase and phosphatase inhibitor cocktails (Roche, Indianapolis, IN, USA). The protein concentrations were measured by the Bradford method (Bio-Rad Laboratories, Hercules, CA, USA). Five μg/lane of cell lysates were separated in SDS-PAGE gels, transferred to Immobilon-PVDF membranes (Millipore, Billerica, MA, USA), blocked with 3% nonfat milk, and then incubated overnight with primary antibodies. Phospho-ERK1/2T202/Y204, phospho-AKTS473, AKT, phospho-CREBS133, CREB, phospho-FOXO1S256 and FOXO1 antibodies were procured from Cell Signaling Technology Inc. (Danvers, MA, USA). ERK1/2 and β-actin antibodies were purchased from Sigma. Peroxidase-conjugated secondary antibody (1:2,000, Vector Laboratories) was used as the secondary antibody. Immunoblotting signals were detected by the enhanced chemiluminescence (ECL) Western blotting detection system (GE Healthcare Biosciences, Pittsburgh, PA, USA) as described previously (Li et al., 2016). All membranes were reblotted with β-actin antibody (1:1,000) as the loading control. The intensity of specific bands was scanned and semiquantified using the image analysis software, TotalLab V (Nonlinear USA Inc., Durham, NC). The optical density of the protein bands was normalized to that of β-actin. Experiments were repeated three times on samples from at least three animals.
Chromatin immunoprecipitation
The ChIP assays were performed using an Imprint Ultra ChIP kit (Sigma) as described previously (Lin et al., 2014; Lin and Lei, 2016). Briefly, theca cells isolated from 60-day-old ovaries were cultured with RPMI-1640 medium supplemented with 10% FBS. The chromatin was crosslinked by treating the cells with 1% formaldehyde for 10 min at room temperature. The cells were harvested and homogenized with a glass Dounce homogenizer (Thermo Fisher Scientific). The crosslinked chromatin was fragmented by sonication (3 × 10 min) with a sonic dismembrator Model 150 (Thermo Fisher Scientific). Rabbit anti-FOXO1 antibody (Cell Signaling Technology) at 1:100 dilution was used for immunoprecipitation. The DNA was then purified and used for PCR with the primer set (5′-ggcaggagaatctctgtgaact-3′ and 5′-aacattcagctaaggtgccaag-3′, Eurofins Genomics LLC) and the conditions (denaturation for 30 s at 95°C, annealing for 30 s at 60°C, and extension for 1 min at 72°C.) for 40 cycles. The PCR products that amplified the Bmp7 promoter from the -5,465 to -5,261 bp region were validated by Pst I (Promega) digestion.
Statistical analysis
The data presented are the means ± SEM of three samples or indicated numbers of samples in the figures. The results were analyzed by one-way analysis of variance (ANOVA) and unpaired Student’s t test using a version 3.06 Instat program (GraphPad Software, San Diego, CA). A p value < 0.05 was considered statistically significant.
Results
Generation of tKrasMT mice
Immunohistochemical staining demonstrated that KRAS was expressed in multiple cell types in gonads, including theca and granulosa cells as well as oocytes in the ovaries (Supplementary Figures S1A,B) and interstitial and spermatogenic cells in the testes (Supplementary Figures S1C,D). By crossing lox-stop-lox-KrasG12D (LslKrasG12D) mice (Johnson et al., 2001) with previously generated Cyp17iCre transgenic mice that express iCre in theca and interstitial cells of ovaries and testes (Bridges et al., 2008) (Figure 1A), LslKrasG12D;Cyp17iCre mice were generated and named tKrasMT mice. tKrasMT male and female mice survived to adulthood.
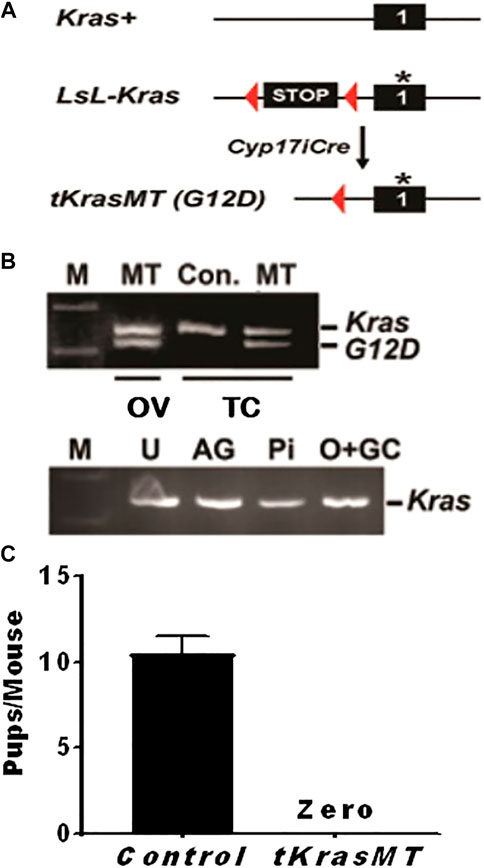
FIGURE 1. Knocking in a constitutively active Kras (KrasG12D) in theca cells (tKrasMT) results in female infertility. (A) A Cre/loxP strategy for the generation of tKrasMT mice. An asterisk represents a G12D mutation in KRAS protein. (B). RT-PCR showing KrasG12D expression in tKrasMT ovaries (OV) and theca cells (TC) but not in uteri (U), adrenal glands (AG), pituitary (Pi), or oocyte and granulosa cell mixtures (O + GC) of tKrasMT mice. KrasG12D expression is visualized by the digestion of PCR products with Hind III that has been introduced into the KrasG12D exon. (C). No pups were produced by mature tKrasMT female mice during the 2-months breeding assay (n = 6).
To determine whether KrasG12D was expressed in the ovaries of tKrasMT mice, total RNA was isolated from the ovaries, uteri, adrenal glands, and pituitaries of 4-week-old tKrasMT mice and then subjected to RT-PCR followed by Hind III digestion of PCR products as described (Aguirre et al., 2003). As shown in Figure 1B, KrasG12D transcripts were detected only in the ovaries but not in the uteri, adrenal glands, or pituitaries of tKrasMT mice. To determine whether KrasG12D was selectively expressed in theca cells of tKrasMT ovaries, theca cells and oocyte-granulosa mixtures were isolated from eCG-primed 21- to 25-day-old tKrasMT females and then subjected to the above described KrasG12D expression analysis. As shown in Figure 1B, KrasG12D transcripts were detected in purified theca cells but not oocyte-granulosa mixtures of tKrasMT mice.
Indistinguishable testicular phenotype of tKrasMT from LslKrasG12D male mice
RT-PCR demonstrated KrasG12D expression in interstitial cells of the testis in tKrasMT males but not in LslKrasG12D control mice (Supplementary Figure S2A). tKrasMT male mice were phenotypically normal and had grossly unaltered fertility and spermatogenesis. The testis, epididymis (Supplementary Figures S2B,C) and other sex accessory organs in tKrasMT males were indistinguishable from those in LslKrasG12D controls. Adult tKrasMT males sired normally (data not shown). Histological examination of adult testes and epididymis revealed normal spermatogenesis in tKrasMT males (Supplementary Figures S2D,E,H,I). There was no difference in the immunostaining intensity of HSD3B1, a critical androgen biosynthesis enzyme, in interstitial cells between tKrasMT mice and LslKrasG12D controls (Supplementary Figures S2F,G). Since no overt testis or fertility phenotypes were observed in tKrasMT males, this report focused on the characterization of ovarian and fertility phenotypes of tKrasMT females.
Female sterility and the absence of corpous leteum in adult tKrasMT female mice
To determine whether there is any fertility defect in tKrasMT female mice, six 6-week-old tKrasMT females and six LslKrasG12D control females were bred with stud males for 2 months. Within the breeding period, all six controls produced two litters of progenies with an average of 10.5 total pups (±1.0) per mouse (Figure 1C). However, tKrasMT females produced no progeny (Figure 1C). Thus, tKrasMT mice displayed female sterility.
To gain insight into the cause of female sterility in tKrasMT mice, we examined the gross morphology and histology of ovaries from LslKrasG12D control and tKrasMT mice at various ages. At the age of 5 weeks, there was no difference in the gross morphology (Figure 2A) or weight of ovaries between controls and tKrasMT mice. The ovarian weight (mg)/body weight (g) was 0.296 ± 0.042 (control, n = 6) vs. 0.306 ± 0.002 (tKrasMT, n = 6, Supplementary Figure S3A). However, hemorrhagic ovaries without visible corpora lutea were observed in 7- or 12-week-old tKrasMT ovaries (Figure 2). Histological studies on serial sections (Figures 2B–G) showed that follicles at each developmental stage (from primordial to preovulatory stage) were present in tKrasMT ovaries despite the presence of some degenerating preantral follicles (Figures 2D–G). Interestingly, follicles at the late antral and preovulatory stages in adult tKrasMT mice were hemorrhagic with visible loss or dislodgement of peripheral mural granulosa cells to form a cyst-like structure (Figures 2D,F). Strikingly, no corpora lutea were found in adult tKrasMT ovaries (Figures 2E,G). Ovarian tumors and hyperplasia in ovarian cells, including theca cells, were not observed in 3- to 12-week-old tKrasMT mice examined.
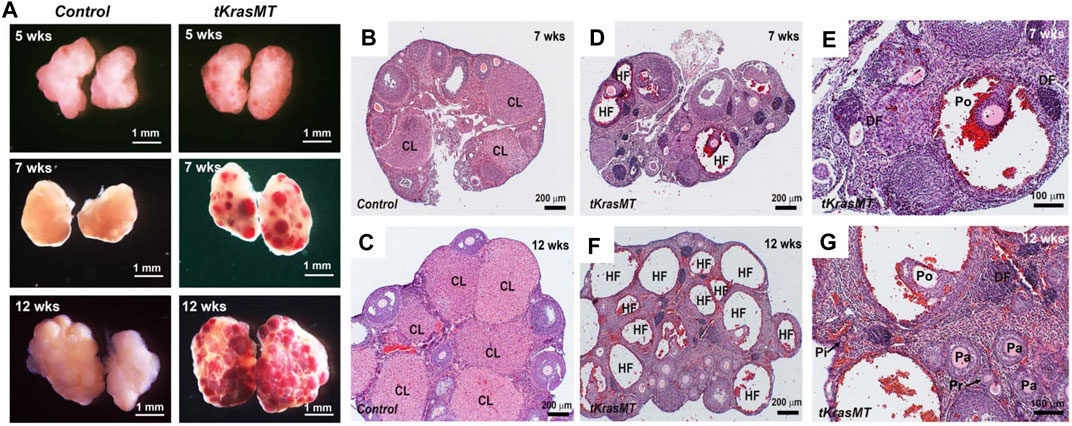
FIGURE 2. Ovarian phenotype of tKrasMT females. (A). Gross ovarian morphology of 5-, 7- and 12-week-old females shows age-dependent ovary hemorrhage in tKrasMT mice. (B–G). Ovarian histology of 7- and 12-week-old controls [LslKrasG12D (B,C)] and tKrasMT (D–G) mice. Follicle hemorrhage and degeneration are obvious, but the corpus luteum is absent in adult tKrasMT ovaries. Panels E and G are higher magnifications of Panels D and F, respectively. CL, corpus luteum; HF, hemorrhagic follicles; DF, degenerating follicles; Pi, primordial follicle; Pr, primary follicle; Pa, preantral follicle; Po, preovulatory follicles. Note the dislodgement of peripheral mural granulosa cells in large antral/preovulatory follicles in F and G to form a cyst-like structure.
Anovulation and no corpus luteal formation in immature tKrasMT mice in response to exogenous gonadotropins
The above morphological and histological studies on adult tKrasMT ovaries indicate that tKrasMT mice likely have a defect in ovulation. To investigate this possibility and to avoid the complexity of endocrine hormones in adult mice for ovulation, immature (3- to 4-week-old) LslKrasG12D control and tKrasMT female mice were subjected to superovulation studies. As shown in Figure 3A, immature tKrasMT females failed to ovulate in response to exogenous gonadotropin stimulation. Gross morphological analyses showed that there was no difference between LslKrasG12D control (Figure 3B) and tKrasMT (Figure 3C) mice after eCG/hCG treatments. Ovarian weight (mg)/body weight (g) was 0.729 ± 0.088 (control, n = 5) vs. 0.788 ± 0.089 (tKrasMT, n = 5, Supplementary Figure S3A). Unlike LslKrasG12D controls (Figure 3D), there was no disruption of follicle development to the preovulatory stage in immature tKrasMT mice after gonadotropin treatments. No corporus luteum and no sign of germinal vesicle breakdown, cumulus expansion, or follicle rupture were observed in the ovaries of gonadotropin-treated immature tKrasMT mice (Figures 3E–H). To further confirm the absence of corporus luteum in eCG/hCG-treated tKrasMT mice, qRT-PCR was performed to examine the expression of a healthy corpus luteal marker gene, Cyp11a1. As shown in Figure 3I, Cyp11a1 mRNA levels were markedly lower in the ovaries of gonadotropin-treated tKrasMT mice than in those of LslKrasG12D control mice.
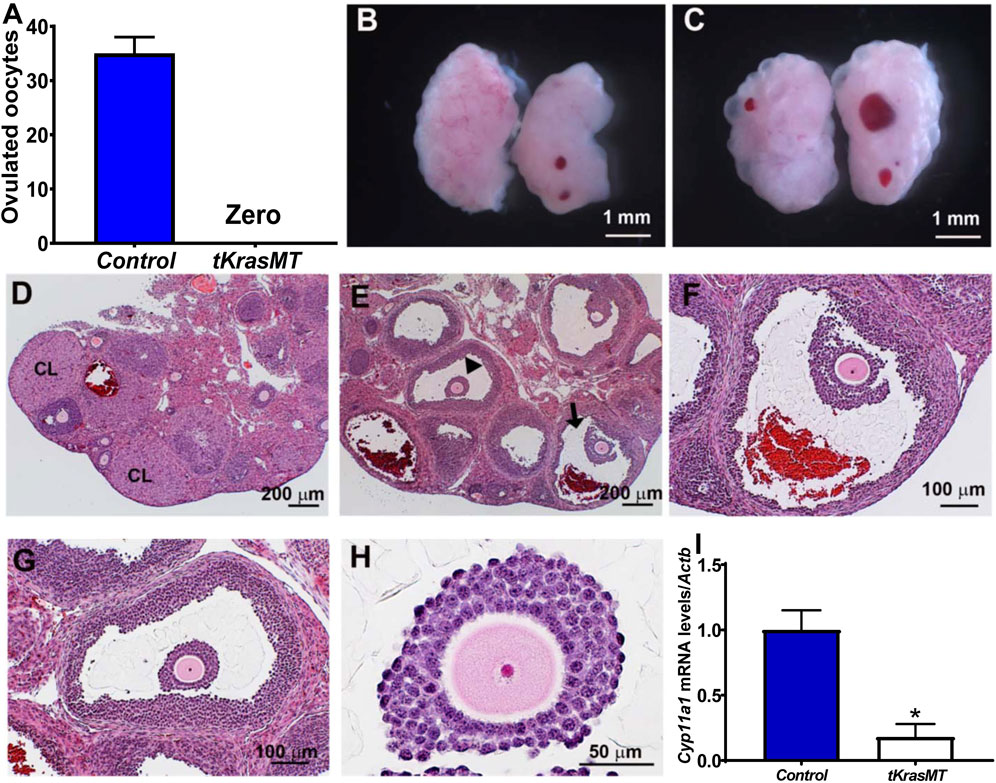
FIGURE 3. Anovulation and no corpus luteum formation in immature tKrasMT mice primed with exogenous gonadotropins. Numbers of ovulated oocytes (n = 6) (A), gross morphology (B,C) and histology (D–H) of ovaries in immature control [LslKrasG12D (B,D)] and tKrasMT (C,E–H) mice treated with eCG for 48 h followed by hCG for 22 h. Panels F & G are a higher magnification of follicles indicated by an arrowhead and an arrow in Panel E, respectively. Panel H is a higher magnification of a preovulatory follicle in tKrasMT ovary in Panel G showing a lack of cumulus expansion in response to exogenous gonadotropins. (I). Reduced Cyp11a1 mRNA levels in tKrasMT ovaries by qRT-PCR. *, p < 0.01 compared to the control (LslkrasG12D) group (n = 6).
In vitro oocyte maturation analysis showed that denuded oocytes from eCG primed prepuberal tKrasMT female mice, the same as the controls, were capable of progressing to metaphase II (Supplementary Figure S3B), indicating that the tKrasMT oocytes were competent for spontaneous maturation.
Downregulation of forkhead box O1 protein levels and enhanced Bmp7 transcription in tKrasMT theca cells
To investigate whether KrasG12D regulates MAPK, PI3K/AKT and PKA signaling in theca cells, theca cells were isolated from eCG-primed LslKrasG12D control and tKrasMT mice, and subjected to Western blot analysis of their representative signaling molecules. As shown in Figure 4, the levels of phospho-ERK1/2 (pERK1/2, indicators of MAPK signaling), but not total ERK1/2, were reduced in tKrasMT theca cells (Figures 4A,B). In contrast, phospho-AKT (pAKT, an indicator of PI3K/AKT signaling), phospho-CREB (pCREB, an indicator of PKA signaling) and their total protein levels were not obviously altered in tKrasMT theca cells (Figure 4A). Downregulation of pERK1/2 levels has been observed in ovarian granulosa cells and nongonadal cells under the condition of persistent activation of RAS proteins, including KRASG12D, and this is caused by the induced expression of phosphatases such as Dusp6 (Camps et al., 2000). To investigate this possibility in theca cells, qRT-PCR was performed to examine Dusp6 expression in tKrasMT theca cells. As shown in Figure 4D, Dusp6 expression was significantly elevated in tKrasMT theca cells. Together, KRASG12D in theca cells mainly influenced ERK1/2 signaling with minimal effects on AKT or PKA signaling.
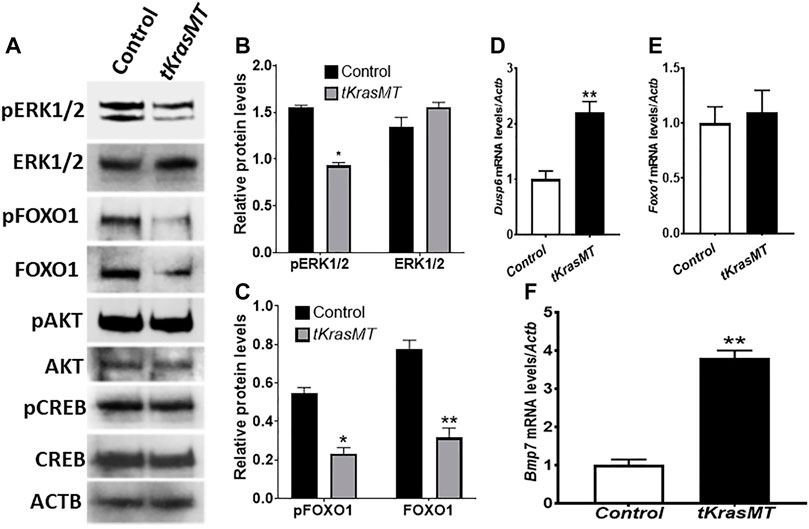
FIGURE 4. Reduced pERK1/2 and FOXO1 protein levels and elevated Bmp7 expression in tKrasMT theca cells. (A). Expression of total and phosphorylated proteins of various signaling molecules in control (LslKrasG12D) and tKrasMT theca cells. Theca cell protein extracts were collected from 3-5 immature control or tKrasMT mice pretreated with eCG for 48 h, and subjected to Western blot analyses. Equal amounts of proteins (5 µg proteins) were loaded in each lane, and data were obtained from the same membrane blot using the indicated antibodies. (B,C). Western blot results show that the relative protein levels of pERK1/2 (B), pFOXO1 and FOXO1 (C) but not ERK1/2 (B) are significantly reduced in tKrasMT theca cells. (D). Increased Dusp6 expression in tKrasMT theca cells by qRT-PCR. (E). No significant change in Foxo1 mRNA expression in tKrasMT theca cells by qRT-PCR. (F). Elevated Bmp7 mRNA expression in tKrasMT theca cells by qRT-PCR. *, p < 0.05; **, p < 0.01 compared to the control (LslkrasG12D) group (n = 5).
Reduced pERK1/2 in theca cells of tKrasMT ovaries prompted us to examine Foxo1 expression at both the protein and mRNA levels. As shown in Figures 4A,C, total FOXO1 and phospho-FOXO1 (pFOXO1) protein levels were markedly decreased in tKrasMT theca cells. However, Foxo1 mRNA expression was not significantly altered in tKrasMT theca cells (Figure 4E).
FOXO1 is a transcription factor that binds forkhead responsive elements (5′-(G/C)(T/A)AA(C/T)AA-3′, FHREs) to regulate gene expression (Furuyama et al., 2000; Huang and Tindall, 2007). Reduced FOXO1 protein levels in tKrasMT theca cells suggest that FOXO1-regulated gene(s) exist in theca cells, which are involved in the regulation of ovulation or corpus luteal formation. To identify the potential FOXO1 target gene(s), qRT-PCR analyses were performed on isolated tKrasMT theca cells. As shown in Figure 4F, the expression of Bmp7, a theca-specific growth factor (Shimasaki et al., 1999; Erickson and Shimasaki, 2003), was significantly elevated in tKrasMT theca cells. In contrast, the expression of Star, Cyp11a1, Hsd3b1, Cyp17a1 and Hsd17b1, which are critical for steroidogenesis, was not significantly altered in tKrasMT theca cells (Supplementary Figure S4D). In addition, sera were also collected from adult tKrasMT mice at diestrus for steroid hormone analyses. The results showed that serum testosterone levels were not significantly altered in tKrasMT mice (Supplementary Figure S4A).
Indirect influences of KRASG12D on Areg, Btc, Cyp19a1 and Cyp11a1 expression in preovulatory granulosa cells
To investigate whether there exists a paracrine regulation of granulosa cell function from theca cells in tKrasMT mice, preovulatory granulosa cells were isolated from eCG-treated control and tKrasMT mice and subjected to qRT-PCR analyses of several genes involved in ovulation processes, estradiol biosynthesis and granulosa cell differentiation. As expected, KrasG12D was not expressed in isolated preovulatory granulosa cells of tKrasMT mice (Figure 5A). Western blot results revealed a reduction in the phosphorylation of ERK1/2 in preovulatory granulosa cells (Figures 5B,C). Analyses of genes involved in the ovulation process showed that the expression of Areg and Btc but not Nrip1, Npr2 and Pten was markedly increased in tKrasMT granulosa cells (Figure 5D). In addition, Fshr, Lhcgr, and Hsd3b1 were not significantly altered in preovulatory granulosa cells from tKrasMT mice (Figure 5D). In contrast, the expression of Cyp19a1 was significantly increased, while the expression of Cyp11a1 was markedly decreased in tKrasMT granulosa cells. In addition, sera of adult tKrasMT mice were also collected and subjected to RIA analyses of estradiol and FSH levels. Serum estradiol but not FSH levels were significantly elevated in tKrasMT mice (Supplementary Figures S4B,C). Together, KrasG12D in theca cells indirectly caused mis-expression of Areg, Btc, Cyp19a1 and Cyp11a1 in preovulatory granulosa cells.
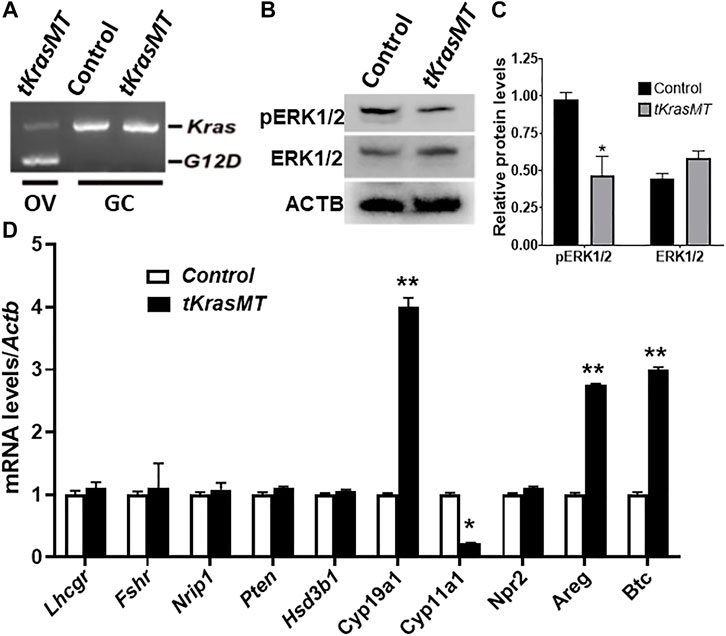
FIGURE 5. Granulosa cells of tKrasMT mice. (A). RT-PCR showing the absence of KrasG12D expression in preovulatory granulosa cells (GC) isolated from eCG-primed immature tKrasMT mice. (B,C). Western blot results show that the relative protein levels of pERK1/2 but not ERK1/2 are markedly reduced in tKrasMT granulosa cells. (D). Misexpression of genes associated with ovulation and oocyte maturation and/or cumulus expansion in preovulatory granulosa cells from tKrasMT mice by qRT-PCR. *, p < 0.05; **, p < 0.01 compared to the control (LslkrasG12D) group (n = 5).
Regulation of Bmp7 expression by FOXO1 in tKrasMT theca cells
To investigate whether Bmp7 is indeed a target of KRAS/FOXO1 signaling in theca cells, ChIP assays were performed. The results showed that endogenous FOXO1 in theca cells was able to interact with the Bmp7 promoter containing FHRE repeats at the -5,357 to -5,339 base-pair region, and this interaction was attenuated in tKrasMT theca cells (Figures 6E,F). Knockdown of Foxo1 in theca cells by RNA interference (Figure 6A) enhanced Bmp7 expression (Figure 6B). In contrast, Bmp7 expression in tKrasMT theca cells (Figure 6C) was curtailed by Foxo1 overexpression (Figure 6D). Therefore, Bmp7 is a target of KRAS/FOXO1 signaling in theca cells.
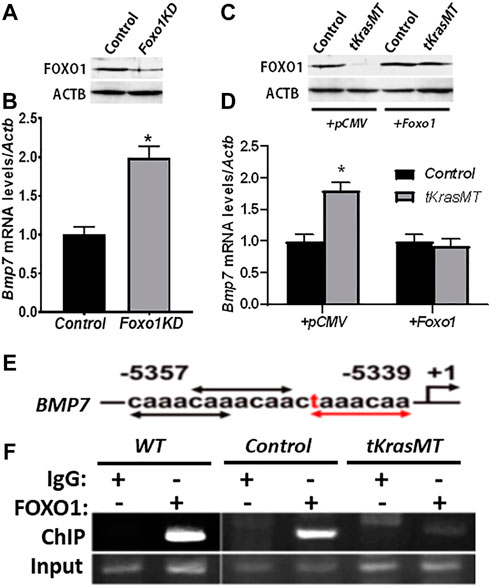
FIGURE 6. Bmp7 is a target of KRAS/FOXO1 signaling in theca cells. (A,B). Foxo1 knockdown (Foxo1KD) by siRNA (A) increases Bmp7 expression in theca cells (B) (n = 3). *, p < 0.05 compared to the control (scrambled siRNA) group. (C,D). Bmp7 expression (D) in empty vector (+pCMV) or pCMV-Foxo1 (+Foxo1) transfected tKrasMT theca cells (C). *, p < 0.05 compared to the control group. (E,F). Association of FOXO1 with the Bmp7 promoter containing consensus (black arrows) and 1-mismatched (red text and arrows) FHREs (E) by chromatin-immunoprecipitation (ChIP) (F), (n = 3).
Discussion
Kras is expressed in ovarian theca cells in addition to oocytes and granulosa cells, which is consistent with previous reports that KRAS protein was also detected in various types of cells (Fan and Richards, 2010; Fan et al., 2012; Richards et al., 2012). KRAS is known to be essential for normal embryonic development (Koera et al., 1997) and the potential functional redundancies among three RAS proteins (Johnson et al., 1997), The loss of KRAS function in theca cells might not allow us to uncover the roles of theca KRAS in gonadal development and fertility. Therefore, a gain-of-function knock-in strategy to selectively express a constitutively active Kras, KrasG12D (Johnson et al., 2001), in theca cells was adopted in this study. tKrasMT female mice exhibited anovulation, absence of corpus luteum formation and infertility. These abnormities are different from those observed in previously reported granulosa-specific Kras mutants. Selective expression of KrasG12D in granulosa cells resulted in abnormal follicle growth arrest leading to premature ovarian failure and female subfertility (Fan et al., 2008b). These findings indicate that despite the universal expression of Kras in the ovary, KRAS in theca cells has distinct functions.
Dysregulated activation of KRAS is commonly associated with oncogenic cell transformation. Tissue-specific expression of KrasG12D in mice resulted in mammary gland, lung, pancreatic and endometrioid ovarian carcinogenesis (Fan et al., 2008b; Ludwig et al., 2016). However, ovarian tumors and hyperplasia in ovarian cells, including theca cells, were not observed in tKrasMT mice. Interestingly, specific activation of KrasG12D in granulosa cells or selective deletion of the tumor suppressor Pten in theca or granulosa cells did not induce sex cord-stromal tumorigenesis in mice (Fan et al., 2008a; Fan et al., 2008b; Lan et al., 2017). Theca and granulosa cells originate from the sex cord (coelomic epithelium) of the embryonic gonads. Ovarian cancers arising from these cell types are relatively rare and represent only approximately 5% of all human ovarian cancers (Richards et al., 2012). The results of the current study further support the idea that ovarian cells derived from the sex cord may be equipped with mechanisms that render them insensitive to tumorous transformation.
LH activation of the LHCGR in theca cells controls androgen biosynthesis (Richards and Ascoli, 2018). Hemorrhagic ovaries, anovulation and infertility observed in tKrasMT females are similar to that of female mice (KiLHRD582G) expressing the constitutively active mutant LHCGR (Narayan, 2015). However, unlike tKrasMT mice, KiLHRD582G females exhibited elevated androgen levels, enlarged ovaries and follicle development arrested at the preantral stage. Constitutive activation of KRAS signaling in theca cells did not significantly affect the ability of theca cells to produce androgens as revealed by normal responses to hCG-induced steroidogenic enzymes key for androgen biosynthesis, and adult tKrasMT mice maintained a comparable serum level of testosterone to that of wild-type females. Moreover, follicles at all developmental stages were present. These results suggest that the anovulatory defect in tKrasMT mice is not due to a dysfunctional LH/LHCGR signaling in theca cells.
In addition to androgens, a number of growth regulatory factors produced by theca cells are also important for normal follicle development and participate in the regulation of ovulation processes (Magoffin, 2005; Young and McNeilly, 2010; Richards et al., 2018). Interestingly, our data showed that Bmp7 expression was impressively upregulated in tKrasMT theca cells. BMP7, a member of the transforming growth factor family, has been shown to inhibit ERK1/2 activity in an autocrine/paracrine manner (Otani et al., 2007; Wang et al., 2017; Ma et al., 2019). Bmp7 is restrictedly expressed in theca cells of rodent ovaries, and its putative type I receptors and downstream effectors are present in granulosa/cumulus cells (Erickson and Shimasaki, 2003; Shimasaki et al., 2004; Edson et al., 2010). Studies have illustrated the inhibitory effects of BMP7 on granulosa cell differentiation (Shimasaki et al., 1999; Erickson and Shimasaki, 2003; Lee et al., 2004; Juengel et al., 2006) and hCG-induced ovulation in rats (Shimasaki et al., 1999; Lee et al., 2001) and oocyte maturation in mud crabs (Shu et al., 2016; Yang et al., 2018). In contrast, increased ovulation was observed in ewes carrying a mutation of a putative BMP7 receptor BMPR1B (Mulsant et al., 2001). Luteinization inhibition of BMP7 was demonstrated by BMP7 suppressing progesterone production while enhancing estrogen synthesis in preovulatory follicles (Shimasaki et al., 1999; Miyoshi et al., 2007; Zhang et al., 2015; Rajesh et al., 2018). Thus, our present and previous results support the notion that elevated Bmp7 expression in tKrasMT theca cells is at least a cause of anovulation and the lack of corpus luteal formation displayed in tKrasMT females.
The LH surge plays a central role in triggering ovulation by exquisitely orchestrating a complex intraovarian network of autocrine and paracrine pathways. Fan et al explicitly demonstrated using knockout mouse models that ERK1/2 activity in granulosa cells was essential for ovulation (Fan et al., 2009b). It is known that the ovulatory LH surge alters the expression of various genes in granulosa cells that are necessary for ovulation (Richards, 2001; Wissing et al., 2014). Constant activation of KRAS signaling in theca cells seems to play a minimal role in the maintenance of high levels of Npr2 expression and downregulation of Nrip1 expression in granulosa cells to inhibit oocyte maturation and/or ovulation. It also appears to be unlikely due to the lack of de novo production of EGF-like factors to initiate ovulation since a hyper-response of hCG-induced Areg and Btc expression was observed. Surprisingly, constant activation of KRAS in theca cells profoundly altered the expression of Cyp11a1, which is a rate-limiting factor involved in the first step of progesterone synthesis, and Cyp19a1, which is a cardinal enzyme for estrogen production, in conterminous granulosa cells in response to hCG treatment and sustained a high serum level of estrogen. The current results provide evidence that aberrantly activated KRAS stimulates Bmp7 expression in theca cells. The elevated theca-derived BMP7 acts in a paracrine manner on adjacent granulosa cells, resulting in ERK1/2 inhibition, Cyp11a1 downregulation and Cyp19a1 upregulation in response to hCG treatment. It is known that the expression of Cyp11a1 rapidly increases after the ovulatory LH surge, whereas the expression of Cyp19a1 rapidly decreases after the LH surge (Su et al., 2006; Wissing et al., 2014). The rapid changes in the expressions of these genes after the LH surge facilitate progesterone production by shifting estrogen synthesis to progesterone synthesis. This functional change in steroidogenesis plays a critical role in follicle rupture and the subsequent corpus luteal formation (Tanaka et al., 1991; Lydon et al., 1995; Okada et al., 2016). The completely reversal of hCG-induced expression of the Cyp11a1 and Cyp19a1 genes in tKrasMT granulosa cells may impair estrogen and progesterone signaling and lead to defective ovulation and the lack of corpus luteal formation. However, the mechanism by which KRAS/BMP7 signaling differentially regulates Cyp11a1, Cyp19a1, Areg and Btc expression in granulosa cells is required further investigation.
FOXO1 is a downstream target of many signaling pathways, including KRAS/ERK1/2 signaling, in nongonadal cells and granulosa cells (Huang and Tindall, 2007, 2011; Richards, 2018; Link, 2019). Although activation of KRAS signaling is able to regulate diverse downstream effectors, our data demonstrated that constitutive activation of KRAS signaling in theca cells specifically reduced phosphorylated and total FOXO1 protein levels. FOXO1 is a member of a subfamily of transcription factors, including FOXO1, FOXO3, FOXO4, and FOXO6. The functions of FOXO1 are controlled by its phosphorylation status. Phosphorylation of AKT and CREB in tKrasMT theca cells was not affected, while phosphorylation of ERK1/2 was diminished. This is possibly due to elevated expression of Dusp6, an ERK1/2-specific phosphatase, caused by persistent activation of KRAS, similar to the scenarios observed in granulosa cells and other cells (Camps et al., 2000; Fan et al., 2008b; Ekerot et al., 2008; Reizel et al., 2010; Donaubauer et al., 2016). Thus, reduced FOXO1 protein in tKrasMT theca cells is likely caused by altered ERK1/2 activity, which may trigger FOXO1 degradation (Huang and Tindall, 2011). Although Foxo1 has been reported to be mainly expressed in granulosa cells (Ting and Zelinski, 2017), the reduced FOXO1 observed in tKrasMT theca cells is unlikely to be due to the potential contamination of granulosa cells. Our reasoning is that 1) KrasG12D was deleted only in theca cells, not granulosa cells, 2) theca cells were isolated using a Percoll gradient, and granulosa cell marker genes Fshr and Cyp19a1 were undetectable by RT-PCR (data not shown), and 3) Foxo1 transcripts and protein were detected in theca cells by both RT-PCR and Western blot, respectively.
FOXO1 is a transcription factor that binds FHREs to regulate gene expression, and this activity can be influenced by AKT and ERK1/2 through a mechanism of phosphorylation and nuclear exclusion of FOXO1 (Huang and Tindall, 2011; Shen et al., 2014; Link, 2019). Bmp7 is likely a downstream target gene of FOXO1 in theca cells, since several putative FHREs were observed on the Bmp7 promoter. More importantly, FOXO1 bound to two of the putative FHREs. FOXO1 binding was attenuated in tKrasMT theca cells. Furthermore, Foxo1 impoverishment increased, whereas Foxo1 overexpression decreased, Bmp7 expression in theca cells. Together, our results suggest that upregulation of Bmp7 expression in tKrasMT theca cells is likely through a decrease in FOXO1 to alleviate the inhibition of Bmp7 promoter activity.
Taken together, our work provides the first in vivo evidence that maintaining normal KRAS activity in theca cells is essential for successful ovulation, corpus luteum formation, and female fertility. One underlying mechanism of KRAS action may be mediated through FOXO1/BMP7 signaling in theca cells. Further understanding of the regulation of FOXO1 stability, molecular and functional characterization of BMP7 controlled by KRAS signaling in ovarian theca cells, and identification of the downstream cascade(s) in granulosa cells in each ovulation event may help to determine the causes of certain types of anovulation and female infertility and to develop potential therapeutic approaches for these disorders.
Data availability statement
The original contributions presented in the study are included in the article/Supplementary Material, further inquiries can be directed to the corresponding authors.
Ethics statement
The animal study was reviewed and approved by the Animal Care and Use Committee of the University of Louisville.
Author contributions
Conceived and designed the experiments: ZL, HW, and PS. Performed the experiments: PS, Z-JL, LL, YC, KG, and XL. Analyzed the data: PS, Z-JL, YC, and XL. Contributed materials: CK. Wrote the paper: ZL, PS, and HW. All authors have read and approved the manuscript.
Funding
This work was supported in part by Department of OB/GYN research funds, University of Louisville, Louisville KY, USA.
Acknowledgments
The authors are thankful for Robin J. Lawrence’s helpful advice in the preparation and editing of this manuscript.
Conflict of interest
Z-JL is currently employed by the Company Alltech Inc.
The remaining authors declare that the research was conducted in the absence of any commercial or financial relationships that could be construed as a potential conflict of interest.
Publisher’s note
All claims expressed in this article are solely those of the authors and do not necessarily represent those of their affiliated organizations, or those of the publisher, the editors and the reviewers. Any product that may be evaluated in this article, or claim that may be made by its manufacturer, is not guaranteed or endorsed by the publisher.
Supplementary material
The Supplementary Material for this article can be found online at: https://www.frontiersin.org/articles/10.3389/fphys.2022.991719/full#supplementary-material
References
Aguirre A. J., Bardeesy N., Sinha M., Lopez L., Tuveson D. A., Horner J., et al. (2003). Activated Kras and Ink4a/Arf deficiency cooperate to produce metastatic pancreatic ductal adenocarcinoma. Genes. Dev. 17 (24), 3112–3126. doi:10.1101/gad.1158703
Anderiesz C., Fong C. Y., Bongso A., Trounson A. O. (2000). Regulation of human and mouse oocyte maturation in vitro with 6-dimethylaminopurine. Hum. Reprod. 15 (2), 379–388. doi:10.1093/humrep/15.2.379
Barbacid M. (1987). Ras genes. Annu. Rev. Biochem. 56, 779–827. doi:10.1146/annurev.bi.56.070187.004023
Bourne H. R., Sanders D. A., McCormick F. (1990). The GTPase superfamily: A conserved switch for diverse cell functions. Nature 348 (6297), 125–132. doi:10.1038/348125a0
Bridges P. J., Koo Y., Kang D. W., Hudgins-Spivey S., Lan Z. J., Xu X., et al. (2008). Generation of Cyp17iCre transgenic mice and their application to conditionally delete estrogen receptor alpha (Esr1) from the ovary and testis. Genesis 46 (9), 499–505. doi:10.1002/dvg.20428
Camps M., Nichols A., Arkinstall S. (2000). Dual specificity phosphatases: A gene family for control of MAP kinase function. FASEB J. 14 (1), 6–16. doi:10.1096/fasebj.14.1.6
Cancer Genome Atlas Research N. (2011). Integrated genomic analyses of ovarian carcinoma. Nature 474 (7353), 609–615. doi:10.1038/nature10166
Cao Y., Liu L., Lin J., Sun P., Guo K., Li S., et al. (2022). Dysregulation of Notch-FGF signaling axis in germ cells results in cystic dilation of the rete testis in mice. J. Cell. Commun. Signal. 16 (1), 75–92. doi:10.1007/s12079-021-00628-0
de Vries G., Rosas-Plaza X., van Vugt M., Gietema J. A., de Jong S. (2020). Testicular cancer: Determinants of cisplatin sensitivity and novel therapeutic opportunities. Cancer Treat. Rev. 88, 102054. doi:10.1016/j.ctrv.2020.102054
Donaubauer E. M., Law N. C., Hunzicker-Dunn M. E. (2016). Follicle-stimulating hormone (FSH)-dependent regulation of extracellular regulated kinase (ERK) phosphorylation by the mitogen-activated protein (MAP) kinase phosphatase MKP3. J. Biol. Chem. 291 (37), 19701–19712. doi:10.1074/jbc.M116.733972
Edson M. A., Nalam R. L., Clementi C., Franco H. L., Demayo F. J., Lyons K. M., et al. (2010). Granulosa cell-expressed BMPR1A and BMPR1B have unique functions in regulating fertility but act redundantly to suppress ovarian tumor development. Mol. Endocrinol. 24 (6), 1251–1266. doi:10.1210/me.2009-0461
Ekerot M., Stavridis M. P., Delavaine L., Mitchell M. P., Staples C., Owens D. M., et al. (2008). Negative-feedback regulation of FGF signalling by DUSP6/MKP-3 is driven by ERK1/2 and mediated by Ets factor binding to a conserved site within the DUSP6/MKP-3 gene promoter. Biochem. J. 412 (2), 287–298. doi:10.1042/BJ20071512
Erickson G. F., Shimasaki S. (2003). The spatiotemporal expression pattern of the bone morphogenetic protein family in rat ovary cell types during the estrous cycle. Reprod. Biol. Endocrinol. 1, 9. doi:10.1186/1477-7827-1-9
Fan H. Y., Liu Z., Cahill N., Richards J. S. (2008a). Targeted disruption of Pten in ovarian granulosa cells enhances ovulation and extends the life span of luteal cells. Mol. Endocrinol. 22 (9), 2128–2140. doi:10.1210/me.2008-0095
Fan H. Y., Liu Z., Mullany L. K., Richards J. S. (2012). Consequences of RAS and MAPK activation in the ovary: The good, the bad and the ugly. Mol. Cell. Endocrinol. 356 (1-2), 74–79. doi:10.1016/j.mce.2011.12.005
Fan H. Y., Liu Z., Paquet M., Wang J., Lydon J. P., DeMayo F. J., et al. (2009a). Cell type-specific targeted mutations of Kras and Pten document proliferation arrest in granulosa cells versus oncogenic insult to ovarian surface epithelial cells. Cancer Res. 69 (16), 6463–6472. doi:10.1158/0008-5472.CAN-08-3363
Fan H. Y., Liu Z., Shimada M., Sterneck E., Johnson P. F., Hedrick S. M., et al. (2009b). MAPK3/1 (ERK1/2) in ovarian granulosa cells are essential for female fertility. Science 324 (5929), 938–941. doi:10.1126/science.1171396
Fan H. Y., Richards J. S. (2010). Minireview: Physiological and pathological actions of RAS in the ovary. Mol. Endocrinol. 24 (2), 286–298. doi:10.1210/me.2009-0251
Fan H. Y., Shimada M., Liu Z., Cahill N., Noma N., Wu Y., et al. (2008b). Selective expression of KrasG12D in granulosa cells of the mouse ovary causes defects in follicle development and ovulation. Development 135 (12), 2127–2137. doi:10.1242/dev.020560
Furuyama T., Nakazawa T., Nakano I., Mori N. (2000). Identification of the differential distribution patterns of mRNAs and consensus binding sequences for mouse DAF-16 homologues. Biochem. J. 349 (2), 629–634. doi:10.1042/0264-6021:3490629
Han C. W., Jeong M. S., Jang S. B. (2021). Understand KRAS and the quest for anti-cancer drugs. Cells 10 (4), 842. doi:10.3390/cells10040842
Huang H., Tindall D. J. (2007). Dynamic FoxO transcription factors. J. Cell. Sci. 120 (15), 2479–2487. doi:10.1242/jcs.001222
Huang H., Tindall D. J. (2011). Regulation of FOXO protein stability via ubiquitination and proteasome degradation. Biochim. Biophys. Acta 1813 (11), 1961–1964. doi:10.1016/j.bbamcr.2011.01.007
Johnson L., Greenbaum D., Cichowski K., Mercer K., Murphy E., Schmitt E., et al. (1997). K-ras is an essential gene in the mouse with partial functional overlap with N-ras. Genes. Dev. 11 (19), 2468–2481. doi:10.1101/gad.11.19.2468
Johnson L., Mercer K., Greenbaum D., Bronson R. T., Crowley D., Tuveson D. A., et al. (2001). Somatic activation of the K-ras oncogene causes early onset lung cancer in mice. Nature 410 (6832), 1111–1116. doi:10.1038/35074129
Juengel J. L., Reader K. L., Bibby A. H., Lun S., Ross I., Haydon L. J., et al. (2006). The role of bone morphogenetic proteins 2, 4, 6 and 7 during ovarian follicular development in sheep: Contrast to rat. Reproduction 131 (3), 501–513. doi:10.1530/rep.1.00958
Kim H. J., Lee H. N., Jeong M. S., Jang S. B. (2021). Oncogenic KRAS: Signaling and drug resistance. Cancers (Basel) 13 (22), 5599. doi:10.3390/cancers13225599
Koera K., Nakamura K., Nakao K., Miyoshi J., Toyoshima K., Hatta T., et al. (1997). K-ras is essential for the development of the mouse embryo. Oncogene 15 (10), 1151–1159. doi:10.1038/sj.onc.1201284
Lan Z. J., Gu P., Xu X., Jackson K. J., DeMayo F. J., O'Malley B. W., et al. (2003). GCNF-dependent repression of BMP-15 and GDF-9 mediates gamete regulation of female fertility. EMBO J. 22 (16), 4070–4081. doi:10.1093/emboj/cdg405
Lan Z. J., Krause M. S., Redding S. D., Li X., Wu G. Z., Zhou H. X., et al. (2017). Selective deletion of Pten in theca-interstitial cells leads to androgen excess and ovarian dysfunction in mice. Mol. Cell. Endocrinol. 444, 26–37. doi:10.1016/j.mce.2017.01.043
Lee W. S., Otsuka F., Moore R. K., Shimasaki S. (2001). Effect of bone morphogenetic protein-7 on folliculogenesis and ovulation in the rat. Biol. Reprod. 65 (4), 994–999. doi:10.1095/biolreprod65.4.994
Lee W. S., Yoon S. J., Yoon T. K., Cha K. Y., Lee S. H., Shimasaki S., et al. (2004). Effects of bone morphogenetic protein-7 (BMP-7) on primordial follicular growth in the mouse ovary. Mol. Reprod. Dev. 69 (2), 159–163. doi:10.1002/mrd.20163
Li S. K., Hearn M. T. (2000). Isolation of thecal cells: An assessment of purity and steroidogenic potential. J. Biochem. Biophys. Methods 45 (2), 169–181. doi:10.1016/s0165-022x(00)00107-x
Li S., Moore A. K., Zhu J., Li X., Zhou H., Lin J., et al. (2016). Ggnbp2 is essential for pregnancy success via regulation of mouse trophoblast stem cell proliferation and differentiation. Biol. Reprod. 94 (2), 41. doi:10.1095/biolreprod.115.136358
Lin J., Lei Z. (2016). Chromatin immunoprecipitation with estrogen receptor 1 and the promoter of Greb1 in TM4 sertoli cells. Methods Mol. Biol. 1366, 67–77. doi:10.1007/978-1-4939-3127-9_7
Lin J., Zhu J., Li X., Li S., Lan Z., Ko J., et al. (2014). Expression of genomic functional estrogen receptor 1 in mouse sertoli cells. Reprod. Sci. 21 (11), 1411–1422. doi:10.1177/1933719114527355
Link W. (2019). Introduction to FOXO biology. Methods Mol. Biol. 1890, 1–9. doi:10.1007/978-1-4939-8900-3_1
Ludwig M. R., Kojima K., Bowersock G. J., Chen D., Jhala N. C., Buchsbaum D. J., et al. (2016). Surveying the serologic proteome in a tissue-specific kras(G12D) knockin mouse model of pancreatic cancer. Proteomics 16 (3), 516–531. doi:10.1002/pmic.201500133
Lydon J. P., DeMayo F. J., Funk C. R., Mani S. K., Hughes A. R., Montgomery C. A., et al. (1995). Mice lacking progesterone receptor exhibit pleiotropic reproductive abnormalities. Genes. Dev. 9 (18), 2266–2278. doi:10.1101/gad.9.18.2266
Ma H., Yuan J., Ma J., Ding J., Lin W., Wang X., et al. (2019). BMP7 improves insulin signal transduction in the liver via inhibition of mitogen-activated protein kinases. J. Endocrinol. 243 (2), 97–110. doi:10.1530/JOE-18-0693
Magoffin D. A., Erickson G. F. (1988). Purification of ovarian theca-interstitial cells by density gradient centrifugation. Endocrinology 122 (5), 2345–2347. doi:10.1210/endo-122-5-2345
Magoffin D. A. (2005). Ovarian theca cell. Int. J. Biochem. Cell. Biol. 37 (7), 1344–1349. doi:10.1016/j.biocel.2005.01.016
Malumbres M., Barbacid M. (2003). RAS oncogenes: The first 30 years. Nat. Rev. Cancer 3 (6), 459–465. doi:10.1038/nrc1097
Miyoshi T., Otsuka F., Inagaki K., Otani H., Takeda M., Suzuki J., et al. (2007). Differential regulation of steroidogenesis by bone morphogenetic proteins in granulosa cells: Involvement of extracellularly regulated kinase signaling and oocyte actions in follicle-stimulating hormone-induced estrogen production. Endocrinology 148 (1), 337–345. doi:10.1210/en.2006-0966
Mulsant P., Lecerf F., Fabre S., Schibler L., Monget P., Lanneluc I., et al. (2001). Mutation in bone morphogenetic protein receptor-IB is associated with increased ovulation rate in Booroola Merino ewes. Proc. Natl. Acad. Sci. U. S. A. 98 (9), 5104–5109. doi:10.1073/pnas.091577598
Murugan A. K., Grieco M., Tsuchida N. (2019). RAS mutations in human cancers: Roles in precision medicine. Semin. Cancer Biol. 59, 23–35. doi:10.1016/j.semcancer.2019.06.007
Nakae J., Kitamura T., Silver D. L., Accili D. (2001). The forkhead transcription factor Foxo1 (Fkhr) confers insulin sensitivity onto glucose-6-phosphatase expression. J. Clin. Investig. 108 (9), 1359–1367. doi:10.1172/JCI12876
Narayan P. (2015). Genetic models for the study of luteinizing hormone receptor function. Front. Endocrinol. 6, 152. doi:10.3389/fendo.2015.00152
Okada M., Lee L., Maekawa R., Sato S., Kajimura T., Shinagawa M., et al. (2016). Epigenetic changes of the Cyp11a1 promoter region in granulosa cells undergoing luteinization during ovulation in female rats. Endocrinology 157 (9), 3344–3354. doi:10.1210/en.2016-1264
Omerovic J., Laude A. J., Prior I. A. (2007). Ras proteins: Paradigms for compartmentalised and isoform-specific signalling. Cell. Mol. Life Sci. 64 (19-20), 2575–2589. doi:10.1007/s00018-007-7133-8
Otani H., Otsuka F., Inagaki K., Takeda M., Miyoshi T., Suzuki J., et al. (2007). Antagonistic effects of bone morphogenetic protein-4 and -7 on renal mesangial cell proliferation induced by aldosterone through MAPK activation. Am. J. Physiol. Ren. Physiol. 292 (5), F1513–F1525. doi:10.1152/ajprenal.00402.2006
Potter S. J., Kumar D. L., DeFalco T. (2016). Origin and differentiation of androgen-producing cells in the gonads. Results Probl. Cell. Differ. 58, 101–134. doi:10.1007/978-3-319-31973-5_5
Rajesh G., Mishra S. R., Paul A., Punetha M., Vidyalakshmi G. M., Narayanan K., et al. (2018). Transcriptional and translational abundance of Bone morphogenetic protein (BMP) 2, 4, 6, 7 and their receptors BMPR1A, 1B and BMPR2 in buffalo ovarian follicle and the role of BMP4 and BMP7 on estrogen production and survival of cultured granulosa cells. Res. Vet. Sci. 118, 371–388. doi:10.1016/j.rvsc.2018.04.002
Reizel Y., Elbaz J., Dekel N. (2010). Sustained activity of the EGF receptor is an absolute requisite for LH-induced oocyte maturation and cumulus expansion. Mol. Endocrinol. 24 (2), 402–411. doi:10.1210/me.2009-0267
Richards J. S., Ascoli M. (2018). Endocrine, paracrine, and autocrine signaling pathways that regulate ovulation. Trends Endocrinol. Metab. 29 (5), 313–325. doi:10.1016/j.tem.2018.02.012
Richards J. S., Fan H. Y., Liu Z., Tsoi M., Lague M. N., Boyer A., et al. (2012). Either Kras activation or Pten loss similarly enhance the dominant-stable CTNNB1-induced genetic program to promote granulosa cell tumor development in the ovary and testis. Oncogene 31 (12), 1504–1520. doi:10.1038/onc.2011.341
Richards J. S. (2018). From follicular development and ovulation to ovarian cancers: An unexpected journey. Vitam. Horm. 107, 453–472. doi:10.1016/bs.vh.2018.01.019
Richards J. S. (2001). Graafian follicle function and luteinization in nonprimates. J. Soc. Gynecol. Investig. 8 (1), S21–S23. doi:10.1016/s1071-5576(00)00100-3
Richards J. S., Ren Y. A., Candelaria N., Adams J. E., Rajkovic A. (2018). Ovarian follicular theca cell recruitment, differentiation, and impact on fertility: 2017 update. Endocr. Rev. 39 (1), 1–20. doi:10.1210/er.2017-00164
Rocks O., Peyker A., Bastiaens P. I. (2006). Spatio-temporal segregation of ras signals: One ship, three anchors, many harbors. Curr. Opin. Cell. Biol. 18 (4), 351–357. doi:10.1016/j.ceb.2006.06.007
Rotgers E., Jorgensen A., Yao H. H. (2018). At the crossroads of fate-somatic cell lineage specification in the fetal gonad. Endocr. Rev. 39 (5), 739–759. doi:10.1210/er.2018-00010
Sarkisian C. J., Keister B. A., Stairs D. B., Boxer R. B., Moody S. E., Chodosh L. A. (2007). Dose-dependent oncogene-induced senescence in vivo and its evasion during mammary tumorigenesis. Nat. Cell. Biol. 9 (5), 493–505. doi:10.1038/ncb1567
Shen M., Liu Z., Li B., Teng Y., Zhang J., Tang Y., et al. (2014). Involvement of FoxO1 in the effects of follicle-stimulating hormone on inhibition of apoptosis in mouse granulosa cells. Cell. Death Dis. 5, e1475. doi:10.1038/cddis.2014.400
Shimasaki S., Moore R. K., Otsuka F., Erickson G. F. (2004). The bone morphogenetic protein system in mammalian reproduction. Endocr. Rev. 25 (1), 72–101. doi:10.1210/er.2003-0007
Shimasaki S., Zachow R. J., Li D., Kim H., Iemura S., Ueno N., et al. (1999). A functional bone morphogenetic protein system in the ovary. Proc. Natl. Acad. Sci. U. S. A. 96 (13), 7282–7287. doi:10.1073/pnas.96.13.7282
Shiraishi K., Ascoli M. (2006). Activation of the lutropin/choriogonadotropin receptor in MA-10 cells stimulates tyrosine kinase cascades that activate ras and the extracellular signal regulated kinases (ERK1/2). Endocrinology 147 (7), 3419–3427. doi:10.1210/en.2005-1478
Shu L., Yang Y., Huang H., Ye H. (2016). A bone morphogenetic protein ligand and receptors in mud crab: A potential role in the ovarian development. Mol. Cell. Endocrinol. 434, 99–107. doi:10.1016/j.mce.2016.06.023
Su Y. Q., Nyegaard M., Overgaard M. T., Qiao J., Giudice L. C. (2006). Participation of mitogen-activated protein kinase in luteinizing hormone-induced differential regulation of steroidogenesis and steroidogenic gene expression in mural and cumulus granulosa cells of mouse preovulatory follicles. Biol. Reprod. 75 (6), 859–867. doi:10.1095/biolreprod.106.052613
Tai P., Ascoli M. (2011). Reactive oxygen species (ROS) play a critical role in the cAMP-induced activation of Ras and the phosphorylation of ERK1/2 in Leydig cells. Mol. Endocrinol. 25 (5), 885–893. doi:10.1210/me.2010-0489
Tanaka N., Espey L. L., Kawano T., Okamura H. (1991). Comparison of inhibitory actions of indomethacin and epostane on ovulation in rats. Am. J. Physiol. 260 (1), E170–E174. doi:10.1152/ajpendo.1991.260.2.E170
Ting A. Y., Zelinski M. B. (2017). Characterization of FOXO1, 3 and 4 transcription factors in ovaries of fetal, prepubertal and adult rhesus macaques. Biol. Reprod. 96 (5), 1052–1059. doi:10.1093/biolre/iox034
Wang Y., Ren S., Liu L., Yao R., Ma X., Chen L. (2017). Bone morphogenetic protein 7 alleviates paraquat-induced pulmonary fibrosis via TGF-β1/Erk1/2 pathway. Int. J. Clin. Exp. Pathol. 10 (8), 8503–8509.
Wissing M. L., Kristensen S. G., Andersen C. Y., Mikkelsen A. L., Host T., Borup R., et al. (2014). Identification of new ovulation-related genes in humans by comparing the transcriptome of granulosa cells before and after ovulation triggering in the same controlled ovarian stimulation cycle. Hum. Reprod. 29 (5), 997–1010. doi:10.1093/humrep/deu008
Yang J. Y., Zhang Y. F., Li Y. X., Guan G. P., Kong X. F., Liang A. M., et al. (2016). Effects of T-2 toxin on the regulation of steroidogenesis in mouse Leydig cells. Toxicol. Ind. Health 32 (10), 1801–1807. doi:10.1177/0748233715590516
Yang Y., Shu L., Jiang Q., Huang H., Ye H. (2018). Does the bone morphogenetic protein 7 inhibit oocyte maturation by autocrine/paracrine in mud crab? Gen. Comp. Endocrinol. 266, 119–125. doi:10.1016/j.ygcen.2018.05.004
Young J. M., McNeilly A. S. (2010). Theca: The forgotten cell of the ovarian follicle. Reproduction 140 (4), 489–504. doi:10.1530/REP-10-0094
Yuan F. P., Li X., Lin J., Schwabe C., Bullesbach E. E., Rao C. V., et al. (2010). The role of RXFP2 in mediating androgen-induced inguinoscrotal testis descent in LH receptor knockout mice. Reproduction 139 (4), 759–769. doi:10.1530/REP-09-0518
Keywords: KRAS, theca cell, Bmp7, ovulation, infertility
Citation: Sun P, Wang H, Liu L, Guo K, Li X, Cao Y, Ko C, Lan Z-J and Lei Z (2022) Aberrant activation of KRAS in mouse theca-interstitial cells results in female infertility. Front. Physiol. 13:991719. doi: 10.3389/fphys.2022.991719
Received: 11 July 2022; Accepted: 28 July 2022;
Published: 19 August 2022.
Edited by:
Kui Liu, The University of Hong Kong, ChinaReviewed by:
Yu Zhao, The University of Hong Kong, ChinaHua Zhang, China Agricultural University, China
Copyright © 2022 Sun, Wang, Liu, Guo, Li, Cao, Ko, Lan and Lei. This is an open-access article distributed under the terms of the Creative Commons Attribution License (CC BY). The use, distribution or reproduction in other forums is permitted, provided the original author(s) and the copyright owner(s) are credited and that the original publication in this journal is cited, in accordance with accepted academic practice. No use, distribution or reproduction is permitted which does not comply with these terms.
*Correspondence: Zhenmin Lei, emhlbm1pbi5sZWlAbG91aXN2aWxsZS5lZHU=; Hongliang Wang, aG9uZ2xpYW5nQGpsdS5lZHUuY24=