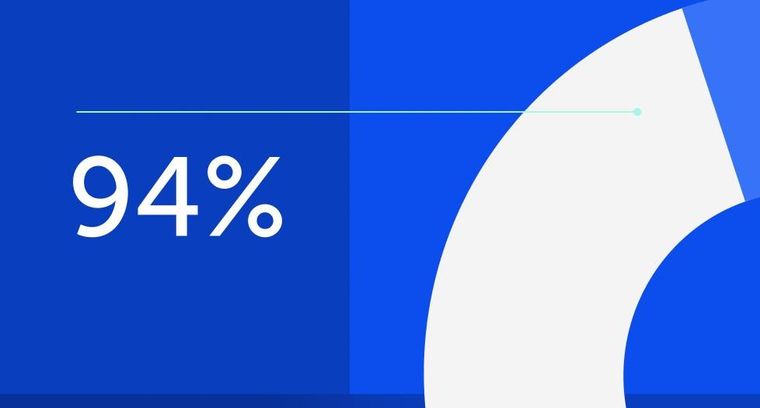
94% of researchers rate our articles as excellent or good
Learn more about the work of our research integrity team to safeguard the quality of each article we publish.
Find out more
ORIGINAL RESEARCH article
Front. Physiol., 30 August 2022
Sec. Invertebrate Physiology
Volume 13 - 2022 | https://doi.org/10.3389/fphys.2022.988907
This article is part of the Research TopicWomen in Invertebrate Physiology: 2021View all 6 articles
Despite the continuous use of chemical interventions, Aedes-borne diseases remain on the rise. Neonicotinoids are new, safer, and relatively effective pharmacological interventions against mosquitoes. Neonicotinoids interact with the postsynaptic nicotinic acetylcholine receptors (nAChRs) of the insect central nervous system, but the absence of nAChR polymorphism in resistant phenotypes makes their involvement in neonicotinoid resistance uncertain. Thus, an investigation was carried out to understand the role of metabolic detoxification and target site insensitivity in imparting acetamiprid resistance in Aedes aegypti larvae. Studies were conducted on the parent susceptible strain (PS), acetamiprid-larval selected strain for five generations (ACSF-5; 8.83-fold resistance) and 10 generations (ACSF-10; 19.74-fold resistance) of Ae. aegypti. The larval selection raised α-esterase and β-esterase activities by 1.32-fold and 1.34-fold, respectively, in ACSF-10 as compared to PS, while the corresponding glutathione-S-transferase and acetylcholinesterase activity increased by 22.5 and 2%. The ace-1 gene in PS and ACSF-10 showed four mismatches in the 1312—1511 bp region due to mutations in the Y455C codon (tyrosine to cysteine) at the 1367th position (TAC→TGC); I457V codon (isoleucine to valine) at 1372 bp and 1374 bp (ATA→GTG); and R494M codon (arginine to methionine) at 1484 bp (AGG→ATG). The R494M mutation was the novel and dominant type, observed in 70% ACSF-10 population, and has not been reported so far. The studies evidenced the combination of metabolic detoxification and target site mutation in imparting acetamiprid resistance in Ae. aegypti.
Aedes aegypti L. is a widespread disease vector posing a wide range of health risks, particularly in tropical and subtropical areas owing to favorable climatic conditions. Ever since the emergence of various Aedes-borne diseases, dengue has become a major public health concern, with reports of 390 million annual dengue infections and 96 million clinically manifested cases (Dey, 2022). Control of dengue and other Aedes-borne diseases is primarily based on Aedes management owing to the absence of successful vaccines and effective medications. Though, traditional methods, such as mosquito bed nets and window screens, are frequently used by the masses for avoiding human–mosquito contact, chemical-based control interventions are still the preferred measures due to their instant and effective actions (Liu et al., 2006; Kumar et al., 2009).
Several insecticides of different chemical nature and modes of action have been formulated and used against mosquitoes. Overutilization of these chemicals over several decades, however, has developed varying frequency and intensity of resistance in Aedes and other species of mosquitoes. Development of notable resistance to various toxicants, such as organochlorines, organophosphates, carbamates, and pyrethroids, used against Ae. aegypti and other mosquito species has been reported from different countries, such as Brazil (Lima et al., 2011), China (Li et al., 2015), Colombia (Fonseca-González et al., 2011), India (Kushwah et al., 2015), Malaysia (Ishak et al., 2015), and Thailand (Yanola et al., 2011).
Currently, neonicotinoids, synthetic derivatives of nicotine, are one of the fastest-growing and investigated insecticides against mosquitoes and are considered relatively safer as compared with conventional insecticides. Neonicotinoids interact with postsynaptic nicotinic acetylcholine receptors (nAChRs) of the insect central nervous system (Li et al., 2012). These bind strongly to a minimum of three nAChR subtypes resulting in a biphasic response causing an initial rise in the frequency of spontaneous discharge leading to nerve propagation blockage and rapid cholinergic transmission imparting toxic effects (Anadón et al., 2020). Acetamiprid, a neonicotinoid, reacts with the postsynaptic receptors of neuronal dendrites, ganglia, and muscle junctions of the central nervous system inducing contact as well as gastrointestinal toxicity (Jian-chu et al., 2002; Kimura-Kuroda et al., 2012; Sanchez-Bayo, 2012). Reports have suggested selective toxicity of acetamiprid against vertebrates due to the weak binding of receptor subtypes, non-accumulation in sediments or aquatic organisms, and comparatively eco-safe characteristics than conventional insecticides (Ambrose, 2003).
The adulticidal activity of different neonicotinoids, acetamiprid (2.3%), thiamethoxam (1.5%), and nitenpyram (2%), has been recorded against Ae. aegypti at 367 mg/m2 dosage (Darriet and Chandre 2013). Application of imidacloprid, at 84 ng/ml (LC50), as an efficient larvicide has been proposed against the local population of Ae. aegypti, without any concern for quick resistance development (Paul et al., 2006). Similarly, acetamiprid and thiamethoxam have been found efficient against laboratory strains of Ae. aegypti in Grenoble, France, but with relatively lower efficacy against resistant strains (Riaz et al., 2013).
The literature study reveals negligible reports of neonicotinoid resistance in mosquitoes (Mouhamadou et al., 2019). Selection of imidacloprid-susceptible strain of Ae. aegypti for eight generations (Imida-R) induced a moderate amount of resistance against imidacloprid (Riaz et al., 2013). Nevertheless, a few studies have reported resistance to neonicotinoids in other insects; Bemisia tabaci (Gennadius) to acetamiprid and thiamethoxam (Horowitz et al., 2004), Musca domestica to thiamethoxam (Kristensen and Jespersen, 2008), Frankliniella occidentalis (Pergande) to imidacloprid and acetamiprid (Gao et al., 2014), and Leptinotarsa decemlineata (Say) to imidacloprid (Mota-Sanchez et al., 2006). Nonetheless, like other insects, mosquitoes are also capable of developing gradual resistance to any toxicant due to alterations in biochemical and molecular levels. As a result, it is critical to estimate the possibility of resistance development to the insecticide to be recommended for mosquito management, comprehend the mechanism of resistance, and formulate the strategies to counter resistance.
It is known that metabolic detoxification of insecticide causing resistance in insects is contributed by three major enzyme groups, esterases, glutathione-S-transferases, and monooxygenases. Involvement of DDT-dehydrochlorinase in imparting glutathione-S-transferase resistance was first acknowledged in M. domestica (Clark and Shamaan, 1984) and subsequently in Anopheles and Aedes mosquitoes (Grant and Matsumura., 1988; Prapanthadara et al., 1995). Esterases are known to contribute toward organophosphate, carbamate, and pyrethroid resistance in mosquitoes while the role of monooxygenases has been reported in the metabolism of pyrethroids, organophosphates, and, to a minor extent, carbamates (Casida, 2011; IRAC, 2011; Győri et al., 2017). The development and mechanism of neonicotinoid resistance in mosquitoes have not been studied extensively. It has been suggested that presumably, these mechanisms, individually or in combination, may be the cause of neonicotinoid resistance in Ae. aegypti as the absence of nAChR polymorphism in resistant phenotypes makes the involvement of these receptors in neonicotinoid resistance uncertain (Bass et al., 2011; Kasai et al., 2014; Ilias et al., 2015).
The presence of multiple resistance mechanisms in mosquitoes may be an obstacle to the future success of mosquito control programs based on ITNs or indoor residual spraying with insecticides. Since the development of insecticide resistance is a multifaceted and vigorous process, metabolic detoxification and acetylcholinesterase target site insensitivity may play a significant role in imparting acetamiprid resistance in Ae. aegypti, because of cross-resistance to other classes of insecticide. Thus, the current study aimed to explore the causative factors involved in the development of acetamiprid resistance in Ae. aegypti larvae. Larvae of a laboratory strain of Ae. aegypti, selected with acetamiprid for 5 and 10 successive generations, possessing 8.83 and 19.74 levels of resistance, were taken for the study. Levels of metabolic detoxifying enzymes, nonspecific esterases, glutathione-S-transferase, and acetylcholinesterase, were estimated to understand their role. In addition, the involvement of acetylcholinesterase target-site insensitivity (ace-1 gene) in developing acetamiprid resistance in Ae. aegypti was estimated. It is believed that these studies may have huge implications in developing successful Aedes control tactics and resistance management strategies.
Larvae and adults of Ae. aegypti have been colonized in the rearing unit of Acharya Narendra Dev College, New Delhi, India, since 2009. Rearing conditions have been set at 28 ± 1°C temperature, 80 ± 5% relative humidity, and a 12 h:12 h (light: dark) photo-regime under sterile conditions (Warikoo et al., 2012; Samal and Kumar, 2018).
Adult Ae. aegypti are housed in netted cages (45 cm × 40 cm × 40 cm) and are provided nutrition through feeding on the juice of deseeded water-soaked raisins. Female mosquitoes are given blood meals from albino mice (procured from the rearing unit of the University of Delhi) on alternate days for at least an hour. The eggs are gathered on the Whatman filter paper strip linings of a plastic bowl filled with dechlorinated water. Larvae are hatched in a plastic tray (25 cm × 30 cm × 5 cm) filled with 1.5–2.0 L of dechlorinated water. Care has been taken not to make the trays crowded limiting to a total of 200 larvae/tray. Larvae are fed upon an artificial diet of powdered dog biscuits and active yeast (3:1 by weight) (Warikoo et al., 2012). Trays are kept free of any dirt and scum by changing the water every day.
The technical grade of acetamiprid (99.9% purity) was procured from M/s Sigma-Aldrich, India. Desired concentrations were prepared in ethanol (eMerck) and stored at 4°C. The chemicals used in the estimation of metabolic detoxifying enzymes and target site insensitivity were procured from eMerck, Qualigen, and Sigma-Aldrich.
Early fourth instars of the parent strain of Ae. aegypti were exposed to acetamiprid and LC50 and LC90 values were calculated (Samal and Kumar, 2021). The strain was selected at the early fourth instar stage by subjecting to acetamiprid selection pressure at the LC90 level, as reported in our previous studies (Samal and Kumar, 2021). The selection was carried out for ten successive generations and the resistance level to acetamiprid was estimated in each generation according to the following equation:
Following strains, selected for the current study, have been maintained in the laboratory under controlled conditions:
1) Insecticide susceptible strain of Ae. aegypti (PS) established in the year 2009 without any selection pressure of any insecticide [susceptibility to Acetamiprid: LC50 = 0.18799 mg/L; LC90 = 1.31547 mg/L]
2) Acetamiprid-selected strain of Ae. aegypti (ACSF-5) subjected to acetamiprid selection pressure at the larval stage at LC90 level for five successive generations and kept under constant selection pressure [susceptibility to acetamiprid: LC50 = 1.65916 mg/L; LC90 = 4.50887 mg/L; resistance ratio (RR) = 8.83]
3) Acetamiprid-selected strain of Ae. aegypti (ACSF-10) subjected to acetamiprid selection pressure at the larval stage at LC90 level for 10 successive generations and kept under constant selection pressure [susceptibility to acetamiprid: LC50 = 3.71057 mg/L; LC90 = 10.08811 mg/L; resistance ratio (RR) = 19.74]
The development of acetamiprid resistance in Ae. aegypti was characterized by estimating levels of various metabolic detoxification enzymes in early fourth instars. Quantification of proteins and detoxifying enzymes; α-esterase, β-esterase, glutathione-S-transferase (GST), and acetylcholinesterase (AChE); was carried out in larvae of PS, ACSF-5, and ACSF-10 strains. The standard WHO protocol to detect insecticide resistance (WHO, 1998) was used with minor modifications (Kona et al., 2018).
Individual larvae of each strain was homogenized in 200 µl of chilled autoclaved water and spun in a refrigerated microfuge at 17,000 × g for 30 s (Hanil science industrial Smart R17 micro-refrigerated centrifuge). Concentrations of proteins, esterases, and glutathione-S-transferase were measured in the supernatant, whilst the AChE level was measured in the crude homogenate. A total of five replicates were carried out, each replicate containing 20 individual larvae (n = 100), and each larva was assayed twice.
The 10 µl supernatant of each larval homogenate was taken in a microtiter plate and was added with 300 µl of the BIORAD protein reagent. The plate was incubated for 10 min and scanned at 570 nm with the help of an ELISA plate reader (Tecan i-control, infinite 200pro). A blank was run with water and a standard was run with BSA instead of the larval homogenate.
The 10 µl supernatant of each larval homogenate pipetted in the microtiter plate was supplemented with the 200 µl of 3 mM α-naphthyl acetate/β-naphthyl acetate (Sigma Aldrich) and incubated for 15 min. Postincubation, each mixture was added with 50 µl of 6.3 mM fast blue stain (freshly prepared). The visual color changes were interpreted by measuring the absorbance at 570 nm (Brogdon and Dickinson, 1983). A standard was run with respective α-naphthol/β-naphthol (Sigma Aldrich). The esterase activity was expressed as nmol of naphthol/min/mg of protein.
The 20µl supernatant of larval homogenate was added with 50 µl of 2 mM GSH and 50 µl of 1 mM of 1-chloro-2,4-nitrobenzene (CDNB) (Sigma Aldrich) in a microtitre plate. Plates were read continuously for 5 min at 340 nm (Brogdon and Barber, 1990). The larval GST activity in Ae. aegypti was expressed as nmol/min/mg protein.
Two replicates of 25 µl of crude insect homogenate taken in a microtiter plate were added with 145 µl of 0.017 M Triton X-100 and 10 µl of 0.01 M dithiobis-2-nitrobenzoic acid (DTNB) (Sigma Aldrich) solution. Subsequently, 25 µl of 0.01 M acetylthiocholine iodide (ASCHI) (Sigma Aldrich) solution was added to one replicate, while 25 µl of 0.01 M ASCHI along with 0.1 M propoxur in a 500:1 ratio was added to the other one. The reaction mixtures were incubated for 1 h and the endpoint reading was taken at 405 nm (Brogdon and Barber, 1987). The endpoint of the reaction was calculated as follows:
Percent inhibition of acetylcholinesterase = 100—(100% × Endpoint).
Data obtained were analyzed using single-way variance analysis (ANOVA) and the means were compared by Tukey’s all pairwise multiple comparison test for statistical significance at p < 0.05 using the PAWS (SPSS) software 19.0 program.
The early fourth instar larvae of Ae. aegypti of PS and ACSF-10 strains were evaluated for the possible occurrence of mutational changes in the target protein leading to acetamiprid resistance.
The genomic DNA was extracted from the early fourth instars using the nucleon spin technique (Angelini et al., 2003). Individual larva of each strain was homogenized in the lysis buffer and mixed with 95:5 of buffer GuEX and Proteinase K solution. The mixture was incubated for 15 min at 37°C and centrifuged at 12,000 × g for 4 min at room temperature. The supernatant was collected and added with the Proteinase K stock solution (10 μl), vortexed, and incubated overnight at 60–65°C. The clear supernatant was subsequently mixed with 400 μl of isopropanol, and put into the nucleoSpin Tissue Column in steps. The mixture was centrifuged at 6,000 × g for 1 min followed by the addition of 500 μl ethanol-containing TE buffer in the spin column and centrifugation at 6,000 × g for 1 min (RT). The mixture was washed twice with buffer and the flow-through was discarded. The washed column was centrifuged for 2 min at 6,000 × g (RT) to completely remove the wash buffer and placed in a 1.5 ml centrifuge tube. The DNA was eluted with 100–200 μl preheated elution buffer (70°C), incubated for 2 min and the mixture was centrifuged at 6,000 × g for 1 min (RT). Finally, the DNA was eluted in 20 μl of elution buffer. The 2.0 μl of DNA was run on a gel to check for isolation, and 2.0 μl was utilized to perform PCR quality checks.
The complete sequence of the ace-1 gene, accession no. AJ621915.1 was obtained in the FASTA format from NCBI (https://www.ncbi.nlm.nih.gov/nuccore/AJ621915.1/). The primers with 55–60°C Tm and high GC content were constructed using primer three plus software (Table 1).
The DNA sample was amplified using RT-PCR. The reaction mixture consisted 1 µl each of the purified genomic DNA, forward primer, reverse primer, and Taq polymerase, added with 3 µl of 10 mm dNTP mixture, 5 µl of 10X assay buffer, and 38 µl of autoclaved water. The constituents were thoroughly mixed in a PCR tube by a short spin at 6000 × g for 20 s followed by loading into a thermocycler (Eppendorf).
Amplified products were purified by removing unused dNTPs and primers using 5 μl of the PCR product. The ExoSAP-IT™ PCR Product Cleanup Reagent (Thermo Fisher) was used for enzymatic cleanup of PCR amplicon by hydrolysis of excess primers and nucleotides in a single step.
The amplified product was sequenced by GeneOmbio Technologies Pvt. Ltd. Baner, Pune, India. BioEdit v7.0.5 was used to edit DNA sequences (Ibis Therapeutics, Carlsbad). Nucleotide and protein blast followed by sequence alignment with Clustal W was used to determine the mutation of the AJ621915.1 ace-1 gene as well as genotype variation (MEGA five Software).
Current investigations employed early fourth instar larvae of Ae. aegypti reared in the laboratory. Susceptible strains (PS) and strains selected with acetamiprid at LC90 level for five (ACSF-5; RR = 8.83) and ten (ACSF-10; RR = 19.74) successive generations at the early fourth instar stage were investigated for biochemical and molecular characterization of acetamiprid resistance.
Corresponding to total protein in the larval body (3.8876 mg/ml), higher mean β-esterase activity (4.5040 nmol/min/mg protein) was recorded in the PS strain as compared to the mean α-esterase activity (2.6943 nmol/min/mg protein) (Table 2). Larval selection with acetamiprid for five generations did not increase the α-esterase activity significantly (p > 0.05), but increased significantly (31.6%) after 10 generations of selection (Table 2; Figure 1). In comparison, the level of β-esterases was reduced by 36.82% in ACSF-5 (p < 0.05) but elevated by 34.11% (p < 0.05) in ACSF-10 (Table 2; Figure 1).
TABLE 2. Comparative mean protein concentration and α-esterase activity in parent and acetamiprid larval-selected strains of Aedes aegypti.
FIGURE 1. Comparative protein concentration and the esterase activity in PS (parent susceptible), ACSF-5 (acetamiprid larval-selected filial-5), and ACSF-10 (acetamiprid larval-selected filial-10) strains of Ae. aegypti. Concentrations of protein/respective esterase indicated by different letters on bars are significantly different (p < 0.05); computed by one-way ANOVA followed by Tukey’s all pairwise multiple comparison test.
The frequency distribution profiles of the α-esterase activity were similar in all three strains with a single peak at 1.2 OD, however, 30% of the ACSF-10 population beyond the threshold value possessed elevated α-esterases levels (Figure 2). In comparison, β-esterase profiles of PS and ACSF-5 strains of Ae. aegypti had a single peak at OD 0.6, which shifted to OD 0.8 in ACSF-10 with 13% of the ACSF-10 population beyond the threshold value indicating an increased β-esterase activity (Figure 3).
FIGURE 2. Frequency distributions of absorbance value (570 nm) as the α-esterases activity (nmol/min/mg of protein) in the larvae of PS (parent susceptible strain), ACSF-5 (acetamiprid larval-selected filial-5), and ACSF-10 (acetamiprid larval-selected filial-10) strains of Aedes aegypti (n = 100). The susceptibility threshold is based on the maximum absorbance in the PS strain. The shaded region represents the resistant population (above the threshold). n= number of larvae; RP = resistant population.
FIGURE 3. Frequency distributions of absorbance value (570 nm) as the β-esterase activity (nmol/min/mg of protein) in the larvae of PS (parent susceptible strain), ACSF-5 (acetamiprid larval-selected filial-5), and ACSF-10 (acetamiprid larval-selected filial-10) strains of Aedes aegypti (n = 100). The susceptibility threshold is based on the maximum absorbance in the PS strain. The shaded region represents the resistant population (beyond the threshold). n= number of larvae; RP = resistant population.
The mean GST kinetics in the PS larvae showed 0.0036 absorbance. The first minute GST activity recorded was 1.8875 nmol/min/ml which gradually increased to 2.7333 nmol/min/ml (p > 0.05) after 5 min (Table 4). The selection with acetamiprid did not alter the GST activity initially (2.5208–2.5583) significantly (p > 0.05), nonetheless a significant increase (p < 0.05) was recorded after 5 min (2.5292–3.0558) with respective 5.94 and 22.49% rise in ACSF-5 and ACSF-10 in comparison to PS (Table 3; Figure 4). The frequency profiles of the GST activity demonstrated insignificant peaks at 0.18 OD and 0.2 OD in PS; a distinct peak at 0.2 OD with 7% larvae beyond the threshold in ACSF-5 and non-prominent, the highest point at 0.2 OD in ACSF-10 (Figure 5).
TABLE 4. Mean percent activity and percent inhibition of the AChE activity in parent and acetamiprid-selected larvae of Aedes aegypti.
FIGURE 4. Comparative absorbance/min and the GST activity in PS (parent susceptible), ACSF-5 (acetamiprid larval-selected filial-5), and ACSF-10 (acetamiprid larval-selected filial-10) strains of Aedes aegypti (n = 100). The absorbance/GST activity indicated by different letters on bars are significantly different (p < 0.05); computed by one-way ANOVA followed by Tukey’s all pairwise multiple comparison test.
FIGURE 5. Frequency distribution of absorbance value (340 nm) as the GST activity in the larvae of PS (parent susceptible strain), ACSF-5 (acetamiprid larval-selected filial-5), and ACSF-10 (acetamiprid larval-selected filial-10) strains of Aedes aegypti (n = 100). The susceptibility threshold is based on the maximum absorbance in the PS strain. The shaded region represents the resistant population (beyond the threshold). n= number of larvae; RP = resistant population.
The endpoint of the AChE activity in PS, ACSF-5, and ACSF-10 ranged from 0.3801 to 0.4093 OD (p < 0.05) (Table 5). The mean %AChE inhibition was in the range of 38% in PS to 41% in ACSF-10 (p < 0.05) inducing %AChE activity from 62% in PS to 59% in ACSF-10 (Table 4; Figure 6). The ACSF-5 and ACSF-10 registered 0.18 and 2.92% reduced inhibition of AChE activity, respectively, in comparison to the PS larvae. The maximum population frequency was observed at 0.3 OD in PS and 0.5 OD in ACSF-5. In comparison, ACSF-10 displayed a distinct peak at 0.4 OD with a broad and flat area from 0.6 to 0.8 OD, and 37% of larvae present beyond the susceptible threshold (Figure 7).
TABLE 5. Analysis of genotype and phenotype correlation of G1484 T mutation in protein sequence of Aedes aegypti based on the mutation frequency in the DNA sequence alignment.
FIGURE 6. Comparative percent inhibition of the AChE activity in PS (parent susceptible), ACSF-5 (acetamiprid larval-selected filial-5), and ACSF-10 (acetamiprid larval-selected filial-10) strains of Aedes aegypti (n = 100). The AChE inhibition/activity indicated by the same letter on bars are not significantly different (p < 0.05); computed by one-way ANOVA followed by Tukey’s all pairwise multiple comparison test.
FIGURE 7. Frequency distribution of the absorbance values of AChE activity inhibition in PS (parent susceptible), ACSF-5 (acetamiprid larval-selected filial-5), and ACSF-10 (acetamiprid larval-selected filial-10) strains of Aedes aegypti (n = 100). The susceptibility threshold is based on maximum absorbance in PS strain. The shaded region represents the resistant population (beyond the threshold). n= number of larvae; RP = resistant population.
The amplified genomic DNA of PS and ACSF-10 yielded a PCR amplicon of 528 bp (Table 1). The sequences obtained were submitted to GENBANK with accession numbers MW013053 and MW013054. Nucleotide sequences of the ace-1 gene of PS and ACSF-10 aligned with the sequence of the Aedes aegypti Rock Strain (Accession No. AJ621915.1) is depicted in Figure 8.
FIGURE 8. Alignment of nucleotide sequence of ace-1 gene of PS (parent susceptible) and ACSF-10 (acetamiprid larval-selected filial-10) strains with rock strain of Aedes aegypti (accession no. AJ621915.1) using Clustal W (MEGA five software) (n = 50).
The ace-1 gene sequence comparison of PS and ACSF-10 showed four mismatches in the 1312 bp to 1511 bp region. Three mismatches were due to adenine being replaced by guanine (A → G; A1367G, A1372G, and A1374G), while the fourth was because of the replacement of guanine by thymine (G → T; G1484T) (Figure 8). Posttranslational analysis of open reading frames (ORFs) showed respective mutations in the Y455C codon (Tyrosine to Cysteine) at the 1367th position (TAC → TGC); in the I457V codon (Isoleucine to Valine) at 1372 bp and 1374 bp (ATA → GTG); and in the R494M codon (Arginine to Methionine) at 1484 bp (AGG to ATG) (Figure 9).
FIGURE 9. Alignment of translated protein sequence of ace-1 gene in PS (parent susceptible) and ACSF-10 (acetamiprid larval-selected filial-10) strains with the rock strain of Aedes aegypti (accession No.—AJ621915.1) using Clustal W (MEGA five software) (n = 50).
Among these mutations, the R494M mutation was observed as a novel and dominant mutation in ACSF-10 present in 70% of the ACSF-10 population, as indicated by the correlation between genotype and phenotype (susceptibility status) of the G1484T codon in Ae. aegypti (Table 5).
Vector control strategies, with the introduction of new interventions, go beyond mosquito nets and indoor residual spray taking into account local specificities. Though the application of larvicides is a significant means in mosquito control programs, its implementation involves complicated logistics and efforts leading to the utilization of alternates or novel chemicals. Thus, the present study investigated a neonicotinoid, acetamiprid, for the management of an Indian strain of Ae. aegypti, and assessed the mechanism of resistance development in Ae. aegypti against acetamiprid in order to devise a strategy to deal with the problem.
Insecticide resistance, considered a preadaptive phenomenon, has emerged as the greatest hindrance to controlling disease vectors. The prolonged and frequent usage of insecticides in crop fields, residential areas, and in public health programs has led to the development of insecticide resistance in mosquitoes by selecting resistant while eliminating susceptible individuals (Uragayala et al., 2015). It is suggested that insects that get selected post-insecticide exposure survive the stress due to altered genome and carry the genetic variance to the successive generation contributing to the resistance gene pool (Faucon et al., 2015). Gradual and sequential selection increase the proportion of resistant organisms which finally outweigh the susceptible population.
Although mechanisms involved in imparting insecticide resistance in vectors are similar across all vector taxa, yet each kind of resistance is unique and comprises a multifaceted resistance foci pattern. Thus, surveillance of vector populations and assessment of their susceptibility to insecticides is significant to design effective control programs (Brogdon and McAllister, 1998). Monitoring insecticide resistance and identifying the underlying mechanism(s) becomes crucial in targeting resistant heterozygotes/homozygotes in the field to manage the mosquito population. The literature though reports the occurrence of increased tolerance of neonicotinoids in the lepidopterans and hemipterans due to their overuse in the fields (Brengues et al., 2003; Ponlawat and Harrington, 2005; Khan and Akram, 2019), yet the resistance, specifically acetamiprid, has not been investigated in mosquitoes. The Arthropod Pesticide Resistance Database (APRD) lists more than 500 cases of resistance to neonicotinoids but not a single report against Aedes (Mota-Sanchez and Wise, 2022). Thus, an investigation was conducted to understand acetamiprid resistance development in Ae. aegypti which resulted in 19.74-fold resistance after 10 generations of successive selection of the parent strain at the larval stage (Samal et al., 2022).
Resistance to neonicotinoids, like any other insecticide, involves either a target site modification due to a gene polymorphism, or an increase in the insecticide degradation by the action of rising titers of metabolic enzymes (Bass et al., 2011; Kasai et al., 2014; Yang and Liu, 2014). Neonicotinoids are known to target acetylcholine receptors (AChR) in insects, however, their involvement in inducing neonicotinoid resistance remains undefined due to the absence of nAChR polymorphism in resistant phenotypes (Ilias et al., 2015). The metabolism-based mechanisms, involving esterases, CYP450s, and glutathione-S-transferase (GSTs) combined with insensitive AChE have been reported in the development of pyrethroid resistance in mosquitoes (Safi et al., 2017). Thus, the current study investigated the possible role of metabolic detoxification enzymes; ɑ-esterases and β-esterases, glutathione-S-transferase and acetylcholinesterase in the development of acetamiprid resistance in Ae. aegypti.
Esterases, a group of heterogeneous enzymes, are present in most organisms. The amplification and/or occasional overexpression of esterase genes increases the production of detoxification proteins negating the effects of toxicants (Vaughan and Hemingway, 1995; Raymond et al., 1998). Continuous selection pressure of acetamiprid increased the ɑ-esterase activity by 1.32-fold and β-esterase by 1.34-fold in ACSF-10 as compared to the susceptible strain suggesting their significant role in acetamiprid hydrolysis and detoxification. Studies implicating the role of esterases in inducing resistance in mosquitoes to different groups of insecticides, primarily pyrethroids and except neonicotinoids, are available. The elevated esterase activity has been reported in deltamethrin-resistant and permethrin-resistant Ae. aegypti, in Guerrero State, Mexico (Aponte et al., 2013). However, the insignificant role of esterases in causing pyrethroid resistance has been reported in an Indian strain of Ae. aegypti (Sahgal et al., 1994). In contrast, similar results demonstrating elevated esterase levels in Ae. aegypti have been reported in north-east Thailand and south-east Asia (Amelia-Yap et al., 2018). Interestingly, in a Santiago de Cuban strain of Ae. aegypti, a rise in the esterase frequency from 0.12 to 0.63 was recorded after six generations of deltamethrin selection, which reduced to 0.38 after 12 generations of selection (Rodriguez et al., 2002).
The current study also investigates the role of glutathione-S-transferase in imparting acetamiprid resistance in Ae. aegypti. The assay showed a1.22-fold increased GST activity in the ACSF-10 strain and a 1.1-fold increase in the ACSF-5 strain of Ae. aegypti. Several studies have shown elevated levels of glutathione S-transferase in insecticide-resistant insects suggesting their role in inducing resistance; most of the studies implicated elevated levels in DDT-resistant mosquitoes (Grant, 1991; Grant and Hammock, 1992; Gunasekaran et al., 2011). The involvement of GST in imparting acetamiprid resistance in Ae. aegypti is significant and needs to be investigated further. In addition to esterases and GSTs, the ACSF-10 strain of Ae. aegypti showed 2.92% decreased AChE inhibition whereas the ACSF-5 strain had 0.18% decreased AChE inhibition and, thus, increased the AChE activity. Inhibited AChE decreases the sensitivity of insecticides in insects helping in resistance development (Ayad and Georghiou 1975; Hemingway and Georghiou, 1983).
These results suggest differential involvement of esterases, glutathione-S-transferases, and AChE in the development of acetamiprid resistance in Ae. aegypti indicating a multifactorial resistance mechanism. The fluctuating detoxifying enzyme level suggests the correlation between selected mechanisms and the metabolic resistance which may be reversed when insecticide pressure ceases. Earlier studies have suggested the role of monooxygenases in the development of acetamiprid resistance in Ae. aegypti which can be reversed by the use of Piperonyl butoxide as a synergist (Samal et al., 2022).
Apart from the involvement of detoxifying enzymes in imparting resistance, alteration in the target site is also one of the potential and major mechanisms involved with the resistance. The study, thus, examined the target-site insensitivity for acetylcholinesterase (ace-1 gene) in the ACSF-10 strain revealing mutations in ace-I which is considered a target of majorly the organophosphates. The results showed the occurrence of mutations in the Arg → Met (R494M) codon, Tyr → Cys (Y555C) codon, and Iso → Val (I457V) of the ACSF-10 strain of Ae. aegypti which possibly caused the target site insensitivity leading to the development of resistance. Different species of mosquitoes exhibit different mutations linked to the development of insecticide resistance. Higher expression and mutation in the ace-1 gene encoding the acetylcholinesterase enzyme (AChE1) has imparted OP and carbamate resistance in An. gambiae and Cx. pipiens (Alout et al., 2008). In contrast, ace-1 gene mutations associated with an insensitive AChE were not observed in Ae. aegypti (Weill et al., 2002).
The report of a single mutation (G119S) associated with insecticide resistance exists in Cx. pipiens and An. gambiae (Weill et al., 2004). The most common resistance mutation observed in Cx. pipiens is G119S (GGC → AGC) located near the catalytic site of the ace-1 gene imparting high insensitivity to carbamates (Weill et al., 2003) by reducing the AChE1 activity in cholinergic synapses (Alout et al., 2008). The Gly → Ser (G119S) substitution has also been reported in other Culex species, Cx. vishnui and Cx. quinquefasciatus, for organophosphate resistance (Weill et al., 2002; Weill et al., 2003; Liu et al., 2006). In contrast, the G119S mutation was not observed in An. stephensi resistant to temephos (Soltani et al., 2015). It has been proposed that possibility of the G119S mutation occurrence in Ae. aegypti is low because of two independent mutations (C→ A and C→G) in DNA (Weill et al., 2003). It is also suggested that the maintenance of polymorphic variation in genes may be due to the duplication of ace-1. Studies about the maintenance of polymorphism/variation of genotypes in the population have been reported in Cx. pipiens (Labbé et al., 2007) and An. gambiae (Djogbenou et al., 2009).
These mechanisms, either separately or together, are thought to constitute the root of the development of cross-resistance in ACSF strains of Ae. aegypti. Although acetylcholine receptors (nAChR) are known to be a target for neonicotinoids in insects, the role of these receptors in neonicotinoid resistance is unclear because resistant phenotypes lack nAChR polymorphism (Ilias et al., 2015). The role of AChE in conferring acetamiprid resistance in Ae. aegypti may be related to the development of cross-resistance to the related class of insecticide as the development of insecticide resistance is a diverse and active process. The current study is the first report on the molecular mechanism of acetamiprid resistance in Ae. aegypti based on mutations in ace-1. The Arg → Met (R494) mutation observed in the ACSF-10 strain of Ae. aegypti was a dominant type and has never been observed earlier. It is proposed that these mutations causing variation in genotypes and translated products supplemented with elevated detoxifying enzymes led to the development of resistance to neonicotinoid. The AChE-insensitive mechanism, together with an overproduced esterase-based mechanism has been found to induce organophosphate and carbamate resistance in a Cuban strain of Cx. quinquefasciatus as compared to resistance imparted by individual mechanisms (Bisset et al., 1990).
These findings suggest that acetamiprid resistance in Ae. aegypti is a multidimensional and dynamic process that is influenced by a variety of factors. The increased prevalence of resistant Ae. aegypti recommends the use of synergists to reverse the resistance or rotation of pesticides with different mechanisms of action to prevent the establishment of resistant homozygotes in the field.
The rising prevalence of insecticide-resistant Ae. aegypti necessitates comprehensive insecticide resistance management for which there is a need to understand the mechanism involved. Current findings assessed the acetamiprid resistance mechanism in Ae. aegypti which point to the possible involvement of metabolic detoxifying enzymes supplemented with target site insensitivity (ace-1) that caused AGG to ATG mutations at 1484 bp. This study suggests the multifactorial resistance mechanism contributing toward acetamiprid resistance, a complex and dynamic process. These outcomes could aid in understanding and devising mosquito management strategies.
The datasets presented in this study can be found in online repositories. The names of the repository/repositories and accession number(s) can be found in the article/Supplementary Material.
RS conceived the idea, conducted experiments, and wrote the manuscript. SK designed and guided the experiments. RS, KP, and PL analyzed results, and SK helped in the analysis. RS and SK were involved in the finalization of the manuscript.
This research was supported by the contingent grant from the Council of Scientific and Industrial Research, New Delhi, India [Award No. 08/529 (0003)/2015-EMR-I].
The authors are highly grateful to the Council of Scientific Research (CSIR), New Delhi, India, for providing financial assistance to carry out the present investigations. They are also highly grateful to the Principal, Acharya Narendra Dev College, for providing infrastructure and research facilities.
The authors declare that the research was conducted in the absence of any commercial or financial relationships that could be construed as a potential conflict of interest.
All claims expressed in this article are solely those of the authors and do not necessarily represent those of their affiliated organizations, or those of the publisher, the editors, and the reviewers. Any product that may be evaluated in this article, or claim that may be made by its manufacturer, is not guaranteed or endorsed by the publisher.
Alout H., Djogbénou L., Berticat C., Chandre F., Weill M. (2008). Comparison of Anopheles gambiae and Culex pipiens acetylcholinesterase 1 biochemical properties. Comp. Biochem. Physiol. B Biochem. Mol. Biol. 150, 271–277. doi:10.1016/j.cbpb.2008.03.008
Ambrose M. L. (2003) Characterization of the insecticidal properties of acetamiprid under field and laboratory conditions. Available at: http://www.lib.ncsu.edu/resolver/1840.16/624 (Accessed June 27, 2022)
Amelia-Yap Z. H., Chen C. D., Sofian-Azirun M., Low V. L. (2018). Pyrethroid resistance in the dengue vector Aedes aegypti in southeast asia: present situation and prospects for management. Parasit. Vectors 11, 332–417. doi:10.1186/s13071-018-2899-0
Anadón A., Ares I., Martínez M., Martínez-Larrañaga M. R., Martínez M. A. (2020). Neurotoxicity of neonicotinoids” in: Advances in neurotoxicology. Cambridge, Massachusetts: Academic Press, 167–207.
Angelini A., Di Febbo C., Baccante G., Di Nisio M., Di Ilio C., Cuccurullo F., et al. (2003). Identification of three genetic risk factors for venous thrombosis using a multiplex allele-specific PCR assay: comparison of conventional and new alternative methods for the preparation of DNA from clinical samples. J. Thromb. Thrombolysis 16, 189–193. doi:10.1023/B:THRO.0000024057.37588.2a
Aponte H. A., Penilla R. P., Dzul-Manzanilla F., Che-Mendoza A., López A. D., Solis F., et al. (2013). The pyrethroid resistance status and mechanisms in Aedes aegypti from the Guerrero state, Mexico. Pestic. Biochem. Physiol. 107, 226–234. doi:10.1016/j.pestbp.2013.07.005
Ayad H., Georghiou G. P. (1975). Resistance to organophosphates and carbamates in Anopheles albimanus based on reduced sensitivity to acetylcholinesterase. J. Econ. Entomol. 68, 295–297. doi:10.1093/jee/68.3.295
Bass C., Puinean A. M., Andrews M., Cutler P., Daniels M., Elias J., et al. (2011). Mutation of a nicotinic acetylcholine receptor β subunit is associated with resistance to neonicotinoid insecticides in the aphid Myzus persicae. BMC Neurosci. 12, 51. doi:10.1186/1471-2202-12-51
Bisset J. A., Rodriguez M. M., Diaz C., Ortiz E., Marquetti M. C., Hemingway J. (1990). The mechanisms of organophosphate and carbamate resistance in Culex quinquefasciatus (Diptera: Culicidae) from Cuba. Cuba. Bull. Entomol. Res. 80, 245–250. doi:10.1017/s0007485300050434
Brengues C., Hawkes N. J., Chandre F., McCarroll L., Duchon S., Guillet P., et al. (2003). Pyrethroid and DDT cross resistance in Aedes aegypti is correlated with novel mutations in the voltage gated sodium channel gene. Med. Vet. Entomol. 17, 87–94. doi:10.1046/j.1365-2915.2003.00412.x
Brogdon W. G., Barber A. M. (1987). Microplate assay of acetylcholinesterase inhibition kinetics in single mosquito homogenates. Pestic. Biochem. Physiol. 29, 252–259. doi:10.1016/0048-3575(87)90155-6
Brogdon W. G., Barber A. M. (1990). Microplate assay of glutathione-S-transferase activity for resistance detection in single mosquito triturates. Comp. Biochem. Physiol. B 96, 339–342. doi:10.1016/0305-0491(90)90385-7
Brogdon W. G., Dickinson C. M. (1983). A microassay system for measuring esterase activity and protein concentration in small samples and in high-pressure liquid chromatography eluate fractions. Anal. Biochem. 131, 499–503. doi:10.1016/0003-2697(83)90204-x
Brogdon W. G., McAllister J. C. (1998). Insecticide resistance and vector control. Emerg. Infect. Dis. 4, 605–613. doi:10.3201/eid0404.980410
Casida J. E. (2011). Neonicotinoid metabolism: compounds, substituents, pathways, enzymes, organisms, and relevance. J. Agri. Food. Chem. 59, 2923–2931. doi:10.1021/jf102438c
Clark A. G., Shamaan N. A. (1984). Evidence that DDT-dehydrochlorinase from the house fly is a glutathione S-transferase. Pestic. Biochem. Physiol. 22, 249–261. doi:10.1016/0048-3575(84)90018-x
Darriet F., Chandre F. (2013). Efficacy of six neonicotinoid insecticides alone and in combination with deltamethrin and piperonyl butoxide against pyrethroid-resistant Aedes aegypti and Anopheles gambiae (Diptera: Culicidae). Pest Manag. Sci. 69, 905–910. doi:10.1002/ps.3446
Dey T. (2022). “Dynamics of disease diffusion: a critical analysis of dengue outbreak in kolkata and adjacent areas,” in Livelihood enhancement through agriculture, tourism and health (Singapore: Springer), 407–423.
Djogbenou L., Labbe P., Chandre N., Pasteur N., Weill M. (2009). Ace-I duplication in Anopheles gambiae: a challenge for malaria control. Malar. J. 8, 70. doi:10.1186/1475-2875-8-70
Faucon F., Dusfour I., Gaude T., Navratil V., Boyer F., Chandre F., et al. (2015). Identifying genomic changes associated with insecticide resistance in the dengue mosquito Aedes aegypti by deep targeted sequencing. Genome Res. 25, 1347–1359. doi:10.1101/gr.189225.115
Fonseca-González I., Quiñones M. L., Lenhart A., Brogdon W. G. (2011). Insecticide resistance status of Aedes aegypti (L.) from Colombia. Pest Manag. Sci. 67, 430–437. doi:10.1002/ps.2081
Gao C. F., Ma S. Z., Shan C. H., Wu S. F. (2014). Thiamethoxam resistance selected in the western flower thrips Frankliniella occidentalis (thysanoptera: Thripidae): cross-resistance patterns, possible biochemical mechanisms and fitness costs analysis. Pestic. Biochem. Physiol. 114, 90–96. doi:10.1016/j.pestbp.2014.06.009
Grant D. F. (1991). Evolution of glutathione S-transferase subunits in culicidae and related nematocera: electrophoretic and immunological evidence for conserved enzyme structure and expression. Insect Biochem. 21, 435–445. doi:10.1016/0020-1790(91)90010-c
Grant D. F., Hammock B. D. (1992). Genetic and molecular evidence for a trans-acting regulatory locus controlling glutathione S-transferase-2 expression in Aedes aegypti. Mol. Gen. Genet. 234, 169–176. doi:10.1007/BF00283836
Grant D. F., Matsumura F. (1988). Glutathione S-transferase-1 in Aedes aegypti larvae. purification and properties. Insect. Biochem. 18, 615–622. doi:10.1016/0020-1790(88)90014-5
Gunasekaran K., Muthukumaravel S., Sahu S. S., Vijayakumar T., Jambulingam P. (2011). Glutathione S transferase activity in Indian vectors of malaria: a defense mechanism against DDT. J. Med. Entomol. 48, 561–569. doi:10.1603/me10194
Győri J., Farkas A., Stolyar O., Székács A., Mörtl M., Vehovszky Á. (2017). Inhibitory effects of four neonicotinoid active ingredients on acetylcholine esterase activity. Acta. Biol. hung. 68, 345–357. doi:10.1556/018.68.2017.4.1
Hemingway J., Georghiou G. P. (1983). Studies on the acetylcholinesterase of Anopheles albimanus resistant and susceptible to organophosphate and carbamate insecticides. Pestic. Biochem. Physiol. 19, 167–171. doi:10.1016/0048-3575(83)90136-0
Horowitz A. R., Kontsedalov S., Ishaaya I. (2004). Dynamics of resistance to the neonicotinoids acetamiprid and thiamethoxam in Bemisia tabaci (Homoptera: Aleyrodidae). J. Econ. Entomol. 97, 2051–2056. doi:10.1093/jee/97.6.2051
Ilias A., Lagnel J., Kapantaidaki D. E., Roditakis E., Tsigenopoulos C. S., Vontas J., et al. (2015). Transcription analysis of neonicotinoid resistance in Mediterranean (MED) populations of B. tabaci reveal novel cytochrome P450s, but no nAChR mutations associated with the phenotype. BMC genomics 16, 939–1023. doi:10.1186/s12864-015-2161-5
IRAC (2011). Prevention and management of insecticide resistance in vectors of medical health importance. Man. by Insectic. Res. Action Comm., 1–72.
Ishak I. H., Jaal Z., Ranson H., Wondji C. S. (2015). Contrasting patterns of insecticide resistance and knockdown resistance (kdr) in the dengue vectors Aedes aegypti and Aedes albopictus from Malaysia. Parasit. Vectors 8, 181–213. doi:10.1186/s13071-015-0797-2
Jian-chu M., Tian-ci Y., Jia-an C., Xiao-gang S. (2002). Lethal and sublethal effects of acetamiprid on the larvae of Culex pipiens pallens. Insect Sci. 9, 45–49. doi:10.1111/j.1744-7917.2002.tb00153.x
Kasai S., Komagata O., Itokawa K., Shono T., Ng L. C., Kobayashi M., et al. (2014). Mechanisms of pyrethroid resistance in the dengue mosquito vector, Aedes aegypti: target site insensitivity, penetration, and metabolism. PLoS Negl. Trop. Dis. 8 (6), e2948. doi:10.1371/journal.pntd.0002948
Khan H. A. A., Akram W. (2019). Resistance status to deltamethrin, permethrin, and temephos along with preliminary resistance mechanism in Aedes aegypti (Diptera: Culicidae) from Punjab, Pakistan. J. Med. Entomol. 56, 1304–1311. doi:10.1093/jme/tjz057
Kimura-Kuroda J., Komuta Y., Kuroda Y., Hayashi M., Kawano H. (2012). Nicotine-like effects of the neonicotinoid insecticides acetamiprid and imidacloprid on cerebellar neurons from neonatal rats. PLoS One 7, e32432. doi:10.1371/journal.pone.0032432
Kona M. P., Kamaraju R., Donnelly M. J., Bhatt R. M., Nanda N., Chourasia M. K., et al. (2018). Characterization and monitoring of deltamethrin-resistance in Anopheles culicifacies in the presence of a long-lasting insecticide-treated net intervention. Malar. J. 17, 414–512. doi:10.1186/s12936-018-2557-1
Kristensen M., Jespersen J. B. (2008). Susceptibility to thiamethoxam of Musca domestica from danish livestock farms. Pest Manag. Sci. 64, 126–132. doi:10.1002/ps.1481
Kumar S., Thomas A., Samuel T., Sahgal A., Verma A., Pillai M. K. K. (2009). Diminished reproductive fitness associated with the deltamethrin resistance in an indian strain of dengue vector mosquito, Aedes aegypti L. Trop. Biomed. 26, 155–164.
Kushwah R. B. S., Dykes C. L., Kapoor N., Adak T., Singh O. P. (2015). Pyrethroid-resistance and presence of two knockdown resistance (kdr) mutations, F1534C and a novel mutation T1520I, in Indian Aedes aegypti. PLoS Negl. Trop. Dis. 9 (1), e3332. doi:10.1371/journal.pntd.0003332
Labbé P., Berthomieu A., Berticat C., Alout H., Raymond M., Lenormand T., et al. (2007). Independent duplications of the acetylcholinesterase gene conferring insecticide resistance in the mosquito Culex pipiens. Mol. Biol. Evol. 24, 1056–1067. doi:10.1093/molbev/msm025
Li C. X., Kaufman P. E., Xue R. D., Zhao M. H., Wang G., Yan T., et al. (2015). Relationship between insecticide resistance and kdr mutations in the dengue vector Aedes aegypti in Southern China. Parasit. Vectors 8, 325–329. doi:10.1186/s13071-015-0933-z
Li T., Zhang L., Reid W. R., Xu Q., Dong K., Liu N. (2012). Multiple mutations and mutation combinations in the sodium channel of permethrin resistant mosquitoes, Culex quinquefasciatus. Sci. Rep. 2, 781–789. doi:10.1038/srep00781
Lima E. P., Paiva M. H. S., de Araújo A. P., da Silva É. V. G., da Silva U. M., de Oliveira L. N., et al. (2011). Insecticide resistance in Aedes aegypti populations from Ceará, Brazil. Parasit. Vector 4, 5–12. doi:10.1186/1756-3305-4-5
Liu N., Xu Q., Zhu F., Zhang L. E. E. (2006). Pyrethroid resistance in mosquitoes. Insect Sci. 13, 159–166. doi:10.1111/j.1744-7917.2006.00078.x
Mota-Sanchez D., Hollingworth R. M., Grafius E. J., Moyer D. D. (2006). Resistance and cross-resistance to neonicotinoid insecticides and spinosad in the colorado potato beetle, Leptinotarsa decemlineata (Say) (Coleoptera: Chrysomelidae). Pest Manag. Sci. 62, 30–37. doi:10.1002/ps.1120
Mota-Sanchez D., Wise J. C. (2022). Arthropod pesticide resistance Database (APRD). East Lansing, Michigan: Michigan State University. Available at: https://www.pesticideresistance.org/search.php (Assessed on 03 17, 2022).
Mouhamadou C. S., de Souza S. S., Fodjo B. K., Zoh M. G., Bli N. K., Koudou B. G. (2019). Evidence of insecticide resistance selection in wild Anopheles coluzzii mosquitoes due to agricultural pesticide use. Infect. Dis. Poverty 8 (1), 64–68. doi:10.1186/s40249-019-0572-2
Paul A., Harrington L. C., Scott J. G. (2006). Evaluation of novel insecticides for control of dengue vector Aedes aegypti (Diptera: Culicidae). J. Med. Entomol. 43, 55–60. doi:10.1603/0022-2585(2006)043[0055:EONIFC]2.0.CO;2
Ponlawat A., Harrington L. C. (2005). Blood feeding patterns of Aedes aegypti and Aedes albopictus in Thailand. J. Med. Entomol. 42, 844–849. doi:10.1093/jmedent/42.5.844
Prapanthadara L. A., Hemingway J., Ketterman A. J. (1995). DDT-resistance in Anopheles gambiae Giles from Zanzibar Tanzania, based on increased DDT-dehydrochlorinase activity of glutathione S-transferases. Bull. Entomol. Res. 85, 267–274. doi:10.1017/s0007485300034350
Raymond M., Chevillon C., Guillemaud T., Lenormand T., Pasteur N. (1998). An overview of the evolution of overproduced esterases in the mosquito Culex pipiens. Philos. Trans. R. Soc. Lond. B Biol. Sci. 353, 1707–1711. doi:10.1098/rstb.1998.0322
Riaz M. A., Chandor-Proust A., Dauphin-Villemant C., Poupardin R., Jones C. M., Strode C., et al. (2013). Molecular mechanisms associated with increased tolerance to the neonicotinoid insecticide imidacloprid in the dengue vector Aedes aegypti. Aquat. Toxicol. 126, 326–337. doi:10.1016/j.aquatox.2012.09.010
Rodriguez M. M., Bisset J., Ruiz M., Soca A. (2002). Cross-resistance to pyrethroid and organophosphate insecticides induced by selection with temephos in Aedes aegypti (Diptera: Culicidae) from Cuba. J. Med. Entomol. 39, 882–888. doi:10.1603/0022-2585-39.6.882
Safi N. H. Z., Ahmadi A. A., Nahzat S., Ziapour S. P., Nikookar S. H., Fazeli-Dinan M., et al. (2017). Evidence of metabolic mechanisms playing a role in multiple insecticides resistance in Anopheles stephensi populations from Afghanistan. Malar. J. 16, 100–110. doi:10.1186/s12936-017-1744-9
Sahgal A., Kumar S., Pillai M. K. K. (1994). Microplate assay of elevated esterase activity in individual pyrethroid-resistant mosquitoes. J. Biosci. 19, 193–199. doi:10.1007/bf02703054
Samal R. R., Kumar S. (2021). Cuticular thickening associated with insecticide resistance in dengue vector, Aedes aegypti L. Int. J. Trop. Insect Sci. 41, 809–820. doi:10.1007/s42690-020-00271-z
Samal R. R., Kumar S. (2018). Susceptibility status of Aedes aegypti L. against different classes of insecticides in New Delhi, India to formulate mosquito control strategy in fields. Open Parasitol. J. 6, 52–62. doi:10.2174/1874421401806010052
Samal R. R., Panmei K., Lanbiliu P., Kumar S. (2022). Reversion of CYP450 monooxygenase-mediated acetamiprid larval resistance in dengue fever mosquito, Aedes aegypti L. Bull. Entomol. Res. 1, 557–566. doi:10.1017/S0007485321001140
Sanchez-Bayo F. P. (2012). Insecticides mode of action in relation to their toxicity to non-target organisms. J. Environ. Anal. Toxicol. S4, 002. doi:10.4172/2161-0525.S4-002
Soltani A., Vatandoost H., Oshaghi M. A., Ravasan N. M., Enayati A. A., Asgarian F. (2015). Resistance mechanisms of Anopheles stephensi (Diptera: Culicidae) to temephos. J. Arthropod. Borne. Dis. 9, 71–83.
Uragayala S., Verma V., Natarajan E., Velamuri P. S., Kamaraju R. (2015). Adulticidal & larvicidal efficacy of three neonicotinoids against insecticide susceptible & resistant mosquito strains. Indian J. Med. Res. 142, S64–S70. doi:10.4103/0971-5916.176624
Vaughan A., Hemingway J. (1995). Mosquito carboxylesterase Estα21 (A2). Cloning and sequence of the full length cDNA for a major insecticide resistance gene worldwide in the mosquito Culex quinquefasciatus. J. Biol. Chem. 270, 17044–17049. doi:10.1074/jbc.270.28.17044
Warikoo R., Ray A., Sandhu J. K., Samal R., Wahab N., Kumar S. (2012). Larvicidal and irritant activities of hexane leaf extracts of Citrus sinensis against dengue vector Aedes aegypti L. Asian pac. J. Trop. Biomed. 2, 152–155. doi:10.1016/S2221-1691(11)60211-6
Weill M., Fort P., Berthomieu A., Dubois M. P., Pasteur N., Raymond M. (2002). A novel acetylcholinesterase gene in mosquitoes codes for the insecticide target and is non-homologous to the ace gene in Drosophila Proc. Biol. Sci. 269, 2007–2016. doi:10.1098/rspb.2002.2122
Weill M., Malcolm C., Chandre F., Mogensen K., Berthomieu A., Marquine M., et al. (2004). The unique mutation in ace-1 giving high insecticide resistance is easily detectable in mosquito vectors. Insect Mol. Biol. 13, 1–7. doi:10.1111/j.1365-2583.2004.00452.x
Weill W., Lutfalla G., Mogensen K., Chandre F., Berthomieu A., Berticat C., et al. (2003). Corrigendum: Insecticide resistance in mosquito vectors. Nature 425, 366. doi:10.1038/425366b
WHO (World Health Organization) (1998). Techniques to detect insecticide resistance mechanisms (Field Laboratory Manual). Geneva: World Health Organization. Available at: http://www.who.int/malaria/publications/atoz/who_cds_cpc_mal_98_6/en/.
Yang T., Liu N. (2014). Permethrin resistance variation and susceptible reference line isolation in a field population of the mosquito, Culex quinquefasciatus (Diptera: Culicidae). Insect Sci. 21, 659–666. doi:10.1111/1744-7917.12071
Yanola J., Somboon P., Walton C., Nachaiwieng W., Somwang P., Prapanthadara L. A. (2011). High throughput assays for detection of the F1534C mutation in the voltage gated sodium channel gene in permethrin resistant Aedes aegypti and the distribution of this mutation throughout Thailand. Trop. Med. Int. Health 16, 501–509. doi:10.1111/j.1365-3156.2011.02725.x
Keywords: Aedes aegypti, acetamiprid, resistance, metabolic detoxification, mutation, target site
Citation: Samal RR, Panmei K, Lanbiliu P and Kumar S (2022) Metabolic detoxification and ace-1 target site mutations associated with acetamiprid resistance in Aedes aegypti L. Front. Physiol. 13:988907. doi: 10.3389/fphys.2022.988907
Received: 07 July 2022; Accepted: 25 July 2022;
Published: 30 August 2022.
Edited by:
Sylvia Anton, Institut National de la Recherche Agronomique (INRA), FranceReviewed by:
Anubha Seth, Yale University, United StatesCopyright © 2022 Samal, Panmei, Lanbiliu and Kumar. This is an open-access article distributed under the terms of the Creative Commons Attribution License (CC BY). The use, distribution or reproduction in other forums is permitted, provided the original author(s) and the copyright owner(s) are credited and that the original publication in this journal is cited, in accordance with accepted academic practice. No use, distribution or reproduction is permitted which does not comply with these terms.
*Correspondence: Sarita Kumar, c2FyaXRha3VtYXJAYW5kYy5kdS5hYy5pbg==
†These authors have contributed equally to the work.
Disclaimer: All claims expressed in this article are solely those of the authors and do not necessarily represent those of their affiliated organizations, or those of the publisher, the editors and the reviewers. Any product that may be evaluated in this article or claim that may be made by its manufacturer is not guaranteed or endorsed by the publisher.
Research integrity at Frontiers
Learn more about the work of our research integrity team to safeguard the quality of each article we publish.