- 1Division of Pulmonary and Critical Care Medicine, Department of Medicine, University of California, San Francisco, San Francisco, CA, United States
- 2Division of Respiratory Diseases, Department of Medicine, Federal University of SP, São Paulo, Brazil
- 3Department of Biochemistry and Molecular Genetics, University of Colorado Anschutz Medical Campus, Aurora, CO, United States
Pulmonary arterial hypertension is an incurable disease marked by dysregulated metabolism, both at the cellular level in the pulmonary vasculature, and at the whole-body level characterized by impaired exercise oxygen consumption. Though both altered pulmonary vascular metabolism and abnormal exercise physiology are key markers of disease severity and pulmonary arterial remodeling, their precise interactions are relatively unknown. Herein we review normal pulmonary vascular physiology and the current understanding of pulmonary vascular cell metabolism and cardiopulmonary response to exercise in Pulmonary arterial hypertension. We additionally introduce a newly developed international collaborative effort aimed at quantifying exercise-induced changes in pulmonary vascular metabolism, which will inform about underlying pathophysiology and clinical management. We support our investigative approach by presenting preliminary data and discuss potential future applications of our research platform.
Introduction
Pulmonary arterial hypertension (PAH), also known as group 1 pulmonary hypertension (PH) by the World Health Organization classification system, is a fatal condition characterized by aberrant proliferation of cells constituting the pulmonary vessels, resulting in vascular wall thickening and luminal obliteration to produce elevated pulmonary vascular resistance (PVR) and subsequent right ventricular (RV) failure (Stacher et al., 2012; Galie et al., 2015; Simonneau et al., 2019). Historically, PAH has been defined by hemodynamic indices measured with right heart catheterization (RHC) while the patient is at rest. PAH resting RHC diagnostic criteria include increased mean pulmonary artery pressure (mPAP) > 20 mmHg and elevated PVR (≥3 Wood units), along with pulmonary arterial wedge pressure (PAWP) ≤ 15 mmHg indicative of a normal left ventricular filling pressure.
Despite introduction of new therapies and improved survival, PAH remains incurable (Hurdman et al., 2012; McGoon et al., 2013; Hensley et al., 2018), and the anticipated 5-years survival of PAH patients can be as low as 50% (Hurdman et al., 2012). Notably, significant pulmonary vascular remodeling persists in the pulmonary arteries of PAH patients treated with modern PAH-targeted pharmacologic modalities (Stacher et al., 2012), underscoring shortcomings of current diagnostic and therapeutic approaches in detecting PAH at its initial stages and achieving reversal of pulmonary vascular remodeling. These rather disappointing outcomes suggest that 1) assessment of PAH based on resting hemodynamic evaluation may not properly characterize pulmonary vascular reserve and early pulmonary vascular dysfunction, and that 2) currently available treatments may not address all relevant pathogenetic mechanisms of PAH.
In this article, we explore two promising and interrelated areas of potential diagnostic and therapeutic implications in PAH: pulmonary vascular metabolism and pulmonary vascular physiology during exercise. We focus on recent discoveries and what they add to the current understanding of PAH pathophysiology and pathobiology. We further discuss an ongoing international collaboration to study pulmonary vascular metabolism during standardized exercise, and we report preliminary data highlighting the scientific and clinical relevance of our investigative work.
Pulmonary vascular metabolism in healthy individuals and the O2 pathway
Daily life activities such as walking, talking and breathing depend on the maintenance of human body’s homeostasis. In turn, homeostasis depends on preserved tissue metabolism and the balance between energy production and consumption (Kisaka et al., 2017; Houstis et al., 2018). Human tissue metabolism is predominantly aerobic, using oxygen (O2) as the main substrate to regenerate adenosine triphosphate (ATP) from adenosine diphosphate (ADP), thereby generating energy for any given activity, even the involuntary ones, such as heart beating (Dhakal et al., 2015; Kisaka et al., 2017; Houstis et al., 2018).
All O2 utilized by the human body is absorbed at the pulmonary level and goes through different systems until tissue absorption; this process is called the O2 pathway (Dhakal et al., 2015; Houstis et al., 2018). The O2 pathway involves alveolar ventilation, diffusion into pulmonary capillary blood and hemoglobin, blood flow through the systemic circulation, diffusion into the tissue cells and, finally, mitochondrial respiration (Dhakal et al., 2015; Houstis et al., 2018). Therefore, the interactions among cardiac, pulmonary vascular, hematologic, and nervous systems are essential to human body metabolism and energy production (Dhakal et al., 2015; Huang et al., 2017; Kisaka et al., 2017; Melamed et al., 2019; Cho et al., 2020; Grafton et al., 2020; Malte et al., 2021). The O2 carried via the O2 pathway and absorbed by the cells can be represented and quantified as O2 uptake (VO2). According to the Fick principle, VO2 is the product of cardiac output (CO) and the arterial-mixed venous O2 content difference (C(a-v)O2) (Dhakal et al., 2015; Kisaka et al., 2017; Melamed et al., 2019). Arterial O2 content (CaO2), can be calculated by:
Upstream of cellular metabolism, O2 must be adequately absorbed from the external environment and transported to all other systems (Tabima et al., 2017; Houstis et al., 2018). In this context, the pulmonary and cardiovascular systems work as a unit and play an important and interdependent role in the O2 pathway (Tabima et al., 2017). At the lung level, the absorption of O2 occurs through the alveolar-capillary membrane; adequate function of this membrane depends on membrane conductance (directly related to pulmonary vascular wall integrity), pulmonary capillary blood volume (directly related to pulmonary vascular blood flow, and therefore RV function), and the degree of remodeling and thickness of the alveolar capillaries (Olson et al., 2016). From a physiological standpoint, it is essential that the alveolar, pulmonary vascular, and cardiac systems work properly and that the interactions among these components are preserved for adequate function of the O2 pathway (Tabima et al., 2017).
Given that pulmonary vascular membrane conductance is highly dependent on the integrity of pulmonary vascular wall tissue, conditions that stimulate excessive growth of pulmonary vascular cells, such as PAH, will affect the diffusion capacity of the alveolo-capillary membrane. As a result, via pulmonary vascular remodeling and associated pulmonary vascular stiffness (Singh et al., 2019a), PAH leads to progressive obliteration of pulmonary arterial lumen, subsequently raising PVR and therefore increasing PAP, ultimately resulting in a dysfunctional RV (Naeije and Chesler, 2012; Naeije, 2013; Dhakal et al., 2015; Olson et al., 2016; Kisaka et al., 2017; Tabima et al., 2017), which in sum adversely impacts the O2 pathway.
Pulmonary vascular metabolism in pulmonary arterial hypertension at the cellular level
In a normal, disease-free state, metabolism plays an important role in maintaining the integrity of vascular structure and function. The pulmonary vasculature is comprised of distinct cell types working in concert, including endothelial cells, smooth muscle cells, and perivascular stromal and immune cells. In each of these cell types, a number of metabolic pathways involving various metabolites, such as glucose, glutamine, and fatty acids among others, regulate cellular functions. For example, in endothelial cells, glycolysis is crucial in the preservation of adherens junctions, repair after injury, and new vessel formation, in addition to ATP generation (Culic et al., 1997; De Bock et al., 2013; Li et al., 2019; Stevens et al., 2021). Interplays among metabolic pathways is similarly important in the homeostasis of the vascular smooth muscle cells (Stenmark et al., 2018) and surrounding immune cells (O'Neill et al., 2016). Interactions between different cell types is also crucial for physiologic function of the pulmonary vasculature (Gao et al., 2016), such as the production of nitric oxide by endothelial cells which regulates smooth muscle cell tone.
A sizeable body of literature on PAH in humans and PH in animal models have underscored an important role of dysregulated and altered pulmonary vascular cell metabolism in disease pathogenesis (Tuder et al., 2012; Cottrill and Chan, 2013; Sutendra and Michelakis, 2014). Historically, the role of dysregulated metabolism as a key driver of disease was first established in cancer studies before its relevance was recognized in pulmonary vascular diseases. In fact, metabolic dysregulation represents one of several pathobiologic similarities between cancer and PAH, comprising a paradigm known as the cancer hypothesis of PAH (Lee et al., 1998; Voelkel et al., 1998; Rai et al., 2008). This hypothesis originated from the observation that proliferating endothelial cells in pulmonary arterial plexiform lesions (a characteristic pulmonary vascular pathology) of idiopathic PAH (IPAH) patients are clonal, suggesting cancer-like selection for cells with genetic growth advantages (Lee et al., 1998); it was subsequently expanded by the recognition of somatic mutations and growth-promoting gene expressions in PAH lungs.
Notably, many pro-survival and pro-proliferation signals affect cell metabolism, and conversely, metabolic dysregulations can have growth-inducing effects. Although once believed to represent passive consequences of cancer-promoting signals (Levine and Puzio-Kuter, 2010), it is now understood that modulation of metabolic pathways can epigenetically program cell behavior, thereby actively influencing tumor growth (Wellen and Thompson, 2012; Hirschey et al., 2015). Given the overlaps between PAH and cancer, and considering that PAH is a disease marked by intimal cell proliferation and medial hypertrophy (Stacher et al., 2012), it is likely that altered pulmonary vascular metabolism significantly contributes to PAH pathobiology.
One well known example of dysregulated cellular metabolism affecting both cancer and PAH is the shift of glucose metabolism toward glycolysis accompanied by reduced mitochondrial glucose oxidation, a phenomenon termed aerobic glycolysis or the Warburg effect (Koppenol et al., 2011). Glucose uptake and glycolysis are increased in the lungs of PAH patients, both at the whole lung (Xu et al., 2007; Hagan et al., 2011; Zhao et al., 2013) and the cellular levels (Fijalkowska et al., 2010; Hernandez-Saavedra et al., 2020). In animal models of experimental PH, both pharmacologic global inhibition of glycolysis (Michelakis et al., 2002; McMurtry et al., 2004; Guignabert et al., 2009) and cell-specific deletion of pro-glycolytic genes (Ball et al., 2014; Cao et al., 2019; Kojima et al., 2019; Luo et al., 2019; Wang et al., 2021) were protective. One mechanism by which cytoplasmic glucose oxidation supports cell growth and proliferation is a resulting flux of glucose carbons into the pentose phosphate pathway, including NADPH production, antioxidant glutathione regeneration, and lipid membrane and nucleotide synthesis (Vander Heiden et al., 2009; Weinberg et al., 2010; Lunt and Vander Heiden, 2011; De Bock et al., 2013). The pathobiological relevance of other glycolysis-related processes, such as lactate fermentation, in PAH will require further studies.
Another group of metabolites that likely contributes to PAH pathobiology is fatty acids. Studies suggest fatty acid metabolism and lipotoxicity-induced promotion of PAH is multi-dimensioned. For instance, fatty acid oxidation is increased at the whole body level in PAH patients (Mey et al., 2020), but it appears to be reduced specifically in their RVs (Hemnes et al., 2014; Brittain et al., 2016; Legchenko et al., 2018); RV fatty acid uptake might be disease-specific (Graham et al., 2015; Talati et al., 2016). Notably, strategies to induce (Legchenko et al., 2018) and inhibit (Sutendra et al., 2010; Lee et al., 2022) fatty acid oxidation at the systemic level both attenuated experimental PH in animals. Collectively, these conflicting observations indicate the complexity underlying the mechanistic link between fatty acid metabolism and PAH, and underscore the importance of probing disease and tissue-specific (e.g., PA vs. RV) roles of fatty acid metabolism. In addition to glucose and fatty acids, other metabolic pathways are also critical in PH pathobiology, including amino acid metabolism and the Krebs cycle, among others (Xu et al., 2021).
The long-recognized link between metabolism and cell growth has been targeted in the cancer field, in which metabolism-altering treatments have been heavily investigated in clinical trials with some approved for clinical use (Luengo et al., 2017; Stine et al., 2022). Despite a large body of evidence describing the pathogenetic role of metabolism in the pulmonary vasculature and the clinical availability of many metabolism-modulating agents, clinical applications targeting metabolic pathways in PH have been limited to a handful of studies (Bhandari and Subramanian, 2007; Finch et al., 2016; Michelakis et al., 2017). For example, by shifting glucose metabolism from glycolysis into the Krebs cycle, the pharmacologic compound dichloroacetate produced clinical improvement in PAH, but this beneficial effect was particularly observed in a subset of susceptible human subjects who were shown not to carry specific pro-glycolytic mutations (Michelakis et al., 2017).
One plausible explanation of this hindrance to clinically targeting metabolism in PAH is our currently limited understanding of how pulmonary vascular metabolism changes with exercise in health and disease, and how it relates to physiology particularly as blood flow through the pulmonary circulation, CO, and VO2 simultaneously increase during physical exertion. Given that dysregulated metabolism is reflective of pulmonary vascular remodeling, which in turn dictates pulmonary vascular and RV reserves, evaluating pulmonary vascular metabolism during exercise is anticipated to provide important advantages. For example, stressing the cardiopulmonary system toward the limit of its reserve may reveal underlying metabolic dysregulations which are not apparent at rest to a now quantifiable level, improving the sensitivity performance of the measurements. Exercise allows a dynamic assessment of the pulmonary vascular reserve in addition to metabolic pathways. Lastly, and perhaps most importantly, studying pulmonary vascular metabolism during exercise allows the identification of pertinent metabolic targets that correlate with exercise capacity, a well-stablished marker of disease severity (Galie et al., 2015), which may point to new pathobiologic targets for further investigation. Before exploring how the interplay between pulmonary vascular physiology and metabolism can be quantified in PAH patients during exercise, we first discuss the two components individually.
Pulmonary vascular physiology in pulmonary arterial hypertension during exercise
Under physiologic conditions, the pulmonary vasculature and the RV are coupled (Singh et al., 2019b; Singh et al., 2020; Singh et al., 2021). RV function depends on its ability to contract (muscular preserved contraction), its preload, and its afterload (i.e., the pulmonary vasculature) (Naeije, 2013; Tabima et al., 2017). The afterload imposed on the RV by the pulmonary vasculature is comprised of several components, including vascular resistance to blood flow, vascular compliance, pulsatile blood flow (i.e., arterial wave reflections) and blood inertance (Tedford, 2014). All these components are influenced by passive (transmural pressure driven by input and outflow pressures, i.e., PAP and PAWP, respectively) and active (sympathetic nervous system stimulation, nitric oxide-mediated mechanisms, and regional alveolar hypoxia) factors at rest, which are exacerbated during exercise (Reeves et al., 1988). Passive factors assist in ventilation and perfusion matching, while active factors dynamically regulate exercise vasodilation and vasoconstriction, further influencing pulmonary vascular distension (Kadowitz and Hyman, 1973; Kane et al., 1994; Reeves et al., 2005). Under physiologic conditions, the pulmonary vasculature is a system of low resistance and high compliance, with the ability to stretch and accommodate with minimal resistance increasing blood flow as CO dynamically rises (Naeije, 2013; Tabima et al., 2017). Therefore, while at rest, the RV requires minimal energy expenditure to eject blood flow through the normal pulmonary vasculature.
In healthy individuals, exercise-induced increases in heart rate and stroke volume result in augmentation of CO (Kovacs et al., 2009; Naeije and Chesler, 2012; Naeije, 2013; Tabima et al., 2017). The increased pulmonary vascular blood flow due to the increased CO raises PAP, left atrial pressure, and right atrial pressure. The increase in left atrial pressure during exercise influences PAP dynamic changes and its pulsatile component (Naeije, 2013; Olson et al., 2016; Tabima et al., 2017). The balance among these forces during exercise is preserved in healthy individuals (Naeije, 2013). However, the pulmonary vascular response during exercise may change with aging (Kovacs et al., 2009; Naeije, 2013; Oliveira et al., 2016a). As a result of the exercise-related increased pulmonary vascular blood flow, PVR will decrease during exercise in heathy individuals, mainly due to dilation of pulmonary vessels and recruitment of unperfused ones (Kovacs et al., 2012; Langleben et al., 2018; Olschewski et al., 2018). As the vessels distend due to the increased pulmonary vascular blood flow, their capacity to further accommodate the increasing blood flow also decreases, resulting in reduction of pulmonary vascular compliance (PVC) (Kovacs et al., 2009; Kovacs et al., 2012; O'Neill and Johnson, 1991; Oliveira et al., 2020). Similar to mPAP/CO exercise responses, older subjects tend to have higher PVR and lower PVC compared with younger individuals (Naeije, 2013; Oliveira et al., 2016a). The higher PVR and lower PVC in the elderly may be explained in part by the greater vascular stiffness associated with aging, and the more pronounced exercise-induced sympathetic nervous system stimulation during physical effort (Naeije, 2013; Oliveira et al., 2016a).
The development of abnormal pulmonary vascular responses to exercise is associated with a reduced exercise capacity. In PAH, exercise intolerance is specifically associated with impaired O2 delivery (DO2) and indices of increased RV and pulmonary vascular load (Oliveira et al., 2017). A decreased DO2 is in turn associated with concurrent reductions of exercise CaO2 and exercise CO. The reduction of CaO2 levels likely reflects the known effect of pulmonary vascular diseases on O2 diffusion in the pulmonary vasculature (Sun et al., 2003). Along these lines, a decreased RV stroke work index (RVWSI) at peak exercise concurrent with a dynamically reduced pulmonary vascular reserve evident by an impaired exercise PVC is associated with decreased peak VO2 (Messina et al., 2021). Failure to appropriately reduce PVR in relation to PVC during exercise due to pulmonary vascular remodeling has been shown to be a potential exercise hallmark of PH (Oliveira et al., 2020). As an early marker of pulmonary vascular remodeling, a dysfunctional PVC during exercise suggests that changes in intrinsic elastic properties of the pulmonary circulation, in addition to increased transmural pressure, underlie the increase in pulmonary vascular stiffness and its association with PH severity (Sanz et al., 2009). Therefore, by analyzing hemodynamic patterns under the stress of exercise, it is possible to infer pulmonary vascular reserve in PAH patients. How exercise-induced hemodynamic changes precisely relates to pulmonary vascular metabolism in PAH, however, remains uncharacterized.
Pulmonary vascular metabolism in pulmonary arterial hypertension during exercise
Most pulmonary vascular metabolic studies to date have focused on metabolism at rest, whereas PAH patients experience symptoms with exertion. In this context, metabolic phenotypes of the pulmonary vasculature in PAH may also become more apparent during exercise, particularly given that exercise capacity in PAH is a direct reflection of pulmonary vascular and RV reserve (Kovacs et al., 2017). The clinical relevance of assessing and correlating hemodynamic changes and pulmonary vascular metabolism during exercise is underscored by studies of patients with heart failure with preserved ejection fraction, in which effects of the nitric oxide donor, sodium nitrite, became much more apparent during exercise compared to at rest (Borlaug et al., 2015).
We found additional evidence supporting the importance of interrogating exercise-induced metabolic changes. In a cohort of 12 non-diabetic patients with systemic sclerosis-associated PAH (SScPAH) who underwent clinically indicated exercise RHC, we measured PA glucose and lactate levels both at rest and at peak exercise, along with routine hemodynamic indices. As anticipated, PA lactate levels increased with exercise, but PA glucose levels did not change (Figure 1). Interestingly, we found at peak exercise an inverse correlation between PA glucose and RVSWI (Figure 2), a load-dependent measurement of RV contractility that reflects the amount of work (i.e., energy expenditure) required by the RV to generate its stroke volume while overcoming the PA pulsatility and hemodynamic oscillations (Brittain et al., 2013; Messina et al., 2021). The data indicate a potential pathobiological relationship between PA glucose metabolism and RV function during exercise. This observation is consistent with the current knowledge that RV glucose uptake is increased in PAH patients, as demonstrated by imaging studies (Oikawa et al., 2005). However, it is unknown if this finding is a reflection of RV metabolic shift, increased stroke work or RV ischemia (Sanz et al., 2019), or a combination of all these factors.
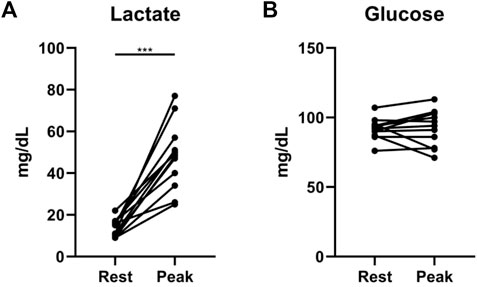
FIGURE 1. Exercise-induced changes in lactate (A) and glucose (B) levels from rest to peak exercise, measured from pulmonary artery catheters in 12 patients with systemic sclerosis-associated pulmonary arterial hypertension. ***p = 0.0005 (Wilcoxon matched-pairs signed rank test).
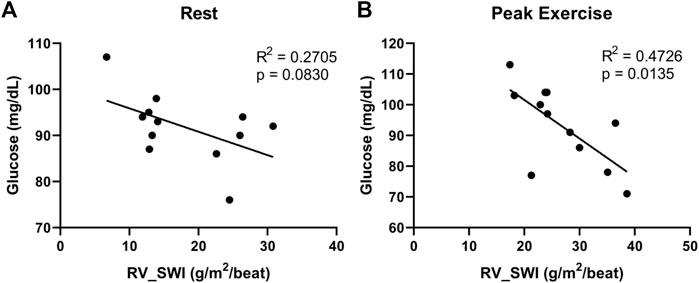
FIGURE 2. Correlations between right ventricular stroke work index (RV_SWI) and pulmonary artery glucose levels, measured at rest (A) and peak exercise (B) in 12 patients with systemic sclerosis-associated pulmonary arterial hypertension.
The potential of transpulmonary metabolomics in pulmonary arterial hypertension during exercise
While previous studies of metabolic changes in PAH patients, both at the cellular (Fessel et al., 2012) and the systemic levels (Lewis et al., 2016; Rhodes et al., 2017), have yielded valuable information, clinical application of these findings have been limited, largely due to the lack of metabolic information specific to the pulmonary vasculature and during exercise when patients are most symptomatic and hemodynamic changes are most pronounced, as discussed above. We have thus developed an international collaborative effort which aims to study transpulmonary metabolite flux during standardized exercise.
One guiding principle of our method is that differences between paired blood draws across a tissue bed can quantify net metabolite uptake versus excretion from that bed. Specifically, reflecting the direction of blood flow, subtracting the concentration of each metabolite measured in blood drawn from a PA catheter (pulmonary circulation) from that in blood drawn from a radial arterial line (systemic circulation) allows the quantification of net metabolic flux (i.e., excretion or uptake) occurring across the pulmonary vascular bed. In this manner, simultaneously collecting blood samples from the PA catheter and the radial arterial line enables specific quantification of pulmonary vascular metabolic flux (Lewis et al., 2016).
Another novel aspect of our experimental approach is the assessment of pulmonary vascular metabolism at distinct, clinically relevant stages of standardized exercise, for example during freewheeling (load-free) and post-exercise recovery, in addition to at rest and peak exercise. Exercise endpoints measured during freewheeling were shown to have prognostic value in PAH (Sayegh et al., 2020), and delayed recovery rates are anticipated in more severe PAH (Ramos et al., 2012; Oliveira et al., 2016b). We therefore hypothesize that metabolomic interrogation during freewheeling and in recovery, in addition to the classical at rest (pre-exercise) and peak exercise timepoints, will demonstrate pathobiological and prognostic significance of changes in pulmonary vascular metabolism in response to changing exercise load.
Our investigative effort represents a teamwork of clinician-scientists and basic scientists in Brazil and the United States. We plan to prospectively enroll PAH patients who will undergo clinically indicated exercise RHC at the Invasive Pulmonary Hemodynamic Assessment Program at the Federal University of São Paulo in Brazil (Ramos et al., 2012; Oliveira et al., 2014a; Oliveira et al., 2014b; Ramos et al., 2014; Oliveira et al., 2018; Vieira et al., 2020; Messina et al., 2021). Briefly, a resting supine RHC will be first performed following standard recommendations (Kovacs et al., 2013; Galie et al., 2015; Kovacs et al., 2017). Next, patients will exercise on a supine cycle ergometer attached to the table, following an incremental step-test with a 10 W increase every 2 min until exhaustion. Measurements include radial artery, right atrial, PA, and PA wedge pressures, as well as radial and pulmonary artery O2 saturations (Boerrigter et al., 2014; Herve et al., 2015; Oliveira et al., 2016a; Oliveira et al., 2016b; Kovacs et al., 2017; Oliveira et al., 2017). CO will be measured in triplicate at rest and once per exercise stage using thermodilution. Our exercise protocol is summarized in Figure 3.
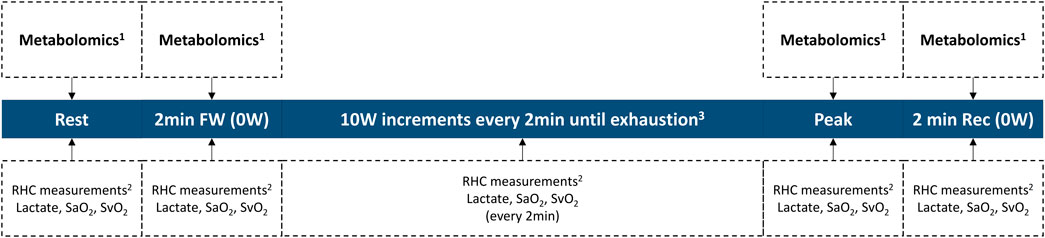
FIGURE 3. Invasive exercise protocol. 1Collected from the pulmonary artery catheter and from the radial artery catheter. 2RHC measurements include heart rate, blood pressure, right atrial pressure, pulmonary arterial pressure, pulmonary arterial wedge pressure, and cardiac output. 3Study length varies on an individual basis, until exhaustion. RHC right heart catheterization; SaO2 radial artery O2 saturation; SvO2 mixed-venous O2 saturation; workload in Watts.
During the study, paired blood samples will be simultaneously collected from the PA catheter and the radial arterial line at the four specified timepoints of clinical relevance (at rest, after 2 min of freewheeling, at peak exercise, and 2 min into recovery). Blood samples will be centrifuged at +4 °C, and the plasma aliquoted in preparation for a mass spectrometry-based metabolomic assessment. Subsequently, the plasma samples will be analyzed and profiled for changes in metabolites central to energy metabolism by the Metabolomics Core at the University of Colorado (United States) (Graham et al., 2018; Hernandez-Saavedra et al., 2020). Untargeted data will be mined in conjunction with the KEGG database for metabolites in carbon and nitrogen metabolism (identifying ∼175 metabolites) (Nemkov et al., 2017; Nemkov et al., 2019). This platform has been successfully applied to multiple studies on human plasma specimens (Thomas et al., 2020; Ye et al., 2021). The transpulmonary gradient of each metabolite is determined by subtracting the value measured in the PA from that in the radial artery, reflecting net uptake or excretion by the pulmonary vascular bed.
Exercise-induced changes in transpulmonary metabolite gradients incorporating both the systemic and the pulmonary circulations have not been reported to date, underscoring the novelty of our experimental design. Moreover, significant metabolomic findings from pulmonary arterial blood samples alone, reported by our group (Figures 1, 2) and others (Sanders et al., 2019), add confidence that our approach will yield invaluable information clarifying the contribution of pulmonary vascular metabolism to PAH pathobiology. It is worth mentioning that similar efforts at quantifying the pulmonary vascular metabolome during exercise are being undertaken by other groups, including the PVDOMICS initiative in the United States (Hemnes et al., 2017; Tang et al., 2020). Given that our patient population consists of Brazilian citizens in São Paulo, who are ethnically and therefore genetically extremely diverse, we expect to make discoveries that are both unique and complementary to those from other patient cohorts. Additionally, our experimental design will capture, for the first time, low-intensity, resistance-free exercise (freewheeling), thereby providing new insights into how routine, non-strenuous daily activities might relate to pulmonary vascular metabolism in PAH patients.
In summary, we propose to perform high-throughput mass spectrometry-based metabolomics on blood samples obtained at four pre-specified stages of standardized exercise. We anticipate that simultaneous, high-throughput measurement of metabolites excreted or taken up by the pulmonary vascular bed will define relationships among pulmonary vascular metabolism, hemodynamic indices of PAH severity, and the degree of underlying pulmonary vascular remodeling and functional reserve. Our approach will allow the correlation of metabolomics with clinical endpoints of pulmonary vascular and exercise physiology, potentially serving as a novel therapeutic and diagnostic tool in managing PAH patients.
Conclusion
The pathophysiology of PAH represents a complex interplay among cardiopulmonary physiology, the O2 pathway, and pulmonary vascular metabolism at the cellular level. Disturbances to each of these individual factors collectively reflect the underlying pathobiology of remodeled pulmonary vasculature, and exercise creates a unique environment allowing a dynamic assessment of pulmonary vascular metabolism and identification of relevant metabolic processes. Interrogating pulmonary vascular metabolism in the context of exercise capacity and hemodynamic variables will clarify how metabolism contributes to PAH and help establish novel diagnostic strategies and rational treatment targets based on both molecular and physiologic parameters.
To investigate these topics, we propose a study that has the potential to significantly impact our understanding of PAH vascular metabolism and the care of PAH patients. Establishment of clinically and pathobiologically important metabolic targets that closely correlate with both physiologic and pathologic metrics will serve as a prognostic tool to monitor disease progression and treatment response. If this platform is effective, metabolism-modulating therapies might be tested for effectiveness in the setting of standardize exercise. Additionally, metabolic characterization may prove useful in selecting at-risk patients who may benefit from close follow-up or early initiation of treatment. Although our focus at this time is on PAH, the same exercise protocol and method of analysis can be used to provide useful insights into disease pathophysiology in other PH etiologies in the future. We plan to bring together the two areas of established relevance in PAH, dysregulated metabolism and exercise physiology, and interrogate their intersection.
Author contributions
All authors contributed to the conception and design of the work; interpretation of data for the work; and drafting and revising the work critically. All authors approved the final version of the manuscript and agreed to be accountable for all its aspects and ensure that questions related to the accuracy or integrity of any part of the work will be appropriately investigated and resolved.
Funding
This work was supported by F32HL151076 and CHEST Foundation Research Grant in Pulmonary Hypertension to MHL; TM was supported by the Coordination for the Improvement of Higher Education Personnel (CAPES, Brazil); P01HL152961 and R01HL135872 to BBG; RO received a grant for research productivity from the National Council for Scientific and Technological Development (CNPq, Brazil, grant 313284/2021-0).
Conflict of interest
The authors declare that the research was conducted in the absence of any commercial or financial relationships that could be construed as a potential conflict of interest.
Publisher’s note
All claims expressed in this article are solely those of the authors and do not necessarily represent those of their affiliated organizations, or those of the publisher, the editors and the reviewers. Any product that may be evaluated in this article, or claim that may be made by its manufacturer, is not guaranteed or endorsed by the publisher.
References
Ball M. K., Waypa G. B., Mungai P. T., Nielsen J. M., Czech L., Dudley V. J., et al. (2014). Regulation of hypoxia-induced pulmonary hypertension by vascular smooth muscle hypoxia-inducible factor-1α. Am. J. Respir. Crit. Care Med. 189, 314–324. doi:10.1164/rccm.201302-0302OC
Bhandari B., Subramanian L. (2007). Ranolazine, a partial fatty acid oxidation inhibitor, its potential benefit in angina and other cardiovascular disorders. Recent Pat. cardiovasc. Drug Discov. 2, 35–39. doi:10.2174/157489007779606095
Boerrigter B. G., Waxman A. B., Westerhof N., Vonk-Noordegraaf A., Systrom D. M. (2014). Measuring central pulmonary pressures during exercise in COPD: How to cope with respiratory effects. Eur. Respir. J. 43, 1316–1325. doi:10.1183/09031936.00016913
Borlaug B. A., Koepp K. E., Melenovsky V. (2015). Sodium nitrite improves exercise hemodynamics and ventricular performance in heart failure with preserved ejection fraction. J. Am. Coll. Cardiol. 66, 1672–1682. doi:10.1016/j.jacc.2015.07.067
Brittain E. L., Pugh M. E., Wheeler L. A., Robbins I. M., Loyd J. E., Newman J. H., et al. (2013). Shorter survival in familial versus idiopathic pulmonary arterial hypertension is associated with hemodynamic markers of impaired right ventricular function. Pulm. Circ. 3, 589–598. doi:10.1086/674326
Brittain E. L., Talati M., Fessel J. P., Zhu H., Penner N., Calcutt M. W., et al. (2016). Fatty acid metabolic defects and right ventricular lipotoxicity in human pulmonary arterial hypertension. Circulation 133, 1936–1944. doi:10.1161/CIRCULATIONAHA.115.019351
Cao Y., Zhang X., Wang L., Yang Q., Ma Q., Xu J., et al. (2019). PFKFB3-mediated endothelial glycolysis promotes pulmonary hypertension. Proc. Natl. Acad. Sci. U. S. A. 116, 13394–13403. doi:10.1073/pnas.1821401116
Cho J., Choi Y., Sajgalik P., No M. H., Lee S. H., Kim S., et al. (2020). Exercise as a therapeutic strategy for sarcopenia in heart failure: Insights into underlying mechanisms. Cells 9, E2284. doi:10.3390/cells9102284
Cottrill K. A., Chan S. Y. (2013). Metabolic dysfunction in pulmonary hypertension: The expanding relevance of the Warburg effect. Eur. J. Clin. Invest. 43, 855–865. doi:10.1111/eci.12104
Culic O., Gruwel M. L., Schrader J. (1997). Energy turnover of vascular endothelial cells. Am. J. Physiol. 273, C205–C213. doi:10.1152/ajpcell.1997.273.1.C205
De Bock K., Georgiadou M., Schoors S., Kuchnio A., Wong B. W., Cantelmo A. R., et al. (2013). Role of PFKFB3-driven glycolysis in vessel sprouting. Cell 154, 651–663. doi:10.1016/j.cell.2013.06.037
Dhakal B. P., Malhotra R., Murphy R. M., Pappagianopoulos P. P., Baggish A. L., Weiner R. B., et al. (2015). Mechanisms of exercise intolerance in heart failure with preserved ejection fraction: The role of abnormal peripheral oxygen extraction. Circ. Heart Fail. 8, 286–294. doi:10.1161/CIRCHEARTFAILURE.114.001825
Fessel J. P., Hamid R., Wittmann B. M., Robinson L. J., Blackwell T., Tada Y., et al. (2012). Metabolomic analysis of bone morphogenetic protein receptor type 2 mutations in human pulmonary endothelium reveals widespread metabolic reprogramming. Pulm. Circ. 2, 201–213. doi:10.4103/2045-8932.97606
Fijalkowska I., Xu W., Comhair S. A., Janocha A. J., Mavrakis L. A., Krishnamachary B., et al. (2010). Hypoxia inducible-factor1alpha regulates the metabolic shift of pulmonary hypertensive endothelial cells. Am. J. Pathol. 176, 1130–1138. doi:10.2353/ajpath.2010.090832
Finch K. T., Stratton E. A., Farber H. W. (2016). Ranolazine for the treatment of pulmonary hypertension associated with heart failure with preserved ejection fraction: A pilot study. J. Heart Lung Transpl. 35, 1370–1373. doi:10.1016/j.healun.2016.07.015
Galie N., Humbert M., Vachiery J. L., Gibbs S., Lang I., Torbicki A., et al. (2015). 2015 ESC/ERS guidelines for the diagnosis and treatment of pulmonary hypertension: The joint task force for the diagnosis and treatment of pulmonary hypertension of the European society of cardiology (ESC) and the European respiratory society (ERS): Endorsed by: Association for European paediatric and congenital cardiology (AEPC), international society for heart and lung transplantation (ISHLT). Eur. Respir. J. 46, 903–975. doi:10.1183/13993003.01032-2015
Gao Y., Chen T., Raj J. U. (2016). Endothelial and smooth muscle cell interactions in the pathobiology of pulmonary hypertension. Am. J. Respir. Cell Mol. Biol. 54, 451–460. doi:10.1165/rcmb.2015-0323TR
Grafton G., Cascino T. M., Perry D., Ashur C., Koelling T. M. (2020). Resting oxygen consumption and heart failure: Importance of measurement for determination of cardiac output with the use of the Fick principle. J. Card. Fail. 26, 664–672. doi:10.1016/j.cardfail.2019.02.004
Graham B. B., Kumar R., Mickael C., Kassa B., Koyanagi D., Sanders L., et al. (2018). Vascular adaptation of the right ventricle in experimental pulmonary hypertension. Am. J. Respir. Cell Mol. Biol. 59, 479–489. doi:10.1165/rcmb.2018-0095OC
Graham B. B., Kumar R., Mickael C., Sanders L., Gebreab L., Huber K. M., et al. (2015). Severe pulmonary hypertension is associated with altered right ventricle metabolic substrate uptake. Am. J. Physiol. Lung Cell. Mol. Physiol. 309, L435–L440. doi:10.1152/ajplung.00169.2015
Guignabert C., Tu L., Izikki M., Dewachter L., Zadigue P., Humbert M., et al. (2009). Dichloroacetate treatment partially regresses established pulmonary hypertension in mice with SM22alpha-targeted overexpression of the serotonin transporter. FASEB J. official Publ. Fed. Am. Soc. Exp. Biol. 23, 4135–4147. doi:10.1096/fj.09-131664
Hagan G., Southwood M., Treacy C., Ross R. M., Soon E., Coulson J., et al. (2011). 18)FDG pet imaging can quantify increased cellular metabolism in pulmonary arterial hypertension: A proof-of-principle study. Pulm. Circ. 1, 448–455. doi:10.4103/2045-8932.93543
Hemnes A. R., Beck G. J., Newman J. H., Abidov A., Aldred M. A., Barnard J., et al. (2017). Pvdomics: A multi-center study to improve understanding of pulmonary vascular disease through phenomics. Circ. Res. 121, 1136–1139. doi:10.1161/CIRCRESAHA.117.311737
Hemnes A. R., Brittain E. L., Trammell A. W., Fessel J. P., Austin E. D., Penner N., et al. (2014). Evidence for right ventricular lipotoxicity in heritable pulmonary arterial hypertension. Am. J. Respir. Crit. Care Med. 189, 325–334. doi:10.1164/rccm.201306-1086OC
Hensley M. K., Levine A., Gladwin M. T., Lai Y. C. (2018). Emerging therapeutics in pulmonary hypertension. Am. J. Physiol. Lung Cell. Mol. Physiol. 314, L769–L781. doi:10.1152/ajplung.00259.2017
Hernandez-Saavedra D., Sanders L., Freeman S., Reisz J. A., Lee M. H., Mickael C., et al. (2020). Publisher Correction: Stable isotope metabolomics of pulmonary artery smooth muscle and endothelial cells in pulmonary hypertension and with TGF-beta treatment. Sci. Rep. 10, 4349. doi:10.1038/s41598-020-60500-w
Herve P., Lau E. M., Sitbon O., Savale L., Montani D., Godinas L., et al. (2015). Criteria for diagnosis of exercise pulmonary hypertension. Eur. Respir. J. 46, 728–737. doi:10.1183/09031936.00021915
Hirschey M. D., DeBerardinis R. J., Diehl A. M. E., Drew J. E., Frezza C., Green M. F., et al. (2015). Dysregulated metabolism contributes to oncogenesis. Semin. Cancer Biol. 35, S129–S150. doi:10.1016/j.semcancer.2015.10.002
Houstis N. E., Eisman A. S., Pappagianopoulos P. P., Wooster L., Bailey C. S., Wagner P. D., et al. (2018). Exercise intolerance in heart failure with preserved ejection fraction: Diagnosing and ranking its causes using personalized O2 pathway analysis. Circulation 137, 148–161. doi:10.1161/CIRCULATIONAHA.117.029058
Huang W., Resch S., Oliveira R. K., Cockrill B. A., Systrom D. M., Waxman A. B. (2017). Invasive cardiopulmonary exercise testing in the evaluation of unexplained dyspnea: Insights from a multidisciplinary dyspnea center. Eur. J. Prev. Cardiol. 24, 1190–1199. doi:10.1177/2047487317709605
Hurdman J., Condliffe R., Elliot C. A., Davies C., Hill C., Wild J. M., et al. (2012). ASPIRE registry: Assessing the spectrum of pulmonary hypertension identified at a REferral centre. Eur. Respir. J. 39, 945–955. doi:10.1183/09031936.00078411
Kadowitz P. J., Hyman A. L. (1973). Effect of sympathetic nerve stimulation on pulmonary vascular resistance in the dog. Circ. Res. 32, 221–227. doi:10.1161/01.res.32.2.221
Kane D. W., Tesauro T., Koizumi T., Gupta R., Newman J. H. (1994). Exercise-induced pulmonary vasoconstriction during combined blockade of nitric oxide synthase and beta adrenergic receptors. J. Clin. Invest. 93, 677–683. doi:10.1172/JCI117020
Kisaka T., Stringer W. W., Koike A., Agostoni P., Wasserman K. (2017). Mechanisms that modulate peripheral oxygen delivery during exercise in heart failure. Ann. Am. Thorac. Soc. 14, S40–S47. doi:10.1513/AnnalsATS.201611-889FR
Kojima H., Tokunou T., Takahara Y., Sunagawa K., Hirooka Y., Ichiki T., et al. (2019). Hypoxia-inducible factor-1 alpha deletion in myeloid lineage attenuates hypoxia-induced pulmonary hypertension. Physiol. Rep. 7, e14025. doi:10.14814/phy2.14025
Koppenol W. H., Bounds P. L., Dang C. V. (2011). Otto Warburg's contributions to current concepts of cancer metabolism. Nat. Rev. Cancer 11, 325–337. doi:10.1038/nrc3038
Kovacs G., Avian A., Olschewski A., Olschewski H. (2013). Zero reference level for right heart catheterisation. Eur. Respir. J. 42, 1586–1594. doi:10.1183/09031936.00050713
Kovacs G., Berghold A., Scheidl S., Olschewski H. (2009). Pulmonary arterial pressure during rest and exercise in healthy subjects: A systematic review. Eur. Respir. J. 34, 888–894. doi:10.1183/09031936.00145608
Kovacs G., Herve P., Barbera J. A., Chaouat A., Chemla D., Condliffe R., et al. (2017). An official European respiratory society statement: Pulmonary haemodynamics during exercise. Eur. Respir. J. 50, 1700578. doi:10.1183/13993003.00578-2017
Kovacs G., Olschewski A., Berghold A., Olschewski H. (2012). Pulmonary vascular resistances during exercise in normal subjects: A systematic review. Eur. Respir. J. 39, 319–328. doi:10.1183/09031936.00008611
Langleben D., Orfanos S. E., Herve P. (2018). Pulmonary capillary recruitment in exercise and pulmonary hypertension. Eur. Respir. J. 51, 1800260. doi:10.1183/13993003.00260-2018
Lee M. H., Sanders L., Kumar R., Hernandez-Saavedra D., Yun X., Ford J. A., et al. (2022). Contribution of fatty acid oxidation to the pathogenesis of pulmonary hypertension. Am. J. Physiology-Lung Cell. Mol. Physiology. doi:10.1152/ajplung.00039.2022
Lee S. D., Shroyer K. R., Markham N. E., Cool C. D., Voelkel N. F., Tuder R. M. (1998). Monoclonal endothelial cell proliferation is present in primary but not secondary pulmonary hypertension. J. Clin. Invest. 101, 927–934. doi:10.1172/JCI1910
Legchenko E., Chouvarine P., Borchert P., Fernandez-Gonzalez A., Snay E., Meier M., et al. (2018). PPARγ agonist pioglitazone reverses pulmonary hypertension and prevents right heart failure via fatty acid oxidation. Sci. Transl. Med. 10, eaao0303. doi:10.1126/scitranslmed.aao0303
Levine A. J., Puzio-Kuter A. M. (2010). The control of the metabolic switch in cancers by oncogenes and tumor suppressor genes. Science 330, 1340–1344. doi:10.1126/science.1193494
Lewis G. D., Ngo D., Hemnes A. R., Farrell L., Domos C., Pappagianopoulos P. P., et al. (2016). Metabolic profiling of right ventricular-pulmonary vascular function reveals circulating biomarkers of pulmonary hypertension. J. Am. Coll. Cardiol. 67, 174–189. doi:10.1016/j.jacc.2015.10.072
Li X., Sun X., Carmeliet P. (2019). Hallmarks of endothelial cell metabolism in health and disease. Cell Metab. 30, 414–433. doi:10.1016/j.cmet.2019.08.011
Luengo A., Gui D. Y., Vander Heiden M. G. (2017). Targeting metabolism for cancer therapy. Cell Chem. Biol. 24, 1161–1180. doi:10.1016/j.chembiol.2017.08.028
Lunt S. Y., Vander Heiden M. G. (2011). Aerobic glycolysis: Meeting the metabolic requirements of cell proliferation. Annu. Rev. Cell Dev. Biol. 27, 441–464. doi:10.1146/annurev-cellbio-092910-154237
Luo Y., Teng X., Zhang L., Chen J., Liu Z., Chen X., et al. (2019). CD146-HIF-1α hypoxic reprogramming drives vascular remodeling and pulmonary arterial hypertension. Nat. Commun. 10, 3551. doi:10.1038/s41467-019-11500-6
Malte H., Lykkeboe G., Wang T. (2021). The magnitude of the Bohr effect profoundly influences the shape and position of the blood oxygen equilibrium curve. Comp. Biochem. Physiol. A Mol. Integr. Physiol. 254, 110880. doi:10.1016/j.cbpa.2020.110880
McGoon M. D., Benza R. L., Escribano-Subias P., Jiang X., Miller D. P., Peacock A. J., et al. (2013). Pulmonary arterial hypertension: Epidemiology and registries. J. Am. Coll. Cardiol. 62, D51–D59. doi:10.1016/j.jacc.2013.10.023
McMurtry M. S., Bonnet S., Wu X., Dyck J. R., Haromy A., Hashimoto K., et al. (2004). Dichloroacetate prevents and reverses pulmonary hypertension by inducing pulmonary artery smooth muscle cell apoptosis. Circ. Res. 95, 830–840. doi:10.1161/01.RES.0000145360.16770.9f
Melamed K. H., Santos M., Oliveira R. K. F., Urbina M. F., Felsenstein D., Opotowsky A. R., et al. (2019). Unexplained exertional intolerance associated with impaired systemic oxygen extraction. Eur. J. Appl. Physiol. 119, 2375–2389. doi:10.1007/s00421-019-04222-6
Messina C. M. S., Ferreira E. V. M., Singh I., Fonseca A. X. C., Ramos R. P., Nery L. E., et al. (2021). Impact of right ventricular work and pulmonary arterial compliance on peak exercise oxygen uptake in idiopathic pulmonary arterial hypertension. Int. J. Cardiol. 331, 230–235. doi:10.1016/j.ijcard.2021.01.027
Mey J. T., Hari A., Axelrod C. L., Fealy C. E., Erickson M. L., Kirwan J. P., et al. (2020). Lipids and ketones dominate metabolism at the expense of glucose control in pulmonary arterial hypertension: A hyperglycaemic clamp and metabolomics study. Eur. Respir. J. 55, 1901700. doi:10.1183/13993003.01700-2019
Michelakis E. D., Gurtu V., Webster L., Barnes G., Watson G., Howard L., et al. (2017). Inhibition of pyruvate dehydrogenase kinase improves pulmonary arterial hypertension in genetically susceptible patients. Sci. Transl. Med. 9, eaao4583. doi:10.1126/scitranslmed.aao4583
Michelakis E. D., McMurtry M. S., Wu X. C., Dyck J. R., Moudgil R., Hopkins T. A., et al. (2002). Dichloroacetate, a metabolic modulator, prevents and reverses chronic hypoxic pulmonary hypertension in rats: Role of increased expression and activity of voltage-gated potassium channels. Circulation 105, 244–250. doi:10.1161/hc0202.101974
Naeije R., Chesler N. (2012). Pulmonary circulation at exercise. Compr. Physiol. 2, 711–741. doi:10.1002/cphy.c100091
Naeije R. (2013). Physiology of the pulmonary circulation and the right heart. Curr. Hypertens. Rep. 15, 623–631. doi:10.1007/s11906-013-0396-6
Nemkov T., Hansen K. C., D'Alessandro A. (2017). A three-minute method for high-throughput quantitative metabolomics and quantitative tracing experiments of central carbon and nitrogen pathways. Rapid Commun. Mass Spectrom. 31, 663–673. doi:10.1002/rcm.7834
Nemkov T., Reisz J. A., Gehrke S., Hansen K. C., D'Alessandro A. (2019). High-throughput metabolomics: Isocratic and gradient mass spectrometry-based methods. Methods Mol. Biol. 1978, 13–26. doi:10.1007/978-1-4939-9236-2_2
O'Neill A. V., Johnson D. C. (1991). Transition from exercise to rest. Ventilatory and arterial blood gas responses. Chest 99, 1145–1150. doi:10.1378/chest.99.5.1145
O'Neill L. A., Kishton R. J., Rathmell J. (2016). A guide to immunometabolism for immunologists. Nat. Rev. Immunol. 16, 553–565. doi:10.1038/nri.2016.70
Oikawa M., Kagaya Y., Otani H., Sakuma M., Demachi J., Suzuki J., et al. (2005). Increased [18F]fluorodeoxyglucose accumulation in right ventricular free wall in patients with pulmonary hypertension and the effect of epoprostenol. J. Am. Coll. Cardiol. 45, 1849–1855. doi:10.1016/j.jacc.2005.02.065
Oliveira R. K., Agarwal M., Tracy J. A., Karin A. L., Opotowsky A. R., Waxman A. B., et al. (2016). Age-related upper limits of normal for maximum upright exercise pulmonary haemodynamics. Eur. Respir. J. 47, 1179–1188. doi:10.1183/13993003.01307-2015
Oliveira R. K., Ferreira E. V., Ramos R. P., Messina C. M., Kapins C. E., Silva C. M., et al. (2014). Usefulness of pulmonary capillary wedge pressure as a correlate of left ventricular filling pressures in pulmonary arterial hypertension. J. Heart Lung Transpl. 33, 157–162. doi:10.1016/j.healun.2013.10.008
Oliveira R. K., Pereira C. A., Ramos R. P., Ferreira E. V., Messina C. M., Kuranishi L. T., et al. (2014). A haemodynamic study of pulmonary hypertension in chronic hypersensitivity pneumonitis. Eur. Respir. J. 44, 415–424. doi:10.1183/09031936.00010414
Oliveira R. K., Waxman A. B., Agarwal M., Badr Eslam R., Systrom D. M. (2016). Pulmonary haemodynamics during recovery from maximum incremental cycling exercise. Eur. Respir. J. 48, 158–167. doi:10.1183/13993003.00023-2016
Oliveira R. K. F., Faria-Urbina M., Maron B. A., Santos M., Waxman A. B., Systrom D. M. (2017). Functional impact of exercise pulmonary hypertension in patients with borderline resting pulmonary arterial pressure. Pulm. Circ. 7, 654–665. doi:10.1177/2045893217709025
Oliveira R. K. F., Ota-Arakaki J. S., Gomes P. S., Gimenez A., Messina C. M. S., Ramos R. P., et al. (2018). Pulmonary haemodynamics and mortality in chronic hypersensitivity pneumonitis. Eur. Respir. J. 51, 1800430. doi:10.1183/13993003.00430-2018
Oliveira R. K. F., Waxman A. B., Hoover P. J., Dellaripa P. F., Systrom D. M. (2020). Pulmonary vascular and right ventricular burden during exercise in interstitial lung disease. Chest 158, 350–358. doi:10.1016/j.chest.2020.02.043
Olschewski H., Kovacs G., Herve P. (2018). Pulmonary capillary recruitment in exercise and pulmonary hypertension. Eur. Respir. J. 51, 1800260. doi:10.1183/13993003.00260-2018
Olson T. P., Johnson B. D., Borlaug B. A. (2016). Impaired pulmonary diffusion in heart failure with preserved ejection fraction. JACC. Heart Fail. 4, 490–498. doi:10.1016/j.jchf.2016.03.001
Rai P. R., Cool C. D., King J. A., Stevens T., Burns N., Winn R. A., et al. (2008). The cancer paradigm of severe pulmonary arterial hypertension. Am. J. Respir. Crit. Care Med. 178, 558–564. doi:10.1164/rccm.200709-1369PP
Ramos R. P., Arakaki J. S., Barbosa P., Treptow E., Valois F. M., Ferreira E. V., et al. (2012). Heart rate recovery in pulmonary arterial hypertension: Relationship with exercise capacity and prognosis. Am. Heart J. 163, 580–588. doi:10.1016/j.ahj.2012.01.023
Ramos R. P., Ota-Arakaki J. S., Alencar M. C., Ferreira E. V., Nery L. E., Neder J. A. (2014). Exercise oxygen uptake efficiency slope independently predicts poor outcome in pulmonary arterial hypertension. Eur. Respir. J. 43, 1510–1512. doi:10.1183/09031936.00167713
Reeves J. T., Linehan J. H., Stenmark K. R. (2005). Distensibility of the normal human lung circulation during exercise. Am. J. Physiol. Lung Cell. Mol. Physiol. 288, L419–L425. doi:10.1152/ajplung.00162.2004
Reeves J. T., Moon R. E., Grover R. F., Groves B. M. (1988). Increased wedge pressure facilitates decreased lung vascular resistance during upright exercise. Chest 93, 97S–99S. doi:10.1378/chest.93.3_supplement.97s
Rhodes C. J., Ghataorhe P., Wharton J., Rue-Albrecht K. C., Hadinnapola C., Watson G., et al. (2017). Plasma metabolomics implicates modified transfer RNAs and altered bioenergetics in the outcomes of pulmonary arterial hypertension. Circulation 135, 460–475. doi:10.1161/CIRCULATIONAHA.116.024602
Sanders J. L., Han Y., Urbina M. F., Systrom D. M., Waxman A. B. (2019). Metabolomics of exercise pulmonary hypertension are intermediate between controls and patients with pulmonary arterial hypertension. Pulm. Circ. 9, 2045894019882623. doi:10.1177/2045894019882623
Sanz J., Kariisa M., Dellegrottaglie S., Prat-Gonzalez S., Garcia M. J., Fuster V., et al. (2009). Evaluation of pulmonary artery stiffness in pulmonary hypertension with cardiac magnetic resonance. JACC. Cardiovasc. Imaging 2, 286–295. doi:10.1016/j.jcmg.2008.08.007
Sanz J., Sanchez-Quintana D., Bossone E., Bogaard H. J., Naeije R. (2019). Anatomy, function, and dysfunction of the right ventricle: JACC state-of-the-art review. J. Am. Coll. Cardiol. 73, 1463–1482. doi:10.1016/j.jacc.2018.12.076
Sayegh A. L. C., Silva B. M., Ferreira E. V. M., Ramos R. P., Fisher J. P., Nery L. E., et al. (2020). Clinical utility of ventilatory and gas exchange evaluation during low-intensity exercise for risk stratification and prognostication in pulmonary arterial hypertension. Respirology 26, 264–272. doi:10.1111/resp.13959
Simonneau G., Montani D., Celermajer D. S., Denton C. P., Gatzoulis M. A., Krowka M., et al. (2019). Haemodynamic definitions and updated clinical classification of pulmonary hypertension. Eur. Respir. J. 53, 1801913. doi:10.1183/13993003.01913-2018
Singh I., Oliveira R. K. F., Heerdt P., Brown M. B., Faria-Urbina M., Waxman A. B., et al. (2020). Dynamic right ventricular function response to incremental exercise in pulmonary hypertension. Pulm. Circ. 10, 2045894020950187. doi:10.1177/2045894020950187
Singh I., Oliveira R. K. F., Heerdt P. M., Pari R., Systrom D. M., Waxman A. B. (2021). Sex-related differences in dynamic right ventricular-pulmonary vascular coupling in heart failure with preserved ejection fraction. Chest 159, 2402–2416. doi:10.1016/j.chest.2020.12.028
Singh I., Oliveira R. K. F., Naeije R., Rahaghi F. N., Oldham W. M., Systrom D. M., et al. (2019). Pulmonary vascular distensibility and early pulmonary vascular remodeling in pulmonary hypertension. Chest 156, 724–732. doi:10.1016/j.chest.2019.04.111
Singh I., Rahaghi F. N., Naeije R., Oliveira R. K. F., Vanderpool R. R., Waxman A. B., et al. (2019). Dynamic right ventricular-pulmonary arterial uncoupling during maximum incremental exercise in exercise pulmonary hypertension and pulmonary arterial hypertension. Pulm. Circ. 9, 2045894019862435. doi:10.1177/2045894019862435
Stacher E., Graham B. B., Hunt J. M., Gandjeva A., Groshong S. D., McLaughlin V. V., et al. (2012). Modern age pathology of pulmonary arterial hypertension. Am. J. Respir. Crit. Care Med. 186, 261–272. doi:10.1164/rccm.201201-0164OC
Stenmark K. R., Frid M. G., Graham B. B., Tuder R. M. (2018). Dynamic and diverse changes in the functional properties of vascular smooth muscle cells in pulmonary hypertension. Cardiovasc. Res. 114, 551–564. doi:10.1093/cvr/cvy004
Stevens R. P., Paudel S. S., Johnson S. C., Stevens T., Lee J. Y. (2021). Endothelial metabolism in pulmonary vascular homeostasis and acute respiratory distress syndrome. Am. J. Physiol. Lung Cell. Mol. Physiol. 321, L358–L376. doi:10.1152/ajplung.00131.2021
Stine Z. E., Schug Z. T., Salvino J. M., Dang C. V. (2022). Targeting cancer metabolism in the era of precision oncology. Nat. Rev. Drug Discov. 21, 141–162. doi:10.1038/s41573-021-00339-6
Sun X. G., Hansen J. E., Oudiz R. J., Wasserman K. (2003). Pulmonary function in primary pulmonary hypertension. J. Am. Coll. Cardiol. 41, 1028–1035. doi:10.1016/s0735-1097(02)02964-9
Sutendra G., Bonnet S., Rochefort G., Haromy A., Folmes K. D., Lopaschuk G. D., et al. (2010). Fatty acid oxidation and malonyl-CoA decarboxylase in the vascular remodeling of pulmonary hypertension. Sci. Transl. Med. 2, 44ra58. doi:10.1126/scitranslmed.3001327
Sutendra G., Michelakis E. D. (2014). The metabolic basis of pulmonary arterial hypertension. Cell Metab. 19, 558–573. doi:10.1016/j.cmet.2014.01.004
Tabima D. M., Philip J. L., Chesler N. C. (2017). Right ventricular-pulmonary vascular interactions. Physiol. (Bethesda, Md) 32, 346–356. doi:10.1152/physiol.00040.2016
Talati M. H., Brittain E. L., Fessel J. P., Penner N., Atkinson J., Funke M., et al. (2016). Mechanisms of lipid accumulation in the bone morphogenetic protein receptor type 2 mutant right ventricle. Am. J. Respir. Crit. Care Med. 194, 719–728. doi:10.1164/rccm.201507-1444OC
Tang W. H. W., Wilcox J. D., Jacob M. S., Rosenzweig E. B., Borlaug B. A., Frantz R. P., et al. (2020). Comprehensive diagnostic evaluation of cardiovascular physiology in patients with pulmonary vascular disease: Insights from the PVDOMICS program. Circ. Heart Fail. 13, e006363. doi:10.1161/CIRCHEARTFAILURE.119.006363
Tedford R. J. (2014). Determinants of right ventricular afterload (2013 Grover Conference series). Pulm. Circ. 4, 211–219. doi:10.1086/676020
Thomas T., Stefanoni D., Reisz J. A., Nemkov T., Bertolone L., Francis R. O., et al. (2020). COVID-19 infection alters kynurenine and fatty acid metabolism, correlating with IL-6 levels and renal status. JCI Insight 5, 140327. doi:10.1172/jci.insight.140327
Tuder R. M., Davis L. A., Graham B. B. (2012). Targeting energetic metabolism: A new frontier in the pathogenesis and treatment of pulmonary hypertension. Am. J. Respir. Crit. Care Med. 185, 260–266. doi:10.1164/rccm.201108-1536PP
Vander Heiden M. G., Cantley L. C., Thompson C. B. (2009). Understanding the Warburg effect: The metabolic requirements of cell proliferation. Science 324, 1029–1033. doi:10.1126/science.1160809
Vieira E. B., Ota-Arakaki J. S., Dal Corso S., Ivanaga I., Fonseca A. X. C., Oliveira R. K. F., et al. (2020). Incremental step test in patients with pulmonary hypertension. Respir. Physiol. Neurobiol. 271, 103307. doi:10.1016/j.resp.2019.103307
Voelkel N. F., Cool C., Lee S. D., Wright L., Geraci M. W., Tuder R. M. (1998). Primary pulmonary hypertension between inflammation and cancer. Chest 114, 225S–230s. doi:10.1378/chest.114.3_supplement.225s
Wang L., Zhang X., Cao Y., Ma Q., Mao X., Xu J., et al. (2021). Mice with a specific deficiency of Pfkfb3 in myeloid cells are protected from hypoxia-induced pulmonary hypertension. Br. J. Pharmacol. 178, 1055–1072. doi:10.1111/bph.15339
Weinberg F., Hamanaka R., Wheaton W. W., Weinberg S., Joseph J., Lopez M., et al. (2010). Mitochondrial metabolism and ROS generation are essential for Kras-mediated tumorigenicity. Proc. Natl. Acad. Sci. U. S. A. 107, 8788–8793. doi:10.1073/pnas.1003428107
Wellen K. E., Thompson C. B. (2012). A two-way street: Reciprocal regulation of metabolism and signalling. Nat. Rev. Mol. Cell Biol. 13, 270–276. doi:10.1038/nrm3305
Xu W., Janocha A. J., Erzurum S. C. (2021). Metabolism in pulmonary hypertension. Annu. Rev. Physiol. 83, 551–576. doi:10.1146/annurev-physiol-031620-123956
Xu W., Koeck T., Lara A. R., Neumann D., DiFilippo F. P., Koo M., et al. (2007). Alterations of cellular bioenergetics in pulmonary artery endothelial cells. Proc. Natl. Acad. Sci. U. S. A. 104, 1342–1347. doi:10.1073/pnas.0605080104
Ye H., Minhajuddin M., Krug A., Pei S., Chou C. H., Culp-Hill R., et al. (2021). The hepatic microenvironment uniquely protects leukemia cells through induction of growth and survival pathways mediated by LIPG. Cancer Discov. 11, 500–519. doi:10.1158/2159-8290.CD-20-0318
Zhao L., Ashek A., Wang L., Fang W., Dabral S., Dubois O., et al. (2013). Heterogeneity in lung (18)FDG uptake in pulmonary arterial hypertension: Potential of dynamic (18)FDG positron emission tomography with kinetic analysis as a bridging biomarker for pulmonary vascular remodeling targeted treatments. Circulation 128, 1214–1224. doi:10.1161/CIRCULATIONAHA.113.004136
Keywords: pulmonary hypertension, pulmonary arterial hypertension, exercise physiology, right heart catheterization, pulmonary vascular reserve, pulmonary vascular metabolism, metabolomics
Citation: Lee MH, Menezes TCF, Reisz JA, Ferreira EVM, Graham BB and Oliveira RKF (2022) Exercise metabolomics in pulmonary arterial hypertension: Where pulmonary vascular metabolism meets exercise physiology. Front. Physiol. 13:963881. doi: 10.3389/fphys.2022.963881
Received: 08 June 2022; Accepted: 23 August 2022;
Published: 12 September 2022.
Edited by:
Vasile Foris, Medical University of Graz, AustriaReviewed by:
Gustavo Heresi, Cleveland Clinic, United StatesJane A. Leopold, Brigham and Women’s Hospital, Harvard Medical School, United States
Copyright © 2022 Lee, Menezes, Reisz, Ferreira, Graham and Oliveira. This is an open-access article distributed under the terms of the Creative Commons Attribution License (CC BY). The use, distribution or reproduction in other forums is permitted, provided the original author(s) and the copyright owner(s) are credited and that the original publication in this journal is cited, in accordance with accepted academic practice. No use, distribution or reproduction is permitted which does not comply with these terms.
*Correspondence: Rudolf K. F. Oliveira, cnVkb2xmLm9saXZlaXJhQHVuaWZlc3AuYnI=