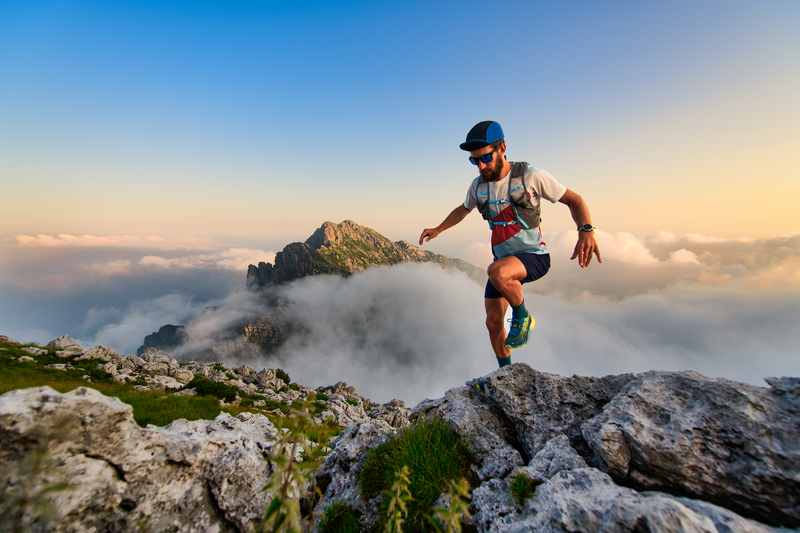
94% of researchers rate our articles as excellent or good
Learn more about the work of our research integrity team to safeguard the quality of each article we publish.
Find out more
ORIGINAL RESEARCH article
Front. Physiol. , 19 August 2022
Sec. Exercise Physiology
Volume 13 - 2022 | https://doi.org/10.3389/fphys.2022.963799
Hyperbaric Oxygen (HBO2) has been proposed as a pre-conditioning method to enhance exercise performance. Most prior studies testing this effect have been limited by inadequate methodologies. Its potential efficacy and mechanism of action remain unknown. We hypothesized that HBO2 could enhance aerobic capacity by inducing mitochondrial biogenesis via redox signaling in skeletal muscle. HBO2 was administered in combination with high-intensity interval training (HIIT), a potent redox stimulus known to induce mitochondrial biogenesis. Aerobic capacity was tested during acute hypobaric hypoxia seeking to shift the limiting site of whole body V̇O2 from convection to diffusion, more closely isolating any effect of improved oxidative capacity. Healthy volunteers were screened with sea-level (SL) V̇O2peak testing. Seventeen subjects were enrolled (10 men, 7 women, ages 26.5±1.3 years, BMI 24.6±0.6 kg m−2, V̇O2peak SL = 43.4±2.1). Each completed 6 HIIT sessions over 2 weeks randomized to breathing normobaric air, “HIIT+Air” (PiO2 = 0.21 ATM) or HBO2 (PiO2 = 1.4 ATM) during training, “HIIT+HBO2” group. Training workloads were individualized based on V̇O2peak SL test. Vastus Lateralis (VL) muscle biopsies were performed before and after HIIT in both groups. Baseline and post-training V̇O2peak tests were conducted in a hypobaric chamber at PiO2 = 0.12 ATM. HIIT significantly increased V̇O2peak in both groups: HIIT+HBO2 31.4±1.5 to 35.2±1.2 ml kg−1·min−1 and HIIT+Air 29.0±3.1 to 33.2±2.5 ml kg−1·min−1 (p = 0.005) without an additional effect of HBO2 (p = 0.9 for interaction of HIIT x HBO2). Subjects randomized to HIIT+HBO2 displayed higher skeletal muscle mRNA levels of PPARGC1A, a regulator of mitochondrial biogenesis, and HK2 and SLC2A4, regulators of glucose utilization and storage. All other tested markers of mitochondrial biogenesis showed no additional effect of HBO2 to HIIT. When combined with HIIT, short-term modest HBO2 (1.4 ATA) has does not increase whole-body V̇O2peak during acute hypobaric hypoxia. (ClinicalTrials.gov Identifier: NCT02356900; https://clinicaltrials.gov/ct2/show/NCT02356900).
Hyperbaric oxygen (HBO2) has been proposed as a method for pre-conditioning to enhance exercise performance. Many athletes have admitted to using HBO2 (for instance, 1.3 - 2.8 atmospheres absolute [ATA] for 50 - 90 minutes) based on the principle that short exposures to HBO2 immediately before maximal exercise testing facilitates “oxygen loading” (Cabric et al., 1991; McGavock et al., 1999; Rozenek et al., 2007; Kawada et al., 2008). While tissue oxygen levels normalize almost immediately after removal from the hyperoxic environment, in one study, HBO2 therapy led to increased peak oxygen uptake (V̇O2peak) that was linked to increased skeletal muscle mitochondrial mass (Hadanny et al., 2022).
Hyperbaric oxygen has also been studied in combination with exercise training, albeit with mixed results. When administered in combination with moderate continuous training in athletes, it resulted in no additional improvements on V̇O2peak compared with training under normoxic conditions (Burgos et al., 2016). However, in a more recent study, HBO2 was administered in combination with high-intensity interval training (HIIT) and led to increased peak work rate and peak minute ventilation compared with air controls, but no differences in V̇O2peak (DeCato et al., 2019). The underlying hypothesis is that the mitochondrial bioenergetic program is synergistically activated by these two stimuli, potentially via redox signaling. The mitochondrial biogenesis program is cytoprotective and generates and distributes healthy mitochondria throughout the cell in response to oxidant and other stresses (Piantadosi and Suliman, 2012). Given the link between mitochondrial mass and aerobic capacity (Weibel et al., 1991), we proposed that induction of the mitochondrial biogenesis program would result in detectable improvements in V̇O2peak.
The co-activator and binding partner peroxisome proliferator-activated receptor gamma coactivator 1-alpha (PGC-1α) is a reliable marker for activation of this bigenomic program in human skeletal muscle. Both endurance and interval exercise training consistently increase its expression (Pilegaard et al., 2003; Calvo et al., 2008; Gibala et al., 2009; Olesen et al., 2010), and there is evidence that the relationship between PGC-1α and exercise may be bidirectional, as overexpression of this molecule in transgenic mice increases mitochondrial content and, in turn, improves exercise performance (Calvo et al., 2008). Activation of skeletal muscle PGC-1α is also seen with low-dose hyperbaric exposure (1.25 ATA with FiO2 0.36) (Takemura and Ishihara, 2017) and other studies have found HBO2 can activate mitochondrial biogenesis in the brain (Gutsaeva et al., 2006; Hsu et al., 2022). Hence, HIIT and HBO2 together could synergistically increase PGC-1α and improve exercise capacity. However, HBO2 showed a neutral effect on V̇O2peak compared to HIIT alone (DeCato et al., 2019).
In the present study we directly examined the combined effect of HBO2 and HIIT on skeletal muscle bioenergetics as well as the attendant changes on V̇O2peak during acute hypobaric hypoxia. We chose a HIIT regimen similar to that employed by DeCato, et al. (DeCato et al., 2019), and measured changes in exercise capacity in a hypobaric hypoxic environment because hypoxic conditions (reduced oxygen supply) were believed to emphasize the role of peripheral determinants of V̇O2 (i.e. capillary surface area and mitochondrial capacity) more so than central determinants (i.e. lung capacity, heart function, and oxygen carrying capacity) (di Prampero, 2003). This strategy therefore allowed us focus as much as possible on the mitochondrial contributions of VO2 and concurrently measure changes in the skeletal muscle mitochondrial biogenesis program.
After institutional review board (IRB) approval and written informed consent, healthy non-smoker volunteers, ages 18 to 35 years, were screened. Subjects were excluded who had chronic comorbidities, pregnancy, sickle cell heterozygosity (African-American candidates were screened), physical deconditioning (sea-level V̇O2peak below 35 mL.kg-1.min-1 for men or 30 mL.kg-1.min-1 for women) (Gill et al., 2014; Bennett-Guerrero et al., 2017; Bennett-Guerrero et al., 2021), or were unable to provide written informed consent in English.
To determine study eligibility, potential subjects underwent a baseline medical history, physical exam, and sea-level (SL) graded maximal exercise test on a cycle ergometer. If eligible for participation, subjects were enrolled and underwent a vastus lateralis (VL) muscle biopsy followed 48 hours later by a graded maximal exercise test to exhaustion during acute hypobaric hypoxia (HH) in a hypobaric chamber decompressed to a barometric pressure of 429 mmHg (PiO2=0.12 atmospheres [ATM]) (Figure 1). Participants were then randomized 1:1 to a supervised, 6 session, high-intensity interval training program while breathing normobaric air, “HIIT+Air” group (PiO2=0.21 ATM), or 100% oxygen in a hyperbaric chamber “HIIT+HBO2” group (PiO2=1.4 ATM). Selection of the PO2 was based on a balance between the likelihood of a positive effect and an acceptable risk of central nervous system oxygen toxicity during exercise (Koch et al., 2013). One day after the last training session, a VL biopsy was obtained from the contralateral leg and a maximal exercise test during acute HH (PiO2=0.12 ATM) was repeated after a two-day recovery period. Subjects were instructed to continue their usual level of activity during the study, to avoid caffeine beverages on the day of exercise testing, and to avoid non-steroidal anti-inflammatory drugs (NSAIDs) 24 hours before muscle biopsies.
FIGURE 1. Study design. After a sea-level screening VO2peak test, subjects underwent a V̇O2peak test at altitude and were then randomized to HIIT + Air or HIIT + HBO2. After six HIIT sessions, subjects underwent a final V̇O2peak test at altitude. Abbreviations: ATM, atmospheres; HBO2, hyperbaric oxygen; HIIT, high-intensity interval training; PiO2, pressure of inspired oxygen; VL, vastus lateralis; V̇O2, oxygen consumption.
All exercise sessions were performed on an upright cycle ergometer (Monark, model 818E). Seat height, handlebar elevation and angle and pedal strapping established during the initial briefing visit were maintained for each subject in all training and testing sessions.
Sea-level maximal exercise testing protocol: Subjects cycled at 75 rpm starting at 50 Watts (W) and workload was increased by 50 W increments every 3 minutes. Verbal encouragement was provided throughout the test to elicit maximal effort. Continuous ECG and pulse-oximetry monitoring were provided. The test was terminated for inability to maintain cadence, muscle cramping or subject request. During each test, subjects wore a nose-clip and breathed air through a two-way non-rebreathing valve (Hans Rudolf, model 2700) connected to a metabolic cart (Consentius Technologies, ParvoMedics TrueMax 2400). Mixed expired oxygen (O2), carbon dioxide (CO2), respiratory rate, minute ventilation, respiratory exchange ratio (RER), O2 consumption (V̇O2), and CO2 elimination (V̇CO2) were measured with the metabolic cart and recorded every 30 s as 30 s averages. V̇O2peak was recorded as the highest O2 uptake (V̇O2) reached during the test. Ventilatory threshold (VT) was calculated using the modified V-slope method (30s averages) (Gaskill et al., 2001).
High Intensity Interval Training: Six training sessions were completed over a two-week period (Figure 1) by subjects in both groups using identical cycle ergometer setups and two-way non-rebreathing valve systems. Continuous ECG and periodic blood pressure monitoring were performed during each session. Subjects in the HIIT+Air group acted as controls training in our physiology laboratory at sea-level with the on-demand breathing system in direct communication with surrounding ambient air. Subjects randomized to train in the HBO2 group were pressurized in a dry hyperbaric chamber to a pressure of 1.4 ATA over 1 minute, or slower depending upon subject comfort. The described on-demand breathing system was connected to a pure oxygen source (FiO2=100%). A study investigator acted as a tender inside the chamber throughout each training session. Subjects were attached to a safety harness to avoid trauma in the event of an oxygen-induced convulsion. The chamber was decompressed back to sea-level pressure (1 ATA) over 2 minutes. Each training session consisted of a total of 30 minutes of exercise: Three minutes of warm-up, followed by six 30-second high-intensity interval periods interspersed with six 4-minute active recovery periods (Figure 2). Prescribed workloads for each period were calculated as percentages of the subject’s own peak workload (Watts) achieved during the screening SL maximal exercise test: 60% of peak output for the warm-up period, 120% for the high-intensity intervals and 50% for the recovery periods (Figure 2). During training, subjects were encouraged to maintain a cadence of 75 rpm while the pedaling resistance was adjusted by the supervising investigator to the prescribed workload for each period.
FIGURE 2. High-Intensity Interval Training. Timeline of a single 30-min HIIT session as a function of percent of V̇O2peak workload (W).
Acute hypobaric hypoxia maximal exercise testing: Maximal exercise tests were performed 1 week before and 1 week after the last HIIT session (Figure 1) in a hypobaric chamber at a simulated atmospheric pressure of 429 mmHg and FiO2=21%, (PiO2=0.12 ATM). Workload increments at altitude were 25W every 3 minutes. For each test, the chamber was depressurized at 2,000 ft per minute for 7.5 minutes to 15,000 ft. Recalibration of the metabolic cart was performed at target atmospheric pressure. A study investigator was present inside the chamber throughout completion of the test and the subject was attached to a safety harness to avoid trauma in the event of hypoxia-induced syncope. In addition to inability to maintain cadence, subject request and muscle cramping, tests were also terminated for severe hypoxemia (persistent O2 saturation of <70% on pulse-oximetry).
Vastus lateralis (VL) muscle biopsies were performed under sterile technique, using local anesthesia and a University College Hospital Muscle Biopsy Needle (120 mm, 5.0 mm OD, Popper & Sons, New Hyde Park, NY). Samples were obtained (total weight 200 mg), snap frozen in liquid nitrogen, and stored at -80°C.
Muscle proteins were separated by SDS-PAGE and identified by Western blot analysis (Suliman et al., 2007a). Membranes were incubated with validated antibodies against mitofusin-2 (Mfn2, 1:800, sc-50331), dynamin-1-like protein (Drp1, 1:1000, sc-271583), citrate synthase (1:2000, ab129095), ATP synthase subunit a (ATPase6, 1:1000, ab219825), cytochrome c oxidase (COI, 1:1000, PA5-26688), NADH dehydrogenase subunit 1 (ND1, 1:1000, ab181848), catalase (1:1500, ab209211), heme oxygenase-1 (HO-1, 1:1000, BML-HC3001), and superoxide dismutase-2 (SOD2, 1:2000, sc-137254), using GAPDH (1:5,000, G9545) or porin (1:5,000, PC548) antibody (Sigma, St. Louis, MO) as a loading control. After three washes in Tris-buffered saline with Tween, membranes were incubated with the appropriate horseradish peroxidase-conjugated secondary antibody (anti-goat, sc-2354, or anti-rabbit sc-2357 antibodies, both 1:10,000). Blots were developed with enhanced chemiluminescence (Western Blotting Luminol Reagent, sc-2048). Proteins were quantified by densitometry on digitized images from the mid dynamic range using Quantity One (Bio-Rad) and expressed relative to GAPDH or porin.
Total RNA was extracted from the muscle samples and analyzed by RT-PCR as previously described (Pecorella et al., 2015). RNA (1 μg) was reverse-transcribed by using random hexamer primers and a Superscript enzyme (Invitrogen) and real-time PCR was performed with a StepOne plus and gene expression master mix (Applied Biosystems, Foster City, CA). Primers and probes from Applied Biosystems were used for nuclear respiratory factor-2 (NRF2), peroxisome proliferator-activated receptor gamma coactivator 1-alpha (PPARGC1A), mitochondrial transcription factor A (TFAM), DNA polymerase subunit gamma-1 (POLG), mitochondrial DNA-directed RNA polymerase (POLRMT), glucose transporter 4 (SLC2A4), glucose transporter 1 (SLC2A1), and hexokinase-2 (HK2). 18S rRNA was used as an internal control. For the quantitative RT-PCR (qPCR) reaction, a difference of 1.0 in Ct value represents a twofold difference in transcript level. QRT-PCR was performed in triplicate. Quantitative PCR was then used to measure mtDNA copy number. Total mtDNA was isolated from muscle samples and determined by real-time PCR as described (Pecorella et al., 2015). Briefly, RT-PCR was performed using SYBR green qPCR Super Mix UDG (Invitrogen). The StepOne plus sequence detector system (Applied Biosystems) was used to record and analyze fluorescence intensities during PCR.
Based on previous studies by our laboratory measuring PGC-1α protein expression in healthy subjects after exercise training, we calculated that a sample size of at least 18 subjects (9 in each group) was required for 80% power to detect a significant change (alpha 0.05) in molecular markers of mitochondrial biogenesis following our intervention. The pre-specified primary outcomes included change (pre-HIIT vs. post-HIIT) in V̇O2peak, V̇O2 at VT, mitochondrial mass, Tfam expression, and PGC-1α expression. Grouped data are reported as means ± SEM unless otherwise indicated, and analyzed via repeated-measures two-way ANOVA (or if missing values, by mixed-effects analysis) with Fisher’s least significant difference post-hoc testing (GraphPad Prism v8, San Diego, CA). p <0.05 was considered statistically significant.
Twenty-five subjects were screened, four were excluded prior to randomization: one for below cut-off sea-level V̇O2peak, one due to syncope during first VL biopsy, and two because of training session scheduling conflicts. Twenty-one subjects completed the study protocol. Four were excluded from the data analysis due to submaximal effort on sea-level or altitude testing (RER<1.0), or due to technical failures related to calorimetric gas analysis at altitude. Therefore, 17 subjects (10 men, 7 women) were included in the data analysis. Nine were in the air group and 8 in the HBO2 group. Ages ranged 19–35 years, BMI 24.6±0.6 kg m−2, sea-level (SL) V̇O2peak 43.4±9 ml kg−1·min−1and baseline hypobaric hypoxia (HH) V̇O2peak 30.1±1.7 ml kg−1·min−1 (Table 1). Baseline characteristics are summarized in Table 1. Subjects in both groups were similar in age, BMI and fitness level (V̇O2peak SL HIIT+HBO2 group = 45.2±2.69 ml·kg·min−1 vs. HIIT+Air 41.7±3.30 ml kg−1·min−1; p = 0.31).
TABLE 1. Baseline Subject Characteristics including all subjects. All measurements performed before training at sea-level (SL) and during hypobaric hypoxia (HH) (mean ± SEM). †p-value represents comparison between groups. HH testing performed in a hypobaric chamber, PiO2 = 0.14. Abbreviations: BMI, body mass index; HR, heart rate; PiO2, pressure of inspired oxygen; RER, respiratory exchange ratio; SBP, systolic blood pressure; SL, sea-level; V̇O2peak, peak oxygen consumption.
Maximal exercise testing during acute HH (barometric pressure = 429mmHg; PiO2 = 0.12 ATM) resulted in an equal (non-statistically significant) Δ V̇O2peak from sea-level in both groups, -13.5±4.6 ml kg−1·min−1 in the HIIT+HBO2 group (48% decrease from baseline) and -13±3.9 ml kg−1·min−1 in the HIIT+Air group (56% decrease from baseline). A linear correlation was observed between Δ V̇O2peak with acute altitude exposure and sea-level V̇O2peak values, with the largest drops occurring in the fittest subjects (R = 0.8; p <0.001). This was in keeping with prior reports on the effect of acute hypoxia upon peak oxygen consumption (Lawler et al., 1988).
Heart rates were measured for each subject during the first, third and sixth recovery periods of the 1st and 6th training sessions but were not statistically significant between the two groups (152 ± 13 in the HIIT+Air group and 142 ± 21 in the HIIT+HBO2 group). Average workload (Watts) performed during each training session was also similar between both groups, 736±53.7 W in the HIIT+Air group and 688±64 W in the HIIT+HBO2 group (p = 0.4). Physiologic and hemodynamic parameters during maximal exercise testing before and after training are detailed in Table 2. The addition of HBO2 to HIIT did not result in an additional effect on V̇O2 at VT or during peak exercise compared to the effect of HIIT alone (p = 0.0045 for training effect, p = 0.9 for training x exposure effect). VT as V̇O2peak % remained unchanged in both groups after training (air, 73.2±2.5 to 70±2.0% and HBO2, 66.2±2.7 to 67±2.8%). There was no significant effect of training or HBO2 exposure on systolic blood pressure (SBP) or heart rate (HR) at rest and at peak exercise. There was, however, a significant effect of HBO2 on attenuating pulse pressure rise during exercise (p = 0.05 for interaction, post-hoc comparison).
TABLE 2. Physiologic effect of training in all subjects. N = 17. All measurements done during acute hypobaric hypoxia (HH), 429 mmHg barometric pressure (PiO2 = 0.14) before and after training (mean ± SEM). *p <0.05. Abbreviations: HH, hypobaric hypoxia; HR, heart rate; PP, pulse pressure; SBP, systolic blood pressure; V̇O2peak, peak oxygen uptake; VT, ventilatory threshold by modified V-slope method.
No adverse events occurred during training. In the HBO2 group, there were no complications related to chamber compression/decompression and no oxygen-induced convulsions or symptoms suggestive of oxygen toxicity, such as seizures or reductions in forced vital capacity. All acute high-altitude exposures were well tolerated, with no syncopal or pre-syncopal events. No tests were stopped due to hypoxemia (defined as SpO2<70%). One subject suffered vasovagal syncope during baseline muscle biopsy with uneventful recovery and one other subject declined repeat biopsy due to discomfort.
Skeletal muscle biopsies from a subset of subjects (HIIT+Air, n = 7; HIIT+HBO2, n = 6) were analyzed for changes in protein and gene expression. We found increased protein expression in mitofusin-2 (Mfn2), citrate synthase, ATPase 6, cytochrome c oxidase (COI), and ND1 from exercise training alone, but the addition of HBO2 had no effect (Figure 3). Neither intervention altered expression of Drp1. Quantitative PCR analysis showed significantly increased muscle expression of NRF2 and TFAM, two genes critical to regulation of mitochondrial biogenesis, from exercise training alone but not from HBO2 (Figure 4). We also found that exercise training but not HBO2 increased gene expression of the polymerases POLG and POLRMT, which are necessary for mitochondrial DNA replication and transcription, respectively. Moreover, compared to baseline values, there was significantly higher PPARGC1A expression and mtDNA copy number in muscle samples taken after training. However, with HIIT+HBO2, PPARGC1A expression was further augmented compared with the HIIT+Air group (p = 0.005).
FIGURE 3. Mitochondrial protein expression. Expression of the following skeletal muscle proteins was measured by western blot: (A) Mfn2, mitofusin-2; (B) Drp1, dynamin-1-like protein; (C) Citrate synthase; (D) ATPase6, mitochondrial ATP synthase subunit a; (E) COI, cytochrome c oxidase subunit 1; and (F) ND1, NADH-ubiquinone oxidoreductase chain 1. Reference proteins were either GAPDH or Porin. Statistical analysis was 2-way ANOVA with repeated measures and Fisher’s LSD post-hoc test. *p <0.05 shows significant within group comparison (i.e. baseline vs. post-HITT). Groups: air, black bars; HBO2, grey bars.
FIGURE 4. Mitochondrial gene expression. mRNA levels of the following genes were measured by quantitative PCR: (A) NRF2, nuclear respiratory factor 2; (B) PPARGC1A, peroxisome proliferator-activated receptor gamma coactivator 1-alpha; (C) TFAM, mitochondrial transcription factor a; (D) POLG, DNA polymerase subunit gamma-1; and (E) POLMRT, mitochondrial DNA-directed RNA polymerase. Reference mRNA was 18S and shown as fold-change. Mitochondrial DNA copy number was measured in (F) by PCR with 18S rDNA as reference and shown as fold-change. Statistical analysis was 2-way ANOVA with repeated measures and Fisher’s LSD post-hoc test. *p <0.05 shows significant within group comparison (i.e. baseline vs. post-HITT) and #p <0.05 shows significant between group comparisons (i.e. air, black bars vs. HBO2, grey bars).
Because both HIIT and HBO2 stimulate ROS production that may activate anti-oxidant responses, we measured expression of select mitochondrial anti-oxidant proteins (Figure 5). We found significantly increased expression of HO-1 and SOD2 with exercise training in both groups but no effect from HBO2. However, compared to baseline and to the HIIT+Air Group, the addition of HBO2 to HIIT significantly increased skeletal muscle catalase expression (p <0.005).
FIGURE 5. Antioxidant response. Antioxidant protein expression of (A) SOD2, superoxide dismutase-2; (B) HO-1, heme oxygenase-1; and (C) catalase were measured by western blot and referenced to GAPDH expression. Statistical analysis was 2-way ANOVA with repeated measures and Fisher’s LSD post-hoc test. *p <0.05 shows significant within group comparison (i.e. baseline vs. post-HITT) and #p <0.05 shows significant between group comparisons (i.e. air, black bars vs. HBO2, grey bars).
Finally, we investigated whether there were changes in markers of substrate (glucose) utilization (Figure 6). We found that exercise training alone significantly increased gene expression of the glucose transporters SLC2A1 and SLC2A4; however, compared with air treated subjects, the addition of HBO2 further increased SLC2A4 expression (p = 0.0007). Additionally, while neither HIIT nor HBO2 altered HK1 expression, exercise training significantly increased HK2 expression that was further increased by addition of HBO2 (p = 0.02).
FIGURE 6. Glucose-related gene expression. mRNA levels of the following genes were measured by quantitative PCR: (A) SLC2A1, Solute carrier family 2, facilitated glucose transporter member 1; (B) SLC2A4, Solute carrier family 2, facilitated glucose transporter member 4; (C) HK1, hexokinase-1; and (D) HK2, hexokinase-2. Reference mRNA was 18S and shown as fold-change. Statistical analysis was 2-way ANOVA with repeated measures and Fisher’s LSD post-hoc test. *p <0.05 shows significant within group comparison (i.e. baseline vs. post-HITT) and #p <0.05 shows significant between group comparisons (i.e. air, black bars vs. HBO2, grey bars).
The aim of our study was to examine the effect of HBO2 combined with HIIT on activation of mitochondrial biogenesis in skeletal muscle and maximal oxygen uptake during acute hypobaric hypoxia. Hypoxic conditions were implemented in an attempt to shift the whole-body oxygen uptake kinetics towards diffusion and extraction (greater dependence on mitochondrial function), and away from convection (di Prampero, 2003). HBO2 was administered in conjunction with HIIT, a known stimulator of mitochondrial biogenesis (Gibala et al., 2009; Little et al., 2010) and highly efficient method for improving aerobic capacity in trained and untrained individuals (Laursen and Jenkins, 2002; Hazell et al., 2010). Our findings found HIIT to be highly efficacious at improving V̇O2peak under acute hypoxic conditions. However, we found no added effect of HBO2 on aerobic capacity.
We also found no effect of HBO2 on the regulation of mitochondrial biogenesis compared with air controls. While PPARGC1A mRNA levels were significantly higher in subjects that trained with HBO2 compared with the air-only group (a primary endpoint, and to which our study was powered), the HBO2 group did not see increases in other associated markers, such as citrate synthase and ATPase6, which are measures of mitochondrial volume density, or NRF2 and TFAM expression, which are measures of mitochondrial biogenesis activation. The reasons for this are not clear, but could possibly relate to differences in peak expression after HBO2, insufficient dose of HBO2 to fully activate mitochondrial biogenesis, or lack of biological effect by HBO2. These findings contrast with recent work by Hadanny, et al. that found increased V̇O2peak in healthy master athletes after HBO2 treatment that was linked to increased skeletal muscle mitochondrial mass (Hadanny et al., 2022). However, we are skeptical of these molecular findings, as MitoTracker Green fluorescence was measured in fixed tissues, but is only validated and specific in live cells, and the PG1-alpha protein measurements were not stated as the nuclear (activated) fraction. Moreover, no changes were seen in MFN1/2 or OPA1, which are markers for mitochondrial quality and integrity. Our study also differed from the Hadanny study, as it included younger subjects, fewer HBO2 sessions, lower doses of HBO2, and added the HIIT intervention. This latter difference may be most relevant, as we found mitochondrial biogenesis (using appropriate techniques) was activated by HIIT alone, leading to increased mitochondrial mass. In fact, the mitochondrial biogenesis program may have been maximally activated by HIIT and may have reduced our ability to detect further effects from HBO2. HIIT alone activated mitochondrial biogenesis (evidenced by increased TFAM, NRF1, and PPARGCA1 gene expression), increased mitochondrial quality and integrity (increased MFN2 protein expression), and increased mitochondrial mass (increased citrate synthase and ATPase 6 protein expression). Furthermore, to our knowledge, this is the first study to translate from rodents to humans that interval exercise training increases gene expression of POLG, the mitochondrial DNA polymerase that repairs mtDNA damage (Clark-Matott et al., 2015) postulated to explain some of the health benefits of regular exercise (Garatachea et al., 2015). We also report for the first time in any species the exercise-induced gene expression of mitochondrial DNA-directed RNA polymerase (POLRMT), which transcribes mtDNA to RNA, and may be a novel biomarker for mitochondrial exercise response.
Breathing hyperbaric oxygen significantly raises tissue oxygen levels and increases production of reactive oxygen species (ROS), predominantly at the mitochondrial electron transport chain, where molecular oxygen is reduced to form superoxide (Jamieson et al., 1986). Superoxide is then converted by superoxide dismutase (SOD) to hydrogen peroxide (H2O2), a less toxic intermediate that is converted to water by catalase. We found that exercise but not HBO2 significantly increased mitochondrial SOD (SOD2) expression. However, only exercise and HBO2 together increased catalase expression. We are not sure why attendant increases in SOD2 after HBO2 were not seen, as HBO2 does increase superoxide formation (Jamieson et al., 1986; Groger et al., 2009), but possible explanations include missed peak expression, or reduced signal due to the highly efficient nature of the SOD system. It is also possible that the HBO2 host response is more dependent on cytosolic SOD3, which we did not measure. Regardless, the higher catalase expression seen after training with HBO2 likely reflects elevated tissue levels of H2O2. Hydrogen peroxide can activate PGC-1α expression (St-Pierre et al., 2006) as well as other cellular redox sensors (e.g. Akt) (Suliman et al., 2007b; Piantadosi and Suliman, 2012), providing other plausible mechanisms for the higher PPARGC1 mRNA levels seen after HBO2.
Glucose is a critical energy source during exercise, and its metabolism is regulated by insulin-dependent cellular uptake via glucose transporters and step-wise enzymatic catabolism. We found evidence this system was upregulated by exercise, consistent with prior studies (Pilegaard et al., 2003; Little et al., 2010), and perhaps further augmented by addition of HBO2. Specifically, compared to sea-level training, HIIT+HBO2 subjects displayed significantly higher gene expression of SLC2A4 (GLUT4), the dominant glucose transporter for skeletal muscle, and hexokinase-2 (HK2), a rate-limiting enzyme in glycolysis that also couples glucose metabolism to oxidative phosphorylation (Roberts and Miyamoto, 2015). These changes could be consistent with improved muscle bioenergetic efficiency, and if so, parallel those seen with exogenous CO administration (Rhodes et al., 2009).
Our study was limited by a number of factors. First, it is possible that maximal exercise testing is not the best test to examine the effects of changes in mitochondrial function, even under hypoxic conditions. Exercise efficiency during steady-state exercise or time to exhaustion during constant speed exertion have been proposed as more accurate methods for this purpose, and should be considered in future studies (Broskey et al., 2015). Secondly, our study design was based on the assumption that the rate-limiting step in V̇O2peak in healthy humans is oxygen supply. This was based on the concept of symmorphosis, which stipulates that biological structure is matched to physiological functional capacity. In the case of oxygen transport (except for lung capacity which exceeds demand), symmorphosis predicts matching of oxygen supply and demand in peripheral tissues at each step of the oxygen cascade (Weibel et al., 1991). Hence, we proposed that an acute reduction in ambient oxygen tension during maximal exercise would allow for a more accurate assessment of peripheral V̇O2 components, and more specifically, of mitochondrial oxidative capacity upon oxygen consumption rate (di Prampero, 2003). However, recent data calls into question the validity of symmorphosis in trained individuals, finding that V̇O2peak is limited by mitochondrial O2 demand rather than supply (Gifford et al., 2016). However, that study was conducted under different conditions (normoxia) and in different participants (endurance-trained athletes) than our study. Nevertheless, it is possible that the use of acute hypobaric hypoxia in our study to isolate mitochondrial O2 demand as a determinant of V̇O2peak may not have worked as expected. Third, it is possible that subjects in the HBO2 group trained at workloads that, although equivalent at sea-level to the Air group, represented a lower level of effort under their hyperoxic training condition. Rate of perceived exertion during training was not captured in our study. Fourth, the lack of morphological analysis of our muscle biopsies did not allow us to assess the effect of our intervention upon capillary density and muscle fiber remodeling, also important factors affecting the diffusion component of whole-body oxygen uptake. Fifth, we did not measure mitochondrial function (due to logistical and technical considerations) which may have uncovered underlying differences between the groups that were not otherwise evident. Finally, we cannot exclude the possibility of a beneficial training effect of higher PO2 (e.g. 2 ATA) when combined with HIIT; however, this was not studied to the greater risk of central nervous system oxygen toxicity.
In conclusion, our study found no beneficial effects of adding modestly-dosed HBO2 to HIIT for improving aerobic fitness and no definite molecular evidence of activation of skeletal muscle mitochondrial biogenesis. Future studies could investigate whether similar HBO2 doses improve glucose utilization and storage after HIIT and whether these accelerate recovery.
The raw data supporting the conclusion of this article will be made available by the authors, without undue reservation.
The studies involving human participants were reviewed and approved by Duke University Institutional Review Board. The patients/participants provided their written informed consent to participate in this study.
MV, SD, and BK drafted the manuscript which was approved by all authors. All authors collected, analyzed and/or interpreted the data. RM conceived the overall study design.
This study was funded by the Duke Center for Hyperbaric Medicine and Environmental Physiology.
The authors are grateful to Claude Piantadosi for his guidance on study design and analysis. We are also grateful for the technical assistance of Eric Schinazi, Aaron Walker, and Kenny Parker, and for the collaborations of Mona Parikh and Brye Roberts.
The authors declare that the research was conducted in the absence of any commercial or financial relationships that could be construed as a potential conflict of interest.
All claims expressed in this article are solely those of the authors and do not necessarily represent those of their affiliated organizations, or those of the publisher, the editors and the reviewers. Any product that may be evaluated in this article, or claim that may be made by its manufacturer, is not guaranteed or endorsed by the publisher.
Bennett-Guerrero E., Lockhart E. L., Bandarenko N., Campbell M. L., Natoli M. J., Jamnik V. K., et al. (2017). A randomized controlled pilot study of VO2 max testing: a potential model for measuring relative in vivo efficacy of different red blood cell products. Transfusion 57 (3), 630–636. doi:10.1111/trf.13918
Bennett-Guerrero E., Rizwan S., Rozensky R., Romeiser J. L., Brittelli J., Makaryus R., et al. (2021). Randomized controlled trial of 7, 28, vs 42 day stored red blood cell transfusion on oxygen delivery (VO2 max) and exercise duration. Transfusion 61 (3), 699–707. doi:10.1111/trf.16237
Broskey N. T., Boss A., Fares E. J., Greggio C., Gremion G., Schluter L., et al. (2015). Exercise efficiency relates with mitochondrial content and function in older adults. Physiol. Rep. 3 (6), e12418. doi:10.14814/phy2.12418
Burgos C., Henriquez-Olguin C., Andrade D. C., Ramirez-Campillo R., Araneda O. F., White A., et al. (2016). Effects of exercise training under hyperbaric oxygen on oxidative stress markers and endurance performance in young soccer players: a pilot study. J. Nutr. Metab. 2016, 5647407. doi:10.1155/2016/5647407
Cabric M., Medved R., Denoble P., Zivkovic M., Kovacevic H. (1991). Effect of hyperbaric oxygenation on maximal aerobic performance in a normobaric environment. J. Sports Med. Phys. Fit. 31 (3), 362–366.
Calvo J. A., Daniels T. G., Wang X., Paul A., Lin J., Spiegelman B. M., et al. (2008). Muscle-specific expression of PPARgamma coactivator-1alpha improves exercise performance and increases peak oxygen uptake. J. Appl. Physiol. (1985) 104 (5), 1304–1312. doi:10.1152/japplphysiol.01231.2007
Clark-Matott J., Saleem A., Dai Y., Shurubor Y., Ma X., Safdar A., et al. (2015). Metabolomic analysis of exercise effects in the POLG mitochondrial DNA mutator mouse brain. Neurobiol. Aging 36 (11), 2972–2983. doi:10.1016/j.neurobiolaging.2015.07.020
DeCato T. W., Bradley S. M., Wilson E. L., Harlan N. P., Villela M. A., Weaver L. K., et al. (2019). Effects of sprint interval training on cardiorespiratory fitness while in a hyperbaric oxygen environment. Undersea Hyperb. Med. 46 (2), 117–124. doi:10.22462/04.06.2019.4
di Prampero P. E. (2003). Factors limiting maximal performance in humans. Eur. J. Appl. Physiol. 90 (3-4), 420–429. doi:10.1007/s00421-003-0926-z
Garatachea N., Pareja-Galeano H., Sanchis-Gomar F., Santos-Lozano A., Fiuza-Luces C., Moran M., et al. (2015). Exercise attenuates the major hallmarks of aging. Rejuvenation Res. 18 (1), 57–89. doi:10.1089/rej.2014.1623
Gaskill S. E., Ruby B. C., Walker A. J., Sanchez O. A., Serfass R. C., Leon A. S. (2001). Validity and reliability of combining three methods to determine ventilatory threshold. Med. Sci. Sports Exerc. 33 (11), 1841–1848. doi:10.1097/00005768-200111000-00007
Gibala M. J., McGee S. L., Garnham A. P., Howlett K. F., Snow R. J., Hargreaves M. (2009). Brief intense interval exercise activates AMPK and p38 MAPK signaling and increases the expression of PGC-1alpha in human skeletal muscle. J. Appl. Physiol. (1985) 106 (3), 929–934. doi:10.1152/japplphysiol.90880.2008
Gifford J. R., Garten R. S., Nelson A. D., Trinity J. D., Layec G., Witman M. A., et al. (2016). Symmorphosis and skeletal muscle VO2 max : in vivo and in vitro measures reveal differing constraints in the exercise-trained and untrained human. J. Physiol. 594 (6), 1741–1751. doi:10.1113/JP271229
Gill M., Natoli M. J., Vacchiano C., MacLeod D. B., Ikeda K., Qin M., et al. (2014). Effects of elevated oxygen and carbon dioxide partial pressures on respiratory function and cognitive performance. J. Appl. Physiol. (1985) 117 (4), 406–412. doi:10.1152/japplphysiol.00995.2013
Groger M., Oter S., Simkova V., Bolten M., Koch A., Warninghoff V., et al. (2009). DNA damage after long-term repetitive hyperbaric oxygen exposure. J. Appl. Physiol. (1985) 106 (1), 311–315. doi:10.1152/japplphysiol.90737.2008
Gutsaeva D. R., Suliman H. B., Carraway M. S., Demchenko I. T., Piantadosi C. A. (2006). Oxygen-induced mitochondrial biogenesis in the rat hippocampus. Neuroscience 137 (2), 493–504. doi:10.1016/j.neuroscience.2005.07.061
Hadanny A., Hachmo Y., Rozali D., Catalogna M., Yaakobi E., Sova M., et al. (2022). Effects of hyperbaric oxygen therapy on mitochondrial respiration and physical performance in middle-aged athletes: A blinded, randomized controlled trial. Sports Med. Open 8 (1), 22. doi:10.1186/s40798-021-00403-w
Hazell T. J., Macpherson R. E., Gravelle B. M., Lemon P. W. (2010). 10 or 30-s sprint interval training bouts enhance both aerobic and anaerobic performance. Eur. J. Appl. Physiol. 110 (1), 153–160. doi:10.1007/s00421-010-1474-y
Hsu H. T., Yang Y. L., Chang W. H., Fang W. Y., Huang S. H., Chou S. H., et al. (2022). Hyperbaric oxygen therapy improves Parkinson's disease by promoting mitochondrial biogenesis via the SIRT-1/PGC-1α pathway. Biomolecules 12 (5), 661. doi:10.3390/biom12050661
Jamieson D., Chance B., Cadenas E., Boveris A. (1986). The relation of free radical production to hyperoxia. Annu. Rev. Physiol. 48, 703–719. doi:10.1146/annurev.ph.48.030186.003415
Kawada S., Fukaya K., Ohtani M., Kobayashi K., Fukusaki C. (2008). Effects of pre-exposure to hyperbaric hyperoxia on high-intensity exercise performance. J. Strength Cond. Res. 22 (1), 66–74. doi:10.1519/JSC.0b013e31815eefa2
Koch A. E., Koch I., Kowalski J., Schipke J. D., Winkler B. E., Deuschl G., et al. (2013). Physical exercise might influence the risk of oxygen-induced acute neurotoxicity. Undersea Hyperb. Med. 40 (2), 155–163.
Laursen P. B., Jenkins D. G. (2002). The scientific basis for high-intensity interval training: optimising training programmes and maximising performance in highly trained endurance athletes. Sports Med. 32 (1), 53–73. doi:10.2165/00007256-200232010-00003
Lawler J., Powers S. K., Thompson D. (1988). Linear relationship between VO2max and VO2max decrement during exposure to acute hypoxia. J. Appl. Physiol. (1985) 64 (4), 1486–1492. doi:10.1152/jappl.1988.64.4.1486
Little J. P., Safdar A., Wilkin G. P., Tarnopolsky M. A., Gibala M. J. (2010). A practical model of low-volume high-intensity interval training induces mitochondrial biogenesis in human skeletal muscle: potential mechanisms. J. Physiol. 588 (6), 1011–1022. doi:10.1113/jphysiol.2009.181743
McGavock J. M., Lecomte J. L., Delaney J. S., Lacroix V. J., Hardy P., Montgomery D. L. (1999). Effects of hyperbaric oxygen on aerobic performance in a normobaric environment. Undersea Hyperb. Med. 26 (4), 219–224.
Olesen J., Kiilerich K., Pilegaard H. (2010). PGC-1alpha-mediated adaptations in skeletal muscle. Pflugers Arch. 460 (1), 153–162. doi:10.1007/s00424-010-0834-0
Pecorella S. R., Potter J. V., Cherry A. D., Peacher D. F., Welty-Wolf K. E., Moon R. E., et al. (2015). The HO-1/CO system regulates mitochondrial-capillary density relationships in human skeletal muscle. Am. J. Physiol. Lung Cell. Mol. Physiol. 309 (8), L857–L871. doi:10.1152/ajplung.00104.2015
Piantadosi C. A., Suliman H. B. (2012). Redox regulation of mitochondrial biogenesis. Free Radic. Biol. Med. 53 (11), 2043–2053. doi:10.1016/j.freeradbiomed.2012.09.014
Pilegaard H., Saltin B., Neufer P. D. (2003). Exercise induces transient transcriptional activation of the PGC-1alpha gene in human skeletal muscle. J. Physiol. 546 (3), 851–858. doi:10.1113/jphysiol.2002.034850
Rhodes M. A., Carraway M. S., Piantadosi C. A., Reynolds C. M., Cherry A. D., Wester T. E., et al. (2009). Carbon monoxide, skeletal muscle oxidative stress, and mitochondrial biogenesis in humans. Am. J. Physiol. Heart Circ. Physiol. 297 (1), H392–H399. doi:10.1152/ajpheart.00164.2009
Roberts D. J., Miyamoto S. (2015). Hexokinase II integrates energy metabolism and cellular protection: akting on mitochondria and TORCing to autophagy. Cell Death Differ. 22 (2), 364–457. doi:10.1038/cdd.2014.208
Rozenek R., Fobel B. F., Banks J. C., Russo A. C., Lacourse M. G., Strauss M. B. (2007). Does hyperbaric oxygen exposure affect high-intensity, short-duration exercise performance? J. Strength Cond. Res. 21 (4), 1037–1041. doi:10.1519/R-21296.1
St-Pierre J., Drori S., Uldry M., Silvaggi J. M., Rhee J., Jager S., et al. (2006). Suppression of reactive oxygen species and neurodegeneration by the PGC-1 transcriptional coactivators. Cell 127 (2), 397–408. doi:10.1016/j.cell.2006.09.024
Suliman H. B., Carraway M. S., Ali A. S., Reynolds C. M., Welty-Wolf K. E., Piantadosi C. A. (2007). The CO/HO system reverses inhibition of mitochondrial biogenesis and prevents murine doxorubicin cardiomyopathy. J. Clin. Invest. 117 (12), 3730–3741. doi:10.1172/JCI32967
Suliman H. B., Carraway M. S., Tatro L. G., Piantadosi C. A. (2007). A new activating role for CO in cardiac mitochondrial biogenesis. J. Cell Sci. 120 (2), 299–308. doi:10.1242/jcs.03318
Takemura A., Ishihara A. (2017). Mild hyperbaric oxygen inhibits growth-related decrease in muscle oxidative capacity of rats with metabolic syndrome. J. Atheroscler. Thromb. 24 (1), 26–38. doi:10.5551/jat.34686
Keywords: high-intensity interval training, hyperbaric oxygenation, mitochondrial turnover, high-altitude, oxygen consumption
Citation: Alvarez Villela M, Dunworth SA, Kraft BD, Harlan NP, Natoli MJ, Suliman HB and Moon RE (2022) Effects of high-intensity interval training with hyperbaric oxygen. Front. Physiol. 13:963799. doi: 10.3389/fphys.2022.963799
Received: 07 June 2022; Accepted: 27 July 2022;
Published: 19 August 2022.
Edited by:
Giuseppe De Vito, University of Padua, ItalyReviewed by:
Graham Ripley McGinnis, University of Nevada, United StatesCopyright © 2022 Alvarez Villela, Dunworth, Kraft, Harlan, Natoli, Suliman and Moon. This is an open-access article distributed under the terms of the Creative Commons Attribution License (CC BY). The use, distribution or reproduction in other forums is permitted, provided the original author(s) and the copyright owner(s) are credited and that the original publication in this journal is cited, in accordance with accepted academic practice. No use, distribution or reproduction is permitted which does not comply with these terms.
*Correspondence: Richard E. Moon, cmljaGFyZC5tb29uQGR1a2UuZWR1
†These authors have contributed equally to this work and share first authorship
Disclaimer: All claims expressed in this article are solely those of the authors and do not necessarily represent those of their affiliated organizations, or those of the publisher, the editors and the reviewers. Any product that may be evaluated in this article or claim that may be made by its manufacturer is not guaranteed or endorsed by the publisher.
Research integrity at Frontiers
Learn more about the work of our research integrity team to safeguard the quality of each article we publish.