- 1Department of Orthopaedics, Xuancheng Central Hospital, Xuancheng, China
- 2Department of Orthopaedics, Xiangya Hospital, Central South University, Changsha, China
Osteoarthritis (OA) is the most prevalent joint disease, characterized by the degradation of articular cartilage, synovial inflammation, and changes in periarticular and subchondral bone. Recent studies have reported that Wnt signaling cascades play an important role in the development, growth, and homeostasis of joints. The Wnt signaling cascade should be tightly regulated to maintain the homeostasis of cartilage in either the over-activation or the suppression of Wnt/β-catenin, as this could lead to OA. This review summarizes the role and mechanism of canonical Wnt cascade and noncanonical Wnt cascade experiments in vivo and in vitro. The Wnt cascade is controlled by several agonists and antagonists in the extracellular medium and the cytoplasm. These antagonists and agonists serve as key molecules in drug intervention into the Wnt pathway and may provide potential approaches for the treatment of OA. However, the complexity of the Wnt signaling cascade and the pharmaceutical effects on its mechanism are still not fully understood, which forces us to conduct further research and develop efficient therapeutic approaches to treat OA.
Introduction
Osteoarthritis (OA) is the most prevalent joint disease, characterized by breakdown of the articular cartilage, abnormal bone remodeling, and osteophytosis that lead to chronic pain and functional restrictions of the affected joints. Radiographic evidence of OA is found in the majority of people by 65 years old, and approximately 80% of people aged over 75 years old are affected (Arden and Nevitt, 2006). A large number of studies have reported the incidence of OA, but its pathogenesis has not been fully elucidated.
Articular cartilage degeneration is a major cause of pathological changes in OA. Cartilage is an avascular, neural, lymphatic, and viscoelastic connective tissue, and its main function is to bear loads, promoting the frictionless movement of the joints (Kuettner et al., 1991). Chondrocytes, the only cell population in adult articular cartilage, are capable of responding to structural changes, including the synthesis of collagen and the degeneration of the extracellular matrix (ECM), although the capacity of adult articular chondrocytes to regenerate normal cartilage matrix architecture is limited and declines with age (Loeser, 2009; Tian et al., 2015). Collagens, mainly type II collagen and aggrecan, are the main components of articular cartilage (Roughley, 2001; Lambert et al., 2019). The degeneration of articular cartilage is thought to be due to a disturbance of the balance between the synthesis and metabolism activity, including decreasing the synthesis of type II collagen and proteoglycan and increasing the synthesis of matrix metalloproteinases (MMPs), thrombospondin motifs, and inflammatory factor (van der Kraan and van den Berg, 2000; Davidson et al., 2006; van der Kraan and van den Berg, 2012; Wiegertjes et al., 2019).
Structure and function of Wnt protein
Wnt signaling cascades are among the most critical biological pathways and are involved in several processes, including joint formation during embryonic skeleton genesis and joint homeostasis and disease in postnatal life (Ladher et al., 2000; Hartmann, 2007; Clevers and Nusse, 2012). Wnts are proteins that have a molecular weight of 36–49 kDa, and their N-terminal peptide contains 350–400 amino acids (Sassi et al., 2014a). At present, Wnt family consists of at least 19 known members in mammals that bind to different intracellular receptors and activate several downstream pathways (Lories et al., 2013; Sassi et al., 2014a). Among the Wnt proteins, the function of Wnt3a was the first to be defined (Willert et al., 2003).
The activation of a Wnt pathway requires the interaction of Wnt proteins with two receptors, one from the Fzl family and the other from the lipoprotein receptor-related protein (LRP) family. The Fzl receptors are generally grouped into seven types belonging to the G-protein–receptor family, and the N-termini of Fzls are extracellular and rich in cysteine, which can interact directly with Wnt protein (Bhanot et al., 1996; Yang-Snyder et al., 1996; Foord et al., 2005). LRP is a transmembrane protein and is considered a coreceptor protein of the Wnt pathway that can transduce canonical Wnt signals via interactions with axin (Mao et al., 2001; Barik et al., 2014).
Several proteins are involved in the Wnt cascade in the cytoplasm. Axin interacts with the C-terminal region of the coreceptor LRP and it antagonizes the intracellular level of Wnt signaling (Zeng et al., 1997; Staal and Clevers, 2000). Adenomatous polyposis coli (APC) is another protein in the cytoplasm, which is combined with axin, disheveled (Dvl), and casein kinase I (CK-I) to form a degeneration complex. It plays an important role in maintaining the balance of β-catenin by degrading excess β-catenin (Munemitsu et al., 1995; Behrens et al., 1998; Staal and Clevers, 2000). β-Catenin is a key mediator for the Wnt canonical cascade and plays a critical role in cell adhesion and interactions among cells (Lories et al., 2013).
The canonical cascade and noncanonical cascades
The canonical cascade is dependent on the activation of β-catenin. The Wnt proteins, the Fzl receptor family, and LRP form a complex that activates the downstream canonical β-catenin pathway. Moreover, Wnt-receptor complexes are mediated and controlled by downstream degradation complexes composed of APC, axin, and glycogen synthase kinase-3 β (GSK-3β) (Behrens et al., 1998; Staal and Clevers, 2000; Li et al., 2012). Once a Wnt protein binds to the Fzl receptor and the LRP protein, the degeneration complex is inhibited in the cytoplasm, while the complex formed by Wnt and its receptors binds to β-catenin, allowing β-catenin to accumulate in the cytoplasm and translocate to the nucleus (Rao and Kuhl, 2010; van den Bosch et al., 2016; De Santis et al., 2018). After translocation, β-catenin interacts with transcription factors from the LEF/TCF family, which is composed of TCF-1, LEF-1, TCF-3, and TCF-4 (Clevers and Nusse, 2012; Yu and Virshup, 2014; Bengoa-Vergniory and Kypta, 2015). Then, β-catenin in the nucleus blocks transcription of the target genes. If the Wnt protein does not bind to the Fzl receptor or the LRP protein, the excess β-catenin proteins are degraded by the destruction complex in the cytoplasm (Figure 1) (Johnson and Kamel, 2007).
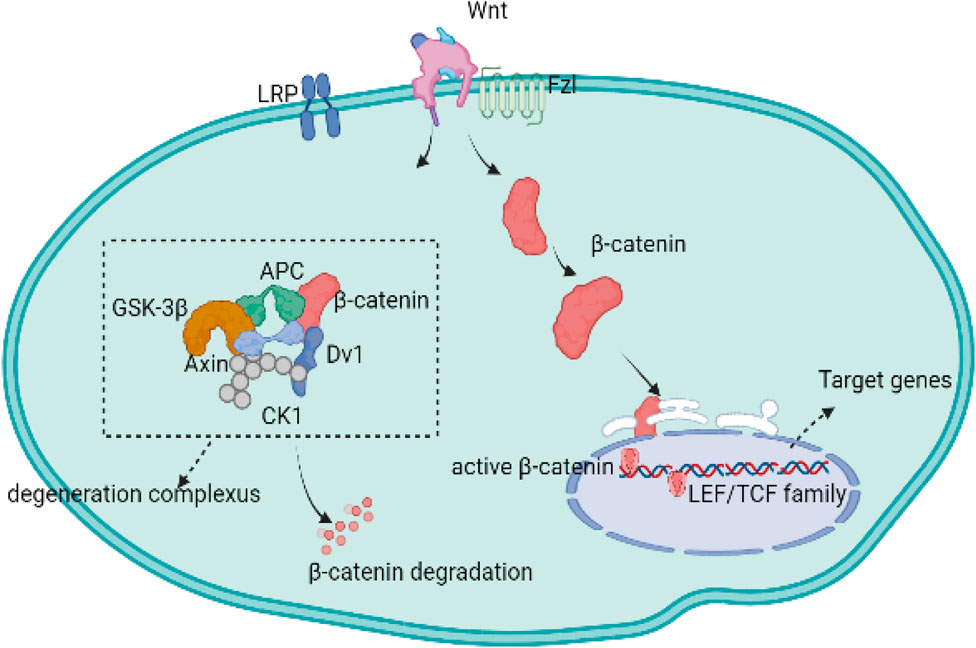
FIGURE 1. The canonical Wnt signaling pathway is dependent on the activation of intracellular molecule β-catenin. In the absence of Wnt-binding Fz receptors, β-catenin is isolated into a protein, phosphorylated, and subsequently degraded by the destruction complex. Upon binding to the Fzl receptors and LRP5/6 co-receptors, the Wnt/β-catenin signaling pathways is activated while the degradation complex of β-catenin is inhibited, allowing the newly synthesized β-catenin to accumulate in the cytoplasm and transfer to the nucleus. Nuclear β-catenin can replace the transcription corepressors in TCF transcription factors and promote the activation of gene transcription programs.
The noncanonical signaling pathways do not depend on the activation of β-catenin. Although the noncanonical Wnt signaling pathway plays an important role in the regulation of cell shape, adhesion, transfer, differentiation, and communication, little research has been reported on these pathways (Sugimura and Li, 2010; Wallingford and Mitchell, 2011; Clark et al., 2012). Under some conditions, it may even lead to the suppression of β-catenin (Ishitani et al., 2003). The noncanonical cascade is largely regulated by several extracellular and intracellular signaling molecules, including G-protein-coupled receptors, c-Jun N-terminal kinase (JNK), p38, and triphosphate (IP3)-intercellular calcium (Sugimura and Li, 2010). The receptors involved in the noncanonical cascade mainly include Frizzled, receptor tyrosine kinase (Ryk), and tyrosine-protein kinase transmembrane receptor (Ror2) (Usami et al., 2016).
The noncanonical cascade includes two types of signaling pathways, grouped according to the molecules regulated in the pathways. One cascade is WNT/PCP through Rho and JNK (c-jun N-terminal kinase), leading to the activation of downstream kinases, such as mitogen-activated kinases, including c-Jun, N-terminal kinase (JNK), and protein kinase, which play an important role in cell migration; the other cascade is WNT/Ca2+, through protein kinase C (PKC) and calcium/calmodulin-dependent kinase II (CaMK II), resulting in intracellular calcium release (Figure 2) (Liu et al., 2008; Sugimura and Li, 2010; Teo and Kahn, 2010; van Amerongen, 2012).
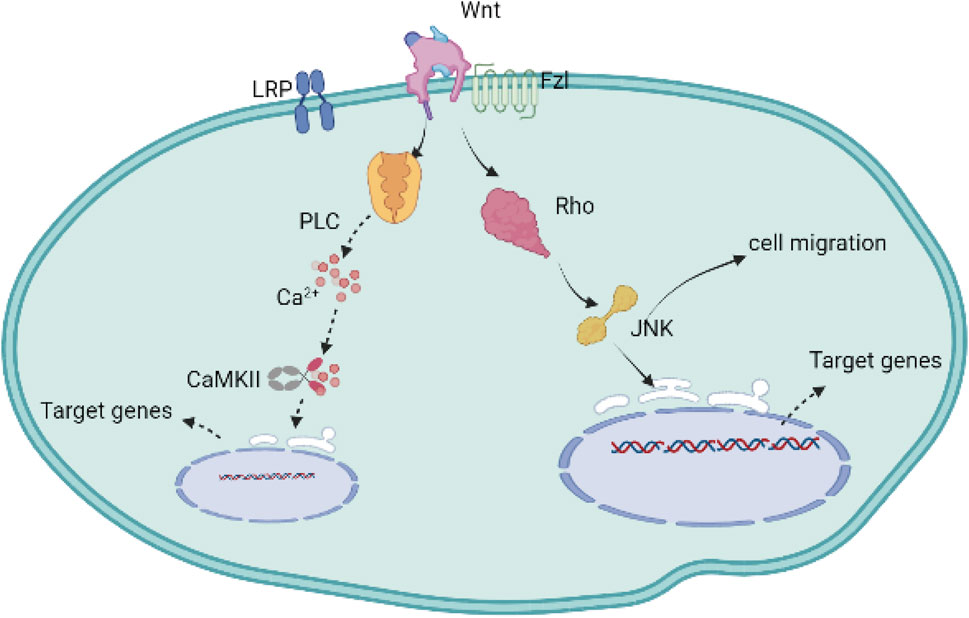
FIGURE 2. The non-canonical Wnt signaling cascade is activated without the involvement and activation of β-catenin. The Wnt protein binds to Fzl and other ligand receptors without the engagement of the LRP co-receptors. One of these cascades is triggered by the stimulation of the intracellular Ca2+ secondary to the production of the cytoplasmic PLC, subsequently activating the transcription factors in cell nucleus through the regulation of CaMKII; the other non-canonical Wnt signaling cascade relies on the increase of Rho following the activation of mitogen-activated kinases such as c-Jun N-terminal kinase (JNK), targeting the genes, and regulating cell proliferation and migration.
Role of Wnts in OA
A large number of studies have studied the role of Wnt in OA; even so, the mechanisms of Wnt protein have not been fully understood. The function of Wnts in OA is reflected in its ability to affect bone formation, endochondral ossification, bone growth and repair, and joint development (Lories et al., 2013). Bone growth requires chondrocytes to go through a complex process, including proliferation, migration, condensation, and adherence. During this process, chondrogenic differentiation passes through different stages in which the skeleton “model” is laid down, and the cells proliferate and become further differentiated via hypertrophy (Lefebvre and Bhattaram, 2010; Lories et al., 2013). Wnt3a can promote chondrocyte hypertrophy and differentiation through the canonical Wnt cascade, and suppression of the expression of Wnt3a could inhibit chondrogenic hypertrophy and EXT1 gene expression; furthermore, the Wnt/β-catenin pathway can regulate the expression of the EXT1 gene (Wang et al., 2019). Studies have indicated that Wnt5a and Wnt5b can induce chondrogenesis to affect chondrocyte proliferation and differentiation through the expression of the cytokines cyclinD1 and p130 (Ladher et al., 2000; Yang et al., 2003; Ge et al., 2017). Church et al. (2002) found that Wnt5a and Wnt5b promote early chondrogenesis through the activation of the Wnt noncanonical signaling pathway. Huang et al. (Shi et al., 2016; Huang et al., 2017) found that Wnt5a could activate the Wnt/β-catenin pathway, which leads to increased release of inflammatory mediators, aggravated cartilage damage and inflammatory responses, and accelerated OA process. The Wnt/β-catenin pathway also affects the activities of osteoblasts and osteoclasts, which play an important role in bone growth and remodeling. The osteocytes can produce Wnt protein and stimulate osteocyte maturation; however, they also produce sclerostin, which competitively antagonizes Wnt protein and binds to the LRP receptor (Li et al., 2005; Robling et al., 2008). Wnt proteins such as Wnt7b, Wnt-receptor LRP, and Wnt antagonist make significant contributions to activating the Wnt pathways and regulating osteocyte maturation and bone growth (Li et al., 2005; Robling et al., 2008).
A previous study also found that Wnt7b is closely related to inflammation in articular cartilage, bone, and synovial tissues derived from OA and RA patients (Nakamura et al., 2005). Wnt7a also plays an important role in the pathological process of OA; it could inhibit IL-1β-induced catabolic gene expression and prevent articular cartilage damage in experimental osteoarthritis. Meanwhile, Wnt7a decreased during human chondrocyte dedifferentiation with both no treatment and IL-1 treatment in vitro, while Wnt5a presented the opposite result against Wnt7a in vitro (Sassi et al., 2014b; Gibson et al., 2017).
Although a series of studies reported that most Wnts could activate the Wnt cascade and noncascade pathway, resulting in cartilage degeneration, Wnt16 could activate Wnt signaling and prevent the exacerbation of cartilage breakdown through exaggerated Wnt activation (Nalesso et al., 2017; Kovacs et al., 2019). Tong et al. (Nalesso et al., 2017; Tong et al., 2019) found that Wnt16 overexpression in chondrocytes in mice significantly inhibits chondrocyte hypertrophy in skeletal development and that Wnt16 activates the PCP/JNK pathway to inhibit chondrocyte hypertrophy. Hua et al. (2022) showed that Wnt16 is a weak activator of β-catenin and that upregulating Wnt16 levels could reduce disease progression through the Wnt/β-catenin pathway in temporomandibular OA.
Due to the large number of studies devoted to investigating the role of Wnt molecules and their influence in OA, our understanding of Wnt biology has rapidly increased, as summarized in Table 1. Although several studies have emphasized the important role of Wnt signaling in bone and joint development, numerous studies have also suggested that the inhibition of Wnts and the Wnt-related pathway is beneficial for OA. Due to the complexity and diversity of Wnt signaling in different cells in joints, abnormally high Wnt activity or low expression of the Wnt signaling pathway in OA may lead to cartilage damage and ultimately accelerate OA (Figure 3). It is necessary and important to maintain balanced biological activity of the Wnt-related pathway due to the complexity of these communication systems in OA.
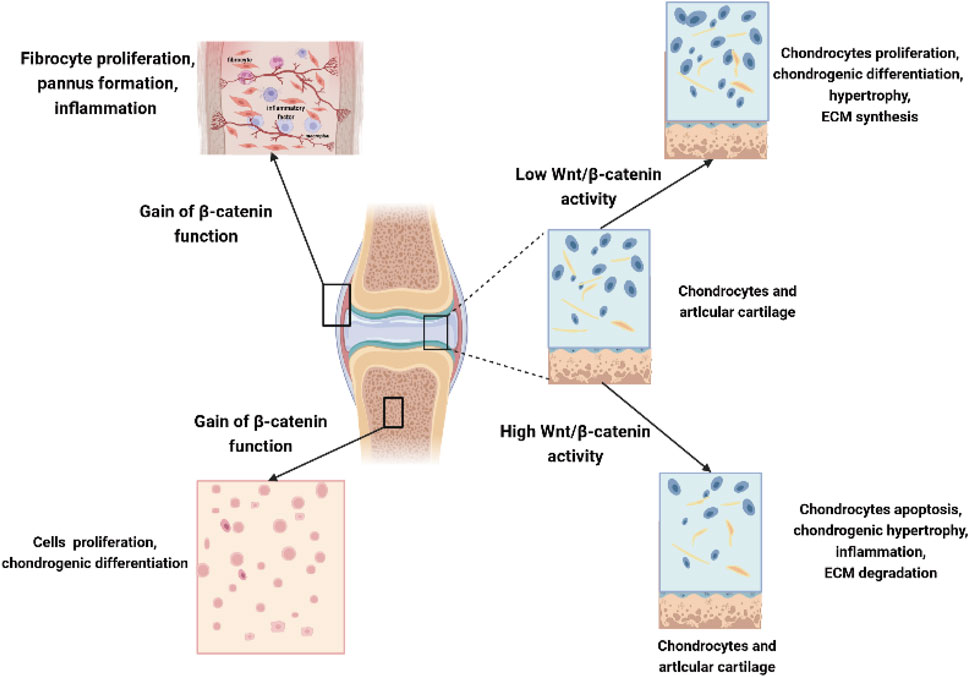
FIGURE 3. Complex role of Wnts in chondrocytes, synoviocytes, and mesenchymal stem cells. Low Wnt/β-catenin activity in chondrocytes promotes chondrocyte proliferation, chondrogenic differentiation, chondrocyte hypertrophy, and increased ECM synthesis, while high Wnt/β-catenin activity leads to chondrocyte apoptosis, hypertrophy, increased inflammation, and degradation of ECM in joints. Overexpression β-catenin contributes to the fibrocyte proliferation, pannus formation, and local inflammation in synoviocytes; moreover, it could also promote the proliferation and chondrogenic differentiation of MSCs in joints.
The role of exosomes in Wnt signaling in OA
In recent years, exosomes are increasingly reported as part of a therapeutic strategy in OA and as having a role in regulating the renewal and regenerative capabilities of chondrocytes. Exosomes are derived from a variety of cell types with 30–100 nm cup-shaped vesicles in diameter (Wu et al., 2020). Exosomes are released from protocells by fusion with the plasma membrane, and they are mainly composed of coding and noncoding RNAs, proteins, antigen-presenting molecules, and DNA (Zhou et al., 2020; Moghiman et al., 2021). Because of their size and composition, exosomes are important therapeutic agents for many diseases and are useful materials for tissue engineering. At present, exosomes are thought to be among the most important factors regulating inflammatory activities in many diseases, such as OA.
OA is influenced by multiple cell types, including chondrocytes and synovial fibroblasts, and both of these cells are regulated by the Wnt signaling pathways and have the ability to secrete exosomes and regulate cartilage metabolism (Kato et al., 2014; Chen et al., 2018). Several researchers have found that exosomes derived from a variety of cells could influence the progression of OA through the Wnt signaling pathway (Table 2). A recent study found that exosomes derived from platelet-rich plasma could promote chondrocyte proliferation and migration and decrease OA chondrocyte apoptosis by inhibiting the expression of Wnt5a/β-catenin in IL-1β-treated chondrocytes (Liu et al., 2019). Zhao et al. (2020) also indicated the role of exosomes in suppressing inflammation by decreasing the proinflammatory markers IL-6, NF-κB, and tumor necrosis factor alpha (TNF-α) and by upregulating the anti-inflammatory cytokine IL-10 level by activating β-catenin. Moreover, animal experiments indicated that exosomes could inhibit the progression of early OA and prevent severe damage to articular cartilage by targeting the Wnt5a gene and promoting chondrocyte proliferation and migration in vitro (Mao et al., 2018). However, other scholars found that exosomes extracted from synovial mesenchymal stem cells go beyond contributing to the proliferation and migration of chondrocytes by causing the side effect of degrading the cartilage matrix. The Wnt molecules Wnt5a and Wnt5b target the downstream protein of YAP/TAZ and are responsible for this process through noncanonical Wnt cascades (Tao et al., 2017). These studies indicate that the Wnt signaling pathway is an important regulatory mechanism for exosomes in decreasing inflammatory activity and chondrocyte apoptosis, as well as promoting cell proliferation and alleviating articular cartilage damage in OA.
Role of miRNAs in the Wnt signaling pathway in OA
MicroRNAs (miRNAs) are small noncoding RNAs with the capability of modulating essential biological processes in animals and plants. Since they were first discovered in Caenorhabditis elegans in 1993, miRNAs have been found to participate in regulating posttranscriptional gene expression, either by inhibiting messenger RNA (mRNA) translation or by promoting mRNA degradation (Saliminejad et al., 2019). More importantly, the abnormal expression of miRNAs could affect the pathological process of various diseases, such as OA, due to their role in regulating and modulating gene expression, which is related to the various physiological and pathological mechanisms in chondrocytes.
In recent decades, miRNA levels, which are posttranscriptionally related, have been extensively studied in OA, and the results show that miRNAs have both protective and destructive effects on articular cartilage in OA. MicroRNAs influence multiple intracellular signaling pathways to alter chondrocyte status, and the Wnt pathway is one of the most important signaling pathways that are targeted by miRNAs in OA. In general, miRNAs can activate or suppress the Wnt signaling pathway by targeting its components, while Wnt signaling also negatively influences the expression of miRNAs. For these reasons, miRNAs and Wnt signaling pathways are especially important for maintaining cartilage metabolism, joint inflammation, chondrocyte proliferation, differentiation, and apoptosis in OA, as presented in Table 3.
As a key component of the Wnt signaling pathway, the activation of β-catenin depends on its transport and accumulation in the nucleus. Once it is in the nucleus, it can bind to TCF/LEF transcription factors to initiate gene transduction. Therefore, many scholars have studied the effect of miRNAs on β-catenin. Dong et al. (2021) found that miR-127-3p can protect chondrocytes from IL-1-induced damage by decreasing the expression of MMP-13, ADAMTS-5, TNF-α, and IL-6 by suppressing the activation of β-catenin. miR-138 has also been proven to have the same effect, alleviating OA cartilage severity by increasing the levels of Col2a1 and aggrecan and reducing MMP-13 levels by targeting the β-catenin gene (Xu et al., 2019). Cheleschi et al. (2017) investigated the effects of miRNAs in both normal and OA human chondrocytes in regulating the levels of MMP-13 and ADAMTS-4. The results showed that several miRNAs, such as miR-27a/b, miR-140, and miR-146a/b, are responsible for modulating the β-catenin level and the aforementioned changes in chondrocytes. Researchers found that miR-10a reduced β-catenin at both the protein and transcriptional levels and inhibited osteogenic differentiation to reduce cartilage damage in OA (Li et al., 2015), and other researchers found that the upregulation of β-catenin induced by miR-195-5p could promote the inflammatory activity and apoptosis rate of chondrocytes (Shu et al., 2019).
Wnt and its receptors can be targeted and modulated by miRNAs. MiR-410 expression is upregulated during the process of chondrogenic differentiation in MSCs and can increase the levels of Col2a1, Sox9, and ACAN by regulating the target gene Wnt3a (Zhang et al., 2017). Wnt3a is also a target gene for miR-497-5p, which regulates the activity of the Wnt signaling pathway in OA (Hou Liying et al., 2019). Indeed, elevated levels of miR-497-5p prominently increased Col2a1 and aggrecan expression and decreased the expression of the catabolic proteinases MMP-13 and ADAMTS-4, while Wnt3a overexpression reversed these effects (Hou Liying et al., 2019). Similarly, miR-92a-3p could reduce cartilage matrix synthesis by targeting Wnt5a and enhancing its expression (Mao et al., 2018). Ntoumou et al. (2017) investigated serum samples collected from healthy people and OA patients and found that miR-140-3p, miR-33b-3p, and miR-671-3p were downregulated in the serum of OA patients and modulated the metabolic processes of OA pathology by regulating the expression of the Wnt5a gene.
Many studies have reported that miRNAs could participate in the regulation of biological processes by regulating the expression level of antagonists of the Wnt signaling pathway. DKK-1 has been extensively studied, and it has been reported that miR-335-5p can downregulate DKK-1 and activate Wnt signaling to promote osteogenic differentiation in mice (Zhang et al., 2011). Colombini et al. (2021) studied the miRNA cargo embedded in extracellular vesicles (EVs) released from adipose-derived mesenchymal stromal cells. Intriguingly, they found that miR-520c-3p was upregulated, but miR-302d-3p was silenced, and both targeted DKK to activate Wnt signaling to modulate inflammatory functions.
Wnt signaling in OA experimental animal models
Some studies have investigated the role of the Wnt signaling pathway in the articular pathologies of OA. Ryu and Chun (2006) studied the effect of IL-1 on cultured rabbit chondrocytes in vitro. They found that IL-1 could increase the expression of Wnt5a, which is strongly related to cartilage destruction, by inhibiting collagen-II in OA. Blom et al. (2009) studied the expression of the Wnt and Fzl genes in experimental OA and human OA. They found that Wnt and Wnt-related genes are highly expressed in the articular cartilage and synovium in experimental OA and human OA. Among these genes, the Wisp1 gene, which induces Wnt gene expression, is significantly highly expressed in chondrocytes. Secreted frizzled-related proteins (sFRPs) are antagonists of the Wnt pathway and play an important role in the Wnt pathway; sFRP (−/−) mice are more liable to develop OA because of the increased activity of MMPs (Lories et al., 2007; Thysen et al., 2015). These findings indicate revealed the effect of the Wnt/β-catenin signaling pathway in OA. Chen et al. (Chen et al., 2008; Zhu et al., 2008; Zhu et al., 2009) confirmed that an increased β-catenin protein level in the nucleus is accompanied by a reduction in β-catenin degradation in chondrocytes. These changes could result in an OA-like phenotype, including chondrocyte differentiation, increased MMP and Col10a expression, loss of articular cartilage, and osteophyte formation.
The ADAMTS family of proteases and matrix metalloproteinase (MMPs) are strongly involved in the cartilage degradation of OA (Glasson et al., 2005; Roach et al., 2005; Majumdar et al., 2007). The deletion of ADAMTS-5 or MMP-13 or double KO of both ADAMTS-4 and ADAMTS-5 prevented cartilage degradation in a surgically induced knee OA mouse model, while the expression of ADAMTS-4 and ADAMTS-5 was increased in β-catenin-overexpressing mice (Majumdar et al., 2007; Wang et al., 2013; Yang et al., 2015).
Several studies have found that the levels of MMP-3, MMP-9, MMP-13, and ADAMTS-5 were closely related to those of Wnt/β-catenin, and inhibiting the expression of β-catenin could reduce the expression of MMPs and ADAMTS-5 and reduce the damage to articular cartilage in an animal OA model (Bai et al., 2019; Li et al., 2019; Xie et al., 2019). These findings revealed that MMPs and ADAMTS4/5 affect cartilage destruction and the progression of OA through the Wnt/β-catenin pathway, and targeting this pathway can be a strategy for treating or preventing OA.
Interventions in the Wnt cascade
The complexity of the Wnt signaling pathway means that regulating it is a complex and challenging process. Pharmaceutical interventions on the Wnt cascade have hitherto mainly focused on secreted Wnt antagonists. Because the mechanism of the Wnt signaling pathway requires a combination of Lrp, Fzl, and Wnt to initiate the downstream β-catenin molecule, their inhibitory actions on Wnt are different. They can be divided into endogenous Wnt pathway inhibitors and pharmaceutical inhibitors according to the mechanism of their action on the signaling pathway.
Endogenous inhibitors of the Wnt signaling pathway
The Wnt pathway is controlled by several endogenous antagonists, and the decreased expression levels of certain antagonists may alleviate the progression of OA. Our current understanding of certain endogenous antagonists of the Wnt signaling pathway in OA, as discussed in the following sections, is summarized in Table 4.
Sclerostin is an antagonist of the Wnt pathway and can antagonize the combination of Wnt with LRP and FZL. Sclerostin inhibits the expression levels of the downstream catabolic effector genes Runx-2, MMP-13, ADAMTS-4, and ADAMTS-5 by decreasing the mRNA expression levels of β-catenin in healthy chondrocytes, while sclerostin knockout mice exhibit increased expression of aggrecanases and Col10a (Lewiecki, 2014; Bouaziz et al., 2015; Wu et al., 2017). Dkk-1 is another Wnt antagonist, and its overexpression can reduce the degeneration of cartilage and OA in animal models induced by destabilization of the medial meniscus (DMM). Dkk-1 can inhibit Wnt-mediated expression of catabolic genes, including MMPs, Runx-2, and ADAMTS-4/5 (Oh et al., 2012; de Rooy et al., 2013; Funck-Brentano et al., 2014). Dkk-3 is another member of the Dkk family of Wnt antagonists, and it inhibits the loss of proteoglycan and collagen from cartilage in a model of OA (Snelling et al., 2016). A previous study indicated that the expression of Dkk-1 is related to inflammation and is consistent with the process of OA, while Dkk-2 and Dkk-3 expression is associated with late stages of the disease (Walsh et al., 2009). WNT-inhibitory factor 1 (WIF-1) is an extracellular Wnt antagonist that has been investigated in several studies. Bleil et al. (2015) found that WIF-1 could reduce the expression of β-catenin in the chondrocytes of OA, while other researchers found a similar tendency and confirmed that the WIF-1 level was negatively correlated with the severity of the disease in OA (Gao et al., 2016; Cassuto et al., 2018). The sFRP family, which has five members (SFRP1–5), was originally identified as an extracellular ligand-binding inhibitor of the Wnt signaling pathway (Thysen et al., 2015). More recent data indicate that SFRPs have a variety of physiological functions, including regulating cell metabolism and intracellular signaling by regulating or inhibiting the activity of the Wnt signaling pathway. The sFRP1 level strongly influences the synthesis of the cartilage matrix, and animal experiments showed that sFRP1(−/−) mice had greater subchondral bone plate thicknesses in a DMM model of OA (Thysen et al., 2015). sFRP3 also negatively regulated the key gene β-catenin in the Wnt signaling pathway in RA and decreased the expression of MMP-3 (Elhaj Mahmoud et al., 2017).
Pharmaceutical inhibitors of the Wnt signaling pathway
Recently, a number of new and classic drugs were investigated in terms of their inhibitory effect on the Wnt pathway in OA. Most of them influence the pathological process of OA by modulating Wnt pathway activity, as summarized in Table 5. Zhong et al. (2018) found that artemisinin (ART) could inhibit the expression of proinflammatory chemokines and cytokines, including IL-1β, IL-6, TNF-α, and MMP-13, suppressing the degeneration of the articular cartilage. These results indicate that ART alleviates the IL-1β-mediated inflammatory response and OA progression by regulating the Wnt5a/β-catenin signaling pathway. Mianserin suppresses Rspo2-induced accumulation of β-catenin and phosphorylation of LRP6, which play an important role in preventing OA progression in a rat model of OA (Okura et al., 2019). Growth differentiation factor-5 (GDF-5) is strongly related to ECM homeostasis. It could inhibit the expression of MMP-13 and ADAMTS-4 and stimulate the expression of the cartilage anabolic genes Acan and Sox9 in human chondrocytes through intervention in the Wnt canonical signaling pathway by the overexpression of Dkk-1 and FRZB (Enochson et al., 2014). Ma et al. (2019) demonstrated that a systemic injection of rapamycin could reduce the degeneration of cartilage by inhibiting β-catenin in an animal model of OA. The mechanism by which rapamycin attenuates OA mainly relies on a reduction of apoptosis and activation of autophagy in chondrocytes. Icariin exerts a chondroprotective effect by inhibiting the expression of β-catenin in vivo and in vitro, and it may have therapeutic potential for the treatment of OA (Zeng et al., 2014). Several researchers have found that the expression of some large molecules, such as FYN and high-molecular-weight fibroblast growth factor-2 (FGF-2), is elevated and correlated with the expression of Wnt/β-catenin in human OA cartilage and mouse models. Inhibiting these molecules can reduce the levels of the extracellular matrix catabolic enzymes and delay articular cartilage degeneration by blocking the β-catenin pathway (Zhao et al., 2015; Li et al., 2018; Meo Burt et al., 2018).
Conclusion
In recent years, there has been growing interest in trying to identify the mechanisms underlying pathological changes in OA for the purpose of developing a targeted treatment strategy for OA. Extensive studies of the Wnt signaling pathway have revealed multiple functions of the Wnt signaling cascade in the maintenance of joint homeostasis, particularly in cartilage. The Wnt cascade should be tightly regulated because either overexpression of Wnt signaling or blockade of its activation may result in cartilage damage and bone erosion. The Wnt signaling pathway involves several levels, including the extracellular, intracytoplasmic, and nuclear levels, so regulation should exist in multiple aspects. New strategies to target and control these different levels of regulatory mechanisms may lead to the preservation of cartilage or the healing of diseased joints. It is absolutely necessary to investigate the Wnt signaling pathway in greater detail, not only to develop specific therapeutic interventions that target this pathway but also to discover and utilize new biomarkers for personalizing medical approaches in the future.
Author contributions
All the authors researched the data for the study, provided substantial contributions to discussions of its content, wrote the manuscript, and reviewed and/or edited the manuscript before submission.
Conflict of interest
The authors declare that the research was conducted in the absence of any commercial or financial relationships that could be construed as a potential conflict of interest.
Publisher’s note
All claims expressed in this article are solely those of the authors and do not necessarily represent those of their affiliated organizations, or those of the publisher, the editors, and the reviewers. Any product that may be evaluated in this article, or claim that may be made by its manufacturer, is not guaranteed or endorsed by the publisher.
References
Arden N., Nevitt M. C. (2006). Osteoarthritis: Epidemiology. Best. Pract. Res. Clin. Rheumatol. 20 (1), 3–25. doi:10.1016/j.berh.2005.09.007
Bai M., Ge L., Chen H., Jin Q. (2019). Calcitonin protects rat chondrocytes from IL-1β injury via the Wnt/β-catenin pathway. Exp. Ther. Med. 18 (3), 2079–2085. doi:10.3892/etm.2019.7806
Barik A., Zhang B., Sohal G. S., Xiong W. C., Mei L. (2014). Crosstalk between agrin and Wnt signaling pathways in development of vertebrate neuromuscular junction. Dev. Neurobiol. 74 (8), 828–838. doi:10.1002/dneu.22190
Behrens J., Jerchow B. A., Wurtele M., Grimm J., Asbrand C., Wirtz R., et al. (1998). Functional interaction of an axin homolog, conductin, with beta-catenin, apc, and Gsk3beta. Science 280 (5363), 596–599. doi:10.1126/science.280.5363.596
Bengoa-Vergniory N., Kypta R. M. (2015). Canonical and noncanonical Wnt signaling in neural stem/progenitor cells. Cell. Mol. Life Sci. 72 (21), 4157–4172. doi:10.1007/s00018-015-2028-6
Bhanot P. B. M., Samos C. H., Hsieh J. C., Wang Y., Macke J. P., Andrew D., et al. (1996). A new member of the frizzled family from Drosophila functions as a wingless receptor. Nature 382 (6588), 225–230. doi:10.1038/382225a0
Bleil J., Sieper J., Maier R., Schlichting U., Hempfing A., Syrbe U., et al. (2015). Cartilage in facet joints of patients with ankylosing spondylitis (as) shows signs of cartilage degeneration rather than chondrocyte hypertrophy: Implications for joint remodeling in as. Arthritis Res. Ther. 17, 170. doi:10.1186/s13075-015-0675-5
Blom A. B., Brockbank S. M., van Lent P. L., van Beuningen H. M., Geurts J., Takahashi N., et al. (2009). Involvement of the Wnt signaling pathway in experimental and human osteoarthritis: Prominent role of wnt-induced signaling protein 1. Arthritis Rheum. 60 (2), 501–512. doi:10.1002/art.24247
Bouaziz W., Funck-Brentano T., Lin H., Marty C., Ea H. K., Hay E., et al. (2015). Loss of sclerostin promotes osteoarthritis in mice via beta-catenin-dependent and -independent Wnt pathways. Arthritis Res. Ther. 17 (1), 24. doi:10.1186/s13075-015-0540-6
Cassuto J., Folestad A., Gothlin J., Malchau H., Karrholm J. (2018). The key role of proinflammatory cytokines, matrix proteins, RANKL/OPG and Wnt/β-catenin in bone healing of hip arthroplasty patients. Bone 107, 66–77. doi:10.1016/j.bone.2017.11.004
Cheleschi S., De Palma A., Pecorelli A., Pascarelli N. A., Valacchi G., Belmonte G., et al. (2017). Hydrostatic pressure regulates MicroRNA expression levels in osteoarthritic chondrocyte cultures via the wnt/β-catenin pathway. Int. J. Mol. Sci. 18 (1), E133. doi:10.3390/ijms18010133
Chen M., Zhu M., Awad H., Li T. F., Sheu T. J., Boyce B. F., et al. (2008). Inhibition of beta-catenin signaling causes defects in postnatal cartilage development. J. Cell Sci. 121 (9), 1455–1465. doi:10.1242/jcs.020362
Chen Y., Xue K., Zhang X., Zheng Z., Liu K. (2018). Exosomes derived from mature chondrocytes facilitate subcutaneous stable ectopic chondrogenesis of cartilage progenitor cells. Stem Cell Res. Ther. 9 (1), 318. doi:10.1186/s13287-018-1047-2
Church V., Nohno T., Linker C., Marcelle C., Francis-West P. (2002). Wnt regulation of chondrocyte differentiation. J. Cell Sci. 115 (24), 4809–4818. doi:10.1242/jcs.00152
Clark C. E., Nourse C. C., Cooper H. M. (2012). The tangled web of non-canonical Wnt signalling in neural migration. Neurosignals. 20 (3), 202–220. doi:10.1159/000332153
Clevers H., Nusse R. (2012). Wnt/β-catenin signaling and disease. Cell 149 (6), 1192–1205. doi:10.1016/j.cell.2012.05.012
Colombini A., Ragni E., Mortati L., Libonati F., Perucca Orfei C., Vigano M., et al. (2021). Adipose-derived mesenchymal stromal cells treated with interleukin 1 beta produced chondro-protective vesicles able to fast penetrate in cartilage. Cells 10(5), 1180. doi:10.3390/cells10051180
Davidson R. K., Waters J. G., Kevorkian L., Darrah C., Cooper A., Donell S. T., et al. (2006). Expression profiling of metalloproteinases and their inhibitors in synovium and cartilage. Arthritis Res. Ther. 8 (4), R124. doi:10.1186/ar2013
de Rooy D. P., Yeremenko N. G., Wilson A. G., Knevel R., Lindqvist E., Saxne T., et al. (2013). Genetic studies on components of the Wnt signalling pathway and the severity of joint destruction in rheumatoid arthritis. Ann. Rheum. Dis. 72 (5), 769–775. doi:10.1136/annrheumdis-2012-202184
De Santis M., Di Matteo B., Chisari E., Cincinelli G., Angele P., Lattermann C., et al. (2018). The role of Wnt pathway in the pathogenesis of oa and its potential therapeutic implications in the field of regenerative medicine. Biomed. Res. Int. 2018, 7402947. doi:10.1155/2018/7402947
Dong J., Li L., Fang X., Zang M. (2021). Exosome-encapsulated microRNA-127-3p released from bone marrow-derived mesenchymal stem cells alleviates osteoarthritis through regulating CDH11-mediated wnt/β-catenin pathway. J. Pain Res. 14, 297–310. doi:10.2147/JPR.S291472
Elhaj Mahmoud D., Sassi N., Drissi G., Barsaoui M., Zitouna K., Sahli H., et al. (2017). Sfrp3 and Dkk1 regulate fibroblast-like synoviocytes markers and Wnt elements expression depending on cellular context. Immunol. Invest.. 46 (3), 314–328. doi:10.1080/08820139.2016.1267204
Enochson L., Stenberg J., Brittberg M., Lindahl A. (2014). Gdf5 reduces Mmp13 expression in human chondrocytes via Dkk1 mediated canonical Wnt signaling inhibition. Osteoarthr. Cartil. 22 (4), 566–577. doi:10.1016/j.joca.2014.02.004
Foord S. M., Bonner T. I., Neubig R. R., Rosser E. M., Pin J. P., Davenport A. P., et al. (2005). International union of pharmacology. Xlvi. G protein-coupled receptor list. Pharmacol. Rev. 57 (2), 279–288. doi:10.1124/pr.57.2.5
Funck-Brentano T., Bouaziz W., Marty C., Geoffroy V., Hay E., Cohen-Solal M. (2014). Dkk-1-Mediated inhibition of Wnt signaling in bone ameliorates osteoarthritis in mice. Arthritis Rheumatol. 66 (11), 3028–3039. doi:10.1002/art.38799
Gao S. G., Zeng C., Liu J. J., Tian J., Cheng C., Zhang F. J., et al. (2016). Association between Wnt inhibitory factor-1 expression levels in articular cartilage and the disease severity of patients with osteoarthritis of the knee. Exp. Ther. Med. 11 (4), 1405–1409. doi:10.3892/etm.2016.3049
Ge X., Shi R., Ma X. (2017). The secreted protein Wnt5a regulates condylar chondrocyte proliferation, hypertrophy and migration. Arch. Oral Biol. 82, 171–179. doi:10.1016/j.archoralbio.2017.06.019
Gibson A. L., Hui Mingalone C. K., Foote A. T., Uchimura T., Zhang M., Zeng L. (2017). Wnt7a inhibits IL-1β induced catabolic gene expression and prevents articular cartilage damage in experimental osteoarthritis. Sci. Rep. 7, 41823. doi:10.1038/srep41823
Glasson S. S., Askew R., Sheppard B., Carito B., Blanchet T., Ma H. L., et al. (2005). Deletion of active Adamts5 prevents cartilage degradation in a murine model of osteoarthritis. Nature 434 (7033), 644–648. doi:10.1038/nature03369
Hou Liying S. H., Wang M., Liu J. (2019). Liu guoqiang microrna-497-5p attenuates il-1β-induced cartilage matrix degradation in chondrocytes via wnt/Β-catenin signal pathway. Int. J. Clin. Exp. Pathol. 12 (8), 3108–3118.
Hua B., Qiu J., Ye X., Liu X. (2022). Intra-articular injection of a novel Wnt pathway inhibitor, Sm04690, upregulates Wnt16 expression and reduces disease progression in temporomandibular joint osteoarthritis. Bone 158, 116372. doi:10.1016/j.bone.2022.116372
Huang G., Chubinskaya S., Liao W., Loeser R. F. (2017). Wnt5a induces catabolic signaling and matrix metalloproteinase production in human articular chondrocytes. Osteoarthr. Cartil. 25 (9), 1505–1515. doi:10.1016/j.joca.2017.05.018
Ishitani T., Kishida S., Hyodo-Miura J., Ueno N., Yasuda J., Waterman M., et al. (2003). The tak1-nlk mitogen-activated protein kinase cascade functions in the wnt-5a/Ca(2+) pathway to antagonize wnt/beta-catenin signaling. Mol. Cell. Biol. 23 (1), 131–139. doi:10.1128/mcb.23.1.131-139.2003
Johnson M. L., Kamel M. A. (2007). The Wnt signaling pathway and bone metabolism. Curr. Opin. Rheumatol. 19 (4), 376–382. doi:10.1097/BOR.0b013e32816e06f9
Kato T., Miyaki S., Ishitobi H., Nakamura Y., Nakasa T., Lotz M. K., et al. (2014). Exosomes from IL-1β stimulated synovial fibroblasts induce osteoarthritic changes in articular chondrocytes. Arthritis Res. Ther. 16 (4), R163. doi:10.1186/ar4679
Kovacs B., Vajda E., Nagy E. E. (2019). Regulatory effects and interactions of the Wnt and opg-rankl-rank signaling at the bone-cartilage interface in osteoarthritis. Int. J. Mol. Sci. 20 (18), E4653. doi:10.3390/ijms20184653
Kuettner K. E., Aydelotte M. B., Thonar E. J. (1991). Articular cartilage matrix and structure: A minireview. J. Rheumatol. Suppl. 27 (27), 46–48.
Ladher R. K., Church V. L., Allen S., Robson L., Abdelfattah A., Brown N. A., et al. (2000). Cloning and expression of the Wnt antagonists sfrp-2 and frzb during chick development. Dev. Biol. 218 (2), 183–198. doi:10.1006/dbio.1999.9586
Lambert C., Borderie D., Dubuc J. E., Rannou F., Henrotin Y. (2019). Type ii collagen peptide coll2-1 is an actor of synovitis. Osteoarthr. Cartil. 27 (11), 1680–1691. doi:10.1016/j.joca.2019.07.009
Lefebvre V., Bhattaram P. (2010). Vertebrate skeletogenesis. Curr. Top. Dev. Biol. 90, 291–317. doi:10.1016/S0070-2153(10)90008-2
Lewiecki E. M. (2014). Role of sclerostin in bone and cartilage and its potential as a therapeutic target in bone diseases. Ther. Adv. Musculoskelet. Dis. 6 (2), 48–57. doi:10.1177/1759720X13510479
Li J., Han G., Ma M., Wei G., Shi X., Guo Z., et al. (2019). Xanthan gum ameliorates osteoarthritis and mitigates cartilage degradation via regulation of the wnt3a/β-catenin signaling pathway. Med. Sci. Monit. 25, 7488–7498. doi:10.12659/MSM.916092
Li J., Zhang Y., Zhao Q., Wang J., He X. (2015). Microrna-10a influences osteoblast differentiation and angiogenesis by regulating beta-catenin expression. Cell. Physiol. biochem. 37 (6), 2194–2208. doi:10.1159/000438576
Li K., Zhang Y., Zhang Y., Jiang W., Shen J., Xu S., et al. (2018). Tyrosine kinase fyn promotes osteoarthritis by activating the beta-catenin pathway. Ann. Rheum. Dis. 77 (6), 935–943. doi:10.1136/annrheumdis-2017-212658
Li V. S., Ng S. S., Boersema P. J., Low T. Y., Karthaus W. R., Gerlach J. P., et al. (2012). Wnt signaling through inhibition of beta-catenin degradation in an intact Axin1 complex. Cell 149 (6), 1245–1256. doi:10.1016/j.cell.2012.05.002
Li X., Zhang Y., Kang H., Liu W., Liu P., Zhang J., et al. (2005). Sclerostin binds to lrp5/6 and antagonizes canonical Wnt signaling. J. Biol. Chem. 280 (20), 19883–19887. doi:10.1074/jbc.M413274200
Liu F., Kohlmeier S., Wang C. Y. (2008). Wnt signaling and skeletal development. Cell. Signal. 20 (6), 999–1009. doi:10.1016/j.cellsig.2007.11.011
Liu X., Wang L., Ma C., Wang G., Zhang Y., Sun S. (2019). Exosomes derived from platelet-rich plasma present a novel potential in alleviating knee osteoarthritis by promoting proliferation and inhibiting apoptosis of chondrocyte via Wnt/β-catenin signaling pathway. J. Orthop. Surg. Res. 14 (1), 470. doi:10.1186/s13018-019-1529-7
Loeser R. F. (2009). Aging and osteoarthritis: The role of chondrocyte senescence and aging changes in the cartilage matrix. Osteoarthr. Cartil. 17 (8), 971–979. doi:10.1016/j.joca.2009.03.002
Lories R. J., Corr M., Lane N. E. (2013). To Wnt or not to Wnt: The bone and joint health dilemma. Nat. Rev. Rheumatol. 9 (6), 328–339. doi:10.1038/nrrheum.2013.25
Lories R. J., Peeters J., Bakker A., Tylzanowski P., Derese I., Schrooten J., et al. (2007). Articular cartilage and biomechanical properties of the long bones in frzb-knockout mice. Arthritis Rheum. 56 (12), 4095–4103. doi:10.1002/art.23137
Ma L., Liu Y., Zhao X., Li P., Jin Q. (2019). Rapamycin attenuates articular cartilage degeneration by inhibiting beta-catenin in a murine model of osteoarthritis. Connect. Tissue Res. 60 (5), 452–462. doi:10.1080/03008207.2019.1583223
Majumdar M. K., Askew R., Schelling S., Stedman N., Blanchet T., Hopkins B., et al. (2007). Double-knockout of adamts-4 and adamts-5 in mice results in physiologically normal animals and prevents the progression of osteoarthritis. Arthritis Rheum. 56 (11), 3670–3674. doi:10.1002/art.23027
Mao G., Zhang Z., Hu S., Zhang Z., Chang Z., Huang Z., et al. (2018). Exosomes derived from mir-92a-3p-overexpressing human mesenchymal stem cells enhance chondrogenesis and suppress cartilage degradation via targeting Wnt5a. Stem Cell Res. Ther. 9 (1), 247. doi:10.1186/s13287-018-1004-0
Mao J., Wang J., Liu B., Pan W., Farr G. H., Flynn C., et al. (2001). Low-density lipoprotein receptor-related protein-5 binds to axin and regulates the canonical Wnt signaling pathway. Mol. Cell 7 (4), 801–809. doi:10.1016/s1097-2765(01)00224-6
Meo Burt P., Xiao L., Hurley M. M. (2018). FGF23 regulates wnt/β-catenin signaling-mediated osteoarthritis in mice overexpressing high-molecular-weight FGF2. Endocrinology 159 (6), 2386–2396. doi:10.1210/en.2018-00184
Moghiman T., Barghchi B., Esmaeili S. A., Shabestari M. M., Tabaee S. S., Momtazi-Borojeni A. A. (2021). Therapeutic angiogenesis with exosomal micrornas: An effectual approach for the treatment of myocardial ischemia. Heart fail. Rev. 26 (1), 205–213. doi:10.1007/s10741-020-10001-9
Munemitsu S., Albert I., Souza B., Rubinfeld B., Polakis P. (1995). Regulation of intracellular beta-catenin levels by the adenomatous polyposis coli (apc) tumor-suppressor protein. Proc. Natl. Acad. Sci. U. S. A. 92 (7), 3046–3050. doi:10.1073/pnas.92.7.3046
Nakamura Y., Nawata M., Wakitani S. (2005). Expression profiles and functional analyses of wnt-related genes in human joint disorders. Am. J. Pathol. 167 (1), 97–105. doi:10.1016/S0002-9440(10)62957-4
Nalesso G., Thomas B. L., Sherwood J. C., Yu J., Addimanda O., Eldridge S. E., et al. (2017). Wnt16 antagonises excessive canonical Wnt activation and protects cartilage in osteoarthritis. Ann. Rheum. Dis. 76 (1), 218–226. doi:10.1136/annrheumdis-2015-208577
Ntoumou E., Tzetis M., Braoudaki M., Lambrou G., Poulou M., Malizos K., et al. (2017). Serum microrna array analysis identifies mir-140-3p, mir-33b-3p and mir-671-3p as potential osteoarthritis biomarkers involved in metabolic processes. Clin. Epigenetics 9, 127. doi:10.1186/s13148-017-0428-1
Oh H., Chun C. H., Chun J. S. (2012). Dkk-1 expression in chondrocytes inhibits experimental osteoarthritic cartilage destruction in mice. Arthritis Rheum. 64 (8), 2568–2578. doi:10.1002/art.34481
Okura T., Ohkawara B., Takegami Y., Ito M., Masuda A., Seki T., et al. (2019). Mianserin suppresses R-spondin 2-induced activation of Wnt/β-catenin signaling in chondrocytes and prevents cartilage degradation in a rat model of osteoarthritis. Sci. Rep. 9 (1), 2808. doi:10.1038/s41598-019-39393-x
Rao T. P., Kuhl M. (2010). An updated overview on Wnt signaling pathways: A prelude for more. Circ. Res. 106 (12), 1798–1806. doi:10.1161/CIRCRESAHA.110.219840
Roach H. I., Yamada N., Cheung K. S., Tilley S., Clarke N. M., Oreffo R. O., et al. (2005). Association between the abnormal expression of matrix-degrading enzymes by human osteoarthritic chondrocytes and demethylation of specific cpg sites in the promoter regions. Arthritis Rheum. 52 (10), 3110–3124. doi:10.1002/art.21300
Robling A. G., Niziolek P. J., Baldridge L. A., Condon K. W., Allen M. R., Alam I., et al. (2008). Mechanical stimulation of bone in vivo reduces osteocyte expression of sost/sclerostin. J. Biol. Chem. 283 (9), 5866–5875. doi:10.1074/jbc.M705092200
Roughley P. J. (2001). Articular cartilage and changes in arthritis: Noncollagenous proteins and proteoglycans in the extracellular matrix of cartilage. Arthritis Res. 3 (6), 342–347. doi:10.1186/ar326
Ryu J. H., Chun J. S. (2006). Opposing roles of wnt-5a and wnt-11 in interleukin-1beta regulation of type ii collagen expression in articular chondrocytes. J. Biol. Chem. 281 (31), 22039–22047. doi:10.1074/jbc.M601804200
Saliminejad K., Khorram Khorshid H. R., Soleymani Fard S., Ghaffari S. H. (2019). An overview of micrornas: Biology, functions, therapeutics, and analysis methods. J. Cell. Physiol. 234 (5), 5451–5465. doi:10.1002/jcp.27486
Sassi N., Laadhar L., Allouche M., Achek A., Kallel-Sellami M., Makni S., et al. (2014). Wnt signaling and chondrocytes: From cell fate determination to osteoarthritis physiopathology. J. Recept. Signal Transduct. Res. 34 (2), 73–80. doi:10.3109/10799893.2013.863919
Sassi N., Laadhar L., Allouche M., Zandieh-Doulabi B., Hamdoun M., Klein-Nulend J., et al. (2014). Wnt signaling is involved in human articular chondrocyte de-differentiation in vitro. Biotech. Histochem. 89 (1), 29–40. doi:10.3109/10520295.2013.811285
Shi S., Man Z., Li W., Sun S., Zhang W. (2016). Silencing of Wnt5a prevents interleukin-1β-induced collagen type II degradation in rat chondrocytes. Exp. Ther. Med. 12 (5), 3161–3166. doi:10.3892/etm.2016.3788
Shu Y., Long J., Guo W., Ye W. (2019). MicroRNA 195 5p inhibitor prevents the development of osteoarthritis by targeting REGγ. Mol. Med. Rep. 19 (6), 4561–4568. doi:10.3892/mmr.2019.10124
Snelling S. J., Davidson R. K., Swingler T. E., Le L. T., Barter M. J., Culley K. L., et al. (2016). Dickkopf-3 is upregulated in osteoarthritis and has a chondroprotective role. Osteoarthr. Cartil. 24 (5), 883–891. doi:10.1016/j.joca.2015.11.021
Staal F. J., Clevers H. (2000). Tcf/lef transcription factors during T-cell development: Unique and overlapping functions. Hematol. J. 1 (1), 3–6. doi:10.1038/sj.thj.6200001
Sugimura R., Li L. (2010). Noncanonical Wnt signaling in vertebrate development, stem cells, and diseases. Birth Defects Res. C Embryo Today 90 (4), 243–256. doi:10.1002/bdrc.20195
Tao S. C., Yuan T., Zhang Y. L., Yin W. J., Guo S. C., Zhang C. Q. (2017). Exosomes derived from mir-140-5p-overexpressing human synovial mesenchymal stem cells enhance cartilage tissue regeneration and prevent osteoarthritis of the knee in a rat model. Theranostics 7 (1), 180–195. doi:10.7150/thno.17133
Teo J. L., Kahn M. (2010). The Wnt signaling pathway in cellular proliferation and differentiation: A tale of two coactivators. Adv. Drug Deliv. Rev. 62 (12), 1149–1155. doi:10.1016/j.addr.2010.09.012
Thysen S., Luyten F. P., Lories R. J. (2015). Loss of frzb and Sfrp1 differentially affects joint homeostasis in instability-induced osteoarthritis. Osteoarthr. Cartil. 23 (2), 275–279. doi:10.1016/j.joca.2014.10.010
Tian J., Zhang F. J., Lei G. H. (2015). Role of integrins and their ligands in osteoarthritic cartilage. Rheumatol. Int. 35 (5), 787–798. doi:10.1007/s00296-014-3137-5
Tong W., Zeng Y., Chow D. H. K., Yeung W., Xu J., Deng Y., et al. (2019). Wnt16 attenuates osteoarthritis progression through a pcp/jnk-mtorc1-pthrp cascade. Ann. Rheum. Dis. 78 (4), 551–561. doi:10.1136/annrheumdis-2018-214200
Usami Y., Gunawardena A. T., Iwamoto M., Enomoto-Iwamoto M. (2016). Wnt signaling in cartilage development and diseases: Lessons from animal studies. Lab. Invest. 96 (2), 186–196. doi:10.1038/labinvest.2015.142
van Amerongen R. (2012). Alternative Wnt pathways and receptors. Cold Spring Harb. Perspect. Biol. 4 (10), a007914. doi:10.1101/cshperspect.a007914
van den Bosch M. H., Gleissl T. A., Blom A. B., van den Berg W. B., van Lent P. L., van der Kraan P. M. (2016). Wnts talking with the tgf-beta superfamily: Wispers about modulation of osteoarthritis. Rheumatol. Oxf. 55 (9), 1536–1547. doi:10.1093/rheumatology/kev402
van der Kraan P. M., van den Berg W. B. (2000). Anabolic and destructive mediators in osteoarthritis. Curr. Opin. Clin. Nutr. Metab. Care 3 (3), 205–211. doi:10.1097/00075197-200005000-00007
van der Kraan P. M., van den Berg W. B. (2012). Chondrocyte hypertrophy and osteoarthritis: Role in initiation and progression of cartilage degeneration? Osteoarthr. Cartil. 20 (3), 223–232. doi:10.1016/j.joca.2011.12.003
Wallingford J. B., Mitchell B. (2011). Strange as it may seem: The many links between Wnt signaling, planar cell polarity, and cilia. Genes Dev. 25 (3), 201–213. doi:10.1101/gad.2008011
Walsh N. C., Reinwald S., Manning C. A., Condon K. W., Iwata K., Burr D. B., et al. (2009). Osteoblast function is compromised at sites of focal bone erosion in inflammatory arthritis. J. Bone Min. Res. 24 (9), 1572–1585. doi:10.1359/jbmr.090320
Wang M., Sampson E. R., Jin H., Li J., Ke Q. H., Im H. J., et al. (2013). Mmp13 is a critical target gene during the progression of osteoarthritis. Arthritis Res. Ther. 15 (1), R5. doi:10.1186/ar4133
Wang X., Cornelis F. M. F., Lories R. J., Monteagudo S. (2019). Exostosin-1 enhances canonical Wnt signaling activity during chondrogenic differentiation. Osteoarthr. Cartil. 27 (11), 1702–1710. doi:10.1016/j.joca.2019.07.007
Wiegertjes R., van Caam A., van Beuningen H., Koenders M., van Lent P., van der Kraan P., et al. (2019). Tgf-Beta dampens il-6 signaling in articular chondrocytes by decreasing il-6 receptor expression. Osteoarthr. Cartil. 27 (8), 1197–1207. doi:10.1016/j.joca.2019.04.014
Willert K., Brown J. D., Danenberg E., Duncan A. W., Weissman I. L., Reya T., et al. (2003). Wnt proteins are lipid-modified and can act as stem cell growth factors. Nature 423 (6938), 448–452. doi:10.1038/nature01611
Wu J., Ma L., Wu L., Jin Q. (2017). Wnt-Beta-catenin signaling pathway inhibition by sclerostin may protect against degradation in healthy but not osteoarthritic cartilage. Mol. Med. Rep. 15 (5), 2423–2432. doi:10.3892/mmr.2017.6278
Wu X., Wang Y., Xiao Y., Crawford R., Mao X., Prasadam I. (2020). Extracellular vesicles: Potential role in osteoarthritis regenerative medicine. J. Orthop. Transl. 21, 73–80. doi:10.1016/j.jot.2019.10.012
Xie W. Q., Zhao Y. J., Li F., Shu B., Lin S. R., Sun L., et al. (2019). Velvet antler polypeptide partially rescue facet joint osteoarthritis-like phenotype in adult beta-catenin conditional activation mice. BMC Complement. Altern. Med. 19 (1), 191. doi:10.1186/s12906-019-2607-4
Xu W., Gao P., Zhang Y., Piao L., Dong D. (2019). microRNA-138 induces cell survival and reduces WNT/β-catenin signaling of osteoarthritis chondrocytes through NEK2. IUBMB Life 71 (9), 1355–1366. doi:10.1002/iub.2050
Yang T., Zhang J., Cao Y., Zhang M., Jing L., Jiao K., et al. (2015). Wnt5a/Ror2 mediates temporomandibular joint subchondral bone remodeling. J. Dent. Res. 94 (6), 803–812. doi:10.1177/0022034515576051
Yang Y., Topol L., Lee H., Wu J. (2003). Wnt5a and Wnt5b exhibit distinct activities in coordinating chondrocyte proliferation and differentiation. Development 130 (5), 1003–1015. doi:10.1242/dev.00324
Yang-Snyder J., Miller J. R., Brown J. D., Lai C. J., Moon R. T. (1996). A frizzled homolog functions in a vertebrate Wnt signaling pathway. Curr. Biol. 6 (10), 1302–1306. doi:10.1016/s0960-9822(02)70716-1
Yu J., Virshup D. M. (2014). Updating the Wnt pathways. Biosci. Rep. 34 (5), e00142–607. doi:10.1042/BSR20140119
Zeng L., Fagotto F., Zhang T., Hsu W., Vasicek T. J., Perry W. L., et al. (1997). The mouse fused locus encodes axin, an inhibitor of the Wnt signaling pathway that regulates embryonic Axis formation. Cell 90 (1), 181–192. doi:10.1016/s0092-8674(00)80324-4
Zeng L., Wang W., Rong X. F., Zhong Y., Jia P., Zhou G. Q., et al. (2014). Chondroprotective effects and multi-target mechanisms of icariin in il-1 beta-induced human sw 1353 chondrosarcoma cells and a rat osteoarthritis model. Int. Immunopharmacol. 18 (1), 175–181. doi:10.1016/j.intimp.2013.11.021
Zhang J., Tu Q., Bonewald L. F., He X., Stein G., Lian J., et al. (2011). Effects of mir-335-5p in modulating osteogenic differentiation by specifically downregulating Wnt antagonist Dkk1. J. Bone Min. Res. 26 (8), 1953–1963. doi:10.1002/jbmr.377
Zhang Y. J., Huang X. H., Yuan Y. H. (2017). Microrna-410 promotes chondrogenic differentiation of human bone marrow mesenchymal stem cells through down-regulating Wnt3a. Am. J. Transl. Res. 9 (1), 136–145.
Zhao C., Chen J. Y., Peng W. M., Yuan B., Bi Q., Xu Y. J. (2020). Exosomes from adiposederived stem cells promote chondrogenesis and suppress inflammation by upregulating Mir145 and Mir221. Mol. Med. Rep. 21 (4), 1881–1889. doi:10.3892/mmr.2020.10982
Zhao Y. P., Liu B., Tian Q. Y., Wei J. L., Richbourgh B., Liu C. J. (2015). Progranulin protects against osteoarthritis through interacting with tnf-alpha and beta-catenin signalling. Ann. Rheum. Dis. 74 (12), 2244–2253. doi:10.1136/annrheumdis-2014-205779
Zhong G., Liang R., Yao J., Li J., Jiang T., Liu J., et al. (2018). Artemisinin ameliorates osteoarthritis by inhibiting the wnt/β-catenin signaling pathway. Cell. Physiol. biochem. 51 (6), 2575–2590. doi:10.1159/000495926
Zhou R., Wang L., Zhao G., Chen D., Song X., Momtazi-Borojeni A. A., et al. (2020). Circulating exosomal micrornas as emerging non-invasive clinical biomarkers in heart failure: Mega bio-roles of a nano bio-particle. IUBMB Life 72 (12), 2546–2562. doi:10.1002/iub.2396
Zhu M., Chen M., Zuscik M., Wu Q., Wang Y. J., Rosier R. N., et al. (2008). Inhibition of beta-catenin signaling in articular chondrocytes results in articular cartilage destruction. Arthritis Rheum. 58 (7), 2053–2064. doi:10.1002/art.23614
Zhu M., Tang D., Wu Q., Hao S., Chen M., Xie C., et al. (2009). Activation of beta-catenin signaling in articular chondrocytes leads to osteoarthritis-like phenotype in adult beta-catenin conditional activation mice. J. Bone Min. Res. 24 (1), 12–21. doi:10.1359/jbmr.080901
Glossary
Acan Aggrecan
ADAMTS a disintegrin and metalloproteinase with thrombospondin motifs
ACLT anterior cruciate ligament transection
ART artemisinin
APC adenomatous polyposis coli
CaMKII calcium/calmodulin-dependent kinase II, calcium/calmodulin-dependent protein kinase type II
CK-I casein kinase I
Col2a1 type II collagen alpha 1 chain
Dkk dickkopf
Dvl disheveled
DMM destabilization of the medial meniscus
ECM extracellular matrix
EVs extracellular vesicles
FGF-2 fibroblast growth factor-2
GSK-3β glycogen synthase kinase-3 β
IL interleukin
JNK c-Jun N-terminal kinase
LRP lipoprotein receptor-related protein
mRNA messenger RNA
miRNA microRNA
MMP-13 matrix metalloproteinase 13
MMx medial meniscus resection
OA osteoarthritis
PKC protein kinase C
PLC phospholipase C
Runx-2 Runt-related transcription factor-2
Ryk receptor tyrosin kinase
sFRPs secreted frizzled-related proteins
Sox9 SRY-box transcription factor 9
TNF-α tumor necrosis factor alpha
Wnt Wingless/integrated
WIF-1 WNT-inhibitory factor-1
Keywords: chondrocyte, wnt signaling, osteoarthritis, β-catenin, therapy
Citation: Cheng J, Li M and Bai R (2022) The Wnt signaling cascade in the pathogenesis of osteoarthritis and related promising treatment strategies . Front. Physiol. 13:954454. doi: 10.3389/fphys.2022.954454
Received: 31 May 2022; Accepted: 08 July 2022;
Published: 02 September 2022.
Edited by:
Anjali P. Kusumbe, University of Oxford, United KingdomReviewed by:
Saeed Khalili, Shahid Rajaee Teacher Training University, IranWenhui Hu, Third Military Medical University (Army Medical University), China
Copyright © 2022 Cheng, Li and Bai. This is an open-access article distributed under the terms of the Creative Commons Attribution License (CC BY). The use, distribution or reproduction in other forums is permitted, provided the original author(s) and the copyright owner(s) are credited and that the original publication in this journal is cited, in accordance with accepted academic practice. No use, distribution or reproduction is permitted which does not comply with these terms.
*Correspondence: Ruijun Bai, YmFpcnVpanVuLWNvbGFAY3N1LmVkdS5jbg==