- 1Center for Molecular Genetics, Institute of Translational Medicine, The Affiliated Hospital of Qingdao University, Qingdao University, Qingdao, China
- 2School of Basic Medicine, Qingdao University, Qingdao, China
- 3Juxian People’s Hospital, Rizhao, China
- 4The Affiliated Cardiovascular Hospital of Qingdao University, Qingdao, China
Patients with diabetes have severe vascular complications, such as diabetic nephropathy, diabetic retinopathy, cardiovascular disease, and neuropathy. Devastating vascular complications lead to increased mortality, blindness, kidney failure, and decreased overall quality of life in people with type 2 diabetes (T2D). Glycolipid metabolism disorder plays a vital role in the vascular complications of T2D. However, the specific mechanism of action remains to be elucidated. In T2D patients, vascular damage begins to develop before insulin resistance and clinical diagnosis. Endothelial dysregulation is a significant cause of vascular complications and the early event of vascular injury. Hyperglycemia and hyperlipidemia can trigger inflammation and oxidative stress, which impair endothelial function. Furthermore, during the pathogenesis of T2D, epigenetic modifications are aberrant and activate various biological processes, resulting in endothelial dysregulation. In the present review, we provide an overview and discussion of the roles of hyperglycemia- and hyperlipidemia-induced endothelial dysfunction, inflammatory response, oxidative stress, and epigenetic modification in the pathogenesis of T2D. Understanding the connections of glucotoxicity and lipotoxicity with vascular injury may reveal a novel potential therapeutic target for diabetic vascular complications.
1 Introduction
Diabetes mellitus (DM) is a heterogeneous disease with multiple etiologies. Diabetes is more common in the middle-aged and elderly populations. However, the age of diabetes onset tends to be younger because of excess nutrition and lack of exercise. Currently, approximately 463 million people have diabetes worldwide (Ogurtsova et al., 2017). Diabetes is generally divided into type 1 diabetes (T1D) and type 2 diabetes (T2D) according to the function of human pancreatic islets, with T2D accounting for most cases (Beckman and Creager, 2016; Schwab et al., 2021; Chen et al., 2022). Insulin resistance is a significant feature of T2D. Unhealthy diet, obesity, and physical inactivity increase the risk of T2D (Teodoro et al., 2018; Georgakis et al., 2021). The T2D phenotype includes the inability to metabolize glucose in blood vessels, resulting in high glucose (HG) contents in blood vessels. Excessive lipid accumulation is associated with glucose intolerance (Nosadini and Tonolo, 2011). Dyslipidemia is another indicator of T2D, as it increases the low-density lipoprotein (LDL) concentration (Wils et al., 2017). Uncontrolled glycolipid metabolism in T2D can damage and destroy blood vessels in various organs, especially the kidneys, eyes, heart, and nerves (Iacobini et al., 2021). The vascular complications of T2D, such as diabetic retinopathy, diabetic nephropathy, diabetic neuropathy, myocardial infarction and stroke, are mostly related to the aforementioned tissues (Figure 1A).
The pathophysiology of the association between T2D and vascular complications is multifactorial. Several studies have revealed that it was closely related to glucotoxicity and lipotoxicity (Teodoro et al., 2018; Opazo-Ríos et al., 2020). Hyperglycemia and hyperlipidemia could trigger oxidative stress through mitochondrial dysfunction, and then enhance the production of reactive oxygen species (ROS), whereas hyperlipidemia releases pro-inflammatory cytokines through adipose tissue. Oxidative stress and inflammatory response are considered major factors in the progression of T2D and its complications. These lesions can manifest as endothelial dysfunction (ED), an early stage of vascular disease and T2D complications. Furthermore, epigenetic reprogramming can affect the genes associated with inflammation and the generation of ROS, resulting in ED. In the present work, we reviewed the blood vessel damage caused by abnormal glucose and lipid metabolism, which may provide insights for developing new strategies for the treatment of T2D and its complications.
2 Vascular wall structure
In general, blood vessels walls are divided into three layers from the lumen to the outside: the tunica intima, tunica media, and tunica adventitia (Jedlicka et al., 2020). The tunica intima is the innermost layer of the tube wall, which is mainly composed of interconnected endothelial cells. However, there are also gaps in some blood vessels, especially in the veins. The tunica media varied in composition and thickness according to the type of blood vessel. The aorta is mainly based on the elastic membrane, and the middle artery is mainly made up of smooth muscle. The tunica adventitia is mainly composed of fibroblasts, elastic fibers, and collagen fibers.
For a long time, the inner membrane cells have been generally believed to be the inner part directly in contact with blood. However, a later study found a colloidal film, called glycocalyx, that has a layer of protein-polysaccharide complex on the inner membrane cells and a thickness in the order of microns (Jedlicka et al., 2020). The vascular endothelial glycocalyx covers the surface of all vascular endothelial cells and regulates vascular endothelial permeability. The endothelial glycocalyx interacts with blood and endothelial cells, mediate blood flow shear force, and induce the release of nitric oxide (NO) (Becker et al., 2010; Becker et al., 2015; Jedlicka et al., 2020) (Figure 1B). Some blood vessels are also differently composed and distributed, and the veins have additional structures, such as venous valves.
3 Vascular damage due to hyperglycemia and dyslipidemia
Hyperglycemia associated with dyslipidemia initiates diabetic vascular complications through metabolic and vascular remodeling and aberrant gene expression. In recent years, many hypotheses have been proposed to elucidate the mechanisms of hyperglycemia and dyslipidemia evoked vascular damage, including ED, the formation of advanced glycation end products (AGEs), oxidative stress, inflammation and epigenetic modification (Figure 2).
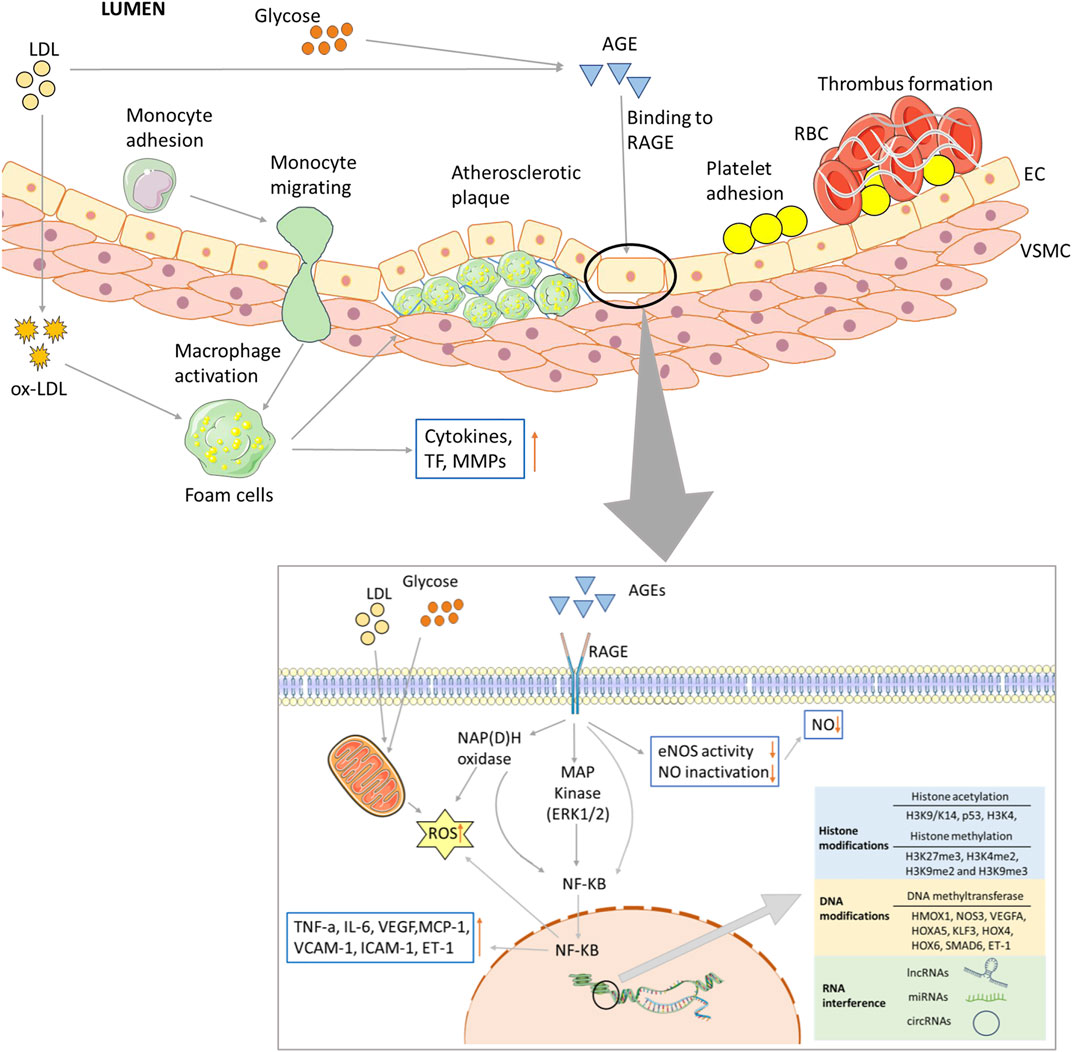
FIGURE 2. Biochemical and major pathways underlying endothelial dysregulation in vascular complications of diabetes. Long-term hyperglycemia and hyperlipidemia can cause endothelial cell dysfunction and increase the adhesion of monocytes and platelets. The former can transform into macrophages, while the latter can recruit blood cells, accumulate in blood vessels, and form thrombi. On the one hand, macrophages invade endothelial cells and engulf ox-LDL, turning into foam cells and forming arterial plaques. Macrophages release inflammatory and transcription factors that aggravate the inflammatory response. Excessive glucose and lipid levels will covalently combine to form AGEs, which can bind to their receptors; activate the MAPK and NF-KB pathways, among others; and reduce the production and utilization of NO. Abnormal glucose and lipid metabolism can also affect mitochondrial function, produce excessive ROS, and lead to insufficient energy supply. Epigenetic modifications are also closely related to vascular injury in the vascular complications of T2D, including histone and DNA modifications, and ncRNA regulation.
3.1 ED and structure remodeling
Endothelial cells not only constitute the vascular network but also are involved in vasodilation and vascular reactivity. The early sign of diabetic vascular injury is endothelial injury. Endothelial cells regulate the tension in blood vessels through mechanoreceptors, which sense shear forces caused by blood flow through their surfaces. Subsequently, endothelial cells transmit these messages to surrounding cells and secrete many biologically active mediators to dilate or contract blood vessels to regulate blood flow (Iliff and Xu, 2018). For example, the active mediators of vasodilation are NO, prostaglandin I2 (PGI2), histamine, and serotonin. The vasoconstrictive mediators are endothelin-1 (ET-1), thromboxane A2, angiotensin II (Ang II), and prostacyclin H2 (Sandoo et al., 2010; Krüger-Genge et al., 2019). HG and LDL levels can change the osmotic pressure of endothelial cells and the permeability of the intima, which may affect the synthesis and release of vascular regulatory factors by endothelial cells, resulting in reduced vascular reactivity.
The normal metabolism of intimal cells and the integrity of the intima are the basis for the normality of vessels. ED is the inability of endothelial cells to maintain vascular homeostasis. The development of ED is related to an increased risk of vascular complications and mortality (Erkens et al., 2017; Heusch, 2020). Oxidative stress has been considered a sign of ED. AGEs promotes cellular glucotoxicity, and long-term ED supports inflammation in the vascular system.
As mentioned earlier, NO is a vasodilator that can induce the relaxation of vascular smooth muscle cells (VSMCs) and vascular vasodilation (Moncada et al., 1991; Rajendran et al., 2019). ED can inhibit the expression of endothelial nitric oxide synthases (eNOS) and decrease NO synthesis. Glycocalyx is a protector of endothelial cells. However, HG levels could directly damage the glycocalyx by promoting the shedding (Jedlicka et al., 2020). Endothelial cells are sensitive to HG levels, and the change in the extracellular environment can induce cell death and influence cell growth (Triggle et al., 2012). The excess glucose can activate NF-kB expression in endothelial cells, which leads to an increase in the expression levels of pro-inflammatory cytokines (Aminzadeh et al., 2013). HG can stimulate the transformation of endothelial cells into fibrous phenotypes, causing vascular injury. Several studies have found fibrotic changes in the heart, liver, and kidneys of diabetic rodent models (Huang et al., 2013; Lucchesi et al., 2015; Alex et al., 2018). ED conditions can induce increased VSMC proliferation and phenotypic transition, increasing the thickness of the vascular middle layer and promoting the stenosis of the vascular lumen.
Oxidized low-density lipoprotein (ox-LDL) can be formed when LDL levels increases in blood vessels, and the lipid hydrogen peroxide produced in the process of ox-LDL production can directly damage endothelial cells, increase cell permeability, and promote cell death. Endothelial cells damage will further cause structure remodeling to blood vessels. Monocytes can migrate into the inner membrane when the tunica intima is incomplete. Macrophages converted from monocytes engulf ox-LDL and turn into foam cells. This will increase the thickness of the median-intimal membrane of blood vessels. In addition, ox-LDL can inhibit the synthesis of the endothelial cell-derived relaxation factor or NO, impairing the normal diastolic function of arterial walls.
3.2 Formation of AGEs
AGEs are compounds of non-enzymatic glycation. Excess glucose, lipids and proteins combine to form covalent add-ons (Barlovic et al., 2011; Yang et al., 2019a; Papachristoforou et al., 2020). The free amino group of proteins and the carbonyl group reduce glucose levels or another carbonyl to develop the Schiff bases. The Schiff bases could undergo Amadori rearrangement reactions and form relatively stable aldehyde and amine products. The Amadori products can result from dehydration, rearrangement and fragmentation reaction, which lead to the formation of AGEs. Even though the last reaction is usually slow, ROS and other catalysts can increase its rate.
The increased levels of AGEs were related to the less endothelial-dependent vasodilation. AGEs can depress the expression of eNOS by receptor-mediated phosphorylation of serine residues in eNOS and increase the degradation of eNOS mRNA (Ren et al., 2017). Tan et al. found that increased concentrations of AGEs were associated with ED by impairing endothelial-dependent vasodilation (Tan et al., 2002). AGEs are also responsible for increases in ET-1 levels and decreases in PGI2 levels, which lead to vasoconstriction (Santilli et al., 2015). AGEs were related to lipid accumulation in vessels. For example, the circulating AGEs can alter the structures of LDL and damage the mechanisms of LDL receptor-mediated cholesterol uptake (Ruiz et al., 2020). Furthermore, the interaction of AGE and LDL could induce pro-inflammatory cytokine production and affect the phagocytosis of macrophages and phenotypic conversion of smooth muscle cells (Iwashima et al., 2000; Barlovic et al., 2011; Byun et al., 2017).
The interaction of AGEs and their receptor (RAGE) plays a vital role in the AGEs that mediate vascular complications. The binding of AGEs to RAGE activates the transcription factor NF-kB which regulate multiple genes, such as ERK (extracellular signal-regulated kinase) 1/2 and the p38 MAP kinase (MAPK) pathway (Bierhaus et al., 2001; Chen et al., 2010a). These signaling pathways lead to an inflammatory response, including the aggregation of cell adhesion molecules. NF-kB regulates various cellular signaling cascades associated with ROS formation, including NAPDH oxidase and protein kinase C (PKC) (Coughlan et al., 2009; Chen et al., 2010a).
3.3 Oxidative stress
Oxidative stress occurs when reactive species are overproduced or when the antioxidant system is weakened. Reaction species are usually derived from oxygen, nitrogen, or sulfur elements, which generate ROS, reactive nitrogen (RNS), and reactive sulfur. ROS and RNS are the major sources of oxidative stress. The free radicals include the superoxide anion radical (O2−), hydroxyl free radical (OH·), hydrogen peroxide (H2O2) and peroxynitrite (ONOO−).
Many experimental and clinical studies have demonstrated that oxidative stress promotes the development of vascular complication. The mitochondrial overproduction of ROS was reported to be the primary origin of vascular damage in hyperglycemia induced diabetic complications (Nishikawa et al., 2000; Iacobini et al., 2021). In hyperglycemia, the flux and voltage gradient of the electron donors into the electron transport chain through the inner mitochondria membrane were increased owing to the increase in pyruvate generated. The electron transfer inside complex III in the electron transport chain is plugged when the voltage gradient reaches a critical point. The electrons return to coenzyme Q and transfer to O2, resulting in superoxide (O2−) (Brownlee, 2005). In addition, NADPH oxidases, xanthine oxidase, uncoupled nitric oxide synthase (NOS), cycloxygenase-2 (COX-2), and endoplasmic reticulum (ER) stress can also contribute to the production of superoxide. Excessive ROS in turn causes mitochondria dysfunction and affects the energy supply of cells. In addition, dysfunctional mitochondria act as a key regulator of inflammatory response, apoptosis, and ferroptosis (Rizwan et al., 2020). Many apoptosis-related factors and key proteins are located in the mitochondria, and mitochondrial dysfunction could activate many signaling pathways (Li et al., 2018) such as the AMP-dependent protein kinase (AMPK)/the mammalian target of rapamycin (mTOR) (Assaf et al., 2022) and phosphatidylinositol 3-kinase (PI3K)/MAPK pathways (Barber et al., 2021).
Oxidative stress can directly induce vascular injury. For instance, oxidative damage can rapidly decrease the glycocalyx level in vascular endothelial cells, reducing the integrity of the vascular wall. ROS are a main factor in the progression of several disease. ROS can inhibit the activity of eNOS, increase the pressure in the mitochondrial and endoplasmic reticulum in endothelial cells, which leads to ED. Hyperglycemia can also increase the ROS production through the polyol and hexosamine pathways and PKC activation. In addition, hyperglycemia can directly trigger the excessive production of ROS by activating the NF-kB pathway which affect the enzymatic cascades (Pitocco et al., 2013). The nuclear factor erythroid 2-related factor (Nrf2) is an important transcription factor that regulates intracellular oxidative stress and is a key regulator for maintaining intracellular redox homeostasis (Baird and Yamamoto, 2020). Zhang et al. (2016) demonstrated that hyperglycemia could inhibit the regulation of Nrf2 activity, which leads to an imbalance in oxidative stress and the aggravation of cellular damage.
The excessive accumulation of ROS promotes the production of ox-LDL and AGEs, which can lead to vascular damage. However, ROS could oxidize lipids, proteins and nucleic acid molecules, including DNA and RNA. Studies have demonstrated that the oxidized nucleic acid content was higher in the urine or blood plasma of patients with T2D (Franzke et al., 2018; Schöttker et al., 2020). ROS can cause the DNA black, which leads to the activation of poly ADP-ribose polymerase (PARP), inhibiting the expression of the glycolytic enzyme glyceraldehyde-3-phosphate dehydrogenase (GAPDH), and promoting the production of early glycolytic intermediates (Shah and Brownlee, 2016). This production promotes ED through the PKC pathway and the formation of the AGE pathway. The oxidization of DNA can affect the its self-reparative ability and hyperglycemia disturbs the self-reparative ability of DNA by ROS, which leads to the cell death and senescence. A previous study revealed that DNA repair contributes to fibrotic remodeling (Kumar et al., 2020). Oxidative DNA can activate the homeo-domain interacting protein kinase 2 (HIPK2) expression, which leads to activation of fibrosis and then increase vascular stiffness (Tuleta and Frangogiannis, 2021).
RAGE is also a critical factor in oxidative stress-induced vascular damage. Many intracellular signaling pathway linked to oxidative stress can be activate when AGEs bind to RAGE. Furthermore, ROS can promote inflammatory response by increasing pro-inflammatory cytokine levels, the expression level of cell adhesion molecules, and growth factors. ROS can also activate multiple signaling molecules such as MAPK, PI3K, Akt (or PKB), mTOR, and JUK. These genes were reported to be associated with cell apoptosis, proliferation, migration, inflammatory response and oxidative stress (Chiarugi and Cirri, 2003; Torres and Forman, 2003; Montezano et al., 2014). Thus, the oxidative stress and inflammatory response mechanism were exacerbated, increasing endothelial cell apoptosis, inhibiting cell proliferation and vascular repair, and aggravating vascular injury.
3.4 Inflammation
Long-term chronic inflammation plays a major role in the pathogenesis and development of diabetic vascular complication, such as diabetic nephropathy and retinopathy. The inflammatory response in blood vessels can be stimulated by the aggregation of monocytes, platelets and the invasion of macrophages. The immune system is associated with the metabolic changes in T2D. Inflammation signaling is coupled with ROS and AGEs. Chronic inflammation can damage vascular components and the vascular endothelial structure and reduce vascular reactivity. The inflammatory reaction in the body directly destroys the glycocalyx, which is also a main hazard of long-term inflammation. Platelets, blood cells, and fibrin are deposited and aggregated to form thrombosis, damaging the endothelial cells in the intima, which recruit white blood cells and further activate the inflammatory response process. The inflammatory cells infiltrate the blood vessel wall, which can impair reactive and increase the stiffness of the blood vessel wall.
In T2D, the activation of inflammation is associated with dysregulated inflammasome. Plasminogen activator inhibitor type-1 (PAI-1) expression was associated with fibrinolysis and high PAI-1 expression is linked with formation of thrombosis. Pandolfi et al. found that PAI-1 levels were increased in the arterial wall of diabetic patients (Pandolfi et al., 2001). Evaluating PAI-1 expression level can help reduce fibrinolysis. HG levels can stimulate the accumulation of NLR family pyrin domain containing 3, resulting in the generation of interleukin-1β (IL-1β) (Sharma et al., 2018). The release of active IL-1β can induce the expression of other inflammatory cytokines and promote chemotactic responses and recruitment of macrophages.
Ox-LDL can enhance the expression of intercellular adhesion molecule-1, which increases the numbers of monocytes, neutrophils and lymphocytes binding to the endothelium, and the binding shows high affinity. Monocytes are transformed into macrophages and even induce ED and structural remodeling. This process can trigger ROS production, which promotes the expressions of IL-1 and tumor necrosis factor α (TNF-α). The increased IL-1 and TNF-α levels upregulated the expression of adhesion molecules and recruited more monocytes into the progression of vascular stiffness. These reactions can induce apoptosis and impair NO release, which leads to ED.
3.5 Epigenetic modification
Epigenetic modifications are stable and heritable changes to epigenetic inheritance and phenotypes, which are independent of changes in the gene sequence. Epigenetic factors could regulate gene expression and control cell phenotypes, including DNA methylation, histone modification and regulation of non-coding RNAs (ncRNAs). In T2D, abnormal regulation of enzymes in the gene promoter region could alter gene expressions, and differentially expressed genes such as SET domain-containing lysine methyltransferase 7 (SET7) and suppressor of variegation 3–9 homolog 1 (SUV39h1) can affect inflammation-related pathways that lead to vascular injury. Various genes can be changed by epigenetic modifications, which are involved in the pathology of diabetic vascular complications (Pirola et al., 2010; Kato and Natarajan, 2014). Those genes are mainly involved in the signaling of inflammation, modification, and oxidative stress.
3.5.1 DNA methylation
DNA methylation refers to the covalent binding of a methyl group to the CpG dinucleotide cytosine 5′ carbon site in the genome without altering the DNA sequence by DNA methyltransferases (DNMTs) (Greenberg and Bourc’his, 2019). Previous studies have demonstrated that DNA methylation is involve in the progress of vascular complications (Rajasekar et al., 2015; Natarajan, 2021). The DNMT family includes DNMT1, DNMT2, and DNMT3 (DNMT3A, DNMT3B, and DNMT3L).
Hyperglycemia can stimulate DNA methylation. For instance, Priola et al. found the hypomethylation of heme oxygenase 1 (HMOX1) and hypermethylation of interleukin 8 precursor (IL8) in human aortic endothelial cells treated with HG (Pirola et al., 2011). Hyperglycemia can cause abnormal DNA methylation in multiple genes such as endothelial NOS, vascular endothelial growth factor A (VEGFA), homeobox A5, Krüppel-like factor 3, homeobox 4, homeobox 6, small mothers against decapentaplegic homolog 6 (SMAD6), and SMAD3, which are involved in angiogenesis, inflammation and migration resulting in ED (Dunn et al., 2015; Aref-Eshghi et al., 2020; Pepin et al., 2021). Another study also found that ET-1 was hypomethylated at the promoter region and during the worsened state of a vascular complication (Biswas et al., 2018).
However, hyperglycemia can also decrease DNA methylation. Mitochondrial adaptor p66shc protein functions as a redox enzyme to regulate ROS generation and the oxidative signals associated with apoptosis. Paneni et al. (2012) found that hyperglycemia decreased DNA methylation at the promoter region of p66shc. Decreased methylation and upregulated p66shc increased the AGEs precursors and promoted apoptosis. In the diabetic condition, the promoter of polymerase gamma 1 is hypermethylated in retinal endothelial cells (Tewari et al., 2012).
3.5.2 Histone modification
Histones are proteins that make up nucleosomes together with DNA, including H2A, H2B, H3, and H4. Histone modification occurs covalently in the N-terminal tail part of the histone, and H3 is the most modified histone protein (Zhang et al., 2021a). These protein modifications can inhibit or activate the transcription of genes. The sites of histone methylation were usually lysine and arginine.
Histone acetylation is mainly related to the activation of genes, which are coordinated by acetyltransferase and deacetylase. For instance, H3K9/K14 acetylation was caused by HG level, which leads to the overexpression of IL-8, HMOX1, and MMP10. The upregulated genes were associated with oxidative stress and inflammation, which could promote the pathological process of T2D vascular complications (Pirola et al., 2011). The expression of sirtuin 1 (SIRT1) was negatively regulated by acetylated p53 under the HG condition. Upregulated SIRT1 inhibited the expression of PARP and mitochondria mediated apoptosis related genes, such as NF-kB and BAX (Orimo et al., 2009; Zheng et al., 2012). Overexpression of SIRT1 also restrained the expression of p66shc and oxidative stress in the vasculature of diabetic mice (Zhou et al., 2011). Chen et al. (2010b) found that hyperglycemia can induce the expression of transcriptional coactivator p300 in human umbilical vein endothelial cells (HUVECs). Increased binding of p300 to ET-1 and fibronectin promoters, increased histone acetylation, H2AX phosphorylation, multi-transcription factor activation, expressions of vasoactive factors, and extracellular matrix protein. In the streptozotocin induced diabetic rat model, the histone acetyltransferases level was decreased and the histone deacetylases level was increased in HG levels (Zhong and Kowluru, 2010). Hyperglycemia triggered the expression of NF-kB, which is associated with increased H3K4 and reduced H3K9 methylation in aortic endothelial cells (Brasacchio et al., 2009).
H3K27me3 in the promoter of eNOS can regulate the expression and affect endothelial reactivity (Dhawan et al., 2022). Liao et al. (2018) found that histone methylation (H3K4me2, H3K9me2, and H3K9me3) at the promoter site reduced the expression levels of NOX4 and eNOS. Dysregulated NOX4 and eNOS contribute to ROS and NO generation, resulting in ED. Zhong et al. found that hyperglycemia reduced the expression of H3K4me1 and me2 but increased the binding of lysine-specific demethylase 1 (LSD1) with superoxide dismutase 2 (SOD2). Downregulation of the expression of LSD1 ameliorates the glucose-induced reduction of SOD2 H3K4 methylation and prevents the decrease in SOD2 gene expression levels (Zhong and Kowluru, 2011; Zhong and Kowluru, 2013). Hyperglycemia can promote the accumulation of SET7 and activate the activity of SET7. SET7 acts on the p65 promoter region of NF-kB, which leads to H3K4 methylation and upregulation of NF-kB expression and affects its dependent inflammatory factors such as the vascular cell adhesion molecule and monocyte chemotactic protein 1 (MCP-1). Previous studies have shown that SET7 knockdown can significantly reduce NF-kB-mediated inflammatory genes in diabetic models (Miao et al., 2006; Li et al., 2008). The increased expression levels of inflammation-related genes are also associated with decreases in the expression levels of repressive regulators in T2D. For example, at the promoter regions of IL-6, MCP-1, and TNF-α, decrease H3K9me3 and SUV39h1 recruitment were found in diabetic mice (Villeneuve et al., 2008). Furthermore, inhibition of the expression of SUV39h1 methyltransferase can reduce H3K9me3 expression in IL-6, which leads to cytokine expression (Villeneuve et al., 2010).
3.5.3 ncRNAs
ncRNAs are transcribed from the genome but does not code proteins. They are involved in translation and regulate the expression of related genes. With the advancements of the sequencing technology and research, the understanding of non-coding RNA has also changed from “useless” to “useful.” Recent reports have implicated ncRNAs in the vascular injury in T2D, including microRNAs (miRNAs), circular RNAs (circRNAs), and long non-coding RNAs (lncRNAs; Table 1).
3.5.3.1 miRNAs
MiRNAs are small-molecule ncRNAs, with a length of 21–24 nucleotides, which function by partial complementary sequence binding to the 3′ untranslated region of the target mRNA to cleat the mRNA or repress the translation process (Ha and Kim, 2014). Each miRNA can have multiple target genes, and multiple miRNAs can regulate the same gene.
Silambarasan et al. (2016) performed a microarray on HUVECs treated with HG and analyzed in patients with T2D and diabetic rats. They found that the expression levels of ten miRNAs were gradually increased with the increase in HG concentration. Among these miRNAs, miR-29b-3p, miR-29c-3p, miR-125b-1-3p, miR-130b-3p, miR-221-3p, miR-320a and miR-192-5p were correlated with endothelial cell apoptosis. The expression of miR-34a was upregulated in the aortic endothelium of diabetic mice. MiR-34a knockdown can attenuate oxidative stress by regulating the expression of sirtuin1 (Sirt1) (Li et al., 2016). The low expression of miR-146a was associated with the significant increase in NF-kB expression level in diabetic rats, activated the inflammation pathway, and promote apoptosis (Yousefzadeh et al., 2015; Habibi et al., 2016). MiR-221 and miR-222 have a similar function, which is to promote the intimal thickening in the arteries of diabetic subjects. The downregulation of miR-221 and miR-222 reduced the VSMC proliferation and migration by metformin, and inhibiting miR-221 and miR-222 was efficacious in the prevention of the vascular complications of T2D (Coleman et al., 2013; Lightell et al., 2018). The miR-342-3p expression was downregulated in endothelial cells isolated from diabetic models. Overexpression of miR-342-3p enhanced endothelial cell proliferation and migration by targeting fibroblast growth factor 11 signaling (Cheng et al., 2018).
MiR-29a/b knockdown could impair the endothelial function in diabetic patients and rat models. Overexpression of miR-29 can promote NO production and restore endothelium-dependent vasodilation by modulating lysophospholipase I (Lypla1) expression (Widlansky et al., 2018). MiR-9 expression was involved in anti-inflammation and apoptosis under HG conditions (Jeyabal et al., 2016). Chen et al. (2021) found that upregulating the miR-9 expression can rescue hyperglycemia-induced ED by inhibiting the Notch1 signaling pathway. Overexpression of miR-24 can inhibit the platelet-derived growth factor pathway, protect against intimal hyperplasia and inhibit intracellular inflammatory responses (Yang et al., 2018). Endothelial injury stimulates platelet aggregation and cell proliferation and differentiation, and activated inflammatory signals can induce thrombosis. Previous studies have shown that the expression of miR-19a can regulate endothelial cell homeostasis and angiogenesis (Qin et al., 2010; Jiang et al., 2015). In addition, Witkowski et al. found that miR-19a expression correlated with miR-126 expression in plasma of diabetic patients and showed anti-thrombotic properties by modulating vascular tissue factor (TF) expression (Witkowski et al., 2018). Other study demonstrated that miR-483-3p could also regulate the endothelial integrity by targeting vascular endothelial zinc finger 1 (VEZF1) (Kuschnerus et al., 2019). Zhou et al. found that downregulation of miR-210 expression induced ED in T2D and the levels of miR-210 were lower in T2D patients. Overexpressed miR-210 can rescue endothelial apoptosis by repressing the expression of protein tyrosine phosphatase 1B (PTP1B) and oxidative stress (Zhou et al., 2022). Torella et al. found that miR-29c and miR-204 can regulate the VSMC hyperplastic phenotype by targeting epithelial membrane protein 2 (Emp2) and caveolin1 (Cav1), respectively (Torella et al., 2018). The expression of miR-181c is associated with endothelial cells damage under abnormal conditions of glycolipid metabolism (Yang et al., 2017). Shen et al. investigated whether miR-181c-3p/5p overexpression could enhance endothelial cell injury by regulating the expression of the leukemia inhibitory factor (Shen et al., 2018).
3.5.3.2 circRNAs
CircRNAs are cyclic ncRNAs produced by non-canonical cleavage and covalently linked upstream and downstream shear sites (Kristensen et al., 2019). Many circRNAs have been identified in eukaryotes by high-throughput RNA sequencing (RNA-seq) and bioinformatics and were found to have tissue-specific expression patterns. circRNAs could act as miRNA sponges to affect the regulation of or interact with related proteins (Kristensen et al., 2019; Zang et al., 2020). To date, circRNAs have been reported to be associated with the pathologies and development of various diseases such as DM, cardiovascular diseases and cancer (Cortés-López et al., 2018; Altesha et al., 2019).
Vascular damage and repair can stimulate VSMC differentiation and proliferation, causing intravascular restenosis. Another reaction to vascular injury is intimal hyperplasia. Chen et al. (2017) performed a microarray and found 983 differentially expressed circRNAs under HG conditions. CircWDR77 (circ_0013509) expression was upregulated in VSMCs cultured with HG. circWDR77 knockdown inhibited cell proliferation and migration by targeting miR-124/growth factor 2 (FGF-2). CircHIPK3 expression was downregulated in HG-treated HUVECs and human aortic endothelial cells. circHIPK3 knockdown exacerbated endothelial cell apoptosis by modulating miR-124 expression (Cao et al., 2018). In the carotid artery injury rat model, overexpressed circDiaph3 (circ_005717) can act as a sponge with miR-148-5p and weaken the inhibitory effect of miR-148a-5p on insulin-like growth factor-1 receptor (IGF1R), which promotes intimal hyperplasia (Xu et al., 2019a).
A previous study found that circBPTF (circ_0045462) expression was upregulated in HUVEC under HG condition (Jin et al., 2019). Zhang and Sui, 2020 revealed that circBPTF knockdown could suppress cell apoptosis and reduce the release of pro-inflammatory cytokines by mediating the miR-384/LIN28B axis. Wang and Zhang, 2021 found that the circ_001209/miR-15b-5p/COL12A1 axis may be the potential regulatory pathway for human retinal vascular endothelial cells. Circ_CLASP2 (circ_0064772) expression was found to be downregulated in HG-induced HUVECs. Overexpression of circ_CLASP2 can decrease ED under HG conditions by inhibiting the expression of miR-140-5p and regulating FBXW7 expression (Zhang et al., 2021b). Depletion of circ_SOD2 alleviated VSMC proliferation by modulating the miR-206/NOTCH3 axis (Mei et al., 2021). Furthermore, Zeng et al. revealed that overexpression of circMAP3K5 was associated with reduced proliferation of VSMCs to sequester miR-22-3p, which inhibited the expression of TET2 (Zeng et al., 2021).
Flow pattern was related to endothelial integrity. Overexpression of circ_0007367 can protect the endothelial integrity by repressing the activity of NF-kB signaling and increasing the eNOS expression level (Li et al., 2022). The circ_0068087 expression level was increased in blood sample from patients with T2D. Circ_0068087 knockdown could ameliorate ox-LDL-induced HUVEC dysfunction by miR-186-5p and roundabout guidance receptor 1 (ROBO1) (Li et al., 2021a). Moreover, circ_0068087 knockdown could also suppress cell dysfunction under HG conditions. Inhibiting miR-197 expression can reverse the circ_0068087 function, and toll-like receptor 4 (TLR4) was the downstream target of miR-197 (Cheng et al., 2019). Li et al. (2021b) found that upregulated circ_0006768 expression rescued human brain microvascular endothelial cells injury by modulating the expression of miR-222-3p and its target, VEZF1.
Circ_0003423 functions as an endogenous miR-589-5p sponge to inhibit miR-589-5p activity that upregulates TET2, which relieves ox-LDL-induced endothelial cell injury (Yu et al., 2021). The circ_0003645 expression was found to be up-regulated in patients with atherosclerosis and in vitro. Cell apoptosis and the expression levels of the NF-kB pathway-related genes were reduced after knockdown of circ_0003645 expression (Qin et al., 2020). Changes in circ_0003204 levels have been detected in HUVECs (Liu et al., 2020; Wan et al., 2021). Silencing circ_0003204 promoted cell viability and decreased inflammation in HUVECs. Circ_0003204 can modulate HDAC9 levels by sponging miR-942-5p. Li et al. (2021c) performed a circRNA microarray to detect aberrant expression of circRNAs in an atherosclerosis mouse model. Finally, the circABCA1 (circ_36781) and circKHDRBS1 (circRNA_37699) expression were significantly upregulated. The following sponging of miR-30 days-3p and miR-140-3p were predicted using bioinformatics analysis, and the target protein TP53RK and MKK6 were identified in vivo and vitro, respectively.
3.5.3.3 lncRNAs
LncRNAs are a diverse class of RNAs with lengths of more than 200 nucleotides. Studies have proposed many functions for lncRNAs, including cis or trans transcription regulation, nuclear domain organization, and interaction with other ncRNAs or specific proteins (Quinn and Chang, 2016). Previous studies have implicated lncRNAs in the progression of numerous pathologies (Gao et al., 2021a; Gao et al., 2021b; Goodall and Wickramasinghe, 2021).
Metastasis-associated lung adenocarcinoma transcript 1 (MALAT1) was initially found to regulate cancer metastasis. The RNA sequencing profile used to analyze lncRNA expression showed that MALAT1 was significantly upregulated in HUVECs treated with hypoxia (Michalik et al., 2014). Silencing of MALAT1 increased basal endothelial cell migration and sprouting in vitro. MALAT1 can activate PI3K and Akt phosphorylation, promoting endothelial apoptosis by sponging miR-126 (Zhang et al., 2020a). Furthermore, MALAT1 can also protect the angiogenesis function through the miR-205-5p/VEGFA pathway (Gao et al., 2020). Microarray data showed that the expression of lncRNA taurine upregulated gene 1 (TUG1) was associated with the ox-LDL concentration. Runt-related transcription factor 2 (Runx2) was detected as the downstream target of TUG1 by RNA pull down, and Runx2 knockdown could reverse the function of aminopeptidase N (ANPEP). TUG1 silencing can promote endothelial injury repair via the repression of Runx2 and ANPEP (Du et al., 2021).
The expression of lncRNA plasmacytoma variant translocation 1 (PVT1) was upregulated in ox-LDL-induced injury in HUVECs. PVT1 knockdown decreased the inflammation and apoptosis in HUVEC via the miR-153-3p/GRB2 axis (Guo et al., 2021). Gao et al. reported that lncRNA H19 could regulate ox-LDL inflammation, apoptosis and HUVECs by targeting the miR-let-7/periostin axis (Cao et al., 2019). The expression level of lncRNA differentiation antagonizing non-protein coding RNA (DANCER) was increased in patients with atherosclerosis and ox-LDL-treated cells. DANCER knockdown significantly reduced the levels of IL-6 and, TNF-α via miR-214-5p sponging, thereby activating the chaperone of cytochrome c oxidase subunit II COX2 (COX20) (Zhang et al., 2022). The down-regulated expression of long intergenic non-coding 00299 (LINC00299) inhibited vascular injury through the miR-135a-5p/XBP1 axis (Chang et al., 2022). The expression level of lncRNA ZEB1-antisense 1 (ZEB1-AS1) was increased in the ox-LDL induced endothelial cell injury model. Nucleotide-binding oligomerization domain 2 (NOD2) was reported to integrate ER stress and innate immunity. ZEB1-AS1 expression can regulate endothelial cell injury via LRPPRC to stabilize NOD2 mRNA (Xu et al., 2019b). The expression level of lncRNA OIP5-AS1 was increased in ox-LDL mediated vascular ED. Zhang et al. demonstrated that OIP5-AS1 knockdown suppressed apoptosis and evaluated cell viability by modulating the expression of miR-320a and regulation of lectin-like oxidized low-density lipoprotein receptor 1 (Zhang et al., 2020b).
4 Conclusion
Diabetic patients have extensive vascular disease. Previous epidemiological data and laboratory mechanism studies have indicated that vascular complications are the leading cause of morbidity and mortality in T2D. During the development of the disease, its damage to blood vessels includes increased thickness of blood vessels, decreased vasodilation, vascular calcification, and impaired vascular response. Awareness of T2D-related diseases and complications, identification of their possible pathogeneses and causes, and development of new interventions are warranted. Recent studies have shown increasing interest in identifying the mechanisms that play a major role in the development of T2D. We reviewed and discussed the roles of ED, inflammation, oxidative stress and epigenetic modification in the pathogenesis of T2D induced by hyperglycemia and hyperlipidemia.
The early symptom of diabetic vascular disease is endothelial damage, which can cause significant dysfunction. The formation of thrombosis and activation of inflammation are the main features of vascular injury, which can decrease vasodilation. The inflammatory process contributes to insulin resistance, and long-term chronic inflammation can further cause endothelial damage and exacerbate oxidative stress. Oxidative stress is mainly due to mitochondria dysfunction. Mitochondria are energy-producing factories, and mitochondrial dysfunction can induce apoptosis and activate inflammation, creating a vicious cycle. In the pathological process of T2D, dysregulation of DNA methylation, histone markers and non-coding RNA activate dormant endothelial cells and initiates various molecular activities, resulting in ED. The interaction between the factors that cause vascular injury is inseparable. ED is the first event to occur and the ultimate point of action of elements.
Continuous experimental, clinical and translational studies have shown that pharmacological interventions targeting glycotoxicity and lipotoxicity play an important role in the treatment of the vascular complications of T2D. Most of these drugs have shown good vascular protection in preclinical and clinical studies. For instance, the common drugs used to treat diabetes, such as insulin and metformin, have been shown to be effective in stimulating the release of NO and maintaining vascular stability (Tousoulis et al., 2008; Aggarwal et al., 2021). Moreover, metformin can regulate AMPK-related pathways to protect endothelial cells (Ding et al., 2021). Sodium-glucose cotransporter 2 inhibitors (SGLT2i) are new-generation drugs for the treatment of T2D and its complications (DeFronzo et al., 2021). The mechanisms of action of SGLT2i include anti-inflammatory, anti-proliferative, and antifibrotic effects (Heerspink et al., 2019; DeFronzo et al., 2021). The protective effects of GLP-1 receptor agonists mainly include maintaining the integrity of the intima, reducing the adhesion of monocytes stimulated by ox-LDL, increasing the level of NO production, reducing the release of inflammatory factor, and blocking inflammatory pathways (Chang et al., 2019; Xu et al., 2019c). Moreover, statins, which are lipid-lowering drugs, can reduce LDL content and increase the bioavailability of NO. They have anti-apoptotic and anti-inflammatory effects, which reduce the release of inflammatory factors and inhibit leukocyte adhesion. Antihypertensive drugs such as calcium channel blockers (CCBs), ARBs, and ACEI can also improve the endothelial function and increase the eNOS expression level (Silva et al., 2019). Other studies suggested that ACEI, and, ARBs could also attenuate ROS-induced injury by enhancing the activity of superoxide dismutase. Anti-inflammatory drugs, including nonselective (aspirin) and selective COX-2 inhibitors, have been studied to reduce cardiovascular risk and recurrence of cardiovascular events in patients with T2D (Huang and Vita, 2006). Some natural ingredients such as colchicine have been used clinically for the treatment of ED and cardiovascular disease. Angelica sinensis polysaccharide, which is purified from the fresh roots of Angelica sinensis, could regulate glucose and lipid metabolisms by reducing the release of inflammatory factors (Wang et al., 2015). Lifestyle changes, including diet and exercise, are also the primary recommendations. The mechanism of action of exercise therapy involves promoting the release of cytokines and increasing the uptake and utilization of glucose and lipid hydrolysis (Yang et al., 2019b).
By better understanding the etiology of vascular diseases, combining the relevant mechanisms of new and old drugs, and developing targeted interventions for the vascular complications of diabetes, cardiovascular morbidity and mortality rates in patients with T2D can be reduced.
Author contributions
JG, PL, and XC conceived this manuscript. XC wrote the manuscript. CS, YW, HY, YZ, and JZ revised the manuscript.
Funding
This work was funded by the Major Research Program of the National Natural Science Foundation of China (91849209), the National Natural Science Foundation of China (81800264) and Launch Fund of Qingdao University (DC1900003122).
Acknowledgments
The graphical abstract was modified from Servier Medical Art (https://smart.servier.com/), licensed under a Creative Common Attribution 3.0 Generic License (https://creativecommons.org/licenses/by/3.0/).
Conflict of interest
The authors declare that the research was conducted in the absence of any commercial or financial relationships that could be construed as a potential conflict of interest.
Publisher’s note
All claims expressed in this article are solely those of the authors and do not necessarily represent those of their affiliated organizations, or those of the publisher, the editors and the reviewers. Any product that may be evaluated in this article, or claim that may be made by its manufacturer, is not guaranteed or endorsed by the publisher.
References
Aggarwal H., Pathak P., Kumar Y., Jagavelu K., Dikshit M. (2021). Modulation of insulin resistance, dyslipidemia and serum metabolome in inos knockout mice following treatment with nitrite, metformin, pioglitazone, and a combination of ampicillin and neomycin. Int. J. Mol. Sci. 23 (1), 195. Epub 2022/01/12. doi:10.3390/ijms23010195
Alex L., Russo I., Holoborodko V., Frangogiannis N. G. (2018). Characterization of a mouse model of obesity-related fibrotic cardiomyopathy that recapitulates features of human heart failure with preserved ejection fraction. Am. J. Physiol. Heart Circ. Physiol. 315 (4), H934–h49. Epub 2018/07/14. doi:10.1152/ajpheart.00238.2018
Altesha M. A., Ni T., Khan A., Liu K., Zheng X. (2019). Circular rna in cardiovascular disease. J. Cell. Physiol. 234 (5), 5588–5600. Epub 2018/10/21. doi:10.1002/jcp.27384
Aminzadeh M. A., Nicholas S. B., Norris K. C., Vaziri N. D. (2013). Role of impaired Nrf2 activation in the pathogenesis of oxidative stress and inflammation in chronic tubulo-interstitial nephropathy. Nephrol. Dial. Transplant. 28 (8), 2038–2045. official publication of the European Dialysis and Transplant Association - European Renal AssociationEpub 2013/03/21. doi:10.1093/ndt/gft022
Aref-Eshghi E., Biswas S., Chen C., Sadikovic B., Chakrabarti S. (2020). Glucose-induced, duration-dependent genome-wide DNA methylation changes in human endothelial cells. Am. J. Physiol. Cell Physiol. 319 (2), C268–c76. Epub 2020/05/28. doi:10.1152/ajpcell.00011.2020
Assaf L., Eid A. A., Nassif J. (2022). Role of ampk/mtor, mitochondria, and ros in the pathogenesis of endometriosis. Life Sci. 306, 120805. Epub 2022/07/20. doi:10.1016/j.lfs.2022.120805
Baird L., Yamamoto M. (2020). The molecular mechanisms regulating the keap1-nrf2 pathway. Mol. Cell. Biol. 40 (13), e00099-20. Epub 2020/04/15. doi:10.1128/mcb.00099-20
Barber T. M., Kyrou I., Randeva H. S., Weickert M. O. (2021). Mechanisms of insulin resistance at the crossroad of obesity with associated metabolic abnormalities and cognitive dysfunction. Int. J. Mol. Sci. 22 (2), E546. Epub 2021/01/13. doi:10.3390/ijms22020546
Barlovic D. P., Soro-Paavonen A., Jandeleit-Dahm K. A. (2011). Rage biology, atherosclerosis and diabetes. Clin. Sci. 121 (2), 43–55. Epub 2011/04/05. doi:10.1042/cs20100501
Becker B. F., Chappell D., Jacob M. (2010). Endothelial glycocalyx and coronary vascular permeability: The fringe benefit. Basic Res. Cardiol. 105 (6), 687–701. Epub 2010/09/23. doi:10.1007/s00395-010-0118-z
Becker B. F., Jacob M., Leipert S., Salmon A. H., Chappell D. (2015). Degradation of the endothelial glycocalyx in clinical settings: Searching for the sheddases. Br. J. Clin. Pharmacol. 80 (3), 389–402. Epub 2015/03/18. doi:10.1111/bcp.12629
Beckman J. A., Creager M. A. (2016). Vascular complications of diabetes. Circ. Res. 118 (11), 1771–1785. Epub 2016/05/28. doi:10.1161/circresaha.115.306884
Bierhaus A., Schiekofer S., Schwaninger M., Andrassy M., Humpert P. M., Chen J., et al. (2001). Diabetes-associated sustained activation of the transcription factor nuclear factor-kappab. Diabetes 50 (12), 2792–2808. Epub 2001/11/28. doi:10.2337/diabetes.50.12.2792
Biswas S., Feng B., Thomas A., Chen S., Aref-Eshghi E., Sadikovic B., et al. (2018). Endothelin-1 regulation is entangled in a complex web of epigenetic mechanisms in diabetes. Physiol. Res. 67 (1), S115–s25. Epub 2018/06/28. doi:10.33549/physiolres.933836
Brasacchio D., Okabe J., Tikellis C., Balcerczyk A., George P., Baker E. K., et al. (2009). Hyperglycemia induces a dynamic cooperativity of histone methylase and demethylase enzymes associated with gene-activating epigenetic marks that coexist on the lysine tail. Diabetes 58 (5), 1229–1236. Epub 2009/02/12. doi:10.2337/db08-1666
Brownlee M. (2005). The pathobiology of diabetic complications: A unifying mechanism. Diabetes 54 (6), 1615–1625. Epub 2005/05/28. doi:10.2337/diabetes.54.6.1615
Byun K., Yoo Y., Son M., Lee J., Jeong G. B., Park Y. M., et al. (2017). Advanced glycation end-products produced systemically and by macrophages: A common contributor to inflammation and degenerative diseases. Pharmacol. Ther. 177, 44–55. Epub 2017/02/23. doi:10.1016/j.pharmthera.2017.02.030
Cao L., Zhang Z., Li Y., Zhao P., Chen Y. (2019). Lncrna H19/mir-let-7 Axis participates in the regulation of ox-ldl-induced endothelial cell injury via targeting periostin. Int. Immunopharmacol. 72, 496–503. Epub 2019/05/06. doi:10.1016/j.intimp.2019.04.042
Cao Y., Yuan G., Zhang Y., Lu R. (2018). High glucose-induced Circhipk3 downregulation mediates endothelial cell injury. Biochem. Biophys. Res. Commun. 507 (1-4), 362–368. Epub 2018/11/21. doi:10.1016/j.bbrc.2018.11.041
Chang M., Liu G., Wang Y., Lv H., Jin Y. (2022). Long non-coding rna Linc00299 knockdown inhibits ox-ldl-induced T/G ha-vsmc injury by regulating mir-135a-5p/xbp1 Axis in atherosclerosis. Panminerva Med. 64 (1), 38–47. Epub 2020/07/24. doi:10.23736/s0031-0808.20.03942-7
Chang W., Zhu F., Zheng H., Zhou Z., Miao P., Zhao L., et al. (2019). Glucagon-like peptide-1 receptor agonist dulaglutide prevents ox-ldl-induced adhesion of monocytes to human endothelial cells: An implication in the treatment of atherosclerosis. Mol. Immunol. 116, 73–79. Epub 2019/10/21. doi:10.1016/j.molimm.2019.09.021
Chen H., Feng Z., Li L., Fan L. (2021). Microrna-9 rescues hyperglycemia-induced endothelial cell dysfunction and promotes arteriogenesis through downregulating Notch1 signaling. Mol. Cell. Biochem. 476 (7), 2777–2789. Epub 2021/03/16. doi:10.1007/s11010-021-04075-8
Chen J., Cui L., Yuan J., Zhang Y., Sang H. (2017). Circular rna Wdr77 target fgf-2 to regulate vascular smooth muscle cells proliferation and migration by sponging mir-124. Biochem. Biophys. Res. Commun. 494 (1-2), 126–132. Epub 2017/10/19. doi:10.1016/j.bbrc.2017.10.068
Chen S., Feng B., George B., Chakrabarti R., Chen M., Chakrabarti S. (2010). Transcriptional coactivator P300 regulates glucose-induced gene expression in endothelial cells. Am. J. Physiol. Endocrinol. Metab. 298 (1), E127–E137. Epub 2009/11/12. doi:10.1152/ajpendo.00432.2009
Chen S. C., Guh J. Y., Hwang C. C., Chiou S. J., Lin T. D., Ko Y. M., et al. (2010). Advanced glycation end-products activate extracellular signal-regulated kinase via the oxidative stress-egf receptor pathway in renal fibroblasts. J. Cell. Biochem. 109 (1), 38–48. Epub 2009/11/04. doi:10.1002/jcb.22376
Chen X., Yu H., Li Z., Ye W., Liu Z., Gao J., et al. (2022). Oxidative rna damage in the pathogenesis and treatment of type 2 diabetes. Front. Physiol. 13, 725919. Epub 2022/04/15. doi:10.3389/fphys.2022.725919
Cheng J., Liu Q., Hu N., Zheng F., Zhang X., Ni Y., et al. (2019). Downregulation of Hsa_Circ_0068087 ameliorates tlr4/nf-?b/nlrp3 inflammasome-mediated inflammation and endothelial cell dysfunction in high glucose conditioned by sponging mir-197. Gene 709, 1–7. Epub 2019/05/21. doi:10.1016/j.gene.2019.05.012
Cheng S., Cui Y., Fan L., Mu X., Hua Y. (2018). T2dm inhibition of endothelial mir-342-3p facilitates angiogenic dysfunction via repression of Fgf11 signaling. Biochem. Biophys. Res. Commun. 503 (1), 71–78. Epub 2018/06/01. doi:10.1016/j.bbrc.2018.05.179
Chiarugi P., Cirri P. (2003). Redox regulation of protein tyrosine phosphatases during receptor tyrosine kinase signal transduction. Trends biochem. Sci. 28 (9), 509–514. Epub 2003/09/19. doi:10.1016/s0968-0004(03)00174-9
Coleman C. B., Lightell D. J., Moss S. C., Bates M., Parrino P. E., Woods T. C. (2013). Elevation of mir-221 and -222 in the internal mammary arteries of diabetic subjects and normalization with metformin. Mol. Cell. Endocrinol. 374 (1-2), 125–129. Epub 2013/05/08. doi:10.1016/j.mce.2013.04.019
Cortés-López M., Gruner M. R., Cooper D. A., Gruner H. N., Voda A. I., van der Linden A. M., et al. (2018). Global accumulation of circrnas during aging in Caenorhabditis elegans. BMC genomics 19 (1), 8. Epub 2018/01/05. doi:10.1186/s12864-017-4386-y
Coughlan M. T., Thorburn D. R., Penfold S. A., Laskowski A., Harcourt B. E., Sourris K. C., et al. (2009). Rage-induced cytosolic ros promote mitochondrial superoxide generation in diabetes. J. Am. Soc. Nephrol. 20 (4), 742–752. Epub 2009/01/23. doi:10.1681/asn.2008050514
DeFronzo R. A., Reeves W. B., Awad A. S. (2021). Pathophysiology of diabetic kidney disease: Impact of Sglt2 inhibitors. Nat. Rev. Nephrol. 17 (5), 319–334. Epub 2021/02/07. doi:10.1038/s41581-021-00393-8
Dhawan P., Vasishta S., Balakrishnan A., Joshi M. B. (2022). Mechanistic insights into glucose induced vascular epigenetic reprogramming in type 2 diabetes. Life Sci. 298, 120490. Epub 2022/03/26. doi:10.1016/j.lfs.2022.120490
Ding Y., Zhou Y., Ling P., Feng X., Luo S., Zheng X., et al. (2021). Metformin in cardiovascular diabetology: A focused review of its impact on endothelial function. Theranostics 11 (19), 9376–9396. Epub 2021/10/15. doi:10.7150/thno.64706
Du H., Yang L., Zhang H., Zhang X., Shao H. (2021). Lncrna Tug1 silencing enhances proliferation and migration of ox-ldl-treated human umbilical vein endothelial cells and promotes atherosclerotic vascular injury repairing via the runx2/anpep Axis. Int. J. Cardiol. 338, 204–214. Epub 2021/05/11. doi:10.1016/j.ijcard.2021.05.014
Dunn J., Thabet S., Jo H. (2015). Flow-dependent epigenetic DNA methylation in endothelial gene expression and atherosclerosis. Arterioscler. Thromb. Vasc. Biol. 35 (7), 1562–1569. Epub 2015/05/09. doi:10.1161/atvbaha.115.305042
Erkens R., Suvorava T., Kramer C. M., Diederich L. D., Kelm M., Cortese-Krott M. M. (2017). Modulation of local and systemic heterocellular communication by mechanical forces: A role of endothelial nitric oxide synthase. Antioxid. Redox Signal. 26 (16), 917–935. Epub 2016/12/09. doi:10.1089/ars.2016.6904
Franzke B., Schober-Halper B., Hofmann M., Oesen S., Tosevska A., Henriksen T., et al. (2018). Age and the effect of exercise, nutrition and cognitive training on oxidative stress - the vienna active aging study (vaas), a randomized controlled trial. Free Radic. Biol. Med. 121, 69–77. Epub 2018/04/27. doi:10.1016/j.freeradbiomed.2018.04.565
Gao C., Zhang C. C., Yang H. X., Hao Y. N. (2020). Malat1 protected the angiogenesis function of human brain microvascular endothelial cells (hbmecs) under oxygen glucose deprivation/Re-oxygenation (ogd/R) challenge by interacting with mir-205-5p/vegfa pathway. Neuroscience 435, 135–145. Epub 2020/03/29. doi:10.1016/j.neuroscience.2020.03.027
Gao J., Chen X., Shan C., Wang Y., Li P., Shao K. (2021). Autophagy in cardiovascular diseases: Role of noncoding rnas. Mol. Ther. Nucleic Acids 23, 101–118. Epub 2020/12/19. doi:10.1016/j.omtn.2020.10.039
Gao J., Chen X., Wei P., Wang Y., Li P., Shao K. (2021). Regulation of pyroptosis in cardiovascular pathologies: Role of noncoding rnas. Mol. Ther. Nucleic Acids 25, 220–236. Epub 2021/08/31. doi:10.1016/j.omtn.2021.05.016
Georgakis M. K., Harshfield E. L., Malik R., Franceschini N., Langenberg C., Wareham N. J., et al. (2021). Diabetes mellitus, glycemic traits, and cerebrovascular disease: A mendelian randomization study. Neurology 96 (13), e1732–e1742. Epub 2021/01/27. doi:10.1212/wnl.0000000000011555
Goodall G. J., Wickramasinghe V. O. (2021). Rna in cancer. Nat. Rev. Cancer 21 (1), 22–36. Epub 2020/10/22. doi:10.1038/s41568-020-00306-0
Greenberg M. V. C., Bourc'his D. (2019). The diverse roles of DNA methylation in mammalian development and disease. Nat. Rev. Mol. Cell Biol. 20 (10), 590–607. Epub 2019/08/11. doi:10.1038/s41580-019-0159-6
Guo J., Li J., Zhang J., Guo X., Liu H., Li P., et al. (2021). Lncrna Pvt1 knockdown alleviated ox-ldl-induced vascular endothelial cell injury and atherosclerosis by mir-153-3p/grb2 Axis via erk/P38 pathway. Nutr. Metab. Cardiovasc. Dis. 31 (12), 3508–3521. Epub 2021/10/11. doi:10.1016/j.numecd.2021.08.031
Ha M., Kim V. N. (2014). Regulation of microrna biogenesis. Nat. Rev. Mol. Cell Biol. 15 (8), 509–524. Epub 2014/07/17. doi:10.1038/nrm3838
Habibi F., Ghadiri Soufi F., Ghiasi R., Khamaneh A. M., Alipour M. R. (2016). Alteration in inflammation-related mir-146a expression in nf-kb signaling pathway in diabetic rat Hippocampus. Adv. Pharm. Bull. 6 (1), 99–103. Epub 2016/04/29. doi:10.15171/apb.2016.015
Heerspink H. J. L., Perco P., Mulder S., Leierer J., Hansen M. K., Heinzel A., et al. (2019). Canagliflozin reduces inflammation and fibrosis biomarkers: A potential mechanism of action for beneficial effects of Sglt2 inhibitors in diabetic kidney disease. Diabetologia 62 (7), 1154–1166. Epub 2019/04/20. doi:10.1007/s00125-019-4859-4
Heusch G. (2020). Myocardial ischaemia-reperfusion injury and cardioprotection in perspective. Nat. Rev. Cardiol. 17 (12), 773–789. Epub 2020/07/06. doi:10.1038/s41569-020-0403-y
Huang A. L., Vita J. A. (2006). Effects of systemic inflammation on endothelium-dependent vasodilation. Trends cardiovasc. Med. 16 (1), 15–20. Epub 2006/01/03. doi:10.1016/j.tcm.2005.10.002
Huang C., Shen S., Ma Q., Chen J., Gill A., Pollock C. A., et al. (2013). Blockade of Kca3.1 ameliorates renal fibrosis through the tgf-ß1/smad pathway in diabetic mice. Diabetes 62 (8), 2923–2934. Epub 2013/05/10. doi:10.2337/db13-0135
Iacobini C., Vitale M., Pesce C., Pugliese G., Menini S. (2021). Diabetic complications and oxidative stress: A 20-year voyage back in time and back to the future. Antioxidants (Basel, Switz. 10 (5), 727. Epub 2021/06/03. doi:10.3390/antiox10050727
Iliff A. J., Xu X. Z. S. (2018). A mechanosensitive gpcr that detects the bloody force. Cell 173 (3), 542–544. Epub 2018/04/21. doi:10.1016/j.cell.2018.04.001
Iwashima Y., Eto M., Hata A., Kaku K., Horiuchi S., Ushikubi F., et al. (2000). Advanced glycation end products-induced gene expression of scavenger receptors in cultured human monocyte-derived macrophages. Biochem. Biophys. Res. Commun. 277 (2), 368–380. Epub 2000/10/18. doi:10.1006/bbrc.2000.3685
Jedlicka J., Becker B. F., Chappell D. (2020). Endothelial glycocalyx. Crit. Care Clin. 36 (2), 217–232. Epub 2020/03/17. doi:10.1016/j.ccc.2019.12.007
Jeyabal P., Thandavarayan R. A., Joladarashi D., Suresh Babu S., Krishnamurthy S., Bhimaraj A., et al. (2016). Microrna-9 inhibits hyperglycemia-induced pyroptosis in human ventricular cardiomyocytes by targeting Elavl1. Biochem. Biophys. Res. Commun. 471 (4), 423–429. Epub 2016/02/24. doi:10.1016/j.bbrc.2016.02.065
Jiang W. L., Zhang Y. F., Xia Q. Q., Zhu J., Yu X., Fan T., et al. (2015). Microrna-19a regulates lipopolysaccharide-induced endothelial cell apoptosis through modulation of apoptosis signal-regulating kinase 1 expression. BMC Mol. Biol. 16, 11. Epub 2015/05/20. doi:10.1186/s12867-015-0034-8
Jin G., Wang Q., Hu X., Li X., Pei X., Xu E., et al. (2019). Profiling and functional analysis of differentially expressed circular rnas in high glucose-induced human umbilical vein endothelial cells. FEBS open bio 9 (9), 1640–1651. Epub 2019/08/02. doi:10.1002/2211-5463.12709
Kato M., Natarajan R. (2014). Diabetic nephropathy-emerging epigenetic mechanisms. Nat. Rev. Nephrol. 10 (9), 517–530. Epub 2014/07/09. doi:10.1038/nrneph.2014.116
Kristensen L. S., Andersen M. S., Stagsted L. V. W., Ebbesen K. K., Hansen T. B., Kjems J. (2019). The biogenesis, biology and characterization of circular rnas. Nat. Rev. Genet. 20 (11), 675–691. Epub 2019/08/10. doi:10.1038/s41576-019-0158-7
Krüger-Genge A., Blocki A., Franke R. P., Jung F. (2019). Vascular endothelial cell biology: An update. Int. J. Mol. Sci. 20 (18), E4411. Epub 2019/09/11. doi:10.3390/ijms20184411
Kumar V., Agrawal R., Pandey A., Kopf S., Hoeffgen M., Kaymak S., et al. (2020). Compromised DNA repair is responsible for diabetes-associated fibrosis. EMBO J. 39 (11), e103477. Epub 2020/04/28. doi:10.15252/embj.2019103477
Kuschnerus K., Straessler E. T., Müller M. F., Lüscher T. F., Landmesser U., Kränkel N. (2019). Increased expression of mir-483-3p impairs the vascular response to injury in type 2 diabetes. Diabetes 68 (2), 349–360. Epub 2018/09/28. doi:10.2337/db18-0084
Li G., Zhu S., Zeng J., Yu Z., Meng F., Tang Z., et al. (2022). Arterial pulsatility augments microcirculatory perfusion and maintains the endothelial integrity during extracorporeal membrane oxygenation via Hsa_Circ_0007367 upregulation in a canine model with cardiac arrest. Oxid. Med. Cell. Longev. 2022, 1630918. Epub 2022/03/01. doi:10.1155/2022/1630918
Li H., Liu X., Sun N., Wang T., Zhu J., Yang S., et al. (2021). Differentially expressed circular non-coding rnas in atherosclerotic aortic vessels and their potential functions in endothelial injury. Front. Cardiovasc. Med. 8, 657544. Epub 2021/07/27. doi:10.3389/fcvm.2021.657544
Li H., Xiao Y., Tang L., Zhong F., Huang G., Xu J. M., et al. (2018). Adipocyte fatty acid-binding protein promotes palmitate-induced mitochondrial dysfunction and apoptosis in macrophages. Front. Immunol. 9, 81. Epub 2018/02/15. doi:10.3389/fimmu.2018.00081
Li J., Wang J., Wang Z. (2021). Circ_0006768 upregulation attenuates oxygen-glucose deprivation/reoxygenation-induced human brain microvascular endothelial cell injuries by upregulating Vezf1 via mir-222-3p inhibition. Metab. Brain Dis. 36 (8), 2521–2534. Epub 2021/06/20. doi:10.1007/s11011-021-00775-8
Li Q., Kim Y. R., Vikram A., Kumar S., Kassan M., Gabani M., et al. (2016). P66shc-Induced microrna-34a causes diabetic endothelial dysfunction by downregulating Sirtuin1. Arterioscler. Thromb. Vasc. Biol. 36 (12), 2394–2403. Epub 2016/10/30. doi:10.1161/atvbaha.116.308321
Li S., Huang T., Qin L., Yin L. (2021). Circ_0068087 silencing ameliorates oxidized low-density lipoprotein-induced dysfunction in vascular endothelial cells depending on mir-186-5p-mediated regulation of roundabout guidance receptor 1. Front. Cardiovasc. Med. 8, 650374. Epub 2021/06/15. doi:10.3389/fcvm.2021.650374
Li Y., Reddy M. A., Miao F., Shanmugam N., Yee J. K., Hawkins D., et al. (2008). Role of the histone H3 lysine 4 methyltransferase, set7/9, in the regulation of nf-kappab-dependent inflammatory genes. Relevance to diabetes and inflammation. J. Biol. Chem. 283 (39), 26771–26781. Epub 2008/07/25. doi:10.1074/jbc.M802800200
Liao Y., Gou L., Chen L., Zhong X., Zhang D., Zhu H., et al. (2018). Nadph oxidase 4 and endothelial nitric oxide synthase contribute to endothelial dysfunction mediated by histone methylations in metabolic memory. Free Radic. Biol. Med. 115, 383–394. Epub 2017/12/23. doi:10.1016/j.freeradbiomed.2017.12.017
Lightell D. J., Moss S. C., Woods T. C. (2018). Upregulation of mir-221 and -222 in response to increased extracellular signal-regulated kinases 1/2 activity exacerbates neointimal hyperplasia in diabetes mellitus. Atherosclerosis 269, 71–78. Epub 2017/12/26. doi:10.1016/j.atherosclerosis.2017.12.016
Liu H., Ma X., Mao Z., Shen M., Zhu J., Chen F. (2020). Circular rna Has_Circ_0003204 inhibits oxldl-induced vascular endothelial cell proliferation and angiogenesis. Cell. Signal. 70, 109595. Epub 2020/03/11. doi:10.1016/j.cellsig.2020.109595
Lucchesi A. N., Cassettari L. L., Spadella C. T. (2015). Alloxan-induced diabetes causes morphological and ultrastructural changes in rat liver that resemble the natural history of chronic fatty liver disease in humans. J. Diabetes Res. 2015, 494578. Epub 2015/03/20. doi:10.1155/2015/494578
Mei X., Cui X. B., Li Y., Chen S. Y. (2021). Circsod2: A novel regulator for smooth muscle proliferation and neointima formation. Arterioscler. Thromb. Vasc. Biol. 41 (12), 2961–2973. Epub 2021/10/22. doi:10.1161/atvbaha.121.316911
Miao F., Li S., Chavez V., Lanting L., Natarajan R. (2006). Coactivator-associated arginine methyltransferase-1 enhances nuclear factor-kappab-mediated gene transcription through methylation of histone H3 at arginine 17. Mol. Endocrinol. 20 (7), 1562–1573. Epub 2006/02/25. doi:10.1210/me.2005-0365
Michalik K. M., You X., Manavski Y., Doddaballapur A., Zörnig M., Braun T., et al. (2014). Long noncoding rna Malat1 regulates endothelial cell function and vessel growth. Circ. Res. 114 (9), 1389–1397. Epub 2014/03/08. doi:10.1161/circresaha.114.303265
Moncada S., Palmer R. M., Higgs E. A. (1991). Nitric oxide: Physiology, pathophysiology, and pharmacology. Pharmacol. Rev. 43 (2), 109–142. Epub 1991/06/01.
Montezano A. C., Nguyen D., Cat A., Rios F. J., Touyz R. M. (2014). Angiotensin ii and vascular injury. Curr. Hypertens. Rep. 16 (6), 431. Epub 2014/04/25. doi:10.1007/s11906-014-0431-2
Natarajan R. (2021). Epigenetic mechanisms in diabetic vascular complications and metabolic memory: The 2020 edwin bierman award lecture. Diabetes 70 (2), 328–337. Epub 2021/01/22. doi:10.2337/dbi20-0030
Nishikawa T., Edelstein D., Brownlee M. (2000). The missing link: A single unifying mechanism for diabetic complications. Kidney Int. Suppl. 77, S26–S30. Epub 2000/09/21. doi:10.1046/j.1523-1755.2000.07705.x
Nosadini R., Tonolo G. (2011). Role of oxidized low density lipoproteins and free fatty acids in the pathogenesis of glomerulopathy and tubulointerstitial lesions in type 2 diabetes. Nutr. Metab. Cardiovasc. Dis. 21 (2), 79–85. Epub 2010/12/28. doi:10.1016/j.numecd.2010.10.002
Ogurtsova K., da Rocha Fernandes J. D., Huang Y., Linnenkamp U., Guariguata L., Cho N. H., et al. (2017). Idf diabetes atlas: Global estimates for the prevalence of diabetes for 2015 and 2040. Diabetes Res. Clin. Pract. 128, 40–50. Epub 2017/04/25. doi:10.1016/j.diabres.2017.03.024
Opazo-Ríos L., Mas S., Marín-Royo G., Mezzano S., Gómez-Guerrero C., Moreno J. A., et al. (2020). Lipotoxicity and diabetic nephropathy: Novel mechanistic insights and therapeutic opportunities. Int. J. Mol. Sci. 21 (7), E2632. Epub 2020/04/16. doi:10.3390/ijms21072632
Orimo M., Minamino T., Miyauchi H., Tateno K., Okada S., Moriya J., et al. (2009). Protective role of Sirt1 in diabetic vascular dysfunction. Arterioscler. Thromb. Vasc. Biol. 29 (6), 889–894. Epub 2009/03/17. doi:10.1161/atvbaha.109.185694
Pandolfi A., Cetrullo D., Polishuck R., Alberta M. M., Calafiore A., Pellegrini G., et al. (2001). Plasminogen activator inhibitor type 1 is increased in the arterial wall of type ii diabetic subjects. Arterioscler. Thromb. Vasc. Biol. 21 (8), 1378–1382. Epub 2001/08/11. doi:10.1161/hq0801.093667
Paneni F., Mocharla P., Akhmedov A., Costantino S., Osto E., Volpe M., et al. (2012). Gene silencing of the mitochondrial adaptor P66(shc) suppresses vascular hyperglycemic memory in diabetes. Circ. Res. 111 (3), 278–289. Epub 2012/06/14. doi:10.1161/circresaha.112.266593
Papachristoforou E., Lambadiari V., Maratou E., Makrilakis K. (2020). Association of glycemic indices (hyperglycemia, glucose variability, and hypoglycemia) with oxidative stress and diabetic complications. J. Diabetes Res. 2020, 7489795. Epub 2020/10/31. doi:10.1155/2020/7489795
Pepin M. E., Schiano C., Miceli M., Benincasa G., Mansueto G., Grimaldi V., et al. (2021). The human aortic endothelium undergoes dose-dependent DNA methylation in response to transient hyperglycemia. Exp. Cell Res. 400 (2), 112485. Epub 2021/01/31. doi:10.1016/j.yexcr.2021.112485
Pirola L., Balcerczyk A., Okabe J., El-Osta A. (2010). Epigenetic phenomena linked to diabetic complications. Nat. Rev. Endocrinol. 6 (12), 665–675. Epub 2010/11/04. doi:10.1038/nrendo.2010.188
Pirola L., Balcerczyk A., Tothill R. W., Haviv I., Kaspi A., Lunke S., et al. (2011). Genome-wide analysis distinguishes hyperglycemia regulated epigenetic signatures of primary vascular cells. Genome Res. 21 (10), 1601–1615. Epub 2011/09/06. doi:10.1101/gr.116095.110
Pitocco D., Tesauro M., Alessandro R., Ghirlanda G., Cardillo C. (2013). Oxidative stress in diabetes: Implications for vascular and other complications. Int. J. Mol. Sci. 14 (11), 21525–21550. Epub 2013/11/02. doi:10.3390/ijms141121525
Qin M., Wang W., Zhou H., Wang X., Wang F., Wang H. (2020). Circular rna Circ_0003645 silencing alleviates inflammation and apoptosis via the nf-?b pathway in endothelial cells induced by oxldl. Gene 755, 144900. Epub 2020/06/20. doi:10.1016/j.gene.2020.144900
Qin X., Wang X., Wang Y., Tang Z., Cui Q., Xi J., et al. (2010). Microrna-19a mediates the suppressive effect of laminar flow on cyclin D1 expression in human umbilical vein endothelial cells. Proc. Natl. Acad. Sci. U. S. A. 107 (7), 3240–3244. Epub 2010/02/06. doi:10.1073/pnas.0914882107
Quinn J. J., Chang H. Y. (2016). Unique features of long non-coding rna biogenesis and function. Nat. Rev. Genet. 17 (1), 47–62. Epub 2015/12/17. doi:10.1038/nrg.2015.10
Rajasekar P., O'Neill C. L., Eeles L., Stitt A. W., Medina R. J. (2015). Epigenetic changes in endothelial progenitors as a possible cellular basis for glycemic memory in diabetic vascular complications. J. Diabetes Res. 2015, 436879. Epub 2015/06/25. doi:10.1155/2015/436879
Rajendran S., Shen X., Glawe J., Kolluru G. K., Kevil C. G. (2019). Nitric oxide and hydrogen sulfide regulation of ischemic vascular growth and remodeling. Compr. Physiol. 9 (3), 1213–1247. Epub 2019/06/13. doi:10.1002/cphy.c180026
Ren X., Ren L., Wei Q., Shao H., Chen L., Liu N. (2017). Advanced glycation end-products decreases expression of endothelial nitric oxide synthase through oxidative stress in human coronary artery endothelial cells. Cardiovasc. Diabetol. 16 (1), 52. Epub 2017/04/22. doi:10.1186/s12933-017-0531-9
Rizwan H., Pal S., Sabnam S., Pal A. (2020). High glucose augments ros generation regulates mitochondrial dysfunction and apoptosis via stress signalling cascades in keratinocytes. Life Sci. 241, 117148. Epub 2019/12/13. doi:10.1016/j.lfs.2019.117148
Ruiz H. H., Ramasamy R., Schmidt A. M. (2020). Advanced glycation end products: Building on the concept of the "common soil" in metabolic disease. Endocrinology 161 (1), bqz006. Epub 2019/10/23. doi:10.1210/endocr/bqz006
Sandoo A., van Zanten J. J., Metsios G. S., Carroll D., Kitas G. D. (2010). The endothelium and its role in regulating vascular tone. Open cardiovasc. Med. J. 4, 302–312. Epub 2011/02/23. doi:10.2174/1874192401004010302
Santilli F., D'Ardes D., Davì G. (2015). Oxidative stress in chronic vascular disease: From prediction to prevention. Vasc. Pharmacol. 74, 23–37. Epub 2015/09/13. doi:10.1016/j.vph.2015.09.003
Schöttker B., Xuan Y., Gào X., Anusruti A., Brenner H. (2020). Oxidatively damaged DNA/rna and 8-isoprostane levels are associated with the development of type 2 diabetes at older age: Results from a large cohort study. Diabetes care 43 (1), 130–136. Epub 2019/10/28. doi:10.2337/dc19-1379
Schwab U., Lankinen M., Laakso M. (2021). Effect of lifestyle intervention on the risk of incident diabetes in individuals with impaired fasting glucose and low or high genetic risk for the development of type 2 diabetes in men: A t2d-gene trial. Food & Nutr. Res. 65. Epub 2021/10/16. doi:10.29219/fnr.v65.7721
Shah M. S., Brownlee M. (2016). Molecular and cellular mechanisms of cardiovascular disorders in diabetes. Circ. Res. 118 (11), 1808–1829. Epub 2016/05/28. doi:10.1161/circresaha.116.306923
Sharma A., Tate M., Mathew G., Vince J. E., Ritchie R. H., de Haan J. B. (2018). Oxidative stress and nlrp3-inflammasome activity as significant drivers of diabetic cardiovascular complications: Therapeutic implications. Front. Physiol. 9, 114. Epub 2018/03/09. doi:10.3389/fphys.2018.00114
Shen X., Li Y., Sun G., Guo D., Bai X. (2018). Mir-181c-3p and -5p promotes high-glucose-induced dysfunction in human umbilical vein endothelial cells by regulating leukemia inhibitory factor. Int. J. Biol. Macromol. 115, 509–517. Epub 2018/04/02. doi:10.1016/j.ijbiomac.2018.03.173
Silambarasan M., Tan J. R., Karolina D. S., Armugam A., Kaur C., Jeyaseelan K. (2016). Micrornas in hyperglycemia induced endothelial cell dysfunction. Int. J. Mol. Sci. 17 (4), 518. Epub 2016/04/14. doi:10.3390/ijms17040518
Silva I. V. G., de Figueiredo R. C., Rios D. R. A. (2019). Effect of different classes of antihypertensive drugs on endothelial function and inflammation. Int. J. Mol. Sci. 20 (14), E3458. Epub 2019/07/25. doi:10.3390/ijms20143458
Tan K. C., Chow W. S., Ai V. H., Metz C., Bucala R., Lam K. S. (2002). Advanced glycation end products and endothelial dysfunction in type 2 diabetes. Diabetes care 25 (6), 1055–1059. Epub 2002/05/29. doi:10.2337/diacare.25.6.1055
Teodoro J. S., Nunes S., Rolo A. P., Reis F., Palmeira C. M. (2018). Therapeutic options targeting oxidative stress, mitochondrial dysfunction and inflammation to hinder the progression of vascular complications of diabetes. Front. Physiol. 9, 1857. Epub 2019/02/02. doi:10.3389/fphys.2018.01857
Tewari S., Zhong Q., Santos J. M., Kowluru R. A. (2012). Mitochondria DNA replication and DNA methylation in the metabolic memory associated with continued progression of diabetic retinopathy. Invest. Ophthalmol. Vis. Sci. 53 (8), 4881–4888. Epub 2012/06/30. doi:10.1167/iovs.12-9732
Torella D., Iaconetti C., Tarallo R., Marino F., Giurato G., Veneziano C., et al. (2018). Mirna regulation of the hyperproliferative phenotype of vascular smooth muscle cells in diabetes. Diabetes 67 (12), 2554–2568. Epub 2018/09/28. doi:10.2337/db17-1434
Torres M., Forman H. J. (2003). Redox signaling and the map kinase pathways. BioFactors Oxf. Engl. 17 (1-4), 287–296. Epub 2003/08/05. doi:10.1002/biof.5520170128
Tousoulis D., Tsarpalis K., Cokkinos D., Stefanadis C. (2008). Effects of insulin resistance on endothelial function: Possible mechanisms and clinical implications. Diabetes Obes. Metab. 10 (10), 834–842. Epub 2007/11/24. doi:10.1111/j.1463-1326.2007.00818.x
Triggle C. R., Samuel S. M., Ravishankar S., Marei I., Arunachalam G., Ding H. (2012). The endothelium: Influencing vascular smooth muscle in many ways. Can. J. Physiol. Pharmacol. 90 (6), 713–738. Epub 2012/05/26. doi:10.1139/y2012-073
Tuleta I., Frangogiannis N. G. (2021). Diabetic fibrosis. Biochim. Biophys. Acta. Mol. Basis Dis. 1867 (4), 166044. Epub 2020/12/31. doi:10.1016/j.bbadis.2020.166044
Villeneuve L. M., Kato M., Reddy M. A., Wang M., Lanting L., Natarajan R. (2010). Enhanced levels of microrna-125b in vascular smooth muscle cells of diabetic Db/Db mice lead to increased inflammatory gene expression by targeting the histone methyltransferase Suv39h1. Diabetes 59 (11), 2904–2915. Epub 2010/08/12. doi:10.2337/db10-0208
Villeneuve L. M., Reddy M. A., Lanting L. L., Wang M., Meng L., Natarajan R. (2008). Epigenetic histone H3 lysine 9 methylation in metabolic memory and inflammatory phenotype of vascular smooth muscle cells in diabetes. Proc. Natl. Acad. Sci. U. S. A. 105 (26), 9047–9052. Epub 2008/06/27. doi:10.1073/pnas.0803623105
Wan H., You T., Luo W. (2021). Circ_0003204 regulates cell growth, oxidative stress, and inflammation in ox-ldl-induced vascular endothelial cells via regulating mir-942-5p/hdac9 Axis. Front. Cardiovasc. Med. 8, 646832. Epub 2021/04/20. doi:10.3389/fcvm.2021.646832
Wang F., Zhang M. (2021). Circ_001209 aggravates diabetic retinal vascular dysfunction through regulating mir-15b-5p/col12a1. J. Transl. Med. 19 (1), 294. Epub 2021/07/09. doi:10.1186/s12967-021-02949-5
Wang K., Cao P., Shui W., Yang Q., Tang Z., Zhang Y. (2015). Angelica sinensis polysaccharide regulates glucose and lipid metabolism disorder in prediabetic and streptozotocin-induced diabetic mice through the elevation of glycogen levels and reduction of inflammatory factors. Food Funct. 6 (3), 902–909. Epub 2015/01/30. doi:10.1039/c4fo00859f
Widlansky M. E., Jensen D. M., Wang J., Liu Y., Geurts A. M., Kriegel A. J., et al. (2018). Mir-29 contributes to normal endothelial function and can restore it in cardiometabolic disorders. EMBO Mol. Med. 10 (3), e8046. Epub 2018/01/28. doi:10.15252/emmm.201708046
Wils J., Favre J., Bellien J. (2017). Modulating putative endothelial progenitor cells for the treatment of endothelial dysfunction and cardiovascular complications in diabetes. Pharmacol. Ther. 170, 98–115. Epub 2016/10/30. doi:10.1016/j.pharmthera.2016.10.014
Witkowski M., Tabaraie T., Steffens D., Friebel J., Dörner A., Skurk C., et al. (2018). Microrna-19a contributes to the epigenetic regulation of tissue factor in diabetes. Cardiovasc. Diabetol. 17 (1), 34. Epub 2018/02/27. doi:10.1186/s12933-018-0678-z
Xu J., Wei G., Wang J., Zhu J., Yu M., Zeng X., et al. (2019). Glucagon-like peptide-1 receptor activation alleviates lipopolysaccharide-induced acute lung injury in mice via maintenance of endothelial barrier function. Lab. Invest. 99 (4), 577–587. Epub 2019/01/20. doi:10.1038/s41374-018-0170-0
Xu J. Y., Chang N. B., Rong Z. H., Li T., Xiao L., Yao Q. P., et al. (2019). Circdiaph3 regulates rat vascular smooth muscle cell differentiation, proliferation, and migration. FASEB J. official Publ. Fed. Am. Soc. Exp. Biol. 33 (2), 2659–2668. Epub 2018/10/12. doi:10.1096/fj.201800243RRR
Xu X., Ma C., Duan Z., Du Y., Liu C. (2019). Lncrna zeb1-as1 mediates oxidative low-density lipoprotein-mediated endothelial cells injury by post-transcriptional stabilization of Nod2. Front. Pharmacol. 10, 397. Epub 2019/05/02. doi:10.3389/fphar.2019.00397
Yang D., Yang Y., Li Y., Han R. (2019). Physical exercise as therapy for type 2 diabetes mellitus: From mechanism to orientation. Ann. Nutr. Metab. 74 (4), 313–321. Epub 2019/04/24. doi:10.1159/000500110
Yang G., Wu Y., Ye S. (2017). Mir-181c restrains nitration stress of endothelial cells in diabetic Db/Db mice through inhibiting the expression of Foxo1. Biochem. Biophys. Res. Commun. 486 (1), 29–35. Epub 2017/02/23. doi:10.1016/j.bbrc.2017.02.083
Yang J., Zeng P., Yang J., Liu X., Ding J., Wang H., et al. (2018). Microrna-24 regulates vascular remodeling via inhibiting pdgf-bb pathway in diabetic rat model. Gene 659, 67–76. Epub 2018/03/22. doi:10.1016/j.gene.2018.03.056
Yang P., Feng J., Peng Q., Liu X., Fan Z. (2019). Advanced glycation end products: Potential mechanism and therapeutic target in cardiovascular complications under diabetes. Oxidative Med. Cell. Longev. 2019, 9570616. Epub 2019/12/31. doi:10.1155/2019/9570616
Yousefzadeh N., Alipour M. R., Soufi F. G. (2015). Deregulation of nf-?b-mir-146a negative feedback loop may Be involved in the pathogenesis of diabetic neuropathy. J. Physiol. Biochem. 71 (1), 51–58. Epub 2015/01/09. doi:10.1007/s13105-014-0378-4
Yu H., Pan Y., Dai M., Xu H., Li J. (2021). Circ_0003423 alleviates ox-ldl-induced human brain microvascular endothelial cell injury via the mir-589-5p/tet2 network. Neurochem. Res. 46 (11), 2885–2896. Epub 2021/07/07. doi:10.1007/s11064-021-03387-x
Zang J., Lu D., Xu A. (2020). The interaction of circrnas and rna binding proteins: An important part of circrna maintenance and function. J. Neurosci. Res. 98 (1), 87–97. Epub 2018/12/24. doi:10.1002/jnr.24356
Zeng Z., Xia L., Fan S., Zheng J., Qin J., Fan X., et al. (2021). Circular rna Circmap3k5 acts as a microrna-22-3p sponge to promote resolution of intimal hyperplasia via tet2-mediated smooth muscle cell differentiation. Circulation 143 (4), 354–371. Epub 2020/11/20. doi:10.1161/circulationaha.120.049715
Zhang C., Yang H., Li Y., Huo P., Ma P. (2020). Lncrna oip5-as1 regulates oxidative low-density lipoprotein-mediated endothelial cell injury via mir-320a/lox1 Axis. Mol. Cell. Biochem. 467 (1-2), 15–25. Epub 2020/02/20. doi:10.1007/s11010-020-03688-9
Zhang L., Yang H., Li W. J., Liu Y. H. (2020). Lncrna Malat1 promotes ogd-induced apoptosis of brain microvascular endothelial cells by sponging mir-126 to repress pi3k/akt signaling pathway. Neurochem. Res. 45 (9), 2091–2099. Epub 2020/06/28. doi:10.1007/s11064-020-03071-6
Zhang Q., Long J., Li N., Ma X., Zheng L. (2021). Circ_Clasp2 regulates high glucose-induced dysfunction of human endothelial cells through targeting mir-140-5p/fbxw7 Axis. Front. Pharmacol. 12, 594793. Epub 2021/03/30. doi:10.3389/fphar.2021.594793
Zhang R., Hao Y., Zhang J. (2022). The lncrna dancr promotes development of atherosclerosis by regulating the mir-214-5p/cox20 signaling pathway. Cell. Mol. Biol. Lett. 27 (1), 15. Epub 2022/02/19. doi:10.1186/s11658-022-00310-2
Zhang W., Sui Y. (2020). Circbptf knockdown ameliorates high glucose-induced inflammatory injuries and oxidative stress by targeting the mir-384/lin28b Axis in human umbilical vein endothelial cells. Mol. Cell. Biochem. 471 (1-2), 101–111. Epub 2020/06/12. doi:10.1007/s11010-020-03770-2
Zhang Y., Sun Z., Jia J., Du T., Zhang N., Tang Y., et al. (2021). Overview of histone modification. Adv. Exp. Med. Biol. 1283, 1–16. Epub 2020/11/07. doi:10.1007/978-981-15-8104-5_1
Zhang Y., Yuan D., Yao W., Zhu Q., Liu Y., Huang F., et al. (2016). Hyperglycemia aggravates hepatic ischemia reperfusion injury by inducing chronic oxidative stress and inflammation. Oxidative Med. Cell. Longev. 2016, 3919627. Epub 2016/09/23. doi:10.1155/2016/3919627
Zheng Z., Chen H., Li J., Li T., Zheng B., Zheng Y., et al. (2012). Sirtuin 1-mediated cellular metabolic memory of high glucose via the lkb1/ampk/ros pathway and therapeutic effects of metformin. Diabetes 61 (1), 217–228. Epub 2011/11/30. doi:10.2337/db11-0416
Zhong Q., Kowluru R. A. (2011). Epigenetic changes in mitochondrial superoxide dismutase in the retina and the development of diabetic retinopathy. Diabetes 60 (4), 1304–1313. Epub 2011/03/02. doi:10.2337/db10-0133
Zhong Q., Kowluru R. A. (2013). Epigenetic modification of Sod2 in the development of diabetic retinopathy and in the metabolic memory: Role of histone methylation. Invest. Ophthalmol. Vis. Sci. 54 (1), 244–250. Epub 2012/12/12. doi:10.1167/iovs.12-10854
Zhong Q., Kowluru R. A. (2010). Role of histone acetylation in the development of diabetic retinopathy and the metabolic memory phenomenon. J. Cell. Biochem. 110 (6), 1306–1313. Epub 2010/06/22. doi:10.1002/jcb.22644
Zhou S., Chen H. Z., Wan Y. Z., Zhang Q. J., Wei Y. S., Huang S., et al. (2011). Repression of P66shc expression by Sirt1 contributes to the prevention of hyperglycemia-induced endothelial dysfunction. Circ. Res. 109 (6), 639–648. Epub 2011/07/23. doi:10.1161/circresaha.111.243592
Keywords: glucose metabolism, lipid metabolism, vascular injury, T2D, therapeutic strategy
Citation: Chen X, Shi C, Wang Y, Yu H, Zhang Y, Zhang J, Li P and Gao J (2022) The mechanisms of glycolipid metabolism disorder on vascular injury in type 2 diabetes. Front. Physiol. 13:952445. doi: 10.3389/fphys.2022.952445
Received: 26 May 2022; Accepted: 12 August 2022;
Published: 31 August 2022.
Edited by:
John D Imig, Medical College of Wisconsin, United StatesReviewed by:
Bing Feng, Swiss Federal Institute of Technology Lausanne, SwitzerlandEdgar Zenteno, National Autonomous University of Mexico, Mexico
Copyright © 2022 Chen, Shi, Wang, Yu, Zhang, Zhang, Li and Gao. This is an open-access article distributed under the terms of the Creative Commons Attribution License (CC BY). The use, distribution or reproduction in other forums is permitted, provided the original author(s) and the copyright owner(s) are credited and that the original publication in this journal is cited, in accordance with accepted academic practice. No use, distribution or reproduction is permitted which does not comply with these terms.
*Correspondence: Peifeng Li, cGVpZmxpQHFkdS5lZHUuY24=; Jinning Gao, Z2puLjExMjdAMTYzLmNvbQ==