- 1SAINBIOSE, U1059, Laboratory of Osteoarticular Tissue Biology, INSERM, University of Lyon, Saint-Etienne, France
- 2Rheumatology Department, University Hospital of Saint-Etienne, Saint-Etienne, France
- 3Biology and Pathology Department, University Hospital of Saint-Etienne, Saint-Etienne, France
Purpose: To evaluate whole-body vibration (WBV) osteogenic potential in physically inactive postmenopausal women using high-frequency and combined amplitude stimuli.
Methods: Two-hundred fifty-five physically inactive postmenopausal women (55–75 years) with 10-year major osteoporotic fracture risk (3%–35%) participated in this 18-month study. For the first 12 months, the vibration group experienced progressive 20-min WBV sessions (up to 3 sessions/week) with rest periods (30–60 s) between exercises. Frequencies (30–50 Hz), with low (0.2–0.4 mm) and high (0.6–0.8 mm) amplitude stimuli were delivered via PowerPlate Pro5 platforms producing accelerations of (0.75–7.04 g). The last 6 months for the treatment group were a follow-up period similar to control. Serum bone remodelling markers [C-terminal crosslinked telopeptide of type-1 collagen (CTX), procollagen type-1 N-terminal propeptide (P1NP), bone alkaline phosphatase (BAP) and sclerostin] were measured at fasting. CTX and P1NP were determined by automated chemiluminescence immunoassay, bone alkaline phosphatase (BAP) by automated spectrophotometric immunoassay, and sclerostin by an enzyme-immunoassay. Bone mineral density (BMD) of the whole-body, proximal femur and lumbar vertebrae was measured by dual-energy X-ray absorptiometry (DXA). Bone microarchitecture of the distal non-dominant radius and tibia was measured by high-resolution peripheral quantitative computed tomography (HR-pQCT).
Results: Femoral neck (p = 0.520) and spine BMD (p = 0.444) failed to improve after 12 months of WBV. Bone macro and microstructural parameters were not impacted by WBV, as well as estimated failure load at the distal radius (p = 0.354) and tibia (p = 0.813). As expected, most DXA and HR-pQCT parameters displayed age-related degradation in this postmenopausal population. BAP and CTX increased over time in both groups, with CTX more marginally elevated in the vibration group when comparing baseline changes to month-12 (480.80 pmol/L; p = 0.039) and month-18 (492.78 pmol/L; p = 0.075). However, no differences were found when comparing group concentrations only at month-12 (506.35 pmol/L; p = 0.415) and month-18 (518.33 pmol/L; p = 0.480), indicating differences below the threshold of clinical significance. Overall, HR-pQCT, DXA bone parameters and bone turnover markers remained unaffected.
Conclusion: Combined amplitude and high-frequency training for one year had no ameliorating effect on DXA and HR-pQCT bone parameters in physically inactive postmenopausal women. Serum analysis did not display any significant improvement in formation and resorption markers and also failed to alter sclerostin concentrations between groups.
Introduction
Osteoporosis affects skeletal integrity, whereby bone mineral density (BMD) is decreased in addition to microarchitectural deterioration (Kanis et al., 2019). This results in an increased risk of fractures impacting mobility (Kanis et al., 2019) and quality of life (Rhodes et al., 2000), with repercussions at an individual and societal level worldwide (Rashki Kemmak et al., 2020; Salari et al., 2021). In Europe, fracture rates and treatment costs are projected to surge by 25% (Willers et al., 2022). Recent European statistics have also demonstrated a stark contrast in the numbers of osteoporotic cases between males and females, with females affected nearly four times as much (Willers et al., 2022). This increased risk in women is largely attributed to the effect of menopause (The ESHRE Capri Workshop Group, 2010), and despite advances in prevention, screening and management of this condition, osteoporosis continues to remain a significant challenge worldwide (Salari et al., 2021; Willers et al., 2022). For this reason, females are the primary focus of this study.
Several pharmacological treatments and exercise interventions are relied upon to manage osteoporosis (Beck, 2022; Palacios, 2022). However, the difficulty with medication is finding the appropriate balance between evidence-based medicine and proven treatment strategies (Ragucci and Shrader, 2011; Gregson et al., 2022). This is further complicated when integrating suitable exercise interventions for the management of this condition. Previous studies have highlighted the effectiveness of exercise in the management of osteoporosis (Gupta and March, 2016; Koshy et al., 2022). However, significant consideration is required since exercise can substantially increase fall risk and needs to be adapted to the individual (Gupta and March, 2016; Benedetti et al., 2018). Furthermore, prevention with physical activity interventions has not always yielded positive effects (Rhodes et al., 2000; Borer, 2005; Ma et al., 2013). As a result, modalities such as whole-body vibration (WBV) were proposed to harness the potential of physical activity by combining simplicity, ease of administration and encouraging adherence. Since WBV can be easily adapted into an everyday routine, it has garnered a lot of attention over the years as a potential treatment modality for osteoporosis prevention, particularly in postmenopausal women (Marin-Puyalto et al., 2018). WBV involves the transmission of mechanical stimuli delivered via different vibration platforms (vertical, rotational, or lateral planes) that transfer forces to skeletal segments like other forms of exercise (Marin-Puyalto et al., 2018). The effects of WBV have been extensively investigated (Verschuren et al., 2004; Gusi et al., 2006; Von Stengel et al., 2011a; Von Stengel et al., 2011b; Slatkovska et al., 2011; Verschueren et al., 2011; Lai et al., 2013; Stolzenberg et al., 2013; Slatkovska et al., 2014; Liphardt et al., 2015; de Oliveira et al., 2019) resulting in disparities between studies.
Following seminal studies by Rubin et al. (2004) to evidence the impact of WBV on bone, abundant studies ranging from short to long-duration exposure to WBV have been tested (Gómez-Cabello et al., 2014; Kiel et al., 2015). In the 11-week study published by Gomez-Cabello et al. (2014), bone parameters in a relatively small population were examined using high-amplitude and high-frequency vibration stimuli. Particular focus was on dual-energy X-ray absorptiometry (DXA) derived parameters at the femoral neck and lumbar spine along with added peripheral quantitative computer tomography (pQCT) parameters examining the cortical and trabecular BMD of the radius and tibia. Despite this combination, no conclusive effects on bone were identified. Similarly, studies 6-month in length showed parallels in signal characteristics observed in the 11-week study; however, muscle strength and hip density were also incorporated into the protocol (Verschueren et al., 2004; Verschueren et al., 2011).
Interestingly, two studies of the same 6-month duration explored effects on bone turnover markers (cross-linked C-telopeptide of type I collagen and osteocalcin) alongside DXA outcomes using similar high-frequency and high-amplitude stimuli. Both reached similar conclusions with varying bone and serum results despite utilising similar vibration platforms (Verschuren et al., 2004; Sen et al., 2020). Some studies of much longer duration (12 months or greater) have shifted the focus more towards incorporating advancements in 3D imaging modalities, moving away from the reliance on traditional 2D DXA parameters to draw more in-depth conclusions associated with longitudinal changes in bone (Slatkovska et al., 2011). Studies have experimented with a range of frequencies (Slatkovska et al., 2011) and exposure intervals (Liphardt et al., 2015), using High Resolution Peripheral Computer Tomography (HR-pQCT) (Slatkovska et al., 2011; Liphardt et al., 2015) or novel Magnetic Resonance Imagining (MRI) techniques (Rajapakse et al., 2021) to examine the effects of WBV on bone geometry and microstructural parameters. However, the extensive focus on 3D imaging modalities has exacerbated differences of opinion regarding the efficacy of WBV on bone.
These studies alone highlight several gaps in the literature. Since the osteogenic response to physical activity reduces with age (Rubin et al., 1992), alterations in the length and frequency of sessions could be necessary (Gómez-Cabello et al., 2014). Weekly sessions have varied between one and three, with some varying the training intensity by modifying the signal characteristics and rest periods (Beck and Norling, 2010; Slatkovska et al., 2011; Sen et al., 2020). Despite modifications to signal attributes seen in studies to date, the role of amplitude in WBV bone response is still in question. Physical activity is known to produce a range of amplitudes depending on the type of exercise performed (Deere et al., 2016). Since WBV is a substitute for physical activity, it is essential to replicate this aspect as closely as possible. Frequency variations (high/low), varying amplitudes (<1 g/> 1 g), as well as exposure intervals to the vibration stimuli, have significantly differed between studies and are critical elements of any vibration signal (Prisby et al., 2008). The lack of studies investigating combined amplitude creates a new research opportunity. Physical activity levels prior to intervention were also alluded to as a possible explanation for the lack of observable effect (Rajapakse et al., 2021), which has not been a consideration in studies thus far. Accounting for this might reveal a relationship between WBV and osteogenic potential. Finally, several studies have already explored the response of formation and resorption of bone markers but have not investigated sclerostin, which is known to increase with age and fluctuate based on activity levels (Robling et al., 2008; Amrein et al., 2012). The inclusion of this could capture the subtle responses to combined amplitude stimuli.
In light of the differences outlined above, this study aimed to investigate the role of high-frequency and combined amplitude stimuli over 12 months on physically inactive postmenopausal women. The primary research objective was to investigate changes specific to the femoral neck, while the secondary objective was to measure bone geometry and microarchitectural changes when exposed to such stimuli. In addition, a 6-month follow-up after vibration training was added to observe any sustained osteogenic benefits. It was hypothesised that bone parameters would be improved, with positive osteogenic effects sustained following WBV therapy.
Materials and methods
Ethical approval
Written informed consent for all experiment protocols was obtained from each participant and conformed to the standards set by the latest revision of the Declaration of Helsinki and the committee of human rights protection, southeast, France (N°0908095). ClinicalTrials.gov Identifier (NCT01982214).
Participant information and study design
255 sedentary (< 2 h of physical activity/week at recruitment) postmenopausal women (55–75 years) with absent menses > 1 year, participated in this 18-month non-randomised clinical trial (Supplementary Material S1; Figures 1, 2). Physical activity levels were screened using a questionnaire and further estimated by a computerized self-administered self-assessment questionnaire (QUANTAP, Version 2.0, Université Poincaré de Nancy, France) to obtain an overview of the participants’ activity levels at recruitment (Supplemental Material S1). The first 12 months consisted of the vibration protocol (vibration group) and regular visits (control group). The 6 months thereafter was a follow-up period for both groups. Since difficulties with randomisation were anticipated, participants were given the choice to select their preferred group allocation for the duration of the study. Potential bias resulting from this was effectively addressed since participants were matched according to their FRAX scores for major osteoporotic fracture (MOF) 10 year absolute risk ranging from (3%–35%) to ensure group comparability.
Training program
Only the vibration group carried out this protocol. All participants familiarised themselves with the vibration protocol and equipment beforehand. Twenty minute sessions of light squatting and stretching exercises (max 3 sessions/week, up to 130 sessions total) during the 12-month vibration protocol were performed and recorded in the participants’ logbook. These exercises were for the sole purpose of maintaining the participants’ motivation given the duration of the vibration training sessions. The first session was shorter and interspersed with rest periods, and a fitness instructor constantly ensured the participants’ safety.
The vibration characteristics were: frequency (30–50 Hz), with a combination of low-amplitude (0.2–0.4 mm) and high-amplitude (0.6–0.8 mm) stimuli were delivered using PowerPlate Pro5 airdaptive system (Performance Health Systems, LLC, NorthBrook, IL, United States) to generate an acceleration profile of (0.75–7.04 g). This system delivered vibration stimuli tri-axially (X,Y,Z axis) and possessed a self-adjusting air cushion system that distributed participants’ weight across the platform to mitigate against signal dampening. The chosen vibration amplitudes and frequencies were applicable to elderly people to obtain an acceleration close to 3 g, reproduced by fast walking or osteogenic sports (Vainionpää et al., 2006). Initially, all participants started with low-amplitude stimuli; however, exercise repetition, vibration frequency and amplitude progressively changed throughout the study (Supplementary Material S2). All participants were requested not to modify their regular activity levels, avoiding impact activities/sports throughout the entire study.
Biochemistry
All samples were obtained in the morning following an overnight fast. Serum concentrations of C-terminal crosslinked telopeptide of type-1 collagen (CTX, pmol/L) and procollagen type-1 N-terminal propeptide (P1NP, µg/L) were determined by automated chemiluminescence immunoassay (IDS-iSYS automated analyser, Boldon, United Kingdom), while bone alkaline phosphatase (BAP, µg/L) was measured using an automated spectrophotometric immunoassay (IDS-iSYS automated analyser, Boldon, United Kingdom). Intra-assay CV were < 4.9%, < 3.0%, and < 2%, while inter-assay CV were < 8.8%, < 5.3, and < 9% for CTX, P1NP and BAP respectively. An enzyme-immunoassay (EIA) kit was used to quantify sclerostin levels (SOST, ng/ml) (Quidel Corporation San Diego, CA, United States) with precision CV’s of 3.7%–4.2% and 4.3%–4.8% for within-run and between run respectively.
Dual-energy X-ray absorptiometry
Fat, lean body and total body mass in grams, along with standard BMD measurements at the femoral neck (g/cm2), total hip (g/cm2), and lumbar spine (L1-L4) (g/cm2), were measured using DXA (GE, Lunar iDXA, Milwaukee, WI, United States). Additional femoral neck analyses were performed using 3D-Shaper (Galgo Medical, Barcelona, Spain) to obtain cortical vBMD (mg/cm3) and trabecular vBMD (mg/cm3) of the femur and femoral neck. Lumbar spine trabecular bone score (g/cm2) was attained using TBS iNsight (Medimaps, Geneva, Switzerland). All measurements were performed by the same qualified technician according to manufacturer’s guidelines.
High-resolution peripheral quantitative computed tomography measurements
All microarchitectural bone measurements were obtained using HR-pQCT (Scanco Medical, Bassersdorf, Switzerland) of the non-dominant radius and tibia unless a fracture was reported in the region of interest. Scans were performed by the same qualified operator by positioning the reference line at the endplate of the radius and tibia on an anteroposterior scout view. Using this line, the standard scan region of interest was found automatically with the first slice 9.5 mm and 22.5 mm down to the reference line for the distal radius and tibia respectively. The following settings were used: peak energy, 60 kVp; X-ray tube current, 900 mA; matrix size, 1,536 × 1,536.A slack of 110 CT slices were acquired over a 9 mm length consisting of 110 CT slices with an isotropic voxel size of 82 µm, with an effective dose of 3 µSv in approximately 3 min. Image quality was scored ranging from grade 1 (highest quality) to grade 5 (unacceptable), using manufacturers’ recommendations (Scanco Medical). A preliminary quality grading was made prior to image acquisition, and repeated measurements were made for all scans with insufficient quality (grade 4 or 5). Thus, only scans with quality grade 1–3 (none, minor or moderate motion artefacts) were used for subsequent image analysis. After manual correction of the periosteal and endosteal contours when needed, total vBMD was calculated as the total amount of mineral divided by the total bone volume within the periosteal contour. This volume was then separated into cortical and trabecular compartments, each compartment being analysed separately. Images were analysed using the advanced cortical evaluation protocol provided by the manufacturer (Burghardt et al., 2010).
The following parameters were measured for the radius and tibia and analysed using Image Processing Language (IPL;version-6, Scanco Medical): total BMD (mg HA/cm3), trabecular bone volume fraction (BV/TV, %), trabecular inhomogeneity (Tb.1/N.SD; mm), trabecular vBMD (mg HA/cm3), cortical vBMD (mg HA/cm3), cortical area (Ct.Ar; mm), cortical thickness (Ct.Th; mm), cortical porosity (Ct.Po; %) and cortical perimeter (Ct.Pm; mm). Finite element analysis using the advanced evaluation script (version 1.0, Scanco Medical) evaluated failure load (FL; kN), compartment load distribution (Loadtrab.prox, Loadtrab.dist; %) and average Von Mises stress (StressVM; Mpa) of the cortical and trabecular compartments. The coefficient of variation has been established elsewhere (Vico et al., 2008). The reproducibility of HR-pQCT density measurements ranged from 0.5% to 1.1%. In comparison, reproducibility of the structural parameters (number of trabeculae, trabecular thickness, and cortical thickness) was slightly lower, with coefficients ranging from 0.7% to 4.5%. The reproducibility of the measurement was similar at the distal radius and the distal tibia. Finally, total volume (mm3) and muscle volume fraction (MV/TV, %) of the distal tibia was performed using soft tissue analysis script provided by Scanco Medical (Erlandson et al., 2017).
Statistical analysis
To estimate the effect size, the DXA BMD at the femoral neck was used. An analysis of four cohort studies (Arlot et al., 1997; Pedrazzoni et al., 2003; Gjesdal et al., 2004; Emaus et al., 2006) evaluating average bone loss in postmenopausal women aged between 55 and 75 years estimated the change in BMD over 1 year and found that a loss of 1% per year was observed. Another study evaluating the effects of walking on BMD in postmenopausal women (Martyn-St James and Carroll, 2008) reported an effect of +2% compared to controls. Additionally, another study comparing the effects of walking with those of WBV (Gusi et al., 2006) observed a +2% difference in WBV from walking on BMD. Thus, we wished to demonstrate an effect of +4% with the WBV compared to the control group (a Delta of 0.04). Considering this desired delta, with a standard deviation of 0.10, a power of 80% and an alpha of 5%, the number of subjects required would consist of 100 participants in each group. A total of 240 women was expected to be included, 120 women per group, taking into account those who would be lost at follow-up.
An independent samples t-test was used to assess differences in baseline characteristics and for the selection of appropriate covariates. All quantities were analysed in STATA-17 (StataCorp LP, College Station, Texas, United States) using a linear mixed model with a random intercept and robust standard errors. This model was selected given the multiple timepoints associated with each participant and the ability to handle missing values. Fixed factors were group (2 levels: control, vibration) and month (4 levels: 0,6,12,18), while the random factor was participants (n = 197). Age was treated as a continuous factor. Overall model fit and normality was assessed using quartile-quartile plots of the residuals. All dependant variables during the vibration phase were analysed in the following way: (Baseline vs. month-6 and Baseline vs. month-12). Baseline compared with follow-up used: (Baseline vs. month-18). Finally, to analyse the prolonged effect of vibration (Month-12 vs. Month-18) was compared. Bonferroni correction was applied for all comparisons with results presented as contrasts between groups, and the significance level was set to p < 0.05.
Results
This non-randomised clinical trial matched participants according to their FRAX scores for major osteoporotic fracture (MOF) 10 years-absolute risk ranging from (3%–35%) to ensure group comparability. Statistical analysis was performed using 197 participants (Figure 1) having four timepoints (baseline, 6, 12 and 18 months). For all 197 participants, HR-pQCT image quality and common region were (≤ 3) and (≥ 70%) respectively (Table 1; Figure 1). Participants’ baseline characteristics can be found in (Tables 2, 3). Vibration protocol adherence was good with 110.02 ± 14.25 (mean ± SD) vibration sessions from a maximum of 130 completed by the vibration group. All within groups differences are presented in (Tables 4–7) and significant between group differences are presented in (Table 8).
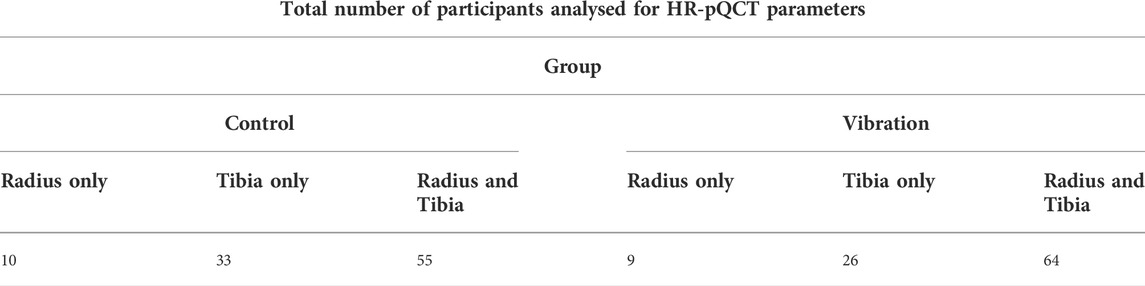
TABLE 1. Breakdown of the total number of participants analysed for HR-pQCT parameters, based on having 4-time points, image quality (≤ 3) and common region (≥ 70%). Total number of participants (n = 197): Control (n = 98), Vibration (n = 99). Common region for tibia (mean ± SD): Control (89.05 ± 7.27) Vibration (88.05 ± 9.06). Common region for radius (mean ± SD): Control (82.00 ± 10.64) Vibration (82.70 ± 12.41).
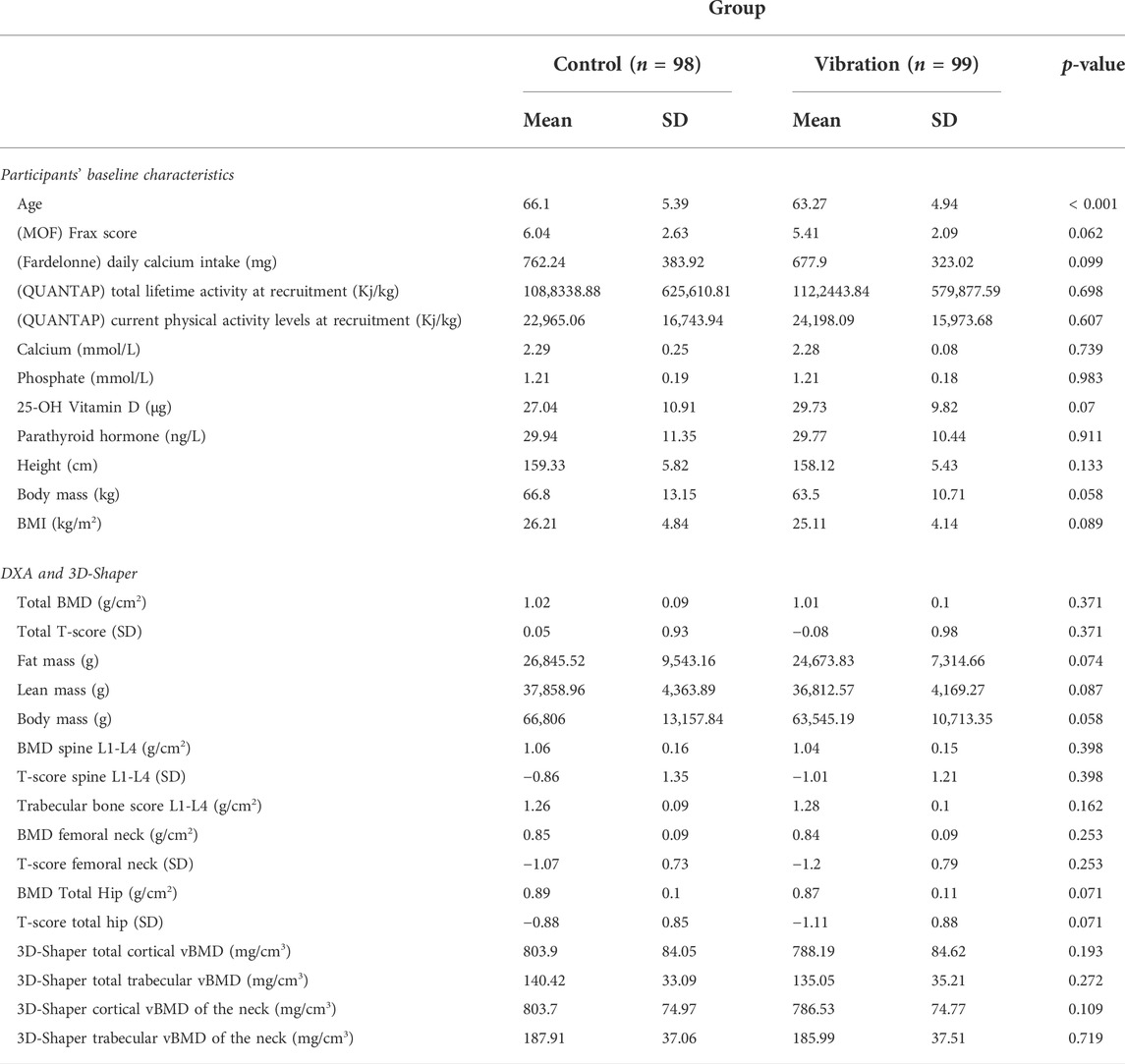
TABLE 2. Participants’ characteristics and DXA parameters at baseline (See Supplementary Material S3) and (Fardellone et al., 1991) for Fardellone self-questionnaire. (See Supplementary Material S1) for lifetime activity questionnaire (QUANTAP). QUANTAP total lifetime activity and current level of physical activity at recruitment were reported in Kilojoules/Kilograms (Kj/Kg).
Dual-energy X-ray absorptiometry and 3D-Shaper
All DXA and 3D-Shaper parameters are presented in (Table 4). Other than a similar and continued decrease over time in all participants, no significant improvement in BMD of the femoral neck, total hip, total cortical and trabecular vBMD of the femur, cortical and trabecular vBMD at the femoral neck, total BMD, bone mass, and BMD and trabecular bone score of the spine (L1-L4) were observed.
A group by month interaction was observed for fat, lean and total body mass (Table 8). Fat mass for the vibration group was significantly lower compared to control, towards the end of the vibration period (−776.06 g, p = 0.043). For lean body mass, a reduction at month-12 compared to baseline produced a difference of (−519.11 g, p = 0.009) between the vibration group compared to control. Total body mass decreased from baseline to month-12 with group differences of (−1,285.72 g, p = 0.003) compared to control. Although not statistically significant, differences from month-18 to baseline demonstrated that these effects were not long lasting.
Tibia high-resolution peripheral quantitative computed tomography
All tibia HR-pQCT parameters are presented in (Table 5). No significant improvement in total BMD compared to control was observed throughout the vibration phase (p = 1.00). No apparent differences could be observed for trabecular volume resulting from vibration exposure. In contrast, BV/TV exhibited a group by month interaction in the vibration group when comparing baseline to month-12 (p = 0.023). However, despite this significant difference between groups, the overall change reflected in BV/TV was negligible (0.0009%, p = 0.050). Trabecular inhomogeneity remained unchanged. No improvement in cortical area or thickness was observed. Apart from a gradual decrease over time no differences in cortical vBMD and perimeter were noted between groups. Finally, cortical porosity continued to increase over time in both the control and training group. Total muscle volume continued to decrease over time and MV/TV showed no overall differences between groups.
Radius high-resolution peripheral quantitative computed tomography
All radius HR-pQCT parameters are presented in (Table 6). No improvement in total bone mineral density was evident. Trabecular volume, BV/TV as well as trabecular inhomogeneity remained unaltered. No significant interactions were observed for any cortical parameters. For cortical area, thickness, perimeter and cortical vBMD no improvement resulting from vibration could be detected. Finally, both groups experienced an increase in cortical porosity levels over time.
Finite element analysis
All finite element parameters for the radius and tibia are presented in (Tables 5, 6). WBV failed to improve finite element parameters in the vibration group. Failure load continued to worsen over time in both the tibia and radius. The ratio of the load supported by the trabecular bone and the load by the whole bone at the distal tibial site continued to decrease (p = 0.016) throughout. In contrast, these changes were not significantly different at the proximal end (p = 0.185). For the radius, both the distal and proximal sites showed reductions over time with values of (p < 0.001) and (p = 0.008), respectively. Cortical Von Mises stress for the tibia and radius moderately decreased over time, however these changes were not statistically significant. Trabecular Von Mises stress on the other hand demonstrated no significant changes over time for the tibia, however, significant decreases in stress loads over time were seen for the radius (p = 0.009).
Serum analysis
All serum markers are presented in (Table 7). Interaction effects between month and group were observed for serum CTX (p = 0.015) (Table 8; Figure 3). Although both groups experienced an increase in CTX concentrations, a more marginal elevation was noted in the vibration group. Compared to baseline, the vibration group at month 12 produced greater differences in CTX concentrations compared to control (480.80 pmol/L; p = 0.039). However, no differences between groups were observed when only comparing groups at month-12 (506.35 pmol/L; p = 0.415) and month-18 (518.33 pmol/L; p = 0.480). No significant differences in sclerostin concentration could be observed. Apart from significant increases over time (p < 0.001), no other differences were observed for BAP. Where P1NP was concerned, no significant alterations were observed.
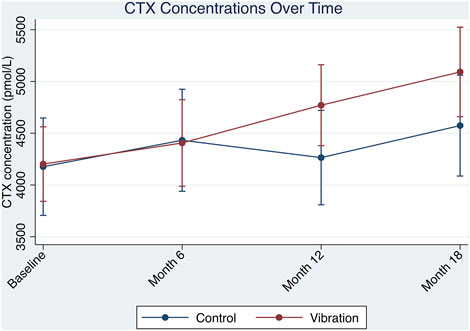
FIGURE 3. Group by month interaction for serum CTX comparing control (n = 98) and vibration (n = 99) groups. Values depicted are estimated marginal means derived from the linear mixed model plotted with 95% CI’s. Vibration period (Baseline to Month-12) in the vibration group and regular visits for the control group (Baseline to Month-12). Follow-up visits for both groups (Month-12–Month-18).
Discussion
This non-randomised clinical trial matched participants according to their FRAX scores for major osteoporotic fracture (MOF) 10 years-absolute risk ranging from (3%–35%) to ensure group comparability investigated the effects of WBV on 197 postmenopausal women over 12 months, with an additional 6-month follow-up to observe post-vibratory outcomes. Treatment responses were measured using DXA, HR-pQCT, and serum analysis. Fat, lean body, and total body mass assessed by DXA very marginally decreased in the vibration group during the vibration phase. No significant differences at the femoral neck or for all other DXA and serum parameters were observed except for an 11% increase in CTX levels in the vibration group, a non-clinically relevant variation which remained present at month-18. Furthermore, no demonstrable benefits from vibration were detected for all bone geometry and microarchitectural parameters measured by HR-pQCT. Finite element analysis demonstrated a continuous decrease in failure load, and disproportionate stress loads were observed for the cortical and trabecular compartments in both groups. Finally, no changes in muscle parameters evaluated by HR-pQCT at the distal tibia were detected.
The primary objective was to assess the effect of WBV on the femoral neck BMD, given that this parameter is a strong predictor of hip and spine fractures in this type of population (Crandall et al., 2021). Some studies have found neutral effects of WBV at the hip and femoral neck (Lau et al., 2011; Ma et al., 2016; Oliveira et al., 2016; Jepsen et al., 2017; Luo et al., 2017) while one demonstrated positive effects (Sitjà-Rabert et al., 2012). Although the objective of previous studies has been on frequency variation and exposure length, none have incorporated combined amplitude in the experimental protocol. Therefore, unique to this study was the incorporation of high and low-amplitude stimuli with an incremental increase throughout the vibration protocol. By further tailoring the signal characteristics that have generally been sufficient to stimulate bone formation (Santos et al., 2017), this protocol was expected to better replicate the role of mechanical stimulation, in particular the ground reaction forces produced during regular jumping and physical activity and to demonstrate its tolerance in this population. However, despite this combination of amplitude and frequency, no discernible benefits were observed at the femoral neck, spine, or HR-pQCT parameters. The continued reduction in failure load with unequal load distributions between cortical and trabecular compartments is consistent with elevated fracture risk (Boutroy et al., 2008) in this population, not significantly negated by vibration therapy.
The results from this study are difficult to compare, given the unique signal characteristics. However, considering studies 12 months in duration, Slatkovska et al. (2011) share several similarities (population size, age range and 20-min training sessions with predominantly European participants) with the current study. Although Slatkovska et al. (2011) mainly focused on frequency variation (90 Hz and 30 Hz), the frequency range in the present study (30 Hz–50 Hz) fell between the two training groups. This is a particular strength as it helps to further expand upon the range of frequencies previously tested while incorporating the application of combined amplitude. However, both Slatkovska et al. (2011) and the present study failed to see any benefits on bone after 12 months of WBV exposure. Another one-year longitudinal study (Liphardt et al., 2015), which incorporated a follow-up period to observe sustained osteogenic benefits of WBV, also failed to detect changes in the bone and observed no improvement in load distribution between groups, which indicates no improvement in fracture risk. Though these studies employed different platforms and signal attributes, the consistency in HR-pQCT results of bone geometry and microarchitecture remains a crucial finding.
The current results can be further contrasted with other studies using the PowerPlate device. Sen et al. (2020) and Verschueren et al. (2004) utilised high-frequency stimuli for 6 months to measure BMD changes at one or more sites (hip, femoral neck, and lumbar region) along with serum bone turnover markers (CTX and osteocalcin). In both studies, the vibration groups demonstrated an improvement in BMD; however, bone turnover marker results were inconsistent. In the present study, no significant benefits were observed at the crucial DXA sites. However, from baseline to the end of the training period, CTX was elevated in both groups with marginally higher concentrations in the vibration group. Nevertheless, when comparing months 12 and 18 alone these differences were not statistically different.
Several explanations could account for the marginal increase in CTX concentrations. Firstly, the two previous studies (Verschueren et al., 2004; Sen et al., 2020) were of much shorter duration, hence CTX would not have changed as it did in this study. Secondly, when taken together, the increased BAP activity with the rising CTX concentrations is indicative of the stimulated bone remodeling process in postmenopausal women (Eastell et al., 2016). Finally, the marginal elevation in CTX concentrations in the vibration group could also be linked with the small reduction in body mass resulting from WBV exposure. The impact of weight loss attributed to dieting and/or exercise on bone turnover markers (CTX) has been previously reported (Eastell and Szulc, 2017).
Sen et al. (2020) and Verschueren et al. (2004) examined the response of WBV on osteocalcin, as a bone formation marker. No group differences were reported by Verschueren et al. (2004) while a decrease was reported by Sen et al. (2020). In the present study, the effects on bone formation were studied using P1NP which was not altered due to vibration training. Elevation in P1NP was observed by Corrie et al. (2015) in a study using a PowerPlate device. However, the age of the participants was considerably higher (79–82 years) than the current study, the training duration was only twelve weeks and focused on both men and women in a small population. It is also important to note that no BMD measurements were taken during this study.
WBV response on bone turnover markers has not been extensively studied, however, the few that have investigated its effects (Russo et al., 2003; Verschueren et al., 2004; Corrie et al., 2015; Kiel et al., 2015) focused mainly on markers of formation and resorption. Even fewer have explored the role of sclerostin in the context of WBV. Sclerostin has emerged over the years as a marker essential to skeletal physiology and homeostasis and is found to be mostly expressed by osteocytes (Poole et al., 2005). It interacts with the Wnt signalling pathway (Baron and Kneissel, 2013) leading to the formation or resorption of bone. Moreover, sclerostin concentrations have been shown to increase or decrease based on the response to mechanical stimuli (Robling et al., 2008) and are also known to increase with age (Amrein et al., 2012). The goal of measuring sclerostin was to evaluate if the signal characteristics in this study could be detected. Since no differences between groups were observed, this further highlights that the effects of combined amplitude stimuli were undetected. However, it does pose the question of whether combined amplitude exposure of longer duration could produce significant group differences.
Previous research has also explored age-related responses to WBV (Slatkovska et al., 2011; Marín-Cascales et al., 2018); however, the relevance within the context of high-frequency and combined amplitude training has not been tested. Moreover, since fracture risk increases substantially beyond age 64 (Arlot et al., 1997; Emaus et al., 2006), this breakpoint provides an opportunity to ascertain dose and bone response associated with age, resulting from WBV exposure. These age-related differences were explored using retrospective statistical analysis by incorporating age groups (≤ 64 and > 64) into the fixed effects of the aforementioned statistical model to further examine whether any effects of WBV could be detected (Supplementary Material S8–S11). This was done to elucidate any preventative effects given that WBV is relied upon more as a preventive treatment modality. Despite such attempts, no significant differences between age groups were noted. Both bone and serum parameters demonstrated no significant improvement, and more importantly, parameters including P1NP, which is a marker sensitive to bone formation (Gillett et al., 2021), exhibited no significant improvement resulting from vibration therapy. As a result, this further substantiates that WBV has neither osteogenic benefits nor negates fracture risk in this type of physically inactive population regardless of the signal characteristics.
The minimal reductions in fat, lean body and total body mass observed towards the end of the vibration protocol could be linked with a few different possibilities. Firstly, the vibration group was aware that they were undergoing treatment, and the possible impact of this awareness cannot be discounted as an influencing factor. As a result, participants could have been influenced in their dietary habits. Nevertheless, aside from this possibility, the results indicate an interesting effect between groups in that the control group saw more significant weight increases over time than the vibration group. The present study is not the only one to report this effect since the impact of vibration on weight has previously been reported by many (Cristi-Montero, 2013). Several hypotheses exist and involve the role of the sympathetic nervous system (Alavinia et al., 2021), osteocalcin and sclerostin (Wang et al., 2021). A recent pilot study also suggested the role of SMP30 on fat mass, given its role in lipid regulation (Pérez-Gómez et al., 2020). Further studies are needed to fully understand the mechanism as it remains largely unknown and poorly understood.
Limitations
To the best of our knowledge, this study was the first to comprehensively investigate the effects of WBV by including an additional 6-month follow-up period to examine bone geometry and microarchitecture, muscle (HR-pQCT distal tibia), serum and DXA with a particular focus on the femoral neck, using high-frequency and combined amplitude training. Despite this, there are several limitations:
1) This study investigated the effects of WBV in a group of Caucasian European postmenopausal women; however, future research will have to explore the effect of menopause on WBV using broader clinical criteria by accounting for the age, stage of menopause, level of exercise and diverse ethnicities.
2) This study was primarily designed to observe the overall effects of WBV on a large physically inactive group and was not specifically tailored to extract age-related responses. However, given the large population, age range, and adherence to the study protocol, a retrospective statistical analysis, showed that 12 months of WBV treatment did not produce any preventative effects specific to the bone.
3) Finally, the exercise profile of all participants at recruitment relied on several questionnaires to account for their physical activity levels. While this provided a fair estimate of both their general and physical activity levels at recruitment, future studies will need to select parameters involving a combination of physiological and questionnaire-based measures to attain a more accurate and comprehensive assessment.
4) In future studies, closer nutritional monitoring between groups along with the use of Magnetic Resonance Imagining (MRI) could help to assess the effects of WBV on weight.
Conclusion
In conclusion, this 18-month non-randomised clinical trial matched participants according to their (MOF) FRAX scores for major osteoporotic fracture and used WBV for 12 months with combined amplitude and high-frequency vibration to improve bone geometry and bone microarchitecture. This study failed to significantly detect an improvement in bone outcomes of physically inactive osteopenic postmenopausal women. Although the 12-month training protocol was well tolerated, DXA BMD measurements of the lumbar spine, femoral neck and hip were not significantly improved compared to control. Moreover HR-pQCT analysis of cortical and trabecular compartments, including finite element analysis did not demonstrate significant improvement resulting from WBV training. Serum markers (P1NP, BAP and sclerostin) also showed no response to mechanical stimuli. A marginal increase in CTX concentrations was observed; however, there was no indication that this could have resulted from vibration exposure. Nevertheless, future studies could expand upon these results by accounting for stratification in age, and stage of menopause to draw further conclusions.
Data availability statement
The original contributions presented in the study are included in the article/Supplementary Material, further inquiries can be directed to the corresponding author.
Ethics statement
The studies involving human participants were reviewed and approved by committee of human rights protection, southeast, France (N°0908095). The patients/participants provided their written informed consent to participate in this study.
Author contributions
PF: data collection and analysis, manuscript writing; MP: experiment setup, data collection and organisation; HL: experiment setup, data collection and organisation; MN: statistical review; CB: processing of biological samples; M-HL: participant recruitment and follow-up; HM: participant recruitment and follow-up; TT: conceptualisation of the experiment, experimental setup, participant recruitment and follow-up; LV: conceptualisation of the experiment, experimental setup, manuscript review and editing. All authors reviewed and approved the final manuscript.
Funding
Translational Clinical Research–INSERM/ DGOS, project N°1208037 (2011).
Acknowledgments
We would like to thank Centre d’Investigation Clinique–CIC 1408, Saint-Etienne University Hospital, Jean-Monnet University, Saint-Etienne for all the methodological and logistical support that was provided. In addition, we would like to extend our gratitude to all the participants and the numerous medical teams and volunteers at Saint-Etienne University hospital. Finally, we also want to thank La Clinique Mutualiste for the fitness studio and Performance Health Systems for providing the PowerPlate devices.
Conflict of interest
The authors declare that the research was conducted in the absence of any commercial or financial relationships that could be construed as a potential conflict of interest.
Publisher’s note
All claims expressed in this article are solely those of the authors and do not necessarily represent those of their affiliated organizations, or those of the publisher, the editors and the reviewers. Any product that may be evaluated in this article, or claim that may be made by its manufacturer, is not guaranteed or endorsed by the publisher.
Supplementary material
The Supplementary Material for this article can be found online at: https://www.frontiersin.org/articles/10.3389/fphys.2022.952140/full#supplementary-material
References
Alavinia S. M., Omidvar M., Craven B. C. (2021). Does whole body vibration therapy assist in reducing fat mass or treating obesity in healthy overweight and obese adults? A systematic review and meta-analyses. Disabil. Rehabil. 43, 1935–1947. doi:10.1080/09638288.2019.1688871
Amrein K., Amrein S., Drexler C., Dimai H. P., Dobnig H., Pfeifer K., et al. (2012). Sclerostin and its association with physical activity, age, gender, body composition, and bone mineral content in healthy adults. J. Clin. Endocrinol. Metab. 97, 148–154. doi:10.1210/jc.2011-2152
Arlot M. E., Sornay-Rendu E., Garnero P., Vey-Marty B., Delmas P. D. (1997). Apparent pre- and postmenopausal bone loss evaluated by DXA at different skeletal sites in women: The OFELY cohort. J. Bone Min. Res. 12, 683–690. doi:10.1359/jbmr.1997.12.4.683
Baron R., Kneissel M. (2013). WNT signaling in bone homeostasis and disease: From human mutations to treatments. Nat. Med. 19, 179–192. doi:10.1038/nm.3074
Beck B. R. (2022). Exercise prescription for osteoporosis: Back to basics. Exerc. Sport Sci. Rev. 50, 57–64. doi:10.1249/JES.0000000000000281
Beck B. R., Norling T. L. (2010). The effect of 8 mos of twice-weekly low- or higher intensity whole body vibration on risk factors for postmenopausal hip fracture. Am. J. Phys. Med. Rehabil. 89, 997–1009. doi:10.1097/PHM.0b013e3181f71063
Benedetti M. G., Furlini G., Zati A., Letizia Mauro G. (2018). The effectiveness of physical exercise on bone density in osteoporotic patients. Biomed. Res. Int. 2018, 4840531. doi:10.1155/2018/4840531
Borer K. T. (2005). Physical activity in the prevention and amelioration of osteoporosis in women : Interaction of mechanical, hormonal and dietary factors. Sports Med. 35, 779–830. doi:10.2165/00007256-200535090-00004
Boutroy S., Rietbergen B. V., Sornay-Rendu E., Munoz F., Bouxsein M. L., Delmas P. D. (2008). Finite element analysis based on in vivo HR-pQCT images of the distal radius is associated with wrist fracture in postmenopausal women. J. Bone Min. Res. 23, 392–399. doi:10.1359/jbmr.071108
Burghardt A. J., Buie H. R., Laib A., Majumdar S., Boyd S. K. (2010). Reproducibility of direct quantitative measures of cortical bone microarchitecture of the distal radius and tibia by HR-pQCT. Bone 47, 519–528. doi:10.1016/j.bone.2010.05.034
Corrie H., Brooke-Wavell K., Mansfield N. J., Cowley A., Morris R., Masud T. (2015). Effects of vertical and side-alternating vibration training on fall risk factors and bone turnover in older people at risk of falls. Age Ageing 44, 115–122. doi:10.1093/ageing/afu136
Crandall C. J., Hunt R. P., LaCroix A. Z., Robbins J. A., Wactawski-Wende J., Johnson K. C., et al. (2021). After the initial fracture in postmenopausal women, where do subsequent fractures occur? EClinicalMedicine 35, 100826. doi:10.1016/j.eclinm.2021.100826
Cristi-Montero C., Cuevas M. J., Collado P. S. (2013). Whole-body vibration training as complement to programs aimed at weight loss. Nutr. Hosp. 28, 1365–1371. doi:10.3305/nh.2013.28.5.6656
de Oliveira L. C., de Oliveira R. G., de Almeida Pires-Oliveira D. A. (2019). Effects of whole-body vibration versus pilates exercise on bone mineral density in postmenopausal women: A randomized and controlled clinical trial. J. Geriatr. Phys. Ther. 42, E23–E31. doi:10.1519/JPT.0000000000000184
Deere K. C., Hannam K., Coulson J., Ireland A., McPhee J. S., Moss C., et al. (2016). Quantifying habitual levels of physical activity according to impact in older people: Accelerometry protocol for the VIBE study. J. Aging Phys. Act. 24, 290–295. doi:10.1123/japa.2015-0066
Eastell R., O’Neill T. W., Hofbauer L. C., Langdahl B., Reid I. R., Gold D. T., et al. (2016). Postmenopausal osteoporosis. Nat. Rev. Dis. Prim. 2, 16069–16116. doi:10.1038/nrdp.2016.69
Eastell R., Szulc P. (2017). Use of bone turnover markers in postmenopausal osteoporosis. Lancet. Diabetes Endocrinol. 5, 908–923. doi:10.1016/S2213-8587(17)30184-5
Emaus N., Berntsen G. K. R., Joakimsen R., Fonnebø V. (2006). Longitudinal changes in forearm bone mineral density in women and men aged 45-84 years: The tromso study, a population-based study. Am. J. Epidemiol. 163, 441–449. doi:10.1093/aje/kwj055
Erlandson M. C., Wong A. K. O., Szabo E., Vilayphiou N., Zulliger M. A., Adachi J. D., et al. (2017). Muscle and myotendinous tissue properties at the distal tibia as assessed by high-resolution peripheral quantitative computed tomography. J. Clin. Densitom. 20, 226–232. doi:10.1016/j.jocd.2016.10.005
Fardellone P., Sebert J. L., Bouraya M., Bonidan O., Leclercq G., Doutrellot C., et al. (1991). Evaluation of the calcium content of diet by frequential self-questionnaire. Rev. Rhum. Mal. Osteoartic. 58, 99–103.
Gillett M. J., Vasikaran S. D., Inderjeeth C. A. (2021). The role of PINP in diagnosis and management of metabolic bone disease. Clin. Biochem. Rev. 42, 3–10. doi:10.33176/AACB-20-0001
Gjesdal C. G., Aanderud S. J., Haga H.-J., Brun J. G., Tell G. S. (2004). Femoral and whole-body bone mineral density in middle-aged and older Norwegian men and women: Suitability of the reference values. Osteoporos. Int. 15, 525–534. doi:10.1007/s00198-003-1573-2
Gómez-Cabello A., González-Agüero A., Morales S., Ara I., Casajús J. A., Vicente-Rodríguez G. (2014). Effects of a short-term whole body vibration intervention on bone mass and structure in elderly people. J. Sci. Med. Sport 17, 160–164. doi:10.1016/j.jsams.2013.04.020
Gregson C. L., Armstrong D. J., Bowden J., Cooper C., Edwards J., Gittoes N. J. L., et al. (2022). UK clinical guideline for the prevention and treatment of osteoporosis. Arch. Osteoporos. 17, 43. doi:10.1007/s11657-017-0324-5
Gupta A., March L. (2016). Treating osteoporosis. Aust. Prescr. 39, 40–46. doi:10.18773/austprescr.2016.028
Gusi N., Raimundo A., Leal A. (2006). Low-frequency vibratory exercise reduces the risk of bone fracture more than walking: A randomized controlled trial. BMC Musculoskelet. Disord. 7, 92. doi:10.1186/1471-2474-7-92
Jepsen D. B., Thomsen K., Hansen S., Jørgensen N. R., Masud T., Ryg J. (2017). Effect of whole-body vibration exercise in preventing falls and fractures: A systematic review and meta-analysis. BMJ Open 7, e018342. doi:10.1136/bmjopen-2017-018342
Kanis J. A., Cooper C., Rizzoli R., Reginster J.-Y. (2019). European guidance for the diagnosis and management of osteoporosis in postmenopausal women. Osteoporos. Int. 30, 3–44. doi:10.1007/s00198-018-4704-5
Kiel D. P., Hannan M. T., Barton B. A., Bouxsein M. L., Sisson E., Lang T., et al. (2015). Low-Magnitude mechanical stimulation to improve bone density in persons of advanced age: A randomized, placebo-controlled trial. J. Bone Min. Res. 30, 1319–1328. doi:10.1002/jbmr.2448
Koshy F. S., George K., Poudel P., Chalasani R., Goonathilake M. R., Waqar S., et al. (2022). Exercise prescription and the minimum dose for bone remodeling needed to prevent osteoporosis in postmenopausal women: A systematic review. Cureus 14, e25993. doi:10.7759/cureus.25993
Lai C.-L., Tseng S.-Y., Chen C.-N., Liao W.-C., Wang C.-H., Lee M.-C., et al. (2013). Effect of 6 months of whole body vibration on lumbar spine bone density in postmenopausal women: A randomized controlled trial. Clin. Interv. Aging 8, 1603–1609. doi:10.2147/CIA.S53591
Lau R. W., Liao L.-R., Yu F., Teo T., Chung R. C., Pang M. Y. (2011). The effects of whole body vibration therapy on bone mineral density and leg muscle strength in older adults: A systematic review and meta-analysis. Clin. Rehabil. 25, 975–988. doi:10.1177/0269215511405078
Liphardt A. M., Schipilow J., Hanley D. A., Boyd S. K. (2015). Bone quality in osteopenic postmenopausal women is not improved after 12 months of whole-body vibration training. Osteoporos. Int. 26, 911–920. doi:10.1007/s00198-014-2995-8
Luo X., Zhang J., Zhang C., He C., Wang P. (2017). The effect of whole-body vibration therapy on bone metabolism, motor function, and anthropometric parameters in women with postmenopausal osteoporosis. Disabil. Rehabil. 39, 2315–2323. doi:10.1080/09638288.2016.1226417
Ma C., Liu A., Sun M., Zhu H., Wu H. (2016). Effect of whole-body vibration on reduction of bone loss and fall prevention in postmenopausal women: A meta-analysis and systematic review. J. Orthop. Surg. Res. 11, 24. doi:10.1186/s13018-016-0357-2
Ma D., Wu L., He Z. (2013). Effects of walking on the preservation of bone mineral density in perimenopausal and postmenopausal women: A systematic review and meta-analysis. Menopause 20, 1216–1226. doi:10.1097/GME.0000000000000100
Marín-Cascales E., Alcaraz P. E., Ramos-Campo D. J., Martinez-Rodriguez A., Chung L. H., Rubio-Arias J. Á. (2018). Whole-body vibration training and bone health in postmenopausal women: A systematic review and meta-analysis. Medicine 97, e11918. doi:10.1097/MD.0000000000011918
Marin-Puyalto J., Gomez-Cabello A., Gonzalez-Agüero A., Gomez-Bruton A., Matute-Llorente A., Casajús J. A., et al. (2018). Is vibration training good for your bones? An overview of systematic reviews. Biomed. Res. Int. 2018, e5178284. doi:10.1155/2018/5178284
Martyn-St James M., Carroll S. (2008). Meta-analysis of walking for preservation of bone mineral density in postmenopausal women. Bone 43, 521–531. doi:10.1016/j.bone.2008.05.012
Oliveira L. C., Oliveira R. G., Pires-Oliveira D. A. A. (2016). Effects of whole body vibration on bone mineral density in postmenopausal women: A systematic review and meta-analysis. Osteoporos. Int. 27, 2913–2933. doi:10.1007/s00198-016-3618-3
Palacios S. (2022). Medical treatment of osteoporosis. Climacteric. 25, 43–49. doi:10.1080/13697137.2021.1951697
Pedrazzoni M., Girasole G., Bertoldo F., Bianchi G., Cepollaro C., Del Puente A., et al. (2003). Definition of a population-specific DXA reference standard in Italian women: The densitometric Italian normative study (DINS). Osteoporos. Int. 14, 978–982. doi:10.1007/s00198-003-1521-1
Pérez-Gómez J., Adsuar J. C., García-Gordillo M. Á., Muñoz P., Romo L., Maynar M., et al. (2020). Twelve weeks of whole body vibration training improve regucalcin, body composition and physical fitness in postmenopausal women: A pilot study. Int. J. Environ. Res. Public Health 17, 3940. doi:10.3390/ijerph17113940
Poole K. E. S., van Bezooijen R. L., Loveridge N., Hamersma H., Papapoulos S. E., Löwik C. W., et al. (2005). Sclerostin is a delayed secreted product of osteocytes that inhibits bone formation. FASEB J. 19, 1842–1844. doi:10.1096/fj.05-4221fje
Prisby R. D., Lafage-Proust M.-H., Malaval L., Belli A., Vico L. (2008). Effects of whole body vibration on the skeleton and other organ systems in man and animal models: What we know and what we need to know. Ageing Res. Rev. 7, 319–329. doi:10.1016/j.arr.2008.07.004
Ragucci K. R., Shrader S. P. (2011). Osteoporosis treatment: An evidence-based approach. J. Gerontol. Nurs. 37, 17–22. doi:10.3928/00989134-20110602-02
Rajapakse C. S., Johncola A. J., Batzdorf A. S., Jones B. C., Al Mukaddam M., Sexton K., et al. (2021). Effect of low-intensity vibration on bone strength, microstructure, and adiposity in pre-osteoporotic postmenopausal women: A randomized placebo-controlled trial. J. Bone Min. Res. 36, 673–684. doi:10.1002/jbmr.4229
Rashki Kemmak A., Rezapour A., Jahangiri R., Nikjoo S., Farabi H., Soleimanpour S. (2020). Economic burden of osteoporosis in the world: A systematic review. Med. J. Islam. Repub. Iran. 34, 154. doi:10.34171/mjiri.34.154
Rhodes E., Martin A., Taunton J., Donnelly M., Warren J., Elliot J. (2000). Effects of one year of resistance training on the relation between muscular strength and bone density in elderly women. Br. J. Sports Med. 34, 18–22. doi:10.1136/bjsm.34.1.18
Robling A. G., Niziolek P. J., Baldridge L. A., Condon K. W., Allen M. R., Alam I., et al. (2008). Mechanical stimulation of bone in vivo reduces osteocyte expression of sost/sclerostin. J. Biol. Chem. 283, 5866–5875. doi:10.1074/jbc.M705092200
Rubin C., Recker R., Cullen D., Ryaby J., McCabe J., McLeod K. (2004). Prevention of postmenopausal bone loss by a low-magnitude, high-frequency mechanical stimuli: A clinical trial assessing compliance, efficacy, and safety. J. Bone Min. Res. 19, 343–351. doi:10.1359/JBMR.0301251
Rubin C. T., Bain S. D., McLeod K. J. (1992). Suppression of the osteogenic response in the aging skeleton. Calcif. Tissue Int. 50, 306–313. doi:10.1007/BF00301627
Russo C. R., Lauretani F., Bandinelli S., Bartali B., Cavazzini C., Guralnik J. M., et al. (2003). High-frequency vibration training increases muscle power in postmenopausal women. Arch. Phys. Med. Rehabil. 84, 1854–1857. doi:10.1016/S0003-9993(03)00357-5
Salari N., Ghasemi H., Mohammadi L., Behzadi M., Rabieenia E., Shohaimi S., et al. (2021). The global prevalence of osteoporosis in the world: A comprehensive systematic review and meta-analysis. J. Orthop. Surg. Res. 16, 609. doi:10.1186/s13018-021-02772-0
Santos L., Elliott-Sale K. J., Sale C. (2017). Exercise and bone health across the lifespan. Biogerontology 18, 931–946. doi:10.1007/s10522-017-9732-6
Sen E. I., Esmaeilzadeh S., Eskiyurt N. (2020). Effects of whole-body vibration and high impact exercises on the bone metabolism and functional mobility in postmenopausal women. J. Bone Min. Metab. 38, 392–404. doi:10.1007/s00774-019-01072-2
Sitjà-Rabert M., Rigau D., Fort Vanmeerghaeghe A., Romero-Rodríguez D., Bonastre Subirana M., Bonfill X. (2012). Efficacy of whole body vibration exercise in older people: A systematic review. Disabil. Rehabil. 34, 883–893. doi:10.3109/09638288.2011.626486
Slatkovska L., Alibhai S. M. H., Beyene J., Hu H., Demaras A., Cheung A. M. (2011). Effect of 12 months of whole-body vibration therapy on bone density and structure in postmenopausal women: A randomized trial. Ann. Intern. Med. 155, 668–679. doi:10.7326/0003-4819-155-10-201111150-00005
Slatkovska L., Beyene J., Alibhai S. M. H., Wong Q., Sohail Q. Z., Cheung A. M. (2014). Effect of whole-body vibration on calcaneal quantitative ultrasound measurements in postmenopausal women: A randomized controlled trial. Calcif. Tissue Int. 95, 547–556. doi:10.1007/s00223-014-9920-1
Stolzenberg N., Belavý D. L., Beller G., Armbrecht G., Semler J., Felsenberg D. (2013). Bone strength and density via pQCT in post-menopausal osteopenic women after 9 months resistive exercise with whole body vibration or proprioceptive exercise. J. Musculoskelet. Neuronal Interact. 13, 66–76.
The ESHRE Capri Workshop Group (2010). Bone fractures after menopause. Hum. Reprod. Update 16, 761–773. doi:10.1093/humupd/dmq008
Vainionpää A., Korpelainen R., Vihriälä E., Rinta–Paavola A., Leppäluoto J., Jämsä T., et al. (2006). Intensity of exercise is associated with bone density change in premenopausal women. Osteoporos. Int. 17, 455–463. doi:10.1007/s00198-005-0005-x
Verschueren S. M. P., Bogaerts A., Delecluse C., Claessens A. L., Haentjens P., Vanderschueren D., et al. (2011). The effects of whole-body vibration training and vitamin D supplementation on muscle strength, muscle mass, and bone density in institutionalized elderly women: A 6-month randomized, controlled trial. J. Bone Min. Res. 26, 42–49. doi:10.1002/jbmr.181
Verschueren S. M. P., Roelants M., Delecluse C., Swinnen S., Vanderschueren D., Boonen S. (2004). Effect of 6-month whole body vibration training on hip density, muscle strength, and postural control in postmenopausal women: A randomized controlled pilot study. J. Bone Min. Res. 19, 352–359. doi:10.1359/JBMR.0301245
Vico L., Zouch M., Amirouche A., Frère D., Laroche N., Koller B., et al. (2008). High-resolution pQCT analysis at the distal radius and tibia discriminates patients with recent wrist and femoral neck fractures. J. Bone Min. Res. 23, 1741–1750. doi:10.1359/jbmr.080704
Von Stengel S., Kemmler W., Bebenek M., Engelke K., Kalender W. A. (2011a). Effects of whole-body vibration training on different devices on bone mineral density. Med. Sci. Sports Exerc. 43, 1071–1079. doi:10.1249/MSS.0b013e318202f3d3
Von Stengel S., Kemmler W., Engelke K., Kalender W. A. (2011b). Effects of whole body vibration on bone mineral density and falls: Results of the randomized controlled ELVIS study with postmenopausal women. Osteoporos. Int. 22, 317–325. doi:10.1007/s00198-010-1215-4
Wang J. S., Mazur C. M., Wein M. N. (2021). Sclerostin and osteocalcin: Candidate bone-produced hormones. Front. Endocrinol. 12, 584147. doi:10.3389/fendo.2021.584147
Keywords: whole-body vibration, age-related bone loss, fracture risk, dose-response, postmenopausal women
Citation: Fernandez P, Pasqualini M, Locrelle H, Normand M, Bonneau C, Lafage Proust M-H, Marotte H, Thomas T and Vico L (2022) The effects of combined amplitude and high-frequency vibration on physically inactive osteopenic postmenopausal women. Front. Physiol. 13:952140. doi: 10.3389/fphys.2022.952140
Received: 24 May 2022; Accepted: 01 August 2022;
Published: 07 September 2022.
Edited by:
Esther Ubago-Guisado, Andalusian School of Public Health, SpainReviewed by:
Dimitris Vlachopoulos, University of Exeter, United KingdomRicardo Ribeiro Agostinete, São Paulo State University, Brazil
Copyright © 2022 Fernandez, Pasqualini, Locrelle, Normand, Bonneau, Lafage Proust, Marotte, Thomas and Vico. This is an open-access article distributed under the terms of the Creative Commons Attribution License (CC BY). The use, distribution or reproduction in other forums is permitted, provided the original author(s) and the copyright owner(s) are credited and that the original publication in this journal is cited, in accordance with accepted academic practice. No use, distribution or reproduction is permitted which does not comply with these terms.
*Correspondence: Peter Fernandez, cGV0ZXIuZmVybmFuZGV6QGtjbC5hYy51aw==