- 1Renal Division, Department of Medicine, Universidade Federal de SP, São Paulo, Brazil
- 2Postgraduate Program of Health and Environment, Universidade Metropolitana de Santos, Santos, Brazil
Sepsis contributes to the high prevalence of acute kidney injury (AKI), which mainly occurs in hospitalized patients. The delay in AKI detection is a risk factor for death and chronicity; thus, early diagnosis is essential for initiating proper treatment strategies. Although serum creatinine is used as biomarker, it is increased in plasma serum creatinine only at late stages of AKI. MicroRNAs (miRNAs), a class of noncoding RNAs responsible for gene regulation, can be found in biological fluids within vesicles such as exosomes and may be promising tools for the early detection of AKI. We aimed to identify potential blood miRNAs that can be used as early biomarkers of sepsis-induced AKI in rats. Adult male Wistar rats received a single dose of lipopolysaccharide (LPS). The earliest significant increase in serum creatinine was detected 4 h after LPS administration. To evaluate whether miRNAs could act as early biomarkers, blood samples were collected before and 2 h after LPS infusion. Serum NGAL levels were used as a comparative marker. Serum miRNAs were derived from exosomes, and their expression were evaluated by the PCR array. miR-181a-5p and miR-23b-3p showed higher expression in LPS-treated rats than in the control animals (p < 0.05). Bioinformatics analysis showed that both miRNAs target molecules associated with transcription factors that regulate genes related to proinflammatory cytokines. Considering that LPS activates transcription factors that lead to the production of proinflammatory cytokines, possible premature changes in the serum levels of miR-181a-5p and miR-23b-3p may be used to identify sepsis-induced AKI earlier.
1 Introduction
Acute kidney injury (AKI) is a clinical syndrome that occurs in approximately one-third of hospitalized patients and is associated with high morbidity and mortality (Bellomo et al., 2004). Currently, sepsis is the most common cause of AKI in hospitalized patients. Septic patients who develop AKI have a worse prognosis and an increased risk of chronic kidney disease (CKD) and death (Schrier and Wang, 2004; Lo et al., 2009; Levey and James, 2017). The pathophysiology of sepsis-induced AKI can occur through hemodynamic and nonhemodynamic mechanisms; intrarenal vasoconstriction, altered blood flow distribution, tissue toxicity and ischemic changes are the most frequent mechanisms (Ma et al., 2019).
AKI is diagnosed by decreased urine output and increased serum creatinine levels (Khwaja, 2012); however, serum creatinine, which is currently the most frequently used marker of the glomerular filtration rate (GFR), can be influenced by other variables that are unrelated to renal damage, such as age, race, nutritional status, muscle mass, enteral/parenteral diets, catabolic status and volume status (Thomas et al., 2015). In addition, the increase in serum creatinine is detectable when renal damage is at a more advanced stage and there is greater impairment of renal function, which in turn makes recovery more difficult. Therefore, it is important to find new, more sensitive and earlier markers of renal injury, which would allow real-time follow-up and predict the risk of developing AKI and/or the risk of progression to more advanced stages.
In recent years, several biomarkers have been considered to detect AKI at an early stage. Among them, the 25 kDa protein neutrophil gelatinase-associated lipocalin (NGAL) stands out. In fact, plasma NGAL concentrations are increased in sepsis (Mårtensson et al., 2010); however, changes in NGAL levels are not exclusive to renal injury and can be high in acute infectious conditions, such as pancreatitis, heart failure and cancer, even with no signs of AKI (Hjortrup et al., 2013).
Thus, microRNAs (miRNAs), a class of small, noncoding RNAs, stand out as potential biomarkers for the early diagnosis of AKI. miRNAs are single-stranded RNAs consisting of approximately 22 nucleotides (Ha and Kim, 2014) that can be totally or partially complementary to the 3′ untranslated region (3′UTR) of target messenger RNAs (Bhatt et al., 2016). Through this binding, miRNAs regulate gene expression by degrading or impeding target mRNA translation. Thus, miRNAs suppress the translation of mRNAs, reducing protein synthesis. In addition, because of its short length, a single miRNA is able to regulate the expression of many mRNAs (Simpson et al., 2016).
The recognition of miRNAs as key factors in cellular physiology and pathophysiology is well established. miRNAs are secreted by cells through microvesicles, including exosomes, and they remain stable in body fluids such as blood, saliva, urine, and feces. (Hu et al., 2010; Gallo et al., 2012; Lv et al., 2013). Several miRNAs have been previously associated with the pathophysiology of AKI of many etiologies (Bhatt et al., 2016; Fan et al., 2016; Liu et al., 2016; Zou and Zhang, 2018), including septic AKI. Because sepsis-induced AKI can alter the expression levels of specific miRNAs, these molecules may potentially be effective tools for the early detection of sepsis-induced renal injury; however, to our knowledge, there are no studies in the literature analyzing the early miRNA profile in sepsis. In the present study, we developed a rodent model of sepsis-induced AKI to identify early miRNAs that were differentially expressed in the serum exosomes of septic animals.
2 Materials and methods
2.1 Rodent model of sepsis-induced acute kidney injury and serum creatinine analysis
All experimental procedures were approved by the Ethics in Research Committee of the Federal University of Sao Paulo (CEUA-UNIFESP #3083130317). Male Wistar rats weighing 150–200 g were used to establish the sepsis-induced AKI model. Animals were purchased from the animal facility of the Federal University of Sao Paulo, Brazil and were housed in collective cages (5 animals/cage) at room temperature with a 12 h light/dark cycle and free access to standard food and tap water. The basal parameters were obtained from blood sampled by venipuncture of the retro-orbital sinus under sedation. After 15–21 days, the animals received 7.5 mg/kg LPS from Escherichia coli (strain 0111:B4) intraperitoneally (i.p.). Pilot experiments were performed to determine the ideal dose of LPS that was enough to induce AKI, and 7.5 mg/kg was determined to the most suitable dose.
First, the animals were divided into 4 groups containing six animals in each group to determine the kinetics of the increases in serum creatinine. Animals received 7.5 mg/kg LPS, and blood samples were collected at different times (2 hr, 4 hr, 6 hr, and 8 h) after LPS administration. After this experiment, another group of animals (n=5) received LPS (7.5 mg/kg). The animals were anesthetized with xylazine/ketamine (5 mg/kg/75 mg/kg), and blood samples were collected before (basal) and 2 h after LPS administration. Animals were euthanized by anesthetic overdose. All blood samples were immediately centrifuged, and the serum was stored at -80 °C until use.
Serum creatinine concentrations were determined by Jaffe’s method. Serum concentrations of NGAL were measured using the rat NGAL ELISA kit (BioPorto, Denmark) according to the manufacturer’s instructions.
2.2 Exosome-derived RNA extraction, cDNA synthesis and preamplification of microRNAs
Total RNA, including miRNAs, was isolated from serum exosomes using the commercial exoRNeasy serum plasma midi kit (Qiagen, Germany) according to the manufacturer’s instructions. Exogenous cel-miR-39-3p (Qiagen) was added to the samples to measure the RNA isolation efficiency, as determined by the manufacturer.
The complementary DNA (cDNA) was then synthesized from 100 ng of total RNA by using the miScript® II RT kit (Qiagen), and miRNA preamplification was performed using the miScript® PreAmp PCR kit (Qiagen) according to the manufacturer’s instructions.
2.3 RT-qPCR array to analyze miRNA expression
The expression levels of each miRNA were determined by RT-qPCR in 5 serum samples from control (basal) animals and 5 serum samples from animals 2 h after LPS administration. PCR arrays were performed using the QuantiStudio 7 system (Life Technologies, EUA) according to the manufacturer’s instructions. The expression levels of the miRNAs were normalized to the cel-miRNA-39-3p, SNORD61, SNORD68, SNORD95, SNORD96A and RNU6-2 controls using Data Analysis Center software (Qiagen).
2.4 Target genes and pathway analysis of the dysregulated microRNAs
The miRNA target gene databases miRDB (http://mirdb.org/) and TargetScan (http://www.targetscan.org/) were used to predict the target genes of the differentially expressed miRNAs. miRDB is an online bioinformatics tool for predicting molecular targets of microRNAs (Wong and Wang, 2014). TargetScan is another online bioinformatics tool for predicting biological targets of miRNAs that searches for the presence of conserved sites that can bind with the seed region of each miRNA (Lewis et al., 2005).
The identified target gene set and the Enrichr database (http://amp.pharm.mssm.edu/Enrichr/) were used to analyze the biological process, molecular function, cellular component and pathways that were significantly enriched by the target genes. This online software integrates several databases and, from the target genes listed, is able to identify enriched transcription factors associated with these genes (Chen et al., 2013).
2.5 Analysis of exosome-enriched extracellular vesicles
The exosome size and concentration were determined using a Malvern Nanosight Tracking Analysis (NTA) system (NS300) (Worcestershire, United Kingdom). All samples were diluted according to the limit capacity of the equipment and the particle concentration (particles/mL) was calculated.
2.6 Statistical analysis
The paired t-test was used to compare mean creatinine levels between the LPS-treated animals and the respective basal values. The Shapiro-Wilk test was used to assess the distribution of NGAL, which did not show a normal distribution. Therefore, the Wilcoxon test was used to compare the NGAL levels in the serum of the animals 2 h after LPS injection compared to basal levels. The data were considered statistically significant when p < 0.05.
3 Results
3.1 Characterization of the rodent sepsis-induced AKI model
Results are presented as mean±standard deviation. High lethality was observed mainly after more prolonged period after LPS administration. One animal died after 2 h, and 4 animals died after 6 and 8 h. As shown in Figure 1, there was an increase in serum creatinine 2 h after LPS administration, but the difference was not significant. Serum creatinine was significantly increased at 4 h, demonstrating that the detection of renal dysfunction by serum creatinine levels was possible at 4 h after LPS administration (Figure 1).
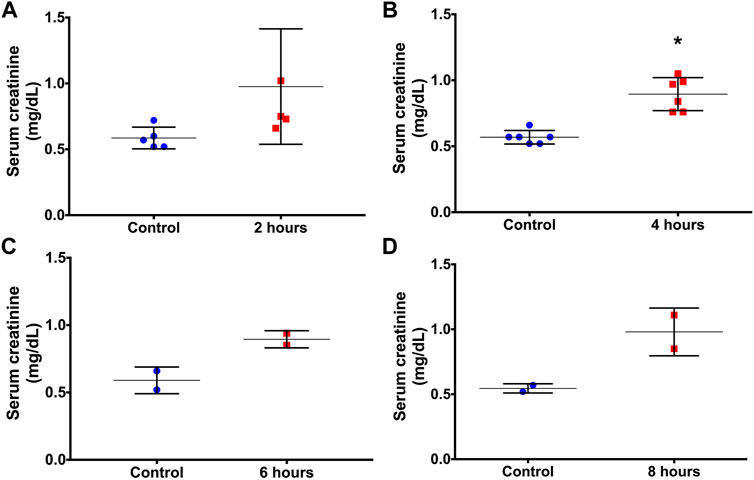
FIGURE 1. Serum creatinine concentrations before (controls) and at 2 (A), 4 (B), 6 (C) and 8 (D) hours after LPS administration. *p < 0.05 vs the control (paired t-test).
3.2 Sepsis-induced AKI induces the release of small exosomes in the serum
Initially, we examined whether LPS stimulation interfered with the release of exosomes. The representative image of size distribution and particle concentration of exosomes between two groups is shown in Figure 2A. Increased exosome release was observed in LPS-stimulated animals compared to basal control (p=0.005; Figure 2B). In addition, a significantly smaller exosome size was observed in the LPS treatment group than in the control group (p=0.036; Figure 2C). These data indicate that LPS influences both the release and size of circulating extracellular vesicles in rats.
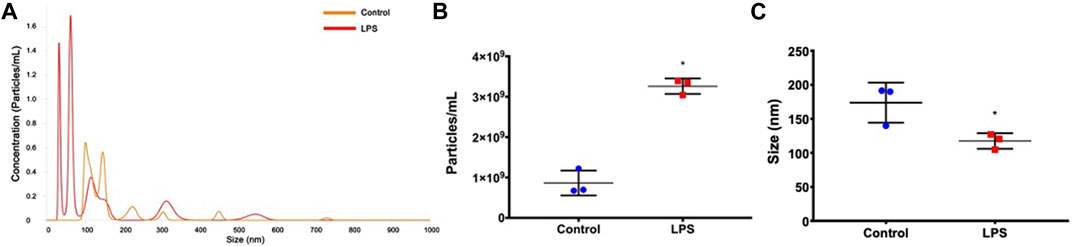
FIGURE 2. Exosome characterization. (A) Histogram representing the profile of nanoparticle size by nanoparticle tracking analysis. (B) Exosome concentration. (C) Exosome size. *p < 0.05 vs the controls (paired t-test).
3.3 Analysis of miRNA profiles
To identify an earlier biomarker of sepsis-induced AKI than creatinine, the expression profile of miRNAs in samples collected 2 h after LPS administration, which was an earlier time than that needed to detect serum creatinine elevation, was evaluated.
The expression heatmap of the miRNAs identified in exosomes isolated from the serum of control or LPS-treated animals is shown in Figure 3. The PCR array results showed that of the 84 miRNAs identified, 40 had increased expression and 4 had decreased expression (Supplementary Table S1) compared to basal control levels. Only 2 upregulated miRNAs were statistically significant, miR-181a-5p and miR-23b-3p, and these miRNAs exhibited increased expression after LPS treatment compared to those of controls (p=0.015 and p=0.035, respectively; Figure 4). Downregulated miRNAs were not statistically significant.
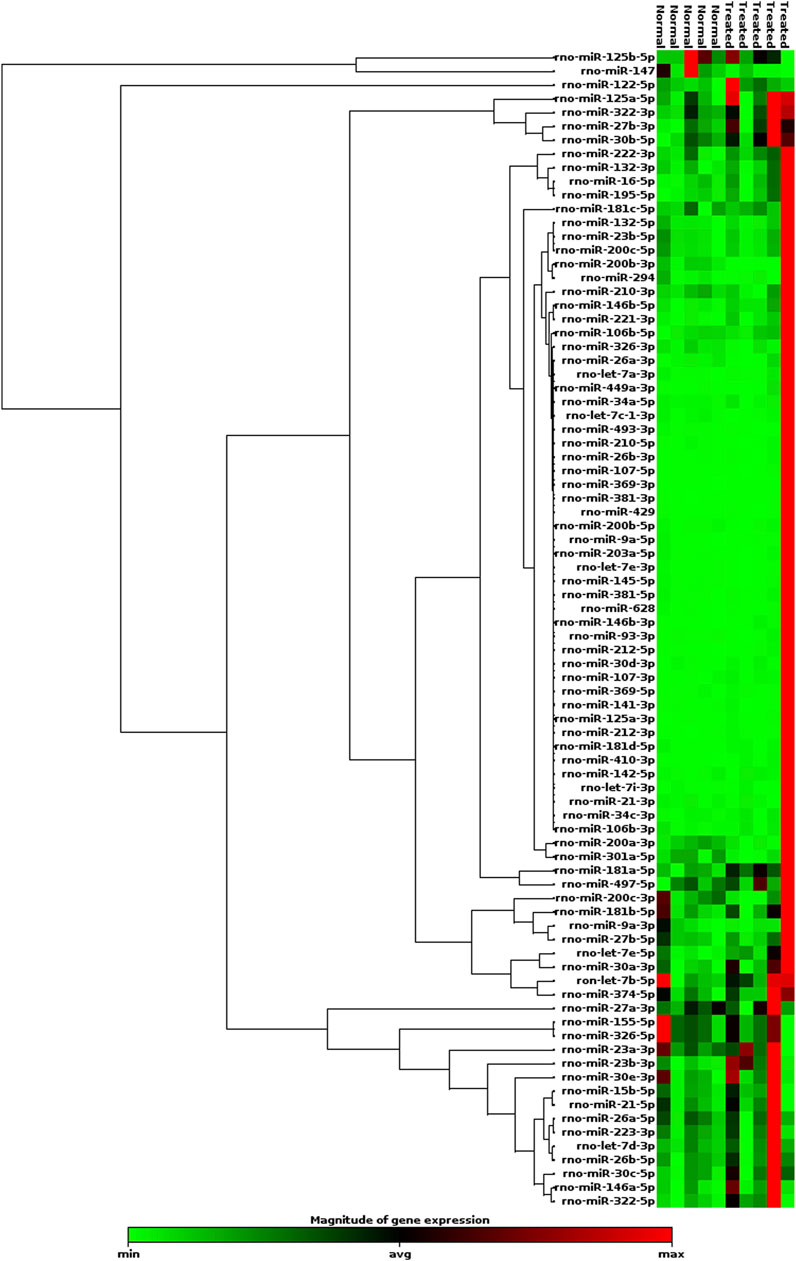
FIGURE 3. Heatmap of the expression of 84 miRNAs evaluated by RT-qPCR array. LPS (n=5); Control (n=5). Red indicates high relative expression, and green denotes low relative expression.
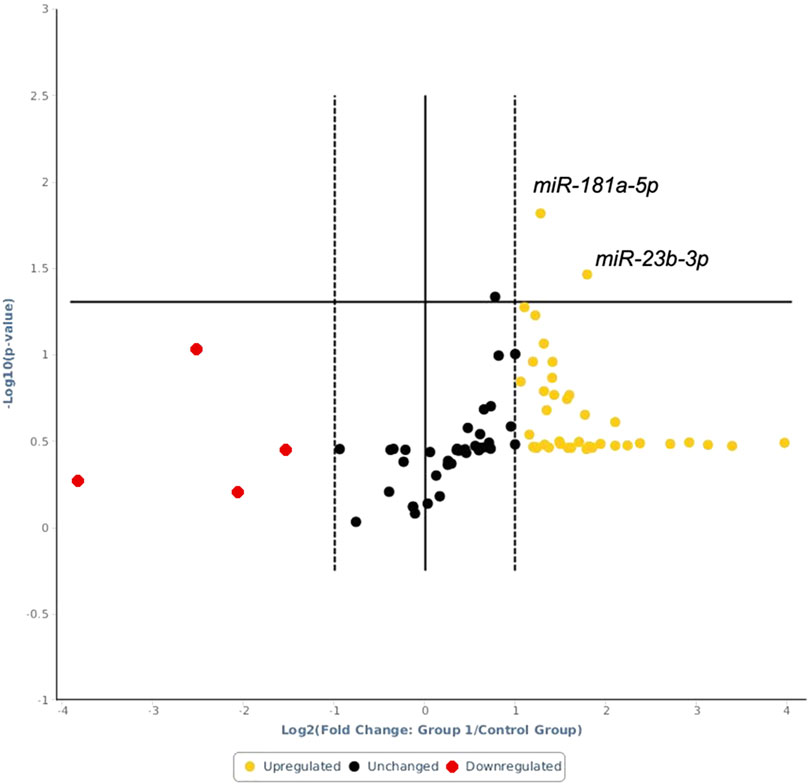
FIGURE 4. Volcano plot of the analyzed miRNAs. Above the horizontal line are the differentially expressed miRNAs with statistical significance in LPS-treated animals relative to the controls. The x-axis shows log 2 (fold change) changes between the LPS-treated animals and the control animals, while the y-axis shows the log-10 of the p value.
3.4 Analysis of miR-181a-5p target genes
Using the miRDB and TargetScan bioinformatics tools, we verified the target genes of miR-181a-5p. Of these target genes, we selected the 10 targets with potential involvement in the pathophysiology of AKI, as shown in Supplementary Table S1. We found that only the ZNF594 gene was identified as a target gene of miR-181a-5p by the two tools. This gene encodes a “zinc finger” nuclear protein that is capable of binding to DNA and is related to mechanisms that control gene transcription (Jen and Wang, 2016).
To better understand the biological role of miR-181a-5p, we used the Enricher platform. As shown in Supplementary Table S2, according to this tool, miR-181a-5p is associated with many physiological processes, including the regulation of transcription, regulation of macromolecule synthesis and regulation of gene expression. Analysis by Enricher demonstrated that miR-181a-5p mediates these mechanisms by interfering with DNA dynamics, as shown in Supplementary Table S3, and is associated with several organelle functions (Supplementary Table S4) and pathophysiological processes, including prostate cancer (Supplementary Table S5). These results suggest that miR-181a-5p has several cellular functions, from gene transcription to vesicle formation.
3.5 Analysis of miR-23b-3p target genes
miR-23b-3p and the top 10 target genes are shown in Supplementary Table S1. The two target genes AUH and FAM234B were identified by the two tools used in this investigation. The Enricher platform identified the biological processes (Supplementary Table S2), molecular functions (Supplementary Table S3), cell components (Supplementary Table S4) and pathways (Supplementary Table S5) associated with miR-23b-3p. Among the many biological processes regulated by miR-23b-3p, the cellular response to drugs (Supplementary Table S2) is of interest since it could have an impact on the results obtained in this study. This activity may be related to the role of miR-23b-3p in upregulating 3′,5′-cyclic AMP and cyclic 3′,5′-nucleotide phosphodiesterase activity (Supplementary Table S3).
3.6 Serum NGAL is increased in the rodent sepsis-induced AKI model
As a possible tool to validate the results obtained, we used the NGAL serum concentration, which was measured in samples of the animals 2 h after LPS injection to verify whether this marker was also altered. We observed that NGAL showed a statistically significant increase (p=0.018) in serum samples from animals 2 h after LPS injection compared to those of the controls (Figure 5).
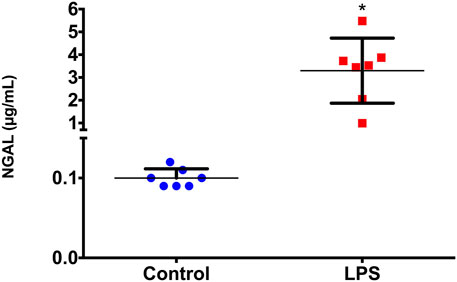
FIGURE 5. Serum NGAL concentrations before (basal) and 2 h after LPS administration. *p < 0.05 vs basal levels (Wilcoxon test).
4 Discussion
Sepsis is one of the most common causes of AKI in clinical practice, is characterized by very high mortality, and mainly occurs in hospitalized patients. The use of early therapeutic strategies, such as dialysis, may help reduce the morbidity and mortality caused by the clinical consequences of sepsis-induced AKI. Identifying the molecules and pathways involved in sepsis-induced AKI is extremely important to better understand the development of this disease, as well as to identify sensible biomarkers capable of predicting the decline in renal function at an earlier stage and, consequently, enabling appropriate therapeutic strategies at the most opportune moments. The identification of urinary markers can be difficult because of the reduced urine output that is typical of most forms of AKI. In addition, several studies have identified miRNAs associated with sepsis-induced AKI (Leelahavanichkul et al., 2015; Colbert et al., 2017; Fu et al., 2017; Ge et al., 2017; Chen Y. et al., 2018; Zheng et al., 2018; Yongjun et al., 2019); however, most of these studies identified tissue miRNAs that would make them unlikely to be AKI biomarkers in clinical practice. miRNAs transported by extracellular vesicles present in the serum are easily obtained with minimal invasiveness and can therefore be used as biomarkers of sepsis-induced AKI. In the present study, two differentially expressed miRNAs were isolated from circulating extracellular vesicles and identified in this sepsis-induced AKI model: miR-181a-5p and miR-23b-3p. Both miRNAs were upregulated before the increase in serum creatinine and therefore at an earlier stage of sepsis-induced AKI development.
miR-181a-5p has been reported to be involved in many pathophysiological mechanisms associated with different types of cancer, (Yu C. et al., 2019; Yu J. et al., 2019; Mao et al., 2019; Zhu et al., 2019), hypertension (Marques Francine et al., 2011; Marques et al., 2015; Mathe et al., 2018), diabetic nephropathy and renal fibrosis (Xu et al., 2017). Interestingly, miR-181a-5p was increased in extracellular vesicles in the plasma of septic mice (Xu et al., 2018) and induced proinflammatory cytokines such as MIP-2, IL-6, IL-β, TNF-α and FB (Xu et al., 2018). Moreover, it has been demonstrated that miR-181a also interferes with the expression of IL-8 by modulating the immune receptor TLR4 (Galicia et al., 2014). Sepsis is characterized by severe inflammatory responses; thus, these results suggest that miR-181a-5p may constitute a very early signal of inflammation in response to sepsis, and its increase in plasma can be detected before serum creatinine elevation. The increase in miR-181a-5p before renal function weakening suggests that miR-181a-5p is a potential biomarker of sepsis-induced AKI; however, its association with the decline in renal function in humans needs additional study.
We also observed upregulation of miR-23b-3p, and similar to miR-181a-5p, this miRNA is also associated with several types of neoplasms (Wei et al., 2018; Xian et al., 2018; Yang et al., 2018; Moreno et al., 2019; Rezaei et al., 2019). Interestingly, the presence of miR-23b-3p in human urinary exosomes has been reported, and its expression was increased in urinary exosomes of patients with nephrotic syndrome (Cheng et al., 2014). There is evidence that miR-23b-3p regulates multiple cellular processes in podocytes, and therefore, increased miR-23b-3p expression may be a consequence of glomerular and podocyte damage (Chen T. et al., 2018). Similar to miR-181a-5p, miR-23b-3p can regulate inflammatory responses (Smith et al., 2003), including the primary T cell immune response (O'Connor et al., 2008). Other aspects related to miR-23b-3p that are relevant in the context of the present study include the regulation of the protein PPARGC1A, which participates in the regulation of nephron segmentation during embryogenesis (Chambers et al., 2018). Additionally, PPARGC1A deficiency was associated with renal inflammation and increased nephrotoxic severity in AKI (tubular cell death and compensatory proliferation of these cells) (Fontecha-Barriuso et al., 2019). Thus, these results, together with those of the present study, reinforce that miR-23b-3p targets important proteins in renal inflammation related to sepsis-induced AKI.
The increase in miR-181a-5p and miR-23b-3p expression 2 h after LPS administration was coincident with the increase in NGAL in the serum of LPS-treated animals. Although NGAL has been indicated as a gold standard biomarker for many forms of AKI, it is important to emphasize that the diagnostic capacity of NGAL remains somewhat controversial, especially in the context of AKI. NGAL is a marker of minimal tubular damage, especially in sepsis (de Geus et al., 2013; Macdonald et al., 2017); however, there is no evidence the accuracy of NGAL measurement in predicting sepsis-induced AKI (de Geus et al., 2013). On the other hand, the increase in serum NGAL 2 h after LPS may validate our results and indicate that the miRNAs identified in the present study can be used as potential early biomarkers of LPS-induced renal damage.
Finally, our results showed that LPS induced an increase in the number of extracellular vesicles in serum. Based on size, these vesicles were mainly exosomes. This result was consistent with those of other studies showing that pathogen infection can stimulate exosome and proinflammatory cytokine release as a mechanism of immune surveillance (Bhatnagar et al., 2007; Bhatnagar and Schorey, 2007; Essandoh et al., 2015). We observed that extracellular vesicles are significantly affected by LPS by decreasing the vesicle size and by regulating the vesicular miRNA content. Exosomes ranged from 30 to 150 nm in size (Chen et al., 2022). The size of the exosomes control group showed an average of 174 nm. However, the highest concentration peaks in the control group are smaller than 150 nm in diameter (Figure 2A). One factor indicating increased exosome size is aggregates of vesicles that can be interpreted as a single vesicle by NTA and showed a larger vesicle size. This data was observed by Novaes et al. in a study that showed aggregates with variable sizes of exosomes by electron microscopy (da Silva Novaes et al., 2019). Our results revealed a significant decrease in the size of the exosome in the LPS group (Figure 2C). Bell et al. examined the size exosome characteristics of AC16 human cardiomyocytes stimulated with LPS. The results revealed that LPS significantly decreased mean exosome size (Bell et al., 2019). These findings corroborate that size of the exosomes decreases substantially in cells stimulated with LPS. Other stimuli also demonstrated similar results in a reduction of the size of exosomes, like extracellular osmotic stress (Fathali et al., 2017), sonication (Nizamudeen et al., 2021), and influence of storage at 37°C, 4°C, and −20 °C (Yuan et al., 2021). Our results showed that LPS stimuli could reduce the size and increase the number of vesicles.
In summary, we have shown that miR-181a-5p and miR-23b-3p were differentially expressed in circulating extracellular vesicles earlier than creatinine elevation in a sepsis-induced AKI rat model. These miRNAs target several molecules related to physiological and pathological conditions, especially transcription factors related to regulatory proteins involved in inflammatory processes. The difference in the expression of these miRNAs may potentially be an important tool for the early identification of sepsis-induced AKI and be useful for discriminating sepsis-induced AKI from other causes of AKI. Finally, it is worth pointing out that these miRNAs can serve as therapeutic targets in sepsis-induced AKI.
Data availability statement
The original contributions presented in the study are included in the article/Supplementary Materials, further inquiries can be directed to the corresponding author.
Ethics statement
The animal study was reviewed and approved by Ethics in Research Committee of the Federal University of Sao Paulo (CEUA-UNIFESP #3083130317).
Author contributions
CD, EM and MB designed the study. CD, EM and TG handled the animals. CD, EM, AA and AN performed the experiments. CD and AA analyzed the data. CD, AA and EM wrote the first draft of the manuscript. MB provided financial support, study materials, and the final approval of the manuscript. All authors reviewed the final version of the manuscript.
Funding
This study was supported by grants from Fundação de Amparo à Pesquisa do Estado de São Paulo (FAPESP; 2015/23345–9), Coordenação de Aperfeiçoamento de Pessoal de Nível Superior (CAPES), Conselho Nacional de Desenvolvimento Científico e Tecnológico (CNPq) and Fundação Oswaldo Ramos.
Acknowledgments
The authors are grateful to Fundação de Amparo à Pesquisa do Estado de São Paulo (FAPESP), Conselho Nacional de Desenvolvimento Científico e Tecnológico (CNPq), Coordenação de Aperfeiçoamento de Pessoal de Nível superior (CAPES) and Fundação Oswaldo Ramos.
Conflict of interest
The authors declare that the research was conducted in the absence of any commercial or financial relationships that could be construed as a potential conflict of interest.
Publisher’s note
All claims expressed in this article are solely those of the authors and do not necessarily represent those of their affiliated organizations, or those of the publisher, the editors and the reviewers. Any product that may be evaluated in this article, or claim that may be made by its manufacturer, is not guaranteed or endorsed by the publisher.
Supplementary material
The Supplementary Material for this article can be found online at: https://www.frontiersin.org/articles/10.3389/fphys.2022.944864/full#supplementary-material
References
Bell C. R., Jones L. B., Crenshaw B. J., Kumar S., Rowe G. C., Sims B., et al. (2019). The role of lipopolysaccharide-induced extracellular vesicles in cardiac cell death. Biol. (Basel) 8 (4), E69. doi:10.3390/biology8040069
Bellomo R., Ronco C., Kellum J. A., Mehta R. L., Palevsky P.Acute Dialysis Quality Initiative workgroup (2004). Acute renal failure - definition, outcome measures, animal models, fluid therapy and information technology needs: the second international consensus conference of the acute dialysis quality initiative (ADQI) group. Crit. Care 8 (4), R204–R212. doi:10.1186/cc2872
Bhatnagar S., Schorey J. S. (2007). Exosomes released from infected macrophages contain Mycobacterium avium glycopeptidolipids and are proinflammatory. J. Biol. Chem. 282, 25779–25789. doi:10.1074/jbc.M702277200
Bhatnagar S., Shinagawa K., Castellino F. J., Schorey J. S. (2007). Exosomes released from macrophages infected with intracellular pathogens stimulate a proinflammatory response in vitro and in vivo. Blood 110 (9), 3234–3244. doi:10.1182/blood-2007-03-079152
Bhatt K., Kato M., Natarajan R. (2016). Mini-review: emerging roles of microRNAs in the pathophysiology of renal diseases. Am. J. Physiol. Ren. Physiol. 310 (2), F109–F118. doi:10.1152/ajprenal.00387.2015
Chambers J. M., Poureetezadi S. J., Addiego A., Lahne M., Wingert R. A. (2018). ppargc1a controls nephron segmentation during zebrafish embryonic kidney ontogeny. eLife 7, e40266. doi:10.7554/eLife.40266
Chen E. Y., Tan C. M., Kou Y., Duan Q., Wang Z., Meirelles G. V., et al. (2013). Enrichr: interactive and collaborative HTML5 gene list enrichment analysis tool. BMC Bioinform. 14, 128. doi:10.1186/1471-2105-14-128
Chen T., Wang C., Yu H., Ding M., Zhang C., Lu X., et al. (2018a). Increased urinary exosomal microRNAs in children with idiopathic nephrotic syndrome. EBioMedicine 39, 552–561. doi:10.1016/j.ebiom.2018.11.018
Chen Y., Qiu J., Chen B., Lin Y., Chen Y., Xie G., et al. (2018b). Long non-coding RNA NEAT1 plays an important role in sepsis-induced acute kidney injury by targeting miR-204 and modulating the NF-κB pathway. Int. Immunopharmacol. 59, 252–260. doi:10.1016/j.intimp.2018.03.023
Chen J., Li P., Zhang T., Xu Z., Huang X., Wang R., et al. (2022). Review on strategies and Technologies for exosome isolation and purification. Front. Bioeng. Biotechnol. 9, 811971. doi:10.3389/fbioe.2021.811971
Cheng L., Sun X., Scicluna B. J., Coleman B. M., Hill A. F. (2014). Characterization and deep sequencing analysis of exosomal and non-exosomal miRNA in human urine. Kidney Int. 86 (2), 433–444. doi:10.1038/ki.2013.502
Colbert J. F., Ford J. A., Haeger S. M., Yang Y., Dailey K. L., Allison K. C., et al. (2017). A model-specific role of microRNA-223 as a mediator of kidney injury during experimental sepsis. Am. J. Physiol. Ren. Physiol. 313 (2), F553–F559. doi:10.1152/ajprenal.00493.2016
da Silva Novaes A., Borges F. T., Maquigussa E., Varela V. A., Dias M. V. S., Boim M. A. (2019). Influence of high glucose on mesangial cell-derived exosome composition, secretion and cell communication. Sci. Rep. 9 (1), 6270. doi:10.1038/s41598-019-42746-1
de Geus H. R. H., Betjes M. G., Schaick R. v., Groeneveld J. A. B. J. (2013). Plasma NGAL similarly predicts acute kidney injury in sepsis and nonsepsis. Biomark. Med. 7 (3), 415–421. doi:10.2217/bmm.13.5
Essandoh K., Yang L., Wang X., Huang W., Qin D., Hao J., et al. (2015). Blockade of exosome generation with GW4869 dampens the sepsis-induced inflammation and cardiac dysfunction. Biochim. Biophys. Acta 1852 (11), 2362–2371. doi:10.1016/j.bbadis.2015.08.010
Fan P.-C., Chen C.-C., Chen Y.-C., Chang Y.-S., Chu P.-H. (2016). MicroRNAs in acute kidney injury. Hum. Genomics 10 (1), 29. doi:10.1186/s40246-016-0085-z
Fathali H., Dunevall J., Majdi S., Cans A. S. (2017). Extracellular osmotic stress reduces the vesicle size while keeping a constant neurotransmitter concentration. ACS Chem. Neurosci. 8 (2), 368–375. doi:10.1021/acschemneuro.6b00350
Fontecha-Barriuso M., Martín-Sánchez D., Martinez-Moreno J., Carrasco S., Ruiz-Andrés O., Monsalve M., et al. (2019). PGC-1α deficiency causes spontaneous kidney inflammation and increases the severity of nephrotoxic AKI. J. Pathol. 249, 65–78. doi:10.1002/path.5282
Fu D., Dong J., Li P., Tang C., Cheng W., Xu Z., et al. (2017). MiRNA-21 has effects to protect kidney injury induced by sepsis. Biomed. Pharmacother. 94, 1138–1144. doi:10.1016/j.biopha.2017.07.098
Galicia J. C., Naqvi A. R., Ko C. C., Nares S., Khan A. A. (2014). MiRNA-181a regulates Toll-like receptor agonist-induced inflammatory response in human fibroblasts. Genes Immun. 15 (5), 333–337. doi:10.1038/gene.2014.24
Gallo A., Tandon M., Alevizos I., Illei G. G. (2012). The majority of microRNAs detectable in serum and saliva is concentrated in exosomes. PLOS ONE 7 (3), e30679. doi:10.1371/journal.pone.0030679
Ge Q.-M., Huang C.-M., Zhu X.-Y., Bian F., Pan S.-M. (2017). Differentially expressed miRNAs in sepsis-induced acute kidney injury target oxidative stress and mitochondrial dysfunction pathways. PLOS ONE 12 (3), e0173292. doi:10.1371/journal.pone.0173292
Ha M., Kim V. N. (2014). Regulation of microRNA biogenesis. Nat. Rev. Mol. Cell Biol. 15, 509–524. doi:10.1038/nrm3838
Hjortrup P. B., Haase N., Wetterslev M., Perner A. (2013). Clinical review: Predictive value of neutrophil gelatinase-associated lipocalin for acute kidney injury in intensive care patients. Crit. Care 17 (2), 211. doi:10.1186/cc11855
Hu Z., Chen X., Zhao Y., Tian T., Jin G., Shu Y., et al. (2010). Serum MicroRNA signatures identified in a genome-wide serum MicroRNA expression profiling predict survival of non–small-cell lung cancer. J. Clin. Oncol. 28 (10), 1721–1726. doi:10.1200/jco.2009.24.9342
Jen J., Wang Y.-C. (2016). Zinc finger proteins in cancer progression. J. Biomed. Sci. 23 (1), 53. doi:10.1186/s12929-016-0269-9
Khwaja A. (2012). KDIGO clinical practice guidelines for acute kidney injury. Nephron. Clin. Pract. 120 (4), c179–c184. doi:10.1159/000339789
Leelahavanichkul A., Somparn P., Panich T., Chancharoenthana W., Wongphom J., Pisitkun T., et al. (2015). Serum miRNA-122 in acute liver injury induced by kidney injury and sepsis in CD-1 mouse models. Hepatol. Res. 45 (13), 1341–1352. doi:10.1111/hepr.12501
Levey A. S., James M. T. (2017). Acute kidney injury. Ann. Intern. Med. 167 (9), ITC66–ITC80. doi:10.7326/aitc201711070
Lewis B. P., Burge C. B., Bartel D. P. (2005). Conserved seed pairing, often flanked by adenosines, indicates that thousands of human genes are MicroRNA targets. Cell 120 (1), 15–20. doi:10.1016/j.cell.2004.12.035
Liu Z., Wang S., Mi Q.-S., Dong Z. (2016). MicroRNAs in pathogenesis of acute kidney injury. Nephron 134 (3), 149–153. doi:10.1159/000446551
Lo L. J., Go A. S., Chertow G. M., McCulloch C. E., Fan D., Ordoñez J. D., et al. (2009). Dialysis-requiring acute renal failure increases the risk of progressive chronic kidney disease. Kidney Int. 76 (8), 893–899. doi:10.1038/ki.2009.289
Lv L.-L., Cao Y., Liu D., Xu M., Liu H., Tang R.-N., et al. (2013). Isolation and quantification of microRNAs from urinary exosomes/microvesicles for biomarker discovery. Int. J. Biol. Sci. 9 (10), 1021–1031. doi:10.7150/ijbs.6100
Ma S., Evans R. G., Iguchi N., Tare M., Parkington H. C., Bellomo R., et al. (2019). Sepsis-induced acute kidney injury: a disease of the microcirculation. Microcirculation 26 (2), e12483. doi:10.1111/micc.12483
Macdonald S. P. J., Bosio E., Neil C., Arendts G., Burrows S., Smart L., et al. (2017). Resistin and NGAL are associated with inflammatory response, endothelial activation and clinical outcomes in sepsis. Inflamm. Res. 66 (7), 611–619. doi:10.1007/s00011-017-1043-5
Mao W., Huang X., Wang L., Zhang Z., Liu M., Li Y., et al. (2019). Circular RNA hsa_circ_0068871 regulates FGFR3 expression and activates STAT3 by targeting miR-181a-5p to promote bladder cancer progression. J. Exp. Clin. Cancer Res. 38 (1), 169. doi:10.1186/s13046-019-1136-9
Marques F. Z., Romaine S. P. R., Denniff M., Eales J., Dormer J., Garrelds I. M., et al. (2015). Signatures of miR-181a on the renal transcriptome and blood pressure. Mol. Med. 21 (1), 739–748. doi:10.2119/molmed.2015.00096
Marques Francine Z., Campain Anna E., Tomaszewski M., Zukowska-Szczechowska E., Yang Yee Hwa J., Charchar Fadi J., et al. (2011). Gene expression profiling reveals renin mRNA overexpression in human hypertensive kidneys and a role for MicroRNAs. Hypertension 58 (6), 1093–1098. doi:10.1161/hypertensionaha.111.180729
Mårtensson J., Bell M., Oldner A., Xu S., Venge P., Martling C.-R. (2010). Neutrophil gelatinase-associated lipocalin in adult septic patients with and without acute kidney injury. Intensive Care Med. 36 (8), 1333–1340. doi:10.1007/s00134-010-1887-4
Mathe A., Arthurs A. L., Corbisier de Meaultsart C., Lumbers E. R., Wang Y., Pringle K. G., et al. (2018). Regulation of the human placental (pro)renin receptor-prorenin-angiotensin system by microRNAs. Mol. Hum. Reprod. 24 (9), 453–464. doi:10.1093/molehr/gay031
Moreno E. C., Pascual A., Prieto-Cuadra D., Laza V. F., Molina-Cerrillo J., Ramos-Muñoz M. E., et al. (2019). Novel molecular characterization of colorectal primary tumors based on miRNAs. Cancers 11 (3), 346. doi:10.3390/cancers11030346
Nizamudeen Z. A., Xerri R., Parmenter C., Suain K., Markus R., Chakrabarti L., et al. (2021). Low-power sonication can alter extracellular vesicle size and properties. Cells 10 (9), 2413. doi:10.3390/cells10092413
O'Connor B. P., Eun S.-Y., Ye Z., Zozulya A. L., Lich J. D., Moore C. B., et al. (2008). Semaphorin 6D regulates the late phase of CD4+ T cell primary immune responses. Proc. Natl. Acad. Sci. U. S. A. 105 (35), 13015–13020. doi:10.1073/pnas.0803386105
Rezaei Z., Sebzari A., Kordi-Tamandani D. M., Dastjerdi K. (2019). Involvement of the dysregulation of miR-23b-3p, miR-195-5p, miR-656-5p, and miR-340-5p in trastuzumab resistance of HER2-positive breast cancer cells and system biology approach to predict their targets involved in resistance. DNA Cell Biol. 38 (2), 184–192. doi:10.1089/dna.2018.4427
Schrier R. W., Wang W. (2004). Acute renal failure and sepsis. N. Engl. J. Med. 351 (2), 159–169. doi:10.1056/NEJMra032401
Simpson K., Wonnacott A., Fraser D. J., Bowen T. (2016). MicroRNAs in diabetic nephropathy: from biomarkers to therapy. Curr. Diab. Rep. 16 (3), 35. doi:10.1007/s11892-016-0724-8
Smith S. J., Brookes-Fazakerley S., Donnelly L. E., Barnes P. J., Barnette M. S., Giembycz M. A. (2003). Ubiquitous expression of phosphodiesterase 7A in human proinflammatory and immune cells. Am. J. Physiol. Lung Cell. Mol. Physiol. 284 (2), L279–L289. doi:10.1152/ajplung.00170.2002
Thomas M. E., Blaine C., Dawnay A., Devonald M. A. J., Ftouh S., Laing C., et al. (2015). The definition of acute kidney injury and its use in practice. Kidney Int. 87 (1), 62–73. doi:10.1038/ki.2014.328
Wei D., Dang Y., Feng Z., Liang L., Zhang L., Tang R., et al. (2018). Biological effect and mechanism of the miR-23b-3p/ANXA2 Axis in pancreatic ductal adenocarcinoma. Cell. Physiol. Biochem. 50 (3), 823–840. doi:10.1159/000494468
Wong N., Wang X. (2014). miRDB: an online resource for microRNA target prediction and functional annotations. Nucleic Acids Res. 43 (D1), D146–D152. doi:10.1093/nar/gku1104
Xian X., Tang L., Wu C., Huang L. (2018). miR-23b-3p and miR-130a-5p affect cell growth, migration and invasion by targeting CB1R via the Wnt/β-catenin signaling pathway in gastric carcinoma. Onco. Targets. Ther. 11, 7503–7512. doi:10.2147/ott.s181706
Xu P., Guan M.-p., Bi J.-g., Wang D., Zheng Z.-j., Xue Y.-m. (2017). High glucose down-regulates microRNA-181a-5p to increase pro-fibrotic gene expression by targeting early growth response factor 1 in HK-2 cells. Cell. Signal. 31, 96–104. doi:10.1016/j.cellsig.2017.01.012
Xu J., Feng Y., Jeyaram A., Jay S. M., Zou L., Chao W. (2018). Circulating plasma extracellular vesicles from septic mice induce inflammation via MicroRNA- and TLR7-dependent mechanisms. J. Immunol. 201 (11), 3392–3400. doi:10.4049/jimmunol.1801008
Yang T., He X., Chen A., Tan K., Du X. (2018). LncRNA HOTAIR contributes to the malignancy of hepatocellular carcinoma by enhancing epithelial-mesenchymal transition via sponging miR-23b-3p from ZEB1. Gene 670, 114–122. doi:10.1016/j.gene.2018.05.061
Yongjun L., Ying D., Shuping S., Man L., Tao W., Feng G. (2019). Expression patterns and prognostic value of miR-210, miR-494, and miR-205 in middle-aged and old patients with sepsis-induced acute kidney injury. Bosn. J. Basic Med. Sci. 19 (3), 249–256. doi:10.17305/bjbms.2019.4131
Yu C., Sun J., Leng X., Yang J. (2019a). Long noncoding RNA SNHG6 functions as a competing endogenous RNA by sponging miR-181a-5p to regulate E2F5 expression in colorectal cancer. Cancer Manag. Res. 11, 611–624. doi:10.2147/cmar.s182719
Yu J., Jiang L., Gao Y., Sun Q., Liu B., Hu Y., et al. (2019b). LncRNA CCAT1 negatively regulates miR-181a-5p to promote endometrial carcinoma cell proliferation and migration. Exp. Ther. Med. 17 (5), 4259–4266. doi:10.3892/etm.2019.7422
Yuan F., Li Y. M., Wang Z. (2021). Preserving extracellular vesicles for biomedical applications: consideration of storage stability before and after isolation. Drug Deliv. 28 (1), 1501–1509. doi:10.1080/10717544.2021.1951896
Zheng G., Qu H., Li F., Ma W., Yang H. (2018). Propofol attenuates sepsis-induced acute kidney injury by regulating miR-290-5p/CCL-2 signaling pathway. Braz. J. Med. Biol. Res. 51 (11), e7655. doi:10.1590/1414-431x20187655
Zhu L., Zhang Q., Li S., Jiang S., Cui J., Dang G. (2019). Interference of the long noncoding RNA CDKN2B-AS1 upregulates miR-181a-5p/TGFβI axis to restrain the metastasis and promote apoptosis and senescence of cervical cancer cells. Cancer Med. 8, 1721–1730. doi:10.1002/cam4.2040
Keywords: acute kidney injure, sepsis, miRNA, microRNA, exosome (vesicle), lipopolyssacharide
Citation: Da-Silva CCS, Anauate AC, Guirao TP, Novaes AdS, Maquigussa E and Boim MA (2022) Analysis of exosome-derived microRNAs as early biomarkers of lipopolysaccharide-induced acute kidney injury in rats. Front. Physiol. 13:944864. doi: 10.3389/fphys.2022.944864
Received: 16 May 2022; Accepted: 29 July 2022;
Published: 26 August 2022.
Edited by:
Denis Drygin, Regulus Therapeutics Inc., United StatesReviewed by:
Daohong Lin, New York Medical College, United StatesBaihai Jiao, University of Connecticut Health Center, United States
Copyright © 2022 Da-Silva, Anauate, Guirao, Novaes, Maquigussa and Boim. This is an open-access article distributed under the terms of the Creative Commons Attribution License (CC BY). The use, distribution or reproduction in other forums is permitted, provided the original author(s) and the copyright owner(s) are credited and that the original publication in this journal is cited, in accordance with accepted academic practice. No use, distribution or reproduction is permitted which does not comply with these terms.
*Correspondence: Edgar Maquigussa, ZS5tYXF1aWd1c3NhQHVuaWZlc3AuYnI=