- 1LPHYS, Département de Cardiologie, Université Libre de Bruxelles, Brussels, Belgium
- 2TIPs, École Polytechnique de Bruxelles, Université Libre de Bruxelles, Brussels, Belgium
- 3Institute of Aerospace Medicine, German Aerospace Center (DLR), Cologne, Germany
- 4Electronic, Information and Biomedical Engineering Department, Politecnico di Milano, Milan, Italy
Microgravity has deleterious effects on the cardiovascular system. We evaluated some parameters of blood flow and vascular stiffness during 60 days of simulated microgravity in head-down tilt (HDT) bed rest. We also tested the hypothesis that daily exposure to 30 min of artificial gravity (1 g) would mitigate these adaptations. 24 healthy subjects (8 women) were evenly distributed in three groups: continuous artificial gravity, intermittent artificial gravity, or control. 4D flow cardiac MRI was acquired in horizontal position before (−9 days), during (5, 21, and 56 days), and after (+4 days) the HDT period. The false discovery rate was set at 0.05. The results are presented as median (first quartile; third quartile). No group or group × time differences were observed so the groups were combined. At the end of the HDT phase, we reported a decrease in the stroke volume allocated to the lower body (−30% [−35%; −22%]) and the upper body (−20% [−30%; +11%]), but in different proportions, reflected by an increased share of blood flow towards the upper body. The aortic pulse wave velocity increased (+16% [+9%; +25%]), and so did other markers of arterial stiffness (
Introduction
In microgravity as well as simulated microgravity, such as during head-down tilt (HDT) bed rest, a shift of blood volume from the lower to the upper body is observed (Shen and Frishman, 2019). It is then followed by a cascade of adaptations, including a decrease in plasma volume (Blomqvist and Stone, 1983). Together with hypokinesia, in the absence of proper countermeasures, long-term exposure to (simulated) microgravity leads to a decrease in stroke volume (Herault et al., 2000) and cardiac strain (Hoffmann et al., 2021), an increase in resting heart rate (
The countermeasures currently used onboard the International Space Station appear to not optimally counteract the microgravity-induced deconditioning (Hargens et al., 2013; Hurst et al., 2019). Artificial gravity has been suggested as a multi-system countermeasure (Hargens et al., 2013) and is now studied in the context of HDT bed rest (Iwasaki et al., 2001; Iwase, 2005; Stenger et al., 2012). However, only basic investigations have been conducted so far and always for limited periods of exposure to (simulated) microgravity. As a consequence, not only longer studies are needed, but it is also necessary to evaluate the comparative efficacy of different protocols regarding the duration, intensity, and activity performed during exposure to artificial gravity (Hargens et al., 2013).
While assessing the cardiovascular state of an astronaut in space is operationally difficult, ground studies offer a relatively easy access to state-of-the-art technologies. Magnetic resonance imaging (MRI) is a good example of such a technology that can be available during HDT bed rest studies, but not in space. In particular, the recent advances in the field of cardiac MRI allow assessing many new parameters relevant to the cardiovascular health. For instance, 4D flow cardiac MRI can be used to provide a time-resolved 3D velocity field on a volume of interest during free breathing (Stankovic et al., 2014). Among others, this type of protocol enables to accurately measure blood flow rates in the aorta (Houriez--Gombaud-Saintonge et al., 2019). In turn, it allows the estimation of aortic pulse wave velocity (
Accordingly, the general objective of this research was to quantify the cardiovascular deconditioning induced by 60-day exposure to simulated microgravity by HDT bed rest, using 4D flow cardiac MRI. We expected to observe a decrease in the parameters of blood flow, leading to a decrease of aortic
Materials and methods
Study population and AGBRESA study
The research was part of the study “Artificial Gravity Bed Rest with European Space Agency” (AGBRESA) organized jointly by the American, European, and German space agencies (NASA, ESA, and DLR, respectively). It took place at the: envihab facility of the DLR in Cologne, Germany, between March and December 2019. The AGBRESA study was approved by the Northern Rhine Medical Association (Ärztekammer Nordrhein, application N°2018143) as well as the Federal Office for Radiation Protection (Bundesamt für Strahlenschutz, application N°22464/2018-074-R-G). Written informed consent was obtained from all the subjects prior to starting the protocols.
Twenty-four healthy subjects (8 women) were included after a thorough medical screening (Kramer et al., 2021) (German Clinical Trials Register – DRKS00015677). They were randomly pre-assigned to three groups matched for sex, age, and weight: a continuous artificial gravity group (cAG, n = 8, 3 women), an intermittent artificial gravity group (iAG, n = 8, 3 women), or a control group (CTRL, n = 8, 2 women). Three subjects had to be discharged from the study for medical reasons that were not identified during the screening phase and that were not related to the study protocol. These three subjects have been replaced and the demographics of the final study population can be found in Table 1.

TABLE 1. Demographics of the subjects enrolled in the AGBRESA study at baseline (BDC-9). Data are presented as median (first quartile; third quartile), except for the number of subjects (number of females in parentheses). cAG: continuous artificial gravity; iAG: intermittent artificial gravity; CTRL: control.
Each subject started with a 14-day baseline data collection (BDC) period before being assigned to one of the three aforementioned groups. Then, they began a 60-day strict (i.e., without a pillow under the head) −6° HDT bed rest period, which was followed by a 14-day recovery (R) period. While the subjects were continuously monitored to remain in HDT position during the whole HDT phase, they were authorized to move freely within the ward during the BDC and R phases. Daily water and energy intakes were standardized and controlled throughout the study. The female subjects did not take oral contraceptives and their menstrual cycle was not controlled for. A few subjects experienced some discomfort, especially at the beginning of the HDT phase, including headache, stuffy nose, and back pain. However, these symptoms were never judged as clinically significant, and we believe that they did not impact the results presented thereafter. Additional details regarding the whole protocol are also given in (Kramer et al., 2021).
During the HDT phase, the cAG and iAG groups underwent daily 30-minute exposure to artificial gravity in horizontal (0°) position, using the short-arm human centrifuge available at: envihab. The position of the subject and rotation speed were adapted on an individual basis to achieve a head-to-foot acceleration of 1 g at the center of mass and 2 g at the feet. The cAG group underwent single continuous 30-minute runs on the centrifuge, while, in the iAG group, these 30 min of daily exposure to artificial gravity were distributed in 6 bouts of 5 min separated by 3-minute breaks. The CTRL group was not exposed to artificial gravity during the whole HDT phase. Additional details regarding the artificial gravity protocol, including average spin rate and radii, can be found in (Frett et al., 2020b) and (Kramer et al., 2021).
4D flow cardiac MRI
The cardiac MRI image acquisition was performed using a Biograph mMR 3-T scanner (Siemens, Erlangen, Germany) with the subject in supine position and no use of contrast agents. The same procedure was repeated at several time points all along the study: before (−9 days, written BDC-9), after (+4 days, written R+4), and during the HDT phase (5, 21, and 56 days, written HDT5, HDT21, and HDT56, respectively). Five subjects had to perform the R+4 data point slightly later (four at +6 days and one at +5 days after the HDT phase) for operational reasons. The cardiac MRI protocol included a free breathing 4D flow acquisition with prospective electrocardiogram- and navigator-gating in a volume encompassing the thoracic aorta. A three-directional encoding velocity of 150 cm/s and the acquisition of 20 images per cardiac cycle were chosen, together with the following parameters: voxel size, 2.375 × 2.375 × 2.00 mm3; repetition time, 38.72 ms; echo time, 2.29 ms; flip angle, 7°; acquisition matrix, 90 × 160; and number of slices, 40. In addition, blood pressure at the right brachial artery was measured (Expression MR400, Philips Healthcare, Eindhoven, Netherlands) during the cardiac MRI protocol, a few minutes before the 4D flow acquisition. This protocol is summarized in Figure 1 together with the steps of the analysis.
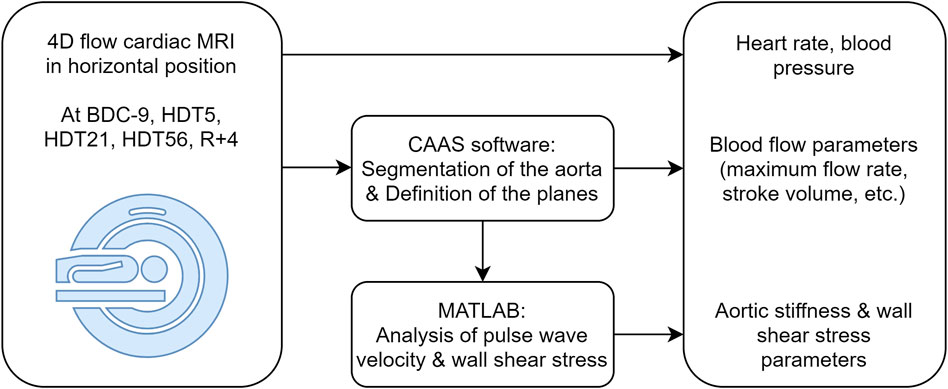
FIGURE 1. Experimental and analysis procedure. BDC: baseline data collection, HDT: head-down tilt, R: recovery.
The post-hoc analysis of the 4D flow images was performed using CAAS MR Solutions 5.2.1 (Pie Medical Imaging BV, Maastricht, The Netherlands) and MATLAB R2018b (The MathWorks, Inc., Natick, Massachusetts, United States). During the pre-processing step, the background phase offsets and velocity aliasing were automatically corrected, as recommended (Dyverfeldt et al., 2015). Then, the aortic volume was semi-automatically segmented and the centerline was extracted using the built-in functionalities available in the commercial software. Four planes were then positioned perpendicularly to the aortic centerline at standardized anatomical landmarks (van Hout et al., 2020) (Figure 2): 1.0 cm after the sinotubular junction (plane 1), just proximal to the brachiocephalic artery (plane 2), 2.0 cm distal to the left subclavian artery (plane 3), and 10.0 cm distal to plane 3 (plane 4).
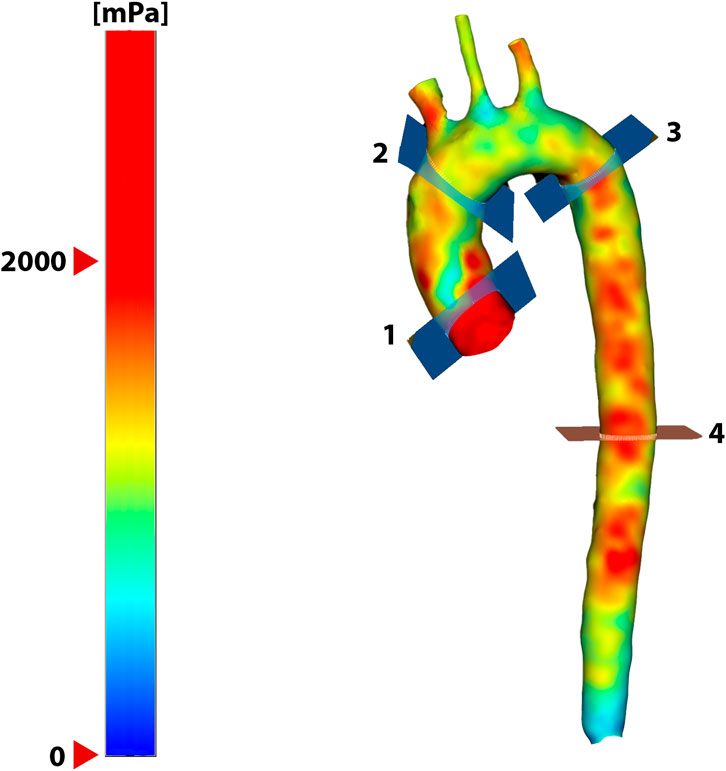
FIGURE 2. Image reconstruction of the aorta of a representative subject with a map of
The total stroke volume (
The maximum blood flow rate in the ascending aorta (
The systemic vascular resistance (
with
The total arterial compliance (
The aortic pulse wave velocity (
with
The
with
This procedure was applied to compute
We also computed the cardio-ankle vascular index (
with
with
The intersection between the segmented aorta and each of the four planes previously defined was performed automatically, before extrapolating to the point of zero velocity to properly locate the aortic wall. Assuming a constant blood viscosity of 4.0 mPa.s, the
with
The relative residence time (
where the proportionality constant between
For the sake of simplicity, in this paper, only the
Statistical analysis
The statistical analysis was performed using GraphPad Prism 9.1.2 (GraphPad Software, San Diego, CA, United States). Three records from two distinct subjects of the CTRL group could not be considered in the analysis due to poor data quality. The effects of time, group, and sex were assessed using a mixed-effects model with the Geisser-Greenhouse correction. If no group and no group × time differences were observed for the metrics studied, the subjects were pooled into a single group of 24 subjects. When the mixed-effects model displayed a significant effect of time, multiple comparisons were performed using the two-stage linear step-up procedure of Benjamini, Krieger, and Yekuteli (Benjamini et al., 2006) to compare each time point to BDC-9. The false discovery rate was set at 0.05. The results are presented as median (first quartile; third quartile), unless otherwise stated. In particular, the relative differences between different conditions are computed based on individual relative differences, before extracting the corresponding quantiles (median and quartiles).
Results
No group, group × time, group × sex, and group × sex × time differences were found at any time point and for any reported feature. The p values corresponding to the group × time effect are given in the penultimate column of Table 2 column of for the blood flow metrics, of Table 3 for the arterial stiffness metrics, and of Table 4 for the
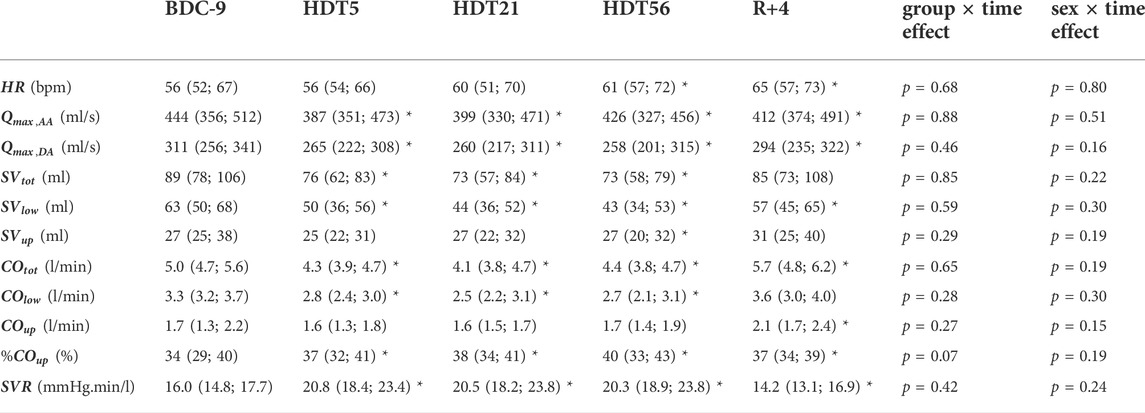
TABLE 2. Evolution of blood flow results in the ascending and the descending aorta (AA and DA, respectively) all over the study. Data are presented as median (first quartile; third quartile). The group × time and sex × time effects are displayed in the last columns.
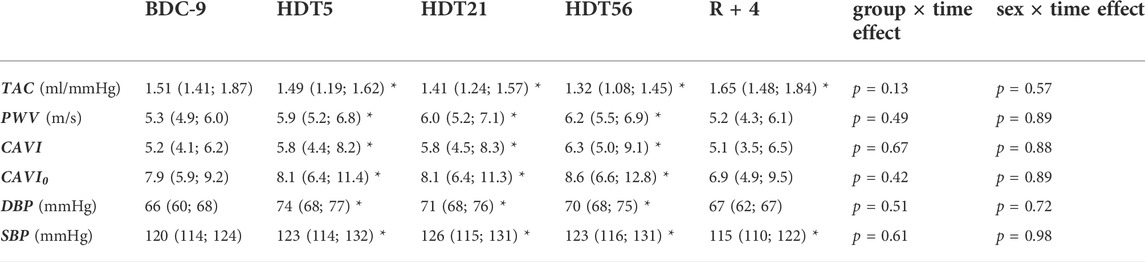
TABLE 3. Evolution of blood pressure and arterial stiffness results computed between planes 1 and 4 (Figure 2) all over the study. Data are presented as median (first quartile; third quartile). The group × time and sex × time effects are displayed in the last columns.

TABLE 4. Evolution of wall shear stress results computed in the ascending aorta (plane 2, Figure 2) all over the study. Data are presented as median (first quartile; third quartile). The group × time and sex × time effects are displayed in the last columns.
The results related to
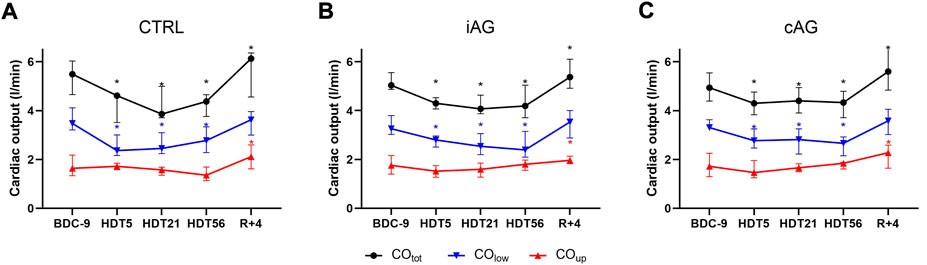
FIGURE 3. Changes in total cardiac output, as well as cardiac output allocated to the lower and upper body (
The results relevant to blood pressure and arterial stiffness are reported in Table 3. Compared to baseline values, both
Table 4 summarizes the results relevant to
The last columns of Table 2, Table 3, and Table 4 present the sex × time effects for the flow, stiffness, and
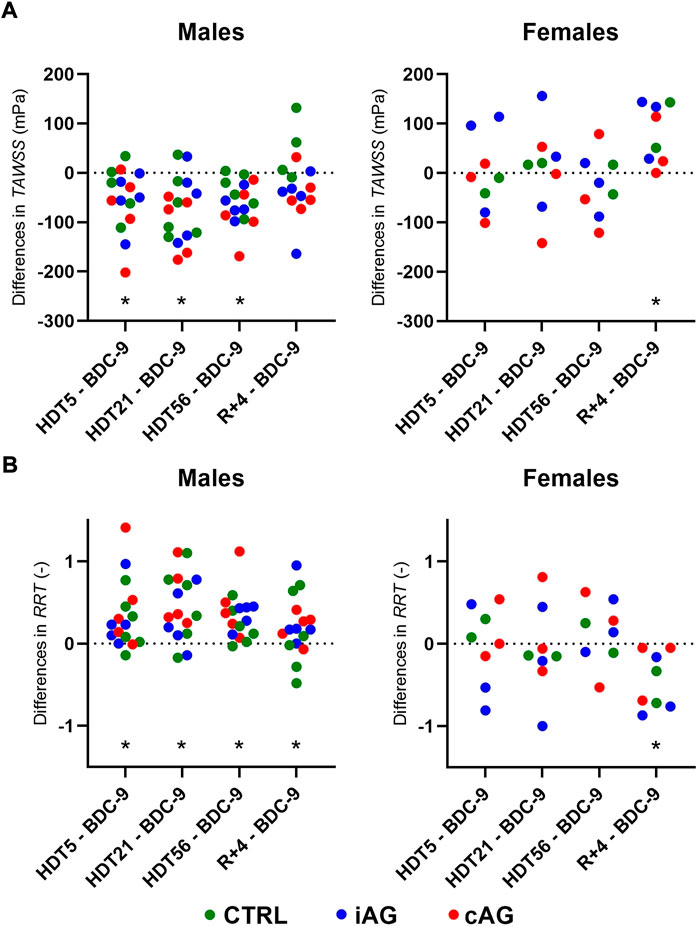
FIGURE 4. Individual evolution of: (A) TAWSS and (B) RRT among males and females and in each group at different timepoints, compared to their baseline values.*: Significant difference with BDC-9 using the two-stage linear step-up procedure of Benjamini, Krieger, and Yekuteli with a false discovery rate set at 0.05. cAG: continuous artificial gravity, iAG: intermittent artificial gravity, CTRL: control, BDC: baseline data collection, HDT: head-down tilt, R: recovery.
Discussion
We used 4D flow cardiac MRI to assess cardiovascular deconditioning among subjects exposed to 60-day strict HDT bed rest. This technique allowed the evaluation of many parameters based on the acquisition of a single volume of interest. The absence of significant differences between the three groups of subjects shows the lack of efficacy of the artificial gravity countermeasure on the estimated parameters. In addition to previously known results relevant to blood flow in (simulated) microgravity, our study confirmed that the cardiac output allocated to the upper and the lower body was differently affected. An increase in several markers of arterial stiffness was observed during the whole HDT phase, with a quick return to baseline values during the recovery phase. The markers related to
These findings could not only be relevant for astronauts, but also for patients forced to prolonged bedridden immobilization. Indeed, both populations suffer from a multi-system deconditioning that requires to be better understood to find effective countermeasures. In fact, it has been shown that the changes in blood distribution may contribute to the observed bone loss (Colleran et al., 2000), while the arterial adaptations contribute to orthostatic intolerance (Delp, 2007), which can lead to falls and fall-related injuries.
Interest of 4D flow
4D flow cardiac MRI allows for a very comprehensive assessment of blood flow dynamics in a pre-defined 3D volume throughout the cardiac cycle. Analysis planes can be placed retrospectively at any location within the acquisition volume. Not only does this allow to perform quality checks of the internal consistency of the data (Wentland et al., 2013), but it can also prevent using several standard 2D cine phase contrast protocols, each requiring accurate positioning of the relevant plane while the subject is lying in the scanner. Even though the scanning time of a 4D flow cardiac MRI protocol is longer than the one of a single 2D cine phase contrast acquisition, it can become advantageous in case a series of 2D acquisitions must be scanned (Dyverfeldt et al., 2015). This is especially important in HDT bed rest studies like this one, since it is not desired to ask subjects to remain in horizontal position for too long, especially during the HDT phase, to avoid physiological changes moving from −6° to 0° position. Nevertheless, the measurements are limited to physiological steady state situations.
In addition to the sole blood flow and its traditional metrics, the analysis of the 4D flow images allows the computation of additional parameters, such as the one presented in Table 2 and Table 3. To properly assess
Interest of artificial gravity as a countermeasure
The absence of any visible efficacy of the countermeasure used in this study is not very surprising. Indeed, it is in line with other investigations conducted with the same subjects during the same study, showing its inefficacy: to maintain aerobic exercise capacity (Kramer et al., 2021), to limit the cardiac adaptations (Hoffmann et al., 2021), and to show benefits on the vascular system (Möstl et al., 2021). A 5-day HDT bed rest study with similar artificial gravity parameters (head-to-foot acceleration of 1 g at the center of mass during 30 min per day, continuous or intermittent) led to similar conclusions, with no effect of the countermeasure on plasma volume and aerobic power (Linnarsson et al., 2015), as well as on cardiac function and mass (Caiani et al., 2014). Increasing the daily exposure to 60 min showed some interest regarding orthostatic tolerance, but did not prevent the reduction of plasma volume, stroke volume, and other indices of the cardiac function in another 21-day HDT bed rest study (Stenger et al., 2012).
It has already been reported that this intensity of artificial gravity can be well tolerated by the subjects: in this study, only 10 runs were prematurely stopped due to pre-syncopal symptoms, out of a total of 960 (Frett et al., 2020b). It can be hypothesized that a larger load factor and/or longer exposure time may be used without compromising the safety of the subjects. To this respect, Iwasaki and colleagues have shown that exposure to a daily load factor of 2 g (at the feet) for 1 h could prevent changes in resting
However, artificial gravity alone simply reproduces the effect of standing in a gravity environment, which is not very demanding for the cardiorespiratory system. Thus, this countermeasure is not effective in maintaining aerobic exercise capacity and does not completely protect against the deterioration of muscle function (Kramer et al., 2021). The subjects remain hypokinetic and, in the absence of regular and significant increases in the cardiorespiratory demand (e.g., via physical exercises), associated with increases in blood flow and
An interesting alternative to provide a fluid redistribution towards the lower body, as in a gravity environment, is the use of lower-body negative pressure (LBNP). Indeed, as opposed to the centrifuge, it does not put the subject in a rotating environment. Besides this, it is much smaller and less demanding in terms of power, technical expertise, etc. In addition to increasing LBNP tolerance (Watenpaugh et al., 1994), the combination of LBNP and physical exercise was proven to be more effective than LBNP alone in improving the orthostatic tolerance among ambulatory subjects, especially among females (Evans et al., 2018). During HDT bed rest, combined LBNP and exercise countermeasures have been effective in preserving blood volume (Guinet et al., 2009) and aerobic capacity (Hargens and Richardson, 2009). Watenpaugh and colleagues also showed the interest of adding a short rest period at the end of exercise, while continuing LBNP (Watenpaugh et al., 2007). Indeed, orthostatic stimulation immediately after exercise provides a greater cardiovascular stress than it does prior to exercise. This can be due to many factors including the ongoing skeletal muscle and cutaneous vasodilation, the reduction in vasoconstrictive sympathetic nerve activity, and the cessation of skeletal muscle pumping (Watenpaugh et al., 2007). Still, LBNP does not apply the exact same pressure gradient in the circulation as gravity, including at the level of the cerebral circulation, carotid, and aortic baroreceptors. It is also unable to stimulate the vestibular system, which impacts vestibulo-vascular control mechanisms (Watenpaugh et al., 2007).
Changes in blood flow
During the HDT phase, the observed increase in
The blood supply is not similarly affected in the upper and lower body, as reflected by the increase of
Even though it was not found in a recent spaceflight study (Lee et al., 2020), the increase of
Evolution of arterial stiffness
In contrast,
The observed changes in these markers of stiffness could be hypothesized to be at least partially due to a decrease in left ventricular ejection time (LVET). Indeed, in silico and clinical results have shown that there is an inverse relationship between LVET and
The median increase in
Hoffmann and colleagues reported no changes in
Changes in wall shear stress
In this study, the observed decrease in the amplitude of aortic
After 5 weeks of HDT bed rest, Palombo and colleagues did not observe such a decrease in peak wall shear rate in the common carotid and femoral arteries, but they also did not report any changes in mean and systolic flows through these arteries (Palombo et al., 2015). Incidentally, what is reported as
When the amplitude of
One could thus argue that the decrease in
An increased vasomotor sympathetic nerve activity and decreased plasma NO has been shown following HDT bed rest (Kamiya et al., 2000). This decreased NO bioavailability increases the vascular tone and promotes the proliferation of smooth muscle cells (Atochin and Huang, 2010). It is thus possible that the observed increase in
In an ex vivo experiment, the smooth muscle activation was shown to lead to an increase in aortic stiffness (Franchini et al., 2022). It seems in line with spaceflight findings, where a positive relationship has been observed between carotid intima-media thickness and β-stiffness (Arbeille et al., 2016). This may be the actual reason why an increase in all the markers of aortic stiffness was found during the HDT phase, with such a quick return to baseline values during recovery. However, it has to be expected that the amplitude of changes and the time of recovery may not be the same in conduit vessels like the aorta and in resistance vessels. Still, experiments conducted on hindlimb unloaded rats have shown that the differential regulation of intracellular Ca2+ in cerebral and small mesenteric arterial smooth muscle cells was no longer visible after only 3 days of recovery (Xue et al., 2011).
Differences between male and female subjects
Up to now, female astronauts represent only a small share of the overall population that has flown to space. The same holds true for the participants to studies in the field of altered gravity, including HDT bed rest studies. Only in the recent years, space agencies have tried to reduce this gap by enrolling more female volunteers in such studies, which is even more important as males and females are known to be affected differently by exposure to microgravity (Platts et al., 2014).
In particular, female astronauts are more susceptible to orthostatic intolerance than their male counterparts, with difficulties in maintaining venous return and cardiac output in the upright posture (Harm et al., 2001). Among other factors, this could be due to the indirect vasodilatory effects of estrogen, leading to a smaller vasoconstrictive responsiveness among women (Fritsch-Yelle et al., 1996). In addition, female astronauts experience a larger decrease in plasma volume following spaceflight (Waters et al., 2002), while HDT bed rest studies have come to contradictory findings regarding this parameter (Platts et al., 2014).
Some gender-dependent changes in arterial stiffness may also explain the differences observed in terms of orthostatic tolerance (Tuday et al., 2011). After 6 months in space, it was found that the β-stiffness index in the carotid artery increased more in women than in men, while the opposite was found for the pulse transit time to the finger and to the posterior tibial artery (Hughson et al., 2016). It was also shown, both in HDT bed rest and spaceflight, that arterial stiffness expressed as the inverse of
Cardiac atrophy, as well as the related impact of exercise, has been shown to occur similarly in males and females during HDT bed rest (Dorfman et al., 2007; Platts et al., 2014; Evans et al., 2018). In addition, no gender differences were observed for stroke volume and cardiac output indices following spaceflight (Waters et al., 2002). This is in line with the absence of a significant effect of sex × time observed here on
During a 28-day HDT bed rest study, a protocol combining LBNP and exercise was shown to be able to reduce orthostatic tolerance, without reporting differences between men and women (Watenpaugh et al., 2007). In another study with ambulatory subjects, 3 weeks of passive artificial gravity training improved orthostatic tolerance in males but not in females (Stenger et al., 2007). Here, no sex × group and no sex × group × time differences were observed for any of the parameters studied.
Due to the very low number of females included in each group, the relevant statistical power may not have been sufficient to provide a clear conclusion regarding the absence of gender-related differences in the different markers, including the distribution of blood flow and aortic stiffness. Accordingly, we encourage future HDT bed rest studies to enroll more women to be able to answer such questions.
Limitations
As most of the HDT bed rest studies, this research is limited by the number of included subjects. In particular, the distribution in three different groups of 8 subjects may have hidden a potential small effect of the applied countermeasure. While, it has been recommended to evaluate gender differences related to artificial gravity countermeasures (Evans et al., 2018), here there were only 2 to 3 women in each group. Even if no gender × group (results not presented) and only a few gender × time differences were observed, it is not possible to come with a clear conclusion regarding gender differences, given the very low number of female subjects in each group.
In such studies involving many experiments and paired groups, it is very difficult to control the timing of a specific protocol with regard to the menstrual cycle phase. Even HDT bed rest studies including only women often lack this type of control (Guinet et al., 2009). However, differences in the balance of ovarian hormones during the menstrual cycle have an impact on autonomic functions (Dimitriev et al., 2007), which can impact orthostatic tolerance. In addition, the menstrual cycle has been reported to have an impact on resting heart rate (Moran et al., 2000), on systolic and diastolic functions of both ventricles (Zengin et al., 2007), as well as on smooth muscle sensitivity to NO and whole-body arterial compliance (Williams et al., 2001), while central arterial stiffness remains relatively preserved (Ounis-Skali et al., 2006). We encourage including more women in future HDT bed rest studies so that the exact impact of this confounding factor can be better understood.
The 4D flow cardiac MRI acquisitions were all started after approximately 40 min in horizontal supine position, even during the HDT phase. On the one hand, this may not be representative of the actual cardiovascular state of the subjects at each step of the study, but on the other hand, it also helps to better compare the results at the different time points.
HDT bed rest results cannot readily be extended to actual microgravity as some dissimilarities remain. Indeed, the body is not in absolute weightlessness during HDT bed rest studies, which may have an impact on some cardiovascular parameters. For instance, Lee and colleagues argued that unweighting of the neck tissue overlying the common carotid artery may play a key role in space, allowing the vessel to expand, while gravity still acts upon the neck tissue in HDT bed rest and the diameter of the common carotid artery remains unchanged (Lee et al., 2020).
In addition, the fitness status of the participants was lower than the one of the astronaut population, especially when correcting for the age (Moore et al., 2014; Kramer et al., 2021). Indeed, at the time of their first flight, astronauts are usually older by about one decade than the participants of this study (Kovacs and Shadden, 2017). Thus, precautions should be taken when trying to extend these results to the whole astronaut population.
Several parameters were computed based on the brachial blood pressure, while the aortic blood pressure would have been more relevant, if it had been possible to measure it. This is particularly the case of
Finally, the 4D flow cardiac MRI protocol had a relatively low temporal resolution, especially for low heart rates, with only 20 images per cardiac cycle. It has been shown that this could lead to an underestimation of peak flow rate (Dyverfeldt et al., 2015), but also of
We believe that these limitations did not preclude any of the conclusion of this research.
Conclusion
We demonstrated the potential of 4D flow cardiac MRI to assess the longitudinal evolution of several cardiovascular parameters through a 60-day strict HDT bed rest study. To our knowledge, this is the first time that 4D flow cardiac MRI is used in this context, thus overcoming limitations of other imaging techniques, and enabling the evaluation of many aortic parameters that were never measured in simulated microgravity. In the absence of significant differences between the different groups studied, we can conclude that the effects of the applied artificial gravity countermeasures were either absent or too weak to reach statistical significance. Future evaluations of potential countermeasures based on artificial gravity should either increase the load factor, the time of exposure, or combine it with physical exercise. The observed changes in blood flow confirmed the different adaptations occurring in the upper and lower body during exposure to (simulated) microgravity. A larger share of blood volume was dedicated to the upper body during the HDT phase, which helps cerebral perfusion. All the markers of arterial stiffness indicated a more rigid aorta during the HDT phase, which may be caused by the decrease in the magnitude of
Data availability statement
The raw data supporting the conclusion of this article will be made available by the authors, without undue reservation.
Ethics statement
The studies involving human participants were reviewed and approved by the Northern Rhine Medical Association (Ärztekammer Nordrhein, application N°2018143) and Federal Office for Radiation Protection (Bundesamt für Strahlenschutz, application N°22464/2018-074-R-G). The patients/participants provided their written informed consent to participate in this study.
Author contributions
EC, JT, and P-FM designed the experiment. DG provided support for the 4D Flow cardiac MRI protocol. FH and JR performed data acquisition. JR and MI had full access to all the data of this study and take responsibility for the integrity of the data and the accuracy of data analysis. JR and MI performed the statistical analysis. JR drafted the manuscript. All the authors revised critically the manuscript and accepted the final version.
Funding
The AGBRESA study was funded by DLR, ESA (contract number 4000113871/15/NL/PG), and NASA (contract number 80JSC018P0078). JR is supported by the Fonds de la Recherche Scientifique (Mandat Aspirant F.R.S.—FNRS FC 29801). FH received funding by the DLR and the German Federal Ministry of Economy and Technology, BMWi (50WB1816). P-FM is supported by a grant from the European Space Agency and the Belgian Federal Scientific Policy Office (PRODEX PEA 4000110826). EC is supported by the Italian Space Agency (contracts 2018-7-U.0, 2022-9-U.0 and 2022-10-U.0).
Acknowledgments
We would like to thank the entire team at the DLR, including Edwin Mulder and Jessica Lee for the management of the whole study, as well as Kerstin Kempter and Annette von Waechter for generating the cardiac MRI data. We would also like to thank the 24 subjects of the AGBRESA study for their cooperation.
Conflict of interest
The authors declare that the research was conducted in the absence of any commercial or financial relationships that could be construed as a potential conflict of interest.
Publisher’s note
All claims expressed in this article are solely those of the authors and do not necessarily represent those of their affiliated organizations, or those of the publisher, the editors and the reviewers. Any product that may be evaluated in this article, or claim that may be made by its manufacturer, is not guaranteed or endorsed by the publisher.
References
Arbeille P., Provost R., Zuj K. (2016). Carotid and femoral artery intima-media thickness during 6 Months of spaceflight. Aerosp. Med. Hum. Perform. 87, 449–453. doi:10.3357/AMHP.4493.2016
Atochin D. N., Huang P. L. (2010). Endothelial nitric oxide synthase transgenic models of endothelial dysfunction. Pflugers Arch. 460, 965–974. doi:10.1007/s00424-010-0867-4
Baevsky R. M., Baranov V. M., Funtova I. I., Diedrich A., Pashenko A. V., Chernikova A. G., et al. (2007). Autonomic cardiovascular and respiratory control during prolonged spaceflights aboard the International Space Station. J. Appl. Physiol. 103, 156–161. doi:10.1152/japplphysiol.00137.2007
Bargiotas I., Mousseaux E., Yu W.-C., Venkatesh B. A., Bollache E., de Cesare A., et al. (2015). Estimation of aortic pulse wave transit time in cardiovascular magnetic resonance using complex wavelet cross-spectrum analysis. J. Cardiovasc. Magn. Reson. 17, 65. doi:10.1186/s12968-015-0164-7
Benjamini Y., Krieger A. M., Yekutieli D. (2006). Adaptive linear step-up procedures that control the false discovery rate. Biometrika 93, 491–507. doi:10.1093/biomet/93.3.491
Blomqvist C. G., Stone H. L. (1983). “Cardiovascular adjustments to gravitational stress,” in Comprehensive Physiology. Editor R. Terjung (New Jersey: Wiley), 1025–1063. doi:10.1002/cphy.cp020328
Caiani E. G., Landreani F., Costantini L., Mulder E., Gerlach D. A., Vaida P., et al. (2018). Effectiveness of high-intensity jump training countermeasure on mitral and aortic flow after 58-days head-down bed-rest assessed by phase-contrast MRI Bremen, Germany). Available at: https://elib.dlr.de/126169/[Accessed May 6, 2022].
Caiani E. G., Massabuau P., Weinert L., Vaïda P., Lang R. M. (2014). Effects of 5 days of head-down bed rest, with and without short-arm centrifugation as countermeasure, on cardiac function in males (BR-AG1 study). J. Appl. Physiol. 117, 624–632. doi:10.1152/japplphysiol.00122.2014
Caiani E. G., Riso G., Landreani F., Martin-Yebra A., Pirola S., Piatti F., et al. (2016). “Aortic flow and morphology adaptation to deconditioning after 21-days of head-down bed-rest assessed by phase contrast MRI,” in Computing in Cardiology Conference (CinC), 2016 (IEEE), Vancouver, BC, Canada, 11-14 September 2016, 73. Available at: http://ieeexplore.ieee.org/abstract/document/7868682/(Accessed May 31, 2017).
Callaghan F., Grieve S. (2018). Normal patterns of thoracic aortic wall shear stress measured using four-dimensional flow MRI in a large population. Am. J. Physiol. Heart Circ. Physiol. 315, H1174–H1181. doi:10.1152/ajpheart.00017.2018
Chen S., Zhang H., Hou Q., Zhang Y., Qiao A. (2022). Multiscale modeling of vascular remodeling induced by wall shear stress. Front. Physiol. 12, 808999. doi:10.3389/fphys.2021.808999
Chen Z., Yu H., Shi Y., Zhu M., Wang Y., Hu X., et al. (2017). Vascular remodelling relates to an elevated oscillatory shear index and relative residence time in spontaneously hypertensive rats. Sci. Rep. 7, 2007. doi:10.1038/s41598-017-01906-x
Cibis M., Potters W. V., Gijsen F. J., Marquering H., Ooij P., vanBavel E., et al. (2016). The effect of spatial and temporal resolution of cine phase contrast MRI on wall shear stress and oscillatory shear index assessment. PLOS ONE 11, e0163316. doi:10.1371/journal.pone.0163316
Colleran P. N., Wilkerson M. K., Bloomfield S. A., Suva L. J., Turner R. T., Delp M. D. (2000). Alterations in skeletal perfusion with simulated microgravity: a possible mechanism for bone remodeling. J. Appl. Physiol. 89, 1046–1054. doi:10.1152/jappl.2000.89.3.1046
Cui Y., Zhang S.-M., Zhang Q.-Y., Fan R., Li J., Guo H.-T., et al. (2010). Modulation of intracellular calcium transient in response to beta-adrenoceptor stimulation in the hearts of 4-wk-old rats during simulated weightlessness. J. Appl. Physiol. Md 1985 108, 838–844. doi:10.1152/japplphysiol.01055.2009
Delp M. D. (2007). Arterial adaptations in microgravity contribute to orthostatic tolerance. J. Appl. Physiol. 102, 836. doi:10.1152/japplphysiol.01347.2006
Demir A., Wiesemann S., Erley J., Schmitter S., Trauzeddel R. F., Pieske B., et al. (2022). Traveling volunteers: A multi-vendor, multi-center study on reproducibility and comparability of 4D flow derived aortic hemodynamics in cardiovascular magnetic resonance. J. Magn. Reson. Imaging. 55, 211–222. doi:10.1002/jmri.27804
Dimitriev D. A., Saperova E. V., Dimitriev A. D., Karpenko I. D. (2007). Features of cardiovascular functioning during different phases of the menstrual cycle. Ross. Fiziol. Zh. Im. I. M. Sechenova 93, 300–305.
Dorfman T. A., Levine B. D., Tillery T., Peshock R. M., Hastings J. L., Schneider S. M., et al. (2007). Cardiac atrophy in women following bed rest. J. Appl. Physiol. 103, 8–16. doi:10.1152/japplphysiol.01162.2006
Dyverfeldt P., Bissell M., Barker A. J., Bolger A. F., Carlhäll C.-J., Ebbers T., et al. (2015). 4D flow cardiovascular magnetic resonance consensus statement. J. Cardiovasc. Magn. Reson. 17, 72. doi:10.1186/s12968-015-0174-5
Evans J. M., Knapp C. F., Goswami N. (2018). Artificial gravity as a countermeasure to the cardiovascular deconditioning of spaceflight: Gender perspectives. Front. Physiol. 9, 716. doi:10.3389/fphys.2018.00716
Fayol A., Malloisel-Delaunay J., Fouassier D., Cristian C., Leguy C., Bareille M. P., et al. (2019). Impact of 60 Day of bedrest on long-term pulse wave velocity evolution. J. Hypertens. Los. Angel. 37, e85. doi:10.1097/01.hjh.0000570256.58051.21
Franchini G., Breslavsky I. D., Giovanniello F., Kassab A., Holzapfel G. A., Amabili M. (2022). Role of smooth muscle activation in the static and dynamic mechanical characterization of human aortas. Proc. Natl. Acad. Sci. U. S. A. 119, e2117232119. doi:10.1073/pnas.2117232119
Frett T., Green D. A., Arz M., Noppe A., Petrat G., Kramer A., et al. (2020a). Motion sickness symptoms during jumping exercise on a short-arm centrifuge. PLOS ONE 15, e0234361. doi:10.1371/journal.pone.0234361
Frett T., Green D. A., Mulder E., Noppe A., Arz M., Pustowalow W., et al. (2020b). Tolerability of daily intermittent or continuous short-arm centrifugation during 60-day 6o head down bed rest (AGBRESA study). PLOS ONE 15, e0239228. doi:10.1371/journal.pone.0239228
Fritsch-Yelle J. M., Whitson P. A., Bondar R. L., Brown T. E. (1996). Subnormal norepinephrine release relates to presyncope in astronauts after spaceflight. J. Appl. Physiol. 81, 2134–2141. doi:10.1152/jappl.1996.81.5.2134
Gao F., Cheng J.-H., Bai Y.-G., Boscolo M., Huang X.-F., Zhang X., et al. (2012). Mechanical properties and composition of mesenteric small arteries of simulated microgravity rats with and without daily -G(x) gravitation. Sheng Li Xue Bao 64, 107–120.
Giudici A., Khir A. W., Reesink K. D., Delhaas T., Spronck B. (2021). Five years of cardio-ankle vascular index (CAVI) and CAVI0: how close are we to a pressure-independent index of arterial stiffness? J. Hypertens. 39, 2128–2138. doi:10.1097/HJH.0000000000002928
Goldstein M. A., Edwards R. J., Schroeter J. P. (1992). Cardiac morphology after conditions of microgravity during COSMOS 2044. J. Appl. Physiol. 73, 94S. doi:10.1152/jappl.1992.73.2.S94
Greaves D., Arbeille P., Guillon L., Zuj K., Caiani E. G. (2019). Effects of exercise countermeasure on myocardial contractility measured by 4D speckle tracking during a 21-day head-down bed rest. Eur. J. Appl. Physiol. 119, 2477–2486. doi:10.1007/s00421-019-04228-0
Greaves D., Guillon L., Besnard S., Navasiolava N., Arbeille P. (2021). 4 Day in dry immersion reproduces partially the aging effect on the arteries as observed during 6 month spaceflight or confinement. Npj Microgravity 7, 43–47. doi:10.1038/s41526-021-00172-6
Guinet P., Schneider S. M., Macias B. R., Watenpaugh D. E., Hughson R. L., Le Traon A. P., et al. (2009). WISE-2005: effect of aerobic and resistive exercises on orthostatic tolerance during 60 days bed rest in women. Eur. J. Appl. Physiol. 106, 217–227. doi:10.1007/s00421-009-1009-6
Haluska B. A., Jeffriess L., Downey M., Carlier S. G., Marwick T. H. (2008). Influence of cardiovascular risk factors on total arterial compliance. J. Am. Soc. Echocardiogr. 21, 123–128. doi:10.1016/j.echo.2007.05.043
Hargens A. R., Bhattacharya R., Schneider S. M. (2013). Space physiology VI: exercise, artificial gravity, and countermeasure development for prolonged space flight. Eur. J. Appl. Physiol. 113, 2183–2192. doi:10.1007/s00421-012-2523-5
Hargens A. R., Richardson S. (2009). Cardiovascular adaptations, fluid shifts, and countermeasures related to space flight. Respir. Physiol. Neurobiol. 169, S30–S33. doi:10.1016/j.resp.2009.07.005
Harloff A., Mirzaee H., Lodemann T., Hagenlocher P., Wehrum T., Stuplich J., et al. (2018). Determination of aortic stiffness using 4D flow cardiovascular magnetic resonance - a population-based study. J. Cardiovasc. Magn. Reson. 20, 43. doi:10.1186/s12968-018-0461-z
Harm D. L., Jennings R. T., Meck J. V., Powell M. R., Putcha L., Sams C. P., et al. (2001). Invited review: Gender issues related to spaceflight: a NASA perspective. J. Appl. Physiol. 91, 2374–2383. doi:10.1152/jappl.2001.91.5.2374
Herault S., Fomina G., Alferova I., Kotovskaya A., Poliakov V., Arbeille P. (2000). Cardiac, arterial and venous adaptation to weightlessness during 6-month MIR spaceflights with and without thigh cuffs (bracelets). Eur. J. Appl. Physiol. 81, 384–390. doi:10.1007/s004210050058
Hitosugi M., Niwa M., Takatsu A. (2000). Rheologic changes in venous blood during prolonged sitting. Thromb. Res. 100, 409–412. doi:10.1016/S0049-3848(00)00348-0
Hoffmann B., Dehkordi P., Khosrow-Khavar F., Goswami N., Blaber A. P., Tavakolian K. (2022). Mechanical deconditioning of the heart due to long-term bed rest as observed on seismocardiogram morphology. Npj Microgravity 8, 25. doi:10.1038/s41526-022-00206-7
Hoffmann F., Möstl S., Luchitskaya E., Funtova I., Jordan J., Baevsky R., et al. (2019). An oscillometric approach in assessing early vascular ageing biomarkers following long-term space flights. Int. J. Cardiol. Hypertens. 2, 100013. doi:10.1016/j.ijchy.2019.100013
Hoffmann F., Rabineau J., Mehrkens D., Gerlach D. A., Moestl S., Johannes B. W., et al. (2021). Cardiac adaptations to 60 day head-down-tilt bed rest deconditioning. Findings from the AGBRESA study. Esc. Heart Fail. 8, 729–744. doi:10.1002/ehf2.13103
Hoogendoorn A., Kok A. M., Hartman E. M. J., de Nisco G., Casadonte L., Chiastra C., et al. (2020). Multidirectional wall shear stress promotes advanced coronary plaque development: comparing five shear stress metrics. Cardiovasc. Res. 116, 1136–1146. doi:10.1093/cvr/cvz212
Houriez--Gombaud-Saintonge S., Mousseaux E., Bargiotas I., De Cesare A., Dietenbeck T., Bouaou K., et al. (2019). Comparison of different methods for the estimation of aortic pulse wave velocity from 4D flow cardiovascular magnetic resonance. J. Cardiovasc. Magn. Reson. 21, 75. doi:10.1186/s12968-019-0584-x
Hu H.-X., Du F.-Y., Fu W.-W., Jiang S.-F., Cao J., Xu S.-H., et al. (2017). A dramatic blood plasticity in hibernating and 14-day hindlimb unloading Daurian ground squirrels (Spermophilus dauricus). J. Comp. Physiol. B 187, 869–879. doi:10.1007/s00360-017-1092-7
Hughson R. L., Robertson A. D., Arbeille P., Shoemaker J. K., Rush J. W. E., Fraser K. S., et al. (2016). Increased postflight carotid artery stiffness and inflight insulin resistance resulting from 6-mo spaceflight in male and female astronauts. Am. J. Physiol. Heart Circ. Physiol. 310, H628–H638. doi:10.1152/ajpheart.00802.2015
Hurst C., Scott J. P. R., Weston K. L., Weston M. (2019). High-intensity interval training: A potential exercise countermeasure during human spaceflight. Front. Physiol. 10, 581. doi:10.3389/fphys.2019.00581
Irace C., Cortese C., Fiaschi E., Carallo C., Farinaro E., Gnasso A. (2004). Wall shear stress is associated with intima-media thickness and carotid atherosclerosis in subjects at low coronary heart disease risk. Stroke 35, 464–468. doi:10.1161/01.STR.0000111597.34179.47
Iwasaki K., Sasaki T., Hirayanagi K., Yajima K. (2001). Usefulness of daily +2Gz load as a countermeasure against physiological problems during weightlessness. Acta Astronaut. 49, 227–235. doi:10.1016/S0094-5765(01)00101-1
Iwase S. (2005). Effectiveness of centrifuge-induced artificial gravity with ergometric exercise as a countermeasure during simulated microgravity exposure in humans. Acta Astronaut. 57, 75–80. doi:10.1016/j.actaastro.2005.03.013
Kamiya A., Iwase S., Michikami D., Fu Q., Mano T., Kitaichi K., et al. (2000). Increased vasomotor sympathetic nerve activity and decreased plasma nitric oxide release after head-down bed rest in humans: disappearance of correlation between vasoconstrictor and vasodilator. Neurosci. Lett. 281, 21–24. doi:10.1016/S0304-3940(00)00804-1
Kovacs G. T. A., Shadden M. (2017). Analysis of age as a factor in NASA astronaut selection and career landmarks. PLoS ONE 12, e0181381. doi:10.1371/journal.pone.0181381
Kramer A., Venegas-Carro M., Mulder E., Lee J. K., Moreno-Villanueva M., Bürkle A., et al. (2020). Cardiorespiratory and neuromuscular demand of daily centrifugation: Results from the 60-day AGBRESA bed rest study. Front. Physiol. 11, 562377. doi:10.3389/fphys.2020.562377
Kramer A., Venegas-Carro M., Zange J., Sies W., Maffiuletti N. A., Gruber M., et al. (2021). Daily 30-min exposure to artificial gravity during 60 days of bed rest does not maintain aerobic exercise capacity but mitigates some deteriorations of muscle function: results from the AGBRESA RCT. Eur. J. Appl. Physiol. 121, 2015–2026. doi:10.1007/s00421-021-04673-w
Kramer L. A., Hasan K. M., Sargsyan A. E., Marshall-Goebel K., Rittweger J., Donoviel D., et al. (2017). Quantitative MRI volumetry, diffusivity, cerebrovascular flow, and cranial hydrodynamics during head-down tilt and hypercapnia: the SPACECOT study. J. Appl. Physiol. 122, 1155–1166. doi:10.1152/japplphysiol.00887.2016
Lee S. M. C., Feiveson A. H., Stein S., Stenger M. B., Platts S. H. (2015). Orthostatic intolerance after ISS and space shuttle missions. Aerosp. Med. Hum. Perform. 86, A54. doi:10.3357/AMHP.EC08.2015
Lee S. M. C., Ribeiro L. C., Martin D. S., Zwart S. R., Feiveson A. H., Laurie S. S., et al. (2020). Arterial structure and function during and after long-duration spaceflight. J. Appl. Physiol. 129, 108–123. doi:10.1152/japplphysiol.00550.2019
Li X.-T., Yang C.-B., Zhu Y.-S., Sun J., Shi F., Wang Y.-C., et al. (2017). Moderate exercise based on artificial gravity preserves orthostatic tolerance and exercise capacity during short-term head-down bed rest. Physiol. Res. 66, 567–580. doi:10.33549/physiolres.933493
Li Y., Shi H., Fan Q., Bai G., Duan Y., Xie J. (2002). Effects of qiang gu kang wei compound on hemorheology in tail-suspended rats. Hang Tian Yi Xue Yu Yi Xue Gong Cheng Space Med. Med. Eng. 15, 327–330.
Lillie J. S., Liberson A. S., Mix D., Schwarz K. Q., Chandra A., Phillips D. B., et al. (2015). Pulse wave velocity prediction and compliance assessment in elastic arterial segments. Cardiovasc. Eng. Technol. 6, 49–58. doi:10.1007/s13239-014-0202-x
Lin L.-J., Hsieh N.-C., Wu S.-F. V., Tan T.-H., Alizargar A., Bai C.-H., et al. (2020). Factors associated with cardio ankle vascular index (CAVI) and its mathematically corrected formula (CAVI0) in community dwelling individuals. Artery Res. 27, 53–58. doi:10.2991/artres.k.201124.002
Linnarsson D., Hughson R. L., Fraser K. S., Clément G., Karlsson L. L., Mulder E., et al. (2015). Effects of an artificial gravity countermeasure on orthostatic tolerance, blood volumes and aerobic power after short-term bed rest (BR-AG1). J. Appl. Physiol. 118, 29–35. doi:10.1152/japplphysiol.00061.2014
Maggioni M. A., Castiglioni P., Merati G., Brauns K., Gunga H.-C., Mendt S., et al. (2018). High-intensity exercise mitigates cardiovascular deconditioning during long-duration bed rest. Front. Physiol. 9, 1553. doi:10.3389/fphys.2018.01553
Mahmoudi M., Farghadan A., McConnell D. R., Barker A. J., Wentzel J. J., Budoff M. J., et al. (2020). The story of wall shear stress in coronary artery atherosclerosis: Biochemical transport and mechanotransduction. J. Biomech. Eng. 143, 041002. doi:10.1115/1.4049026
Markl M., Wallis W., Strecker C., Gladstone B. P., Vach W., Harloff A. (2012). Analysis of pulse wave velocity in the thoracic aorta by flow-sensitive four-dimensional MRI: Reproducibility and correlation with characteristics in patients with aortic atherosclerosis. J. Magn. Reson. Imaging 35, 1162–1168. doi:10.1002/jmri.22856
Meloni A., Zymeski H., Pepe A., Lombardi M., Wood J. C. (2014). Robust estimation of pulse wave transit time using group delay. J. Magn. Reson. Imaging 39, 550–558. doi:10.1002/jmri.24207
Moore A. D., Downs M. E., Lee S. M. C., Feiveson A. H., Knudsen P., Ploutz-Snyder L. (2014). Peak exercise oxygen uptake during and following long-duration spaceflight. J. Appl. Physiol. 117, 231–238. doi:10.1152/japplphysiol.01251.2013
Moran V. H., Leathard H. L., Coley J. (2000). Cardiovascular functioning during the menstrual cycle. Clin. Physiol. 20, 496–504. doi:10.1046/j.1365-2281.2000.00285.x
Möstl S., Orter S., Hoffmann F., Bachler M., Hametner B., Wassertheurer S., et al. (2021). Limited effect of 60-days strict head down tilt bed rest on vascular aging. Front. Physiol. 12, 685473. doi:10.3389/fphys.2021.685473
Navasiolava N., Yuan M., Murphy R., Robin A., Coupé M., Wang L., et al. (2020). Vascular and microvascular dysfunction induced by microgravity and its analogs in humans: Mechanisms and countermeasures. Front. Physiol. 11, 952. doi:10.3389/fphys.2020.00952
Nürnberger J., Saez A. O., Dammer S., Mitchell A., Wenzel R. R., Philipp T., et al. (2003). Left ventricular ejection time: a potential determinant of pulse wave velocity in young, healthy males. J. Hypertens. 21, 2125–2132. doi:10.1097/01.hjh.0000098125.00558.40
Orter S., Möstl S., Bachler M., Hoffmann F., Mayer C. C., Kaniusas E., et al. (2022). A comparison between left ventricular ejection time measurement methods during physiological changes induced by simulated microgravity. Exp. Physiol. 107, 213–221. doi:10.1113/EP090103
Ounis-Skali N., Mitchell G. F., Solomon C. G., Solomon S. D., Seely E. W. (2006). Changes in central arterial pressure waveforms during the normal menstrual cycle. J. Investig. Med. 54, 321–326. doi:10.2310/6650.2006.05055
Palombo C., Morizzo C., Baluci M., Lucini D., Ricci S., Biolo G., et al. (2015). Large artery remodeling and dynamics following simulated microgravity by prolonged head-down tilt bed rest in humans. Biomed. Res. Int. 2015, e342565. doi:10.1155/2015/342565
Pawelczyk J. A., Zuckerman J. H., Blomqvist C. G., Levine B. D. (2001). Regulation of muscle sympathetic nerve activity after bed rest deconditioning. Am. J. Physiol. Heart Circ. Physiol. 280, H2230–H2239. doi:10.1152/ajpheart.2001.280.5.H2230
Pedley T. J. (Editor) (1980). The fluid mechanics of large blood vessels (Cambridge, UK: Cambridge University Press).
Perhonen M. A., Zuckerman J. H., Levine B. D. (2001). Deterioration of left ventricular chamber performance after bed rest. Circulation 103, 1851–1857. doi:10.1161/01.CIR.103.14.1851
Platts S. H., Bairey Merz C. N., Barr Y., Fu Q., Gulati M., Hughson R., et al. (2014). Effects of sex and gender on adaptation to space: Cardiovascular alterations. J. Womens Health 23, 950–955. doi:10.1089/jwh.2014.4912
Platts S. H., Martin D. S., Stenger M. B., Perez S. A., Ribeiro C. L., Summers R., et al. (2009). Cardiovascular adaptations to long-duration head-down bed rest. Aviat. Space Environ. Med. 80, A29–A36. doi:10.3357/ASEM.BR03.2009
Rabineau J., Hossein A., Landreani F., Haut B., Mulder E., Luchitskaya E., et al. (2020). Cardiovascular adaptation to simulated microgravity and countermeasure efficacy assessed by ballistocardiography and seismocardiography. Sci. Rep. 10, 17694. doi:10.1038/s41598-020-74150-5
Ray C. A., Vasques M., Miller T. A., Wilkerson M. K., Delp M. D. (2001). Effect of short-term microgravity and long-term hindlimb unloading on rat cardiac mass and function. J. Appl. Physiol. 91, 1207–1213. doi:10.1152/jappl.2001.91.3.1207
Rikhtegar F., Knight J. A., Olgac U., Saur S. C., Poulikakos D., Marshall W., et al. (2012). Choosing the optimal wall shear parameter for the prediction of plaque location—a patient-specific computational study in human left coronary arteries. Atherosclerosis 221, 432–437. doi:10.1016/j.atherosclerosis.2012.01.018
Ryou M. (2003). Role of blood viscosity in the regulation of hematocrit in tail-suspended rats (Rattus norvegicus) treated with pentoxifylline. Available at: https://esirc.emporia.edu/handle/123456789/1070 [Accessed May 9, 2022].
Salvi P., Palombo C., Salvi G. M., Labat C., Parati G., Benetos A. (2013). Left ventricular ejection time, not heart rate, is an independent correlate of aortic pulse wave velocity. J. Appl. Physiol. 115, 1610–1617. doi:10.1152/japplphysiol.00475.2013
Saunders D. K., Roberts A. C., Aldrich K. J., Cuthbertson B. (2002). Hematological and blood viscosity changes in tail-suspended rats. Aviat. Space Environ. Med. 73, 647–653.
Shen M., Frishman W. H. (2019). Effects of spaceflight on cardiovascular physiology and health. Cardiol. Rev. 27, 122–126. doi:10.1097/CRD.0000000000000236
Shen X., Dong Q., Chen J., Meng J., Jin Y., Wen Z., et al. (1997). Erythrocyte deformation in simulated weightless human and rabbits. J. Gravit. Physiol. 4, 61–65.
Shirai K., Suzuki K., Tsuda S., Shimizu K., Takata M., Yamamoto T., et al. (2019). Comparison of cardio-ankle vascular index (CAVI) and CAVI0 in large healthy and hypertensive populations. J. Atheroscler. Thromb. 26, 603–615. doi:10.5551/jat.48314
Shirai K., Utino J., Otsuka K., Takata M. (2006). A novel blood pressure-independent arterial wall stiffness parameter; cardio-ankle vascular index (CAVI). J. Atheroscler. Thromb. 13, 101–107. doi:10.5551/jat.13.101
Soulis J. V., Lampri O. P., Fytanidis D. K., Giannoglou G. D. (2011). “Relative residence time and oscillatory shear index of non-Newtonian flow models in aorta,” in 2011 10th International Workshop on Biomedical Engineering, Kos Island, 5-7 Oct. 2011, 1–4. doi:10.1109/IWBE.2011.6079011
Spaak J., Montmerle S., Sundblad P., Linnarsson D. (2005). Long-term bed rest-induced reductions in stroke volume during rest and exercise: cardiac dysfunction vs. volume depletion. J. Appl. Physiol. 98, 648–654. doi:10.1152/japplphysiol.01332.2003
Spronck B., Avolio A. P., Tan I., Butlin M., Reesink K. D., Delhaas T. (2017). Arterial stiffness index beta and cardio-ankle vascular index inherently depend on blood pressure but can be readily corrected. J. Hypertens. 35, 98–104. doi:10.1097/HJH.0000000000001132
Spronck B., Heusinkveld M. H. G., Vanmolkot F. H., Roodt J. O. ’t., Hermeling E., Delhaas T., et al. (2015). Pressure-dependence of arterial stiffness: potential clinical implications. J. Hypertens. 33, 330–338. doi:10.1097/HJH.0000000000000407
Stalder A. f., Russe M. f., Frydrychowicz A., Bock J., Hennig J., Markl M. (2008). Quantitative 2D and 3D phase contrast MRI: Optimized analysis of blood flow and vessel wall parameters. Magn. Reson. Med. 60, 1218–1231. doi:10.1002/mrm.21778
Stankovic Z., Allen B. D., Garcia J., Jarvis K. B., Markl M. (2014). 4D flow imaging with MRI. Cardiovasc. Diagn. Ther. 4, 173–192. doi:10.3978/j.issn.2223-3652.2014.01.02
Stenger M. B., Evans J. M., Knapp C. F., Lee S. M. C., Phillips T. R., Perez S. A., et al. (2012). Artificial gravity training reduces bed rest-induced cardiovascular deconditioning. Eur. J. Appl. Physiol. 112, 605–616. doi:10.1007/s00421-011-2005-1
Stenger M. B., Evans J. M., Patwardhan A. R., Moore F. B., Hinghofer-Szalkay H., Rössler A., et al. (2007). Artificial gravity training improves orthostatic tolerance in ambulatory men and women. Acta Astronaut. 60, 267–272. doi:10.1016/j.actaastro.2006.08.008
Sun B., Zhang L.-F., Gao F., Ma X.-W., Zhang M.-L., Liu J., et al. (2004). Daily short-period gravitation can prevent functional and structural changes in arteries of simulated microgravity rats. J. Appl. Physiol. 97, 1022–1031. doi:10.1152/japplphysiol.00188.2004
Sun X.-Q., Yao Y.-J., Yang C.-B., Jiang S.-Z., Jiang C.-L., Liang W.-B. (2005). Effect of lower-body negative pressure on cerebral blood flow velocity during 21 days head-down tilt bed rest. Med. Sci. Monit. 11, CR1-5.
Takahashi K., Yamamoto T., Tsuda S., Maruyama M., Shirai K. (2020). The background of calculating CAVI: Lesson from the discrepancy between CAVI and CAVI0. Vasc. Health Risk Manag. 16, 193–201. doi:10.2147/VHRM.S223330
Takehara Y. (2022). Clinical application of 4D flow MR imaging for the abdominal aorta. Magn. Reson. Med. Sci. 21, 354–364. doi:10.2463/mrms.rev.2021-0156
Tanaka K., Nishimura N., Kawai Y. (2017). Adaptation to microgravity, deconditioning, and countermeasures. J. Physiol. Sci. 67, 271–281. doi:10.1007/s12576-016-0514-8
Tang B. T., Cheng C. P., Draney M. T., Wilson N. M., Tsao P. S., Herfkens R. J., et al. (2006). Abdominal aortic hemodynamics in young healthy adults at rest and during lower limb exercise: quantification using image-based computer modeling. Am. J. Physiol. Heart Circ. Physiol. 291, H668–H676. doi:10.1152/ajpheart.01301.2005
The Reference Values for Arterial Stiffness’ Collaboration (2010). Determinants of pulse wave velocity in healthy people and in the presence of cardiovascular risk factors: ‘establishing normal and reference values. Eur. Heart J. 31, 2338–2350. doi:10.1093/eurheartj/ehq165
Thijssen D. H. J., Green D. J., Hopman M. T. E. (2011a). Blood vessel remodeling and physical inactivity in humans. J. Appl. Physiol. 111, 1836–1845. doi:10.1152/japplphysiol.00394.2011
Thijssen D. H. J., Scholten R. R., van den Munckhof I. C. L., Benda N., Green D. J., Hopman M. T. E. (2011b1979). Acute change in vascular tone alters intima-media thickness. Hypertension 58, 240–246. doi:10.1161/HYPERTENSIONAHA.111.173583
Traon A. P., Vasseur P., Arbeille P., Güell A., Bes A., Gharib C., et al. (1995). Effects of 28-day head-down tilt with and without countermeasures on lower body negative pressure responses. Aviat. Space Environ. Med. 66, 982–991.
Trenti C., Ziegler M., Bjarnegård N., Ebbers T., Lindenberger M., Dyverfeldt P. (2022). Wall shear stress and relative residence time as potential risk factors for abdominal aortic aneurysms in males: a 4D flow cardiovascular magnetic resonance case–control study. J. Cardiovasc. Magn. Reson. 24, 18. doi:10.1186/s12968-022-00848-2
Tuday E. C., Meck J. V., Nyhan D., Shoukas A. A., Berkowitz D. E. (2007). Microgravity-induced changes in aortic stiffness and their role in orthostatic intolerance. J. Appl. Physiol. 102, 853–858. doi:10.1152/japplphysiol.00950.2006
Tuday E. C., Platts S. H., Nyhan D., Shoukas A. A., Berkowitz D. E. (2011). A retrospective analysis on gender differences in the arterial stiffness response to microgravity exposure. Gravitational Space Res. 25. Available at: http://gravitationalandspaceresearch.org/index.php/journal/article/view/533 (Accessed July 11, 2022).
van der Palen R. L. F., Roest A. A. W., van den Boogaard P. J., de Roos A., Blom N. A., Westenberg J. J. M. (2018). Scan–rescan reproducibility of segmental aortic wall shear stress as assessed by phase-specific segmentation with 4D flow MRI in healthy volunteers. Magn. Reson. Mat. Phys. Biol. Med. 31, 653–663. doi:10.1007/s10334-018-0688-6
van Duijnhoven N. T. L., Green D. J., Felsenberg D., Belavý D. L., Hopman M. T. E., Thijssen D. H. J. (2010). Impact of bed rest on conduit artery remodeling: effect of exercise countermeasures. Hypertension 56, 240–246. doi:10.1161/HYPERTENSIONAHA.110.152868
van Hout M. J., Scholte A. J., Juffermans J. F., Westenberg J. J., Zhong L., Zhou X., et al. (2020). How to measure the aorta using MRI: A practical guide. J. Magn. Reson. Imaging 52, 971–977. doi:10.1002/jmri.27183
Vernikos J., Ludwig D. A., Ertl A. C., Wade C. E., Keil L., O’Hara D. (1996). Effect of standing or walking on physiological changes induced by head down bed rest: implications for spaceflight. Aviat. Space Environ. Med. 67, 1069–1079.
Vlachopoulos C., Aznaouridis K., Stefanadis C. (2010). Prediction of cardiovascular events and all-cause mortality with arterial stiffness: a systematic review and meta-analysis. J. Am. Coll. Cardiol. 55, 1318–1327. doi:10.1016/j.jacc.2009.10.061
Wang Y.-C., Yang C.-B., Wu Y.-H., Gao Y., Lu D.-Y., Shi F., et al. (2011). Artificial gravity with ergometric exercise as a countermeasure against cardiovascular deconditioning during 4 days of head-down bed rest in humans. Eur. J. Appl. Physiol. 111, 2315–2325. doi:10.1007/s00421-011-1866-7
Watenpaugh D. E., Ballard R. E., Stout M. S., Murthy G., Whalen R. T., Hargens A. R. (1994). Dynamic leg exercise improves tolerance to lower body negative pressure. Aviat. Space Environ. Med. 65, 412–418.
Watenpaugh D. E., Hargens A. R. (2011). “The cardiovascular system in microgravity,” in Comprehensive Physiology. Editor R. Terjung (Hoboken, NJ, USA: John Wiley & Sons). doi:10.1002/cphy.cp040129
Watenpaugh D. E., O’Leary D. D., Schneider S. M., Lee S. M. C., Macias B. R., Tanaka K., et al. (2007). Lower body negative pressure exercise plus brief postexercise lower body negative pressure improve post-bed rest orthostatic tolerance. J. Appl. Physiol. 103, 1964–1972. doi:10.1152/japplphysiol.00132.2007
Waters W. W., Ziegler M. G., Meck J. V. (2002). Postspaceflight orthostatic hypotension occurs mostly in women and is predicted by low vascular resistance. J. Appl. Physiol. 92, 586–594. doi:10.1152/japplphysiol.00544.2001
Wentland A. L., Grist T. M., Wieben O. (2013). Repeatability and internal consistency of abdominal 2D and 4D phase contrast MR flow measurements. Acad. Radiol. 20, 699–704. doi:10.1016/j.acra.2012.12.019
Williams B., Mancia G., Spiering W., Al E., Bochud M., Messerli F., et al. (2018). 2018 ESC/ESH guidelines for the management of arterial hypertension: The task force for the management of arterial hypertension of the European society of cardiology and the European society of hypertension: The task force for the management of arterial hypertension of the European society of cardiology and the European society of hypertension. J. Hypertens. 36, 1953–2041. doi:10.1097/HJH.0000000000001940
Williams M. R., Westerman R. A., Kingwell B. A., Paige J., Blombery P. A., Sudhir K., et al. (2001). Variations in endothelial function and arterial compliance during the menstrual cycle. J. Clin. Endocrinol. Metab. 86, 5389–5395. doi:10.1210/jcem.86.11.8013
Xue J.-H., Chen L.-H., Zhao H.-Z., Pu Y.-D., Feng H.-Z., Ma Y.-G., et al. (2011). Differential regulation and recovery of intracellular Ca2+ in cerebral and small mesenteric arterial smooth muscle cells of simulated microgravity rat. PLOS ONE 6, e19775. doi:10.1371/journal.pone.0019775
Yang C.-B., Wang Y.-C., Gao Y., Geng J., Wu Y.-H., Zhang Y., et al. (2011). Artificial gravity with ergometric exercise preserves the cardiac, but not cerebrovascular, functions during 4days of head-down bed rest. Cytokine 56, 648–655. doi:10.1016/j.cyto.2011.09.004
Yang C.-B., Zhang S., Zhang Y., Wang B., Yao Y.-J., Wang Y.-C., et al. (2010). Combined short-arm centrifuge and aerobic exercise training improves cardiovascular function and physical working capacity in humans. Med. Sci. Monit. 16, CR575–83.
Young L. R., Hecht H., Lyne L. E., Sienko K. H., Cheung C. C., Kavelaars J. (2001). Artificial gravity: head movements during short-radius centrifugation. Acta Astronaut. 49, 215–226. doi:10.1016/S0094-5765(01)00100-X
Yuan M., Alameddine A., Coupé M., Navasiolava N. M., Li Y., Gauquelin-Koch G., et al. (2015). Effect of Chinese herbal medicine on vascular functions during 60-day head-down bed rest. Eur. J. Appl. Physiol. 115, 1975. doi:10.1007/s00421-015-3176-y
Zengin K., Tokac M., Duzenli M. A., Soylu A., Aygul N., Ozdemir K. (2007). Influence of menstrual cycle on cardiac performance. Maturitas 58, 70–74. doi:10.1016/j.maturitas.2007.06.002
Zhang B., Gu J., Qian M., Niu L., Zhou H., Ghista D. (2017). Correlation between quantitative analysis of wall shear stress and intima-media thickness in atherosclerosis development in carotid arteries. Biomed. Eng. OnLine 16, 137. doi:10.1186/s12938-017-0425-9
Zhang L.-F. (2013). Region-specific vascular remodeling and its prevention by artificial gravity in weightless environment. Eur. J. Appl. Physiol. 113, 2873–2895. doi:10.1007/s00421-013-2597-8
Zhang L.-F., Sun B., Cao X.-S., Liu C., Yu Z.-B., Zhang L.-N., et al. (2003). Effectiveness of intermittent -Gx gravitation in preventing deconditioning due to simulated microgravity. J. Appl. Physiol. 95, 207–218. doi:10.1152/japplphysiol.00969.2002
Zhang R., Zuckerman J. H., Pawelczyk J. A., Levine B. D. (1997). Effects of head-down-tilt bed rest on cerebral hemodynamics during orthostatic stress. J. Appl. Physiol. 83, 2139–2145. doi:10.1152/jappl.1997.83.6.2139
Glossary
AA Ascending aorta
AGBRESA Artificial gravity bed rest with European space agency
BDC Baseline data collectionc
AG Continuous artificial gravity group
cAG Continuous artificial gravity group
CTRL Control group
DA Descending aorta
DLR Deutsches Zentrum für Luft- und Raumfahrt (German aerospace center)
ESA European space agency
g Earth’s gravitational acceleration
HDT Head-down tilt
iAG Intermittent artificial gravity group
LVET Left ventricular ejection time
MRI Magnetic resonance imaging
n Number of subjects
NASA National aeronautics and space administration
NO Nitric oxide
R Recovery
Keywords: bed rest, microgravity, artificial gravity, cardiac MRI, 4D flow, cardiovascular deconditioning, wall shear stress, pulse wave velocity
Citation: Rabineau J, Issertine M, Hoffmann F, Gerlach D, Caiani EG, Haut B, van de Borne P, Tank J and Migeotte P-F (2022) Cardiovascular deconditioning and impact of artificial gravity during 60-day head-down bed rest—Insights from 4D flow cardiac MRI. Front. Physiol. 13:944587. doi: 10.3389/fphys.2022.944587
Received: 15 May 2022; Accepted: 13 September 2022;
Published: 07 October 2022.
Edited by:
Marc-Antoine Custaud, Université d’Angers, FranceReviewed by:
Shereen M. Hamza, University of Alberta, CanadaJamila H. Siamwala, Brown University, United States
Copyright © 2022 Rabineau, Issertine, Hoffmann, Gerlach, Caiani, Haut, van de Borne, Tank and Migeotte. This is an open-access article distributed under the terms of the Creative Commons Attribution License (CC BY). The use, distribution or reproduction in other forums is permitted, provided the original author(s) and the copyright owner(s) are credited and that the original publication in this journal is cited, in accordance with accepted academic practice. No use, distribution or reproduction is permitted which does not comply with these terms.
*Correspondence: Jeremy Rabineau, SmVyZW15LnJhYmluZWF1QHVsYi5iZQ==