- 1Department of Biology, University of Ottawa, Ottawa, ON, Canada
- 2Brain and Mind Research Institute, University of Ottawa, ON, Ottawa, Canada
Despite the large body of work describing vertebrate ventilatory responses to hypoxia, remarkably little is known about the receptors and afferent pathways mediating these responses in fishes. In this review, we aim to summarize all receptor types to date implicated in the neurotransmission or neuromodulation associated with O2 sensing in the gills of fish. This includes serotonergic, cholinergic, purinergic, and dopaminergic receptor subtypes. Recent transcriptomic analysis of the gills of zebrafish using single-cell RNA sequencing has begun to elucidate specific receptor targets in the gill; however, the absence of receptor characterization at the cellular level in the gill remains a major limitation in understanding the neurochemical control of hypoxia signalling.
Introduction
Adequate oxygen delivery to tissue is important for maintaining cellular processes and homeostasis. Thus, an animal’s ability to detect low oxygen, and respond appropriately, is crucial for survival. Vertebrate respiratory chemoreceptors detect such chemical changes in the environment, or arterial blood supply, and upon stimulation initiate corrective autonomic reflexes, such as the hyperventilatory response (HVR) to hypoxia (Perry et al., 2009; Nurse, 2010; Jonz, 2018). The most well described vertebrate chemoreceptors are the type 1 (or glomus) cells of the mammalian carotid body. Hypoxic activation of these cells involves the modulation of K+ conductance, membrane depolarization, and resulting Ca2+-dependent neurotransmitter release to act on sensory terminals of the carotid sinus nerve (Lopez-Barneo et al., 1988; Gonzalez et al., 1994; Nurse, 2010; Kumar and Prabhakar, 2012).
The neurochemistry involved in transmitting the hypoxic signal in the carotid body is well characterized. Adenosine triphosphate (ATP) is released by type 1 cells to act on afferent terminal purinergic P2X3 receptors, and is one of the main excitatory neurotransmitters involved in hypoxic signalling (Zhang et al., 2000; Nurse, 2005). Adenosine, produced by the breakdown of extracellular ATP, acts on adenosine A2a receptors to further enhance the hypoxic response by type 1 cells (Nurse, 2014). Acetylcholine (ACh), co-released with ATP, is also largely responsible for excitatory signalling in the carotid body via post-synaptic nicotinic receptors (Zhang et al., 2000). Dopamine, acting on D2 receptors, plays a modulatory role in mediating the response to hypoxia in the carotid body (Benot and López-Barneo, 1990; Iturriaga and Alcayaga, 2004). Additionally, embedded within the respiratory epithelium of neonatal mammals lie clusters of chemosensory neuroepithelial bodies (NEBs), of which serotonin (5-hydroxytryptamine, 5-HT) is the major neurotransmitter released during hypoxia (Fu et al., 2002).
In contrast, remarkably little is known about the receptors and afferent pathways mediating HVR in fishes. In this review, we aim to summarize the available evidence that has linked specific receptor types in the gills to neurochemical signalling associated with O2 sensing in fish.
Fish Oxygen Chemoreceptors
Neuroepithelial cells (NECs) were first identified in the gill by Dunel-Erb et al. (1982), who noted a similar morphology to mammalian pulmonary chemoreceptors. NECs were characterized by the presence of dense-cored vesicles and contained the monoamine, serotonin (5-hydroxytrymptamine, 5-HT; Dunel Erb et al., 1982). Due to the homology between the carotid body and the first gill arch in fish, NECs are believed to be homologues of type 1 cells (Milsom and Burleson, 2007). More recent evidence has suggested they may have a closer evolutionary link to pulmonary NEBs (Hockman et al., 2017).
The first direct evidence for the involvement of gill NECs in O2 chemoreception came from studies using zebrafish (Danio rerio; Jonz et al., 2004). Using whole-cell patch-clamp recordings of NECs isolated from the gills, it was shown that NECs responded to a decreased PO2 by inhibition of background K+ channels and membrane depolarization. Subsequent studies showed similar O2 sensitivity of gill NECs in channel catfish (Ictalurus punctatus; Burleson et al., 2006), and isolated NECs from adult goldfish (Carassius auratus) responded to hypoxia by Ca2+-dependent vesicular recycling (Zachar et al., 2017), which is consistent with neurotransmitter release and activation of sensory nerve fibres to initiate the hypoxic response. Currently there is no direct evidence of the neurotransmitters released during hypoxia and the receptors they act on remain uncharacterized.
Receptor Control of Oxygen Sensing and Ventilation
Despite the lack of receptor characterization in the gill, there are numerous studies identifying neurotransmitters and neuroendocrine factors that may be associated with O2 sensing in the gills of fish (recently reviewed by Porteus et al., 2012; Pan and Perry, 2020). The following sections will summarize the major candidate receptor subtypes suggested to play a role in facilitating and mediating the ventilatory responses to hypoxia. These studies are summarized in Table 1.
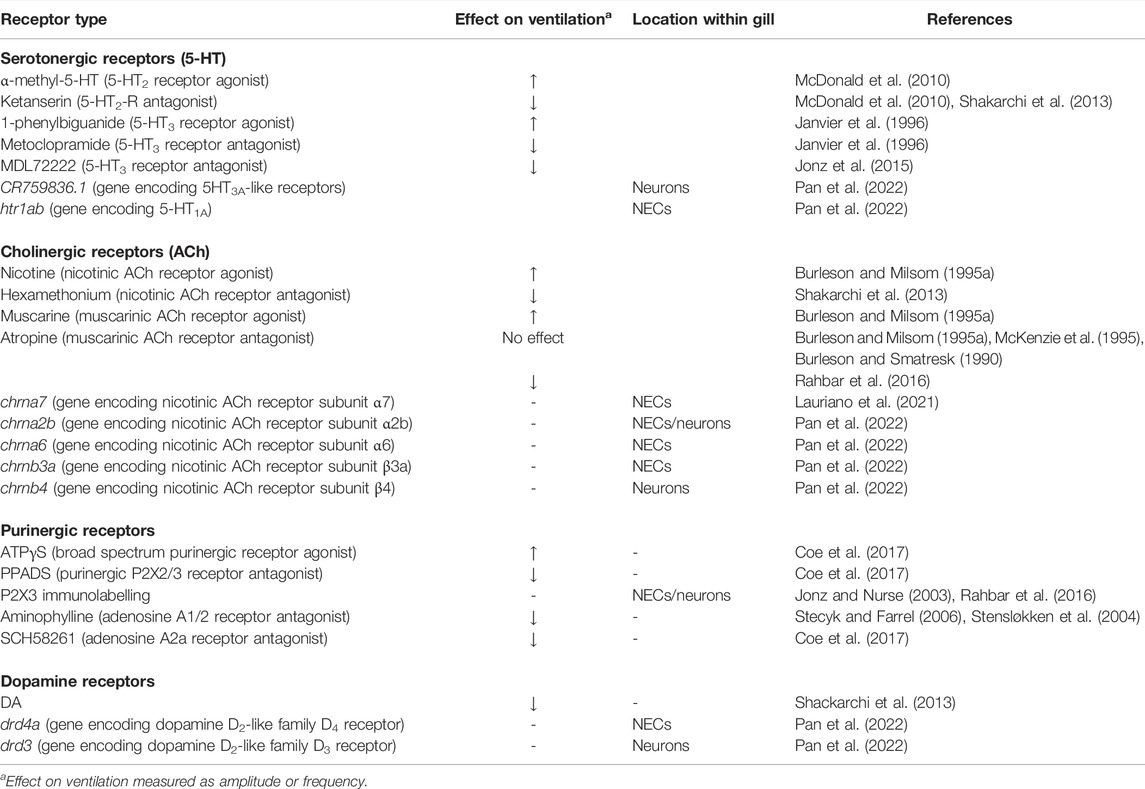
TABLE 1. Summary of receptor types reported to effect changes in ventilation amplitude and/or frequency and their location within the gill, if available. The first column indicates receptor type, as characterized by pharmacological studies, gene expression analysis or immunohistochemistry.
Serotonergic Receptors
Serotonergic receptors are divided into seven distinct classes, 5-HT1-7, largely based on their structural and operational characteristics within the serotonergic system (Peroutka and Howell, 1994; Hoyer et al., 2002). 5-HT receptors belong to the G-protein-coupled receptor (GPCR) superfamily, with the exception of the 5-HT3 receptor, which is a ligand-gated ion channel (Hoyer et al., 2002). Of the seven distinct classes, there are also a number of different 5-HT1-7 receptor subtypes, each having a distinct pattern of distribution and function in the nervous system, giving rise to many different signalling capabilities (reviewed by Barnes and Sharp, 1999). Some post-synaptic 5-HT receptor subtypes are known to cause neuronal depolarization (5-HT2A, 5-HT2C, 5-HT3 and 5-HT4 receptors) leading to excitatory signalling, or neuronal hyperpolarization (5-HT1A receptor) inhibiting signalling (Barnes and Sharp, 1999).
As NECs characteristically contain 5-HT, it has been long hypothesized that 5-HT is involved in the neurotransmission of the hypoxic signal. Early evidence of this came from isolated gills of rainbow trout (Oncorhynchus mykiss), where 5-HT elicited a modest transient burst of chemoreceptor activity (Burleson and Milsom, 1995a). Additionally, rainbow trout intra-arterial injections of 5-HT also caused an elevation in gill ventilation and heart rate (Burleson and Milsom, 1995b).
Pharmacological studies targeting specific 5-HT receptor subtypes have revealed a potential excitatory role of 5-HT2 and 5-HT3 receptors. In the European eel (Anguilla anguilla), intravenous administration of 5-HT elicited a large increase in ventilatory frequency and amplitude (Janvier et al., 1996). Janvier et al. also showed the 5-HT-induced hyperventilatory response could be mimicked by the 5-HT3 receptor agonist, 1-phenylbiguanide, and blocked by the 5-HT3 receptor antagonist, metoclopramide. In the Gulf toadfish (Opsanus beta), injection with the 5-HT2 receptor agonist, α-methyl-5-HT, increased ventilation amplitude and this response was attenuated by the 5-HT2 receptor antagonist, ketanserin (McDonald et al., 2010). Similarly, 5-HT mimicked hypoxia by increasing ventilation frequency of 7 days post-fertilization (d.p.f) zebrafish larvae, and subsequent addition of ketanserin reversibly reduced ventilation frequency (Shakarchi et al., 2013). In zebrafish larvae, 5-HT3 receptor blockade with tropanyl 3,5-dichlorobenzoate (MDL 72222) also reduced the hyperventilatory response to acute hypoxia (Jonz et al., 2015). These results suggest an excitatory role for 5-HT2 and 5-HT3 receptors; however, it is unknown if these effects were limited to the gills, as whole animal experiments do not exclude potential targets in the central nervous system (CNS). Additionally, in the gills of the Antarctic fish (Pagothenia borchgrevinki), branchial vasoconstriction was mediated by 5-HT2 receptors (Sundin et al., 1998); and previous studies demonstrated the innervation of the filament arteries by serotonergic neurons in trout and zebrafish (Bailly et al., 1989; Jonz and Nurse, 2003). Given the effects of hypoxia on blood flow in the gills (Booth, 1979), an additional role of 5-HT2 receptors in controlling vascular responses to hypoxia is conceivable.
Recent single-cell transcriptomic analysis of chemosensory NECs and other cell types of the gills of adult zebrafish has begun to provide compelling evidence for potential actions of these receptor types by localizing them to, and within, the gill. The gene encoding 5-HT3A-like receptors shows highest expression in gill neurons (Pan et al., 2022). As NECs are innervated by at least one population of intrabranchial neurons, as well as extrabranchial nerve fibres (Jonz et al., 2004), there is a plausible mechanism for chemotransduction via 5-HT3A receptors in the gill. This would be similar to the carotid body, where post-synaptic excitation during hypoxia is mediated primarily by ionotropic 5-HT3 receptors and, to a lesser extent, 5-HT2 (Zhong et al., 1999; Nurse, 2005). Further work localizing 5-HT3A receptors to the specific neuronal population innervating NECs would be needed to confirm this.
Interestingly, the gene encoding the inhibitory 5-HT1A receptor, htr1ab, was found to be most abundant in NECs above any other cell type in the gill (Pan et al., 2022). This may suggest a specific O2-sensing role for these receptors, possibly through a negative feedback or autoreceptor mechanism, which has not yet been explored pharmacologically.
Cholinergic Receptors
Cholinergic receptors are subdivided into nicotinic and muscarinic types. Nicotinic receptors (nAChRs) are ligand-gated ion channels formed by the assembly of five transmembrane subunits and mediate fast neurotransmission in the central and peripheral nervous systems (Ho et al., 2020). Many different nAChR subtypes exist, each consisting of a specific combination of subunits (α1-10, β1-4, γ, δ, and ε; Kalamida et al., 2007). Muscarinic receptors (mAChRs) are G-protein coupled receptors, of which five main subtypes exist (M1-5), and have actions in the central and peripheral nervous systems (Caulfield, 1993).
A role for ACh in gill neurotransmission is not as clear as in the mammalian carotid body. Non-serotonergic, NEC-like cells containing the vesicular ACh transporter, VAChT, have been identified in zebrafish (Shakarchi et al., 2013; Zachar et al., 2017) and mangrove rivulus (Kryptolebias marmoratus; Regan et al., 2011), though it is currently unclear if these cells function as O2 chemoreceptors. Ventilatory responses to ACh and nicotine have been reported in several species (Burleson and Milsom, 1995b; Shakarchi et al., 2013). The effects of muscarine, however, appear to be more uncertain. In rainbow trout, muscarine elicited moderate afferent nerve activity in gill arch preparations and increased ventilation frequency in whole animal experiments. The muscarinic antagonist, atropine, prevented stimulation by hypoxia; however, it had no effect on ventilation (Burleson and Milsom, 1995a; 1995b). Similarly, atropine had no effect on ventilatory responses to hypoxia in the Adriatic sturgeon (Acipenser naccarii; McKenzie et al., 1995) or the channel catfish (Burleson and Smatresk, 1990). In zebrafish, atropine abolished the ventilatory response to hypoxia, but only at very high concentrations (Rahbar et al., 2016). Administration of atropine also abolished the hypercarbia-induced ventilatory responses observed in Pacific spiny dogfish (Squalus acanthias; McKendry et al., 2001).
Additionally, there appears to be a time-dependent emergence of cholinergic control of ventilation during development. Exogenous application of ACh did not affect ventilation frequency in early stage zebrafish larvae (7–10 d.p.f); however, in late stage larvae (14–21 d.p.f) ACh had a stimulatory effect on ventilation frequency. The nicotinic ACh receptor antagonist, hexamethonium, did not inhibit hypoxia-induced hyperventilation at 10 d.p.f. but did after 12 d.p.f. (Shakarchi et al., 2013).
In the Asian catfish (Heteropneustes fossilis) the nicotinic receptor α7 subunit is expressed in the NECs and mucous cells in the gill and respiratory air sac (Lauriano et al., 2021). In addition, semi-quantitative PCR detected low expression of the AChR γ-like subunit in the gills of adult transparent Pristella maxillaris (Ma et al., 2021). RNA sequencing of the adult zebrafish gills has further narrowed down potential ACh receptor subunit targets within the gill. The nicotinic receptor α2b subunit gene, chrna2b, was highly expressed in NECs and neurons; whereas the α6 subunit gene (chrna6) and β3a subunit gene (chrnb3a) were expressed primarily in NECs, and the β4 subunit gene (chrnb4) was mainly present in neurons (Pan et al., 2022). The location of these subunits supports a model in which VAChT-positive cells release ACh during hypoxic stimulation, leading to excitatory post-synaptic or paracrine effects on ACh receptors of neurons or NECs (Pan et al., 2022). Like NECs, VAChT-positive cells of the zebrafish gills are closely apposed to nerve fibres (Zachar et al., 2017).
Purinergic Receptors
There are two families of purinergic receptors—P1 (adenosine) receptors, including four subtypes (A1, A2a, A2b and A3), and P2 (ATP) receptors, which are further subdivided into ionotropic P2X (P2X1-7) and metabotropic P2Y (P2Y1-12) receptors (reviewed by Burnstock, 2018). ATP is a major excitatory neurotransmitter in the mammalian carotid body (Zhang et al., 2000; Nurse, 2005), and adenosine, produced by the extracellular breakdown of ATP, enhances the excitatory response (Nurse, 2014). Such a role in the gills of fish has been less explored.
The broad-spectrum purinergic agonist, ATPγS, elicited a hyperventilatory response in zebrafish larvae. Further, the purinergic receptor antagonist, PPADS, which targets purinergic P2X2 and P2X3 receptors, inhibited the hyperventilatory response to hypoxia (Coe et al., 2017). Immunohistochemical staining of P2X3 receptors showed co-localization with NECs and 5-HT-positive neurons (Rahbar et al., 2016). P2X3 receptors were also found in 5-HT-positve cells in the tips of zebrafish lamellae (Jonz and Nurse, 2003).
In the common carp (Cyprinus carpio), purinergic blockade with aminophylline, an A1 and A2 receptor antagonist, reversed the increases in respiration rate that occurred with the onset of hypoxia (Stecyk and Farrell, 2006). Adenosine injection initiated a biphasic response in ventilation frequency (a decrease followed by an increase) in the epaulette shark (Hemiscyllium ocellatum), which was also blocked by aminophylline (Stensløkken et al., 2004). Moreover, in zebrafish, the A2a receptor antagonist, SCH58261, inhibited the ventilatory response to hypoxia (Coe et al., 2017).
The above studies provide evidence for an excitatory role of P2X2/3 and A1/2 receptors in control of the hyperventilatory response to hypoxia in fish. Further, these results suggest a similar mechanism to the carotid body, where pre- and post-synaptic A2a receptors are believed to enhance the response to hypoxia (Nurse, 2014).
Dopaminergic Receptors
Dopamine receptors are G-protein coupled and include the D1-like receptor subtypes (D1 and D5) which activate adenylyl cyclase, and the D2-like subfamily (D2, D3, and D4) which inhibit adenylyl cyclase and activate K+ channels (reviewed by Martel and Gatti McArthur, 2020). Dopamine is an important inhibitory neuromodulator in carotid body hypoxia signalling (Nurse, 2010). Dopamine released by type 1 cells has an autocrine-paracrine action on dopaminergic D2 receptors located on type 1 cells to inhibit Ca2+ channels, leading to negative feedback regulation of further neurotransmitter release during hypoxia (Benot and López-Barneo, 1990).
Early evidence for a role of dopamine in the gills was shown in isolated gills of rainbow trout, where dopamine caused a small and brief burst in chemoreceptor activity followed by a mild inhibition of receptor discharge (Burleson and Milsom, 1995a). In zebrafish larvae, exogenous application of dopamine has been shown to decrease ventilation frequency as early as 7 d.p.f. (Shakarchi et al., 2013). RNA sequencing of the adult zebrafish gill detected dopamine receptors in the D2-like family, including drd4a in NECs and drd3 highly expressed in neurons (Pan et al., 2022). This recent work using zebrafish suggests an inhibitory dopaminergic mechanism in the gill, possibly via D2-like receptors, and is an area for potential future developments. Pharmacologically targeting the specific dopamine receptor subtypes controlling the ventilatory responses to hypoxia may be an interesting extension of this work.
Conclusion
Since the initial discovery of gill NECs, much work has been done to identify the neurotransmitters and their respective receptor types involved in mediating the physiological responses to hypoxia. Recent RNA sequencing has begun to further localize some of these receptor subtypes to NECs and neurons within the gill; however, future work is needed to continue to localize specific receptor subtypes within the gill and provide physiological evidence of their involvement in O2 sensing.
Author Contributions
The manuscript was written by MR, and revised and edited by MR and MJ.
Funding
This research was supported by Natural Sciences and Engineering Research Council of Canada (NSERC) Grant No. 05571 to MJ.
Conflict of Interest
The authors declare that the research was conducted in the absence of any commercial or financial relationships that could be construed as a potential conflict of interest.
Publisher’s Note
All claims expressed in this article are solely those of the authors and do not necessarily represent those of their affiliated organizations, or those of the publisher, the editors and the reviewers. Any product that may be evaluated in this article, or claim that may be made by its manufacturer, is not guaranteed or endorsed by the publisher.
Acknowledgments
This review article was an invited contribution for the special issue, Insights in Aquatic Physiology: 2021. We thank the topic editors for this invitation.
References
Bailly Y., Dunel-Erb S., Geffard M., Laurent P. (1989). The Vascular and Epithelial Serotonergic Innervation of the Actinopterygian Gill Filament with Special Reference to the Trout, Salmo Gairdneri. Cell Tissue Res. 258, 349–363. doi:10.1007/BF00239455
Barnes N. M., Sharp T. (1999). A Review of Central 5-HT Receptors and Their Function. Neuropharmacology 38, 1083–1152. doi:10.1016/S0028-3908(99)00010-6
Benot A. R., Lopez-Barneo J. (1990). Feedback Inhibition of Ca2+ Currents by Dopamine in Glomus Cells of the Carotid Body. Eur. J. Neurosci. 2, 809–812. doi:10.1111/j.1460-9568.1990.tb00473.x
Booth J. H. (1979). The Effects of Oxygen Supply, Epinephrine, and Acetylcholine on the Distribution of Blood Flow in Trout Gills. J. Exp. Biol. 83, 31–39. doi:10.1242/jeb.83.1.31
Burleson M. L., Milsom W. K. (1995b). Cardio-ventilatory Control in Rainbow Trout: II. Reflex Effects of Exogenous Neurochemicals. Respir. Physiol. 101, 289–299. doi:10.1016/0034-5687(95)00029-D
Burleson M. L., Milsom W. K. (1995a). Cardio-ventilatory Control in Rainbow Trout: I. Pharmacology of Branchial, Oxygen-Sensitive Chemoreceptors. Respir. Physiol. 100, 231–238. doi:10.1016/0034-5687(95)91595-X
Burleson M. L., Mercer S. E., Wilk-Blaszczak M. A. (2006). Isolation and Characterization of Putative O2 Chemoreceptor Cells from the Gills of Channel Catfish (Ictalurus punctatus). Brain Res. 1092, 100–107. doi:10.1016/J.BRAINRES.2006.03.085
Burleson M. L., Smatresk N. J. (1990). Evidence for Two Oxygen-Sensitive Chemoreceptor Loci in Channel Catfish,Ictalurus punctatus. Physiol. Zool. 63, 208–221. doi:10.1086/physzool.63.1.30158162
Burnstock G. (2018). Purine and Purinergic Receptors. Brain Neurosci. Adv. 2, 2398212818817494. doi:10.1177/2398212818817494
Caulfield M. P. (1993). Muscarinic Receptors-Characterization, Coupling and Function. Pharmacol. Ther. 58, 319–379. doi:10.1016/0163-7258(93)90027-B
Coe A. J., Picard A. J., Jonz M. G. (2017). Purinergic and Adenosine Receptors Contribute to Hypoxic Hyperventilation in Zebrafish (Danio rerio). Comp. Biochem. Physiology Part A Mol. Integr. Physiology 214, 50–57. doi:10.1016/j.cbpa.2017.09.013
Dunel-Erb S., Bailly Y., Laurent P. (1982). Neuroepithelial Cells in Fish Gill Primary Lamellae. J. Appl. Physiology 53, 1342–1353. doi:10.1152/jappl.1982.53.6.1342
Fu X. W., Nurse C. A., Wong V., Cutz E. (2002). Hypoxia-induced Secretion of Serotonin from Intact Pulmonary Neuroepithelial Bodies in Neonatal Rabbit. J. Physiology 539, 503–510. doi:10.1113/jphysiol.2001.013071
Gonzalez C., Almaraz L., Obeso A., Rigual R. (1994). Carotid Body Chemoreceptors: from Natural Stimuli to Sensory Discharges. Physiol. Rev. 74, 829–898. doi:10.1152/physrev.1994.74.4.829
Ho T. N. T., Abraham N., Lewis R. J. (2020). Structure-Function of Neuronal Nicotinic Acetylcholine Receptor Inhibitors Derived from Natural Toxins. Front. Neurosci. 14. 609005. Available at: https://www.frontiersin.org/article/10.3389/fnins.2020.609005.doi:10.3389/fnins.2020.609005
Hockman D., Burns A. J., Schlosser G., Gates K. P., Jevans B., Mongera A., et al. (2017). Evolution of the Hypoxia-Sensitive Cells Involved in Amniote Respiratory Reflexes. Elife 6, 1–28. doi:10.7554/eLife.21231
Hoyer D., Hannon J. P., Martin G. R. (2002). Molecular, Pharmacological and Functional Diversity of 5-HT Receptors. Pharmacol. Biochem. Behav. 71, 533–554. doi:10.1016/S0091-3057(01)00746-8
Iturriaga R., Alcayaga J. (2004). Neurotransmission in the Carotid Body: Transmitters and Modulators between Glomus Cells and Petrosal Ganglion Nerve Terminals. Brain Res. Rev. 47, 46–53. doi:10.1016/j.brainresrev.2004.05.007
Janvier J.-J., Peyraud-Waïtzenegger M., Soulier P. (1996). Mediation of Serotonin-Induced Hyperventilation via 5-HT3-Receptor in European Eel Anguilla anguilla. J. Comp. Physiol. B 165, 640–646. doi:10.1007/BF00301132
Jonz M. G., Fearon I. M., Nurse C. A. (2004). Neuroepithelial Oxygen Chemoreceptors of the Zebrafish Gill. J. Physiol. 560, 737–752. doi:10.1113/jphysiol.2004.069294
Jonz M. G. (2018). Insights into the Evolution of Polymodal Chemoreceptors. Acta Histochem. 120, 623–629. doi:10.1016/j.acthis.2018.08.008
Jonz M. G., Nurse C. A. (2003). Neuroepithelial Cells and Associated Innervation of the Zebrafish Gill: A Confocal Immunofluorescence Study. J. Comp. Neurol. 461, 1–17. doi:10.1002/cne.10680
Jonz M. G., Zachar P. C., Da Fonte D. F., Mierzwa A. S. (2015). Peripheral Chemoreceptors in Fish: A Brief History and a Look Ahead. Comp. Biochem. Physiology Part A Mol. Integr. Physiology 186, 27–38. doi:10.1016/j.cbpa.2014.09.002
Kalamida D., Poulas K., Avramopoulou V., Fostieri E., Lagoumintzis G., Lazaridis K., et al. (2007). Muscle and Neuronal Nicotinic Acetylcholine Receptors. FEBS J. 274, 3799–3845. doi:10.1111/j.1742-4658.2007.05935.x
Kumar P., Prabhakar N. R. (2012). Peripheral Chemoreceptors: Function and Plasticity of the Carotid Body. Compr. Physiol. 2, 141–219. doi:10.1002/cphy.c100069
Lauriano E. R., Capillo G., Icardo J. M., Fernandes J. M. O., Kiron V., Kuciel M., et al. (2021). Neuroepithelial Cells (NECs) and Mucous Cells Express a Variety of Neurotransmitters and Neurotransmitter Receptors in the Gill and Respiratory Air-Sac of the Catfish Heteropneustes Fossilis (Siluriformes, Heteropneustidae): a Possible Role in Local Immune Defence. Zoology 148, 125958. doi:10.1016/J.ZOOL.2021.125958
López-Barneo J., López-López J. R., Ureña J., Gonzalez C. (1988). Chemotransduction in the Carotid Body: K + Current Modulated by P O 2 in Type I Chemoreceptor Cells. Science 241 (4865), 580–582. doi:10.1126/science.2456613
Ma K., Chen Y., Zhou L., Liu Z., Liu Z. (2021). Cloning and Characterization of Nicotinic Acetylcholine Receptor γ-like Gene in Adult Transparent Pristella Maxillaris. Gene 769, 145193. doi:10.1016/J.GENE.2020.145193
Martel J. C., Gatti McArthur S. (2020). Dopamine Receptor Subtypes, Physiology and Pharmacology: New Ligands and Concepts in Schizophrenia. Front. Pharmacol. 11, 1003. Available at:. doi:10.3389/fphar.2020.01003https://www.frontiersin.org/article/10.3389/fphar.2020.01003
McDonald M. D., Gilmour K. M., Walsh P. J., Perry S. F. (2010). Cardiovascular and Respiratory Reflexes of the Gulf Toadfish (Opsanus beta) during Acute Hypoxia. Respir. Physiology Neurobiol. 170, 59–66. doi:10.1016/j.resp.2009.12.012
McKendry J. E., Milsom W. K., Perry S. F. (2001). Branchial CO(2) Receptors and Cardiorespiratory Adjustments during Hypercarbia in Pacific Spiny Dogfish (Squalus acanthias). J. Exp. Biol. 204, 1519–1527. doi:10.1242/jeb.204.8.1519
McKenzie D. J., Taylor E. W., Bronzi P., Bolis C. L. (1995). Aspects of Cardioventilatory Control in the Adriatic Sturgeon (Acipenser naccarii). Respir. Physiol. 100, 45–53. doi:10.1016/0034-5687(94)00121-F
Milsom W. K., Burleson M. L. (2007). Peripheral Arterial Chemoreceptors and the Evolution of the Carotid Body. Respir. Physiology Neurobiol. 157, 4–11. doi:10.1016/J.RESP.2007.02.007
Nurse C. A. (2005). Neurotransmission and Neuromodulation in the Chemosensory Carotid Body. Aut. Neurosci. 120, 1–9. doi:10.1016/J.AUTNEU.2005.04.008
Nurse C. A. (2010). Neurotransmitter and Neuromodulatory Mechanisms at Peripheral Arterial Chemoreceptors. Exp. Physiol. 95, 657–667. doi:10.1113/expphysiol.2009.049312
Nurse C. A. (2014). Synaptic and Paracrine Mechanisms at Carotid Body Arterial Chemoreceptors. J. Physiol. 592, 3419–3426. doi:10.1113/jphysiol.2013.269829
Pan W., Godoy R. S., Cook D. P., Scott A. L., Nurse C. A., Jonz M. G. (2022). Single-cell Transcriptomic Analysis of Neuroepithelial Cells and Other Cell Types of the Gills of Zebrafish (Danio rerio) Exposed to Hypoxia. Sci. Rep. 12(1):10144. doi:10.1038/s41598-022-13693-1Accepted 26 May
Pan Y. K., Perry S. F. (2020). Neuroendocrine Control of Breathing in Fish. Mol. Cell. Endocrinol. 509, 110800. doi:10.1016/j.mce.2020.110800
Peroutka S. J., Howell T. A. (1994). The Molecular Evolution of G Protein-Coupled Receptors: Focus on 5-hydroxytryptamine Receptors. Neuropharmacology 33, 319–324. doi:10.1016/0028-3908(94)90060-4
Perry S. F., Jonz M. G., Gilmour K. M. (2009). “Chapter 5 Oxygen Sensing and the Hypoxic Ventilatory Response,” Fish Physiology. 27, 193–253. doi:10.1016/S1546-5098(08)00005-8
Porteus C. S., Brink D. L., Milsom W. K. (2012). Neurotransmitter Profiles in Fish Gills: Putative Gill Oxygen Chemoreceptors. Respir. Physiology Neurobiol. 184, 316–325. doi:10.1016/J.RESP.2012.06.019
Rahbar S., Pan W., Jonz M. G. (2016). Purinergic and Cholinergic Drugs Mediate Hyperventilation in Zebrafish: Evidence from a Novel Chemical Screen. PLoS One 11, e0154261. doi:10.1371/journal.pone.0154261
Regan K. S., Jonz M. G., Wright P. A. (2011). Neuroepithelial Cells and the Hypoxia Emersion Response in the Amphibious fishKryptolebias Marmoratus. J. Exp. Biol. 214, 2560–2568. doi:10.1242/jeb.056333
Shakarchi K., Zachar P. C., Jonz M. G. (2013). Serotonergic and Cholinergic Elements of the Hypoxic Ventilatory Response in Developing Zebrafish. J. Exp. Biol. 216, 869–880. doi:10.1242/jeb.079657
Stecyk J. A. W., Farrell A. P. (2006). Regulation of the Cardiorespiratory System of Common Carp (Cyprinus carpio) during Severe Hypoxia at Three Seasonal Acclimation Temperatures. Physiological Biochem. Zoology 79, 614–627. doi:10.1086/501064
Stensløkken K.-O., Sundin L., Renshaw G. M. C., Nilsson G. E. (2004). Adenosinergic and Cholinergic Control Mechanisms during Hypoxia in the Epaulette Shark (Hemiscyllium ocellatum), with Emphasis on Branchial Circulation. J. Exp. Biol. 207, 4451–4461. doi:10.1242/jeb.01291
Sundin L., Davison W., Forster M., Axelsson M. (1998). A Role of 5-HT2 Receptors in the Gill Vasculature of the Antarctic Fish Pagothenia Borchgrevinki. J. Exp. Biol. 201, 2129–2138. doi:10.1242/jeb.201.14.2129
Zachar P. C., Pan W., Jonz M. G. (2017). Characterization of Ion Channels and O2 Sensitivity in Gill Neuroepithelial Cells of the Anoxia-Tolerant Goldfish (Carassius auratus). J. Neurophysiology 118, 3014–3023. doi:10.1152/jn.00237.2017
Zhang M., Zhong H., Vollmer C., Nurse C. A. (2000). Co-release of ATP and ACh Mediates Hypoxic Signalling at Rat Carotid Body Chemoreceptors. J. Physiology 525, 143–158. doi:10.1111/j.1469-7793.2000.t01-1-00143.x
Keywords: neuroepithelial cell, chemoreception, serotonergic receptors, hypoxia, cholinergic receptors, zebrafish
Citation: Reed M and Jonz MG (2022) Neurochemical Signalling Associated With Gill Oxygen Sensing and Ventilation: A Receptor Focused Mini-Review. Front. Physiol. 13:940020. doi: 10.3389/fphys.2022.940020
Received: 09 May 2022; Accepted: 21 June 2022;
Published: 13 July 2022.
Edited by:
Pung Pung Hwang, Academia Sinica, TaiwanReviewed by:
Thorsten Schwerte, University of Innsbruck, AustriaM. Danielle McDonald, University of Miami, United States
Copyright © 2022 Reed and Jonz. This is an open-access article distributed under the terms of the Creative Commons Attribution License (CC BY). The use, distribution or reproduction in other forums is permitted, provided the original author(s) and the copyright owner(s) are credited and that the original publication in this journal is cited, in accordance with accepted academic practice. No use, distribution or reproduction is permitted which does not comply with these terms.
*Correspondence: Michael G. Jonz, bWpvbnpAdW90dGF3YS5jYQ==