- 1Department of Otolaryngology, Shandong Provincial Hospital Affiliated to Shandong First Medical University, Jinan, China
- 2Department of Thyroid and Breast Surgery, Shandong Provincial Third Hospital, Jinan, China
Objectives: Obstructive sleep apnea (OSA) is an independent risk factor for metabolic syndrome (MetS). Recent studies have indicated that circadian clock genes were dysregulated in OSA. In addition, it is clear that the impairment of circadian clocks drives the progression of MetS. Therefore, we hypothesized that circadian rhythm disruption links OSA with MetS.
Methods: A total of 118 participants, who underwent polysomnography (PSG) and were diagnosed as healthy snorers (control, n = 29) or OSA (n = 89) patients based on the apnea–hypopnea index (AHI), were enrolled in the present study. General information, anthropometric data, blood biochemical indicators, clock gene expressions, and levels of oxidative and inflammatory indicators were collected, determined, and compared in all the participants.
Results: We found that Brain and muscle aryl hydrocarbon receptor nuclear translocator-like protein 1 (Bmal1) and Differentiated embryo chondrocyte 1 (Dec1) were upregulated, while Period 1 (Per1) was reduced in OSA patients. In addition, these changing trends were closely associated with the hypoxia indicator of AHI and have a significant impact on the presence of MetS components, such as hyperglycemia (Dec1 and Per1, p < 0.05 and 0.001, respectively), hypertension (Bmal1 and Dec1, p < 0.001 and 0.01, respectively), hyperlipidemia (Dec1, p < 0.01), and obesity (Dec1, p < 0.05). Notably, expressions of Dec1 correlated with IR and predicted the presence of MetS in OSA patients. Finally, we also observed that Dec1 expression was interrelated with levels of both oxidative indicators and inflammatory biomarkers (IL-6) in OSA.
Conclusion: This study concluded that circadian clock disruptions, especially Dec1, link OSA with MetS in an oxidative and inflammatory-related manner. Circadian clock Dec1 can be used as a specific biomarker (p < 0.001) and therapeutic target in OSA combined with Mets patients.
Introduction
Obstructive sleep apnea (OSA), characterized by the recurrent complete or partial collapse of the upper airway during sleep, is the most prevalent hypoxia-related disorder worldwide (Benjafield et al., 2019). Chronic intermittent hypoxemia (CIH) is one of the hallmarks of this disease and leads to oxidative stress and inflammatory reaction, which are the potential pathogenesis of endothelial dysfunctions and metabolic disorders (Yamauchi et al., 2005; Gao et al., 2021; Makhout et al., 2021; Maniaci et al., 2021; Ruaro et al., 2021; Cai et al., 2022). Therefore, it is not surprising that OSA has been found to be independently associated with not only cardiovascular or cardiometabolic diseases but also tumors, and neurodegenerative or musculoskeletal disorders (Hirotsu et al., 2018; Benjafield et al., 2019). Among these, metabolic syndrome (MetS) is a clustering of cardiometabolic risk factors that include obesity, dyslipidemia, hyperglycemia, and hypertension. More than half of the OSA combined with MetS and vice versa (Hirotsu et al., 2018). Indeed, emerging evidence suggests the complex pathophysiological interactions between OSA and MetS, mainly including insulin resistance (IR) and endothelial dysfunction (Murphy et al., 2017; Hirotsu et al., 2018). Given nocturnal hypoxemia significantly increases the cardiometabolic risks in the population, understanding these physiological perturbations promoted by the OSA is so paramount as that could help to find risk indicators and early therapeutic targets for this common disorder.
Circadian clocks are time- and tissue-specific output genes, which manage the behavioral and molecular processes to synchronize with environmental changes in light and nutrients (Bass and Lazar, 2016). These clock-controlled genes include members of Brain and muscle aryl hydrocarbon receptor nuclear translocator-like protein (BMAL1 and BMAL2), Circadian locomotor output cycles kaput (CLOCK), Cryptochrome (CRY1 and CRY2), Period (PER1-3), Differentiated embryo chondrocyte (DEC), the reverse strand of ERB (REV-ERBα and REV-ERBβ), and kinases (e.g., CSNK1E) (Takahashi, 2017). Recent studies have identified the potential effect of OSA on the disruption of these biological clocks (Burioka et al., 2008; Hou et al., 2019; Yang et al., 2019; Gabryelska et al., 2020; Gaspar et al., 2021). Furthermore, the misalignment of these components is intimately associated with metabolic diseases, such as hypertension, diabetes, obesity, and metabolic syndrome (Yun et al., 2002; Ando et al., 2011; Chen et al., 2015; Chang et al., 2018; Nakashima et al., 2018; Noshiro et al., 2018; Yu et al., 2019; Noshiro et al., 2020; Parameswaran and Ray, 2022). Based on these clues, it is conceivable that OSA-induced disruption of circadian clocks may contribute to its combined metabolic diseases.
Both OSA and MetS are closely associated with oxidative stress and systemic inflammation, which were critical physiological rhythms regulated by the clock genes (Gabryelska et al., 2022). As an independent risk factor for MetS, OSA patients are often in a state of IR and endothelial dysfunction (Stenvers et al., 2019). We speculated that disrupted clock proteins might impact the inflammation and oxidative stress in OSA patients, which in turn facilitated IR and endothelial dysfunction of MetS. However, there is no report regarding the role of circadian clocks in the relationship between OSA and MetS. Therefore, the study aimed to evaluate how biomarkers of oxidative stress and systemic inflammation are linked with clock genes, and further determine whether circadian clock disruptions contribute to the presence of MetS components in OSA patients.
Materials and methods
Study subjects
We conducted an observational non-randomized cohort study in Shandong Provincial Hospital Affiliated with Shandong First Medical University. This study was approved by the local ethics committees and was in accordance with the Declaration of Helsinki. All participants provided written informed consent before enrollment. The prevalence of OSA is predominantly male and clock rhythm changes by gender; we therefore, choose a male participant for further investigation. A total of 235 male participants were recruited due to suspected OSA and were recommended for overnight polysomnography (PSG) in the Sleep Center of the Department of Otolaryngology between July 2019 and June 2021. Finally, 117 cases were excluded and 118 cases were selected as eligible participants. A detailed flowchart is shown in Figure 1.
The apnea–hypopnea index (AHI) was defined as the number of apnea and hypopnea events per hour during sleep, according to the overnight PSG results. Based on the severity of the apnea–hypopnea index (AHI), 118 eligible participants were divided into control (healthy snorers, AHI <5 events/h, n = 29) and OSA groups (AHI ≥5 events/h, n = 89). The OSA patients were further classified into mild (5–14.9 events/h, n = 41), moderate (15–29.9 events/h, n = 28), and severe (AHI ≥30 events/h, n = 20) OSA subgroups.
Clinical data collection
General information of the participants, such as age and BMI (weight in kg/height2 in meter), were collected from the medical records. Anthropometric data, such as systolic blood pressure (SBP) and diastolic blood pressure (DBP), were measured by a digital sphygmomanometer according to a standardized protocol. Blood biochemical indicators, such as glycosylated hemoglobin (HbA1c), fasting blood glucose (FBG), fasting insulin (FIns), homeostasis model assessment of IR (HOMA-IR, FBG in mmol/L × fasting insulin in mU/L/22.5), triglyceride (TG), high-density lipoprotein (HDL), and 12-cytokines (IL-5, INF-α, IL-2, IL-2, IN-6, IL-1β, IL-10, IL-γ, IL-8, IL-17, IL-4, IL-12P70, and TNF-α) were measured by the hospital laboratory department according to routine procedures.
MetS assessment
According to the diagnostic criteria for MetS by the Chinese Medical Association Diabetes Branch (Lu et al., 2006), the OSA patients were further assigned to OSA without MetS (OSA-non-MetS, n = 39) and OSA with MetS (OSA-MetS, n = 50) subgroups. In brief, any three of the four following risk factors were identified as MetS: 1) BMI ≥25; 2) FPG ≥6.1 mmol/L, and (or) 2-hour postprandial blood glucose ≥7.8 mmol/L; and (or) validated diabetes; and/or undergoing hypoglycemic therapy; 3) SBP/DBP ≥130/85 mmHg, and (or) confirmed hypertension or in those on anti-hypertensive treatment; and 4) fasting TG ≥ 1.7 mmol/L and (or) high-density lipoprotein cholesterol (HDL-C) <1.04 mmol/L.
Blood sample processing
For evaluating expression levels of the clock genes and estimation of the oxidative stress status, fasting blood samples (15–20 ml) were obtained from the antecubital vein of the OSA or control patients, and collected in a vacutainer (EDTA tube) at 7:00 a.m. after overnight PSG. Then, blood tubes were sampled by centrifugation (800 g, 20 min, at room temperature). First, the plasma samples were obtained and stored at −80°C until analysis for oxidative detection. Next, peripheral blood mononuclear cells (PBMCs) were collected from the interface bands and subsampled into a clean falcon tube, stored at −80°C for further assays, and thawed at 4°C prior to use, as reported in a previous study (Gaspar et al., 2021).
Assessment of clock gene expressions by RT-PCR
The total RNA of PBMCs was extracted and purified as recommended by the instructions of the Trizol reagent (Invitrogen). Relative mRNA expressions of the clock genes, such as Bmal1, Clock, Cry1, Cry2, CSNK1E, Dec1, NR1D1, Per1, Per2, and Per3, were evaluated by the real-time quantitative reverse transcriptase polymerase chain (qRT-PCR) as reported previously (Xiao et al., 2019). Primer sequences obtained from Sangon Biotech Co., (Shanghai, China) for the qRT-PCR amplification are listed in Supplementary Table S1. Evaluation of the relative mRNA expressions was performed by the 2−△△CT method (Teng et al., 2012). Fold change of relative mRNA levels was calculated as the average fold change of the OSA samples compared to that of the control samples. The experiments were repeated three times.
Oxidative stress measurements
Oxidative stress index was evaluated by the detection of the total antioxidant status (TAS), total oxidative status (TOS), and oxidative stress index (OSI) in plasma samples from all subjects. Measurements of TAS and TOS were performed by the standard colorimetric and spectrophotometric methods, using a commercially available diagnostic ImAnOx (TAS/TAC) Kit (Immundiagnostik, Bensheim, Germany). OSI was calculated as a ratio of TOS to TAS.
Statistical analysis
Statistical analyses were performed using Graph Pad Prism 6.00 (GraphPad Software, Inc.,). Data were shown as the mean ± standard deviation (SD) and analyzed using Student’s t-test. Normal distribution of data was determined by the Kolmogorov–Smirnov test. Variables not normally distributed were logarithmically transformed prior to analyses. Correlations of clock gene expressions with AHI, inflammatory markers, or oxidative indicators were assessed by Pearson’s correlation analysis. Based on expression levels of clock genes, receiver-operating characteristic (ROC) curves were calculated to predict the presence of MetS or IR in OSA patients. An area under curve (AUC) of 0.5 represents no predictive power, whereas an AUC of 1 represents perfect diagnostic accuracy. p-value < 0.05 was considered as statistically significant.
Results
OSA increases the presence of MetS components
A total of 89 OSA patients (39 cases of OSA-non-MetS and 50 cases of OSA-MetS) and 29 healthy snorers (control) were included in the present study, according to the PSG results and diagnostic criteria for MetS. Characteristics of the anthropometric, biochemical, and metabolic data are shown and compared in Table 1. No significant differences were observed in age distribution between the subgroups. As expected, AHI, mean SaO2%, and monitoring time spent with oxygen saturation <90% were significantly increased in the OSA-MetS group compared with the OSA-non-MetS group. In addition, BMI, SBP, DBP, HbA1c (%), FPG, FIns, HOMA-IR, TG, and HDL-C in the OSA-MetS groups were much higher than those of the OSA-non-MetS group. Notably, clinical parameters, such as BMI, HbA1c (%), FIns, and HOMA-IR in the OSA group, were higher than those of the control group. Other clinical parameters showed no significant difference between these subgroups. Collectively, these data revealed that a patient with OSA has more frequently MetS components.
OSA dysregulated the circadian clocks
Circadian clock disruptions had been considered as a hallmark of OSA and are reported to play a key role in regulating the pathogenesis of MetS (Burioka et al., 2008; Bass and Lazar, 2016; Yang et al., 2019; Gabryelska et al., 2020; Gaspar et al., 2021). We speculated that some of the major clock alterations may be the key link between OSA and MetS. Therefore, we assessed concentrations of the clock outputs in PBMCs by RT-PCR in the study cohort and further investigated the effect of OSA on the biological clock. The results are shown in Figure 2. Compared with the control group, levels of Baml1 and Dec1 were significantly increased, while that of Per1 was markedly decreased in the OSA group. However, no significant differences were observed for expression levels of Clock, Cry1, Cry2, CSNK1E, NR1D1, Per2, and Per3 between the control and OSA groups. These results indicated that the expression of Bmal1, Dec1, and Per1 in PBMCs is altered in OSA patients.
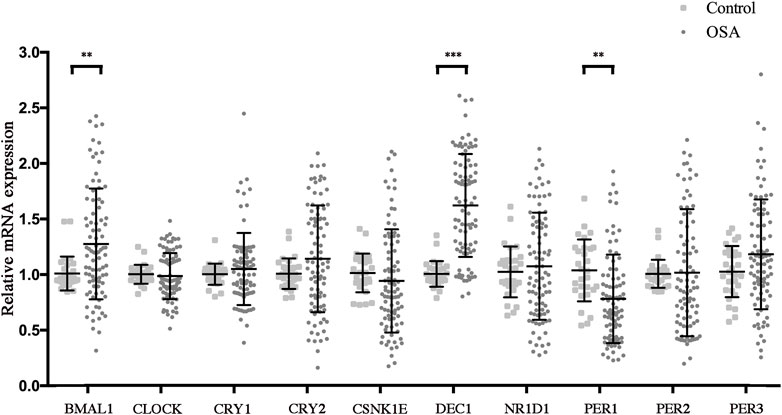
FIGURE 2. OSA dysregulated the circadian clocks. Relative mRNA expressions of circadian clock genes in the peripheral blood mononuclear cells from control and OSA patients. Mean values from the control group were set at 1.0. Experiments were repeated three independent times. **, p < 0.01; ***, p < 0.001.
CIH is associated with the disrupted BMAL1, Dec1, and Per1 in OSA
To further investigate the effect of hypoxia on alternations of clock genes, we further compared the relative mRNA expressions of Bmal1, Dec1, and Per1 in control and OSA patients. As shown in Figure 3A, mRNA expressions of Bmal1 were significantly higher in the severe OSA patients than mRNA expressions of Bmal1 in the mild or moderate OSA patients (p < 0.001 for both). Similarly, mRNA expressions of Dec1 also increased with the severity of OSA (mild vs. moderate, p < 0.001; moderate vs. severe, p < 0.01). However, Per1 mRNA expression was significantly lower in the severe OSA than Per1 mRNA expression in mild OSA (p < 0.05). No significant differences were found for mRNA expressions of Bmal1, Dec1, and Per1 between control and mild OSA groups. We next performed the linear regression analyses to determine associations of AHI with expression levels of BMAL1, Dec1, and Per1 in OSA patients. As shown in Figures 3B,C, both Baml1 and Dec1 were positively correlated with the AHI (r = 0.343, p = 0.001 for Bmal1; r = 0.480 p < 0.001 for Dec1). However, increased AHI conferred a gentler decreasing trend for Per1 mRNA expressions in OSA (r = −0.227, p = 0.032).
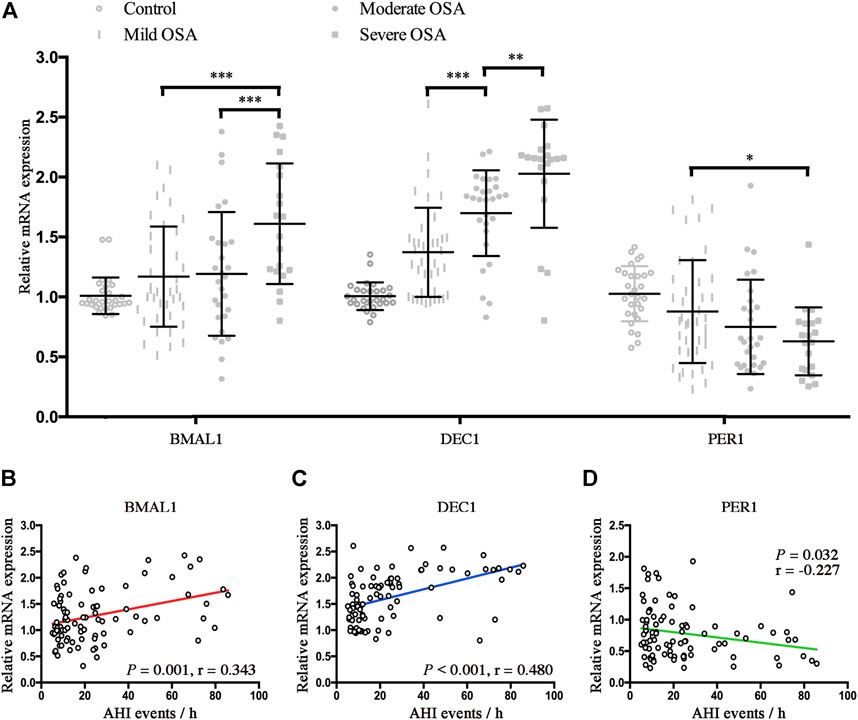
FIGURE 3. CIH is associated with the disrupted BMAL1, Dec1, and Per1 in OSA. (A) Expression levels of the disrupted clock genes (Bmal1, Dec1, and Per1) in the peripheral blood mononuclear cells from control and OSA subgroups (mild, moderate, and severe). (B–D) Linear regression between expression levels of Baml1 (B), Dec1 (C), Per1 (D), and AHI. *, p < 0.05; **, p < 0.01; ***, p < 0.001.
Disrupted clock genes are risk factors for the presence of MetS components in OSA
To determine the effect of the disrupted clocks on MetS components, we compared mRNA levels of Bmal1, Dec1, and Per1 in OSA patients with or without hyperglycemia, hypertension, hyperlipidemia, and obesity, which were considered as the major components of MetS. In OSA with hyperglycemia (fasting glucose ≥6.1 mmol/L) patients, mRNA levels of Dec1 were higher (p < 0.05), and those of Per1 were lower (p < 0.001) than those of OSA without hyperglycemia patients (fasting glucose<6.1 mmol/L). No significant differences in mRNA expressions of Bmal1 were found between OSA with and without hyperglycemia groups (Figure 4A). We also found mRNA levels of Bmal1 and Dec1 were significantly increased in the OSA with hypertension group (blood pressure ≥130/85 mmHg) compared to the OSA without hypertension group (blood pressure <130/85 mmHg, p < 0.001 for Bmal1 and p < 0.01 for Dec1), while no significant difference for Per1 was found between these two groups (Figure 4B). In addition, Dec1 mRNA expression was much higher in OSA with hyperlipidemia (triglycerides ≥1.7 mmol/L or HDL cholesterol <1.4 mmol/L, p < 0.01) or obesity (BMI ≥25, p < 0.05) comparing to that of the control groups (Figures 4C,D). Collectively, all these data indicated that OSA-disrupted expressions of Baml1, Dec1, and Per1 are involved in the pathogenesis of MetS components.
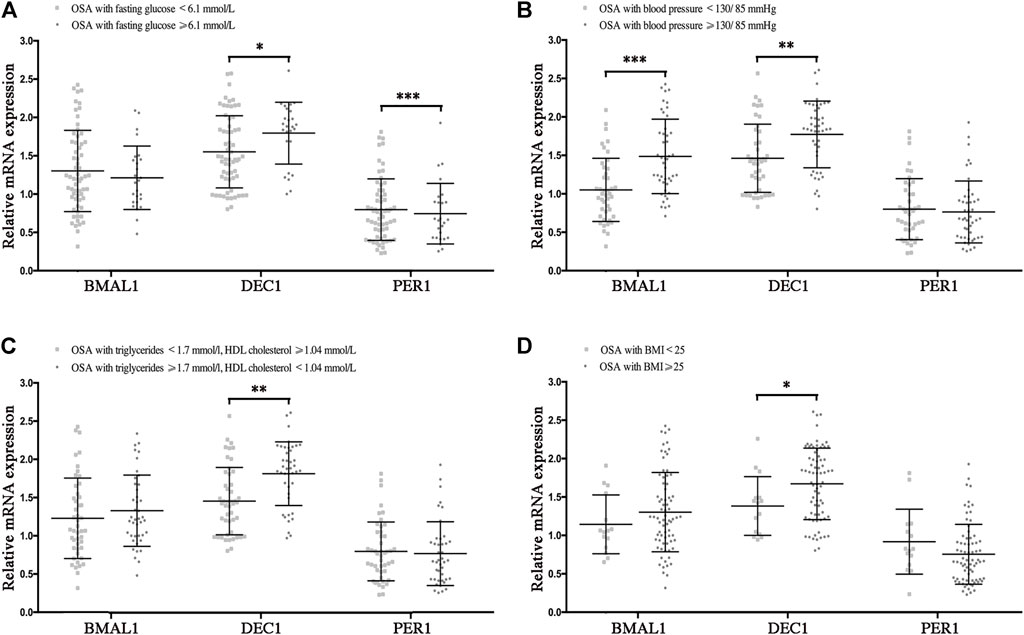
FIGURE 4. Disrupted clock genes are risk factors for the presence of MetS components in OSA. Expression levels of the disrupted clock genes (Bmal1, Dec1, and Per1) in the peripheral blood mononuclear cells from the following indicated OSA subgroups: (A) fasting glucose<6.1 mmol/L vs. fasting glucose ≥6.1 mmol/L; (B) blood pressure <130/85 mmHg vs. blood pressure ≥130/85 mmHg; (C) triglycerides <1.7 mmol/L and HDL cholesterol ≥1.4 mmol/L vs. triglycerides ≥1.7 mmol/L or HDL cholesterol<1.4 mmol/L; (D) BMI <25, BMI ≥25; *, p < 0.05; **, p < 0.01; ***, p < 0.001.
Increased DEC1 anticipates MetS and insulin resistance in OSA
To determine which disrupted clock genes were involved in the presence of MetS, we further compared mRNA levels of Bmal1, Dec1, and Per1 in OSA patients with or without MetS. As shown in Figure 5A, mRNA expression levels of Dec1 were significantly increased in the OSA-MetS group compared to the OSA-non-MetS group (p < 0.001). However, no significant differences were found for Baml1 and Per1 between OSA-MetS and OSA-non-MetS groups. All these data suggested circadian clock Dec1, increased by the extent of CIH, may play a critical role in MetS pathogenesis.
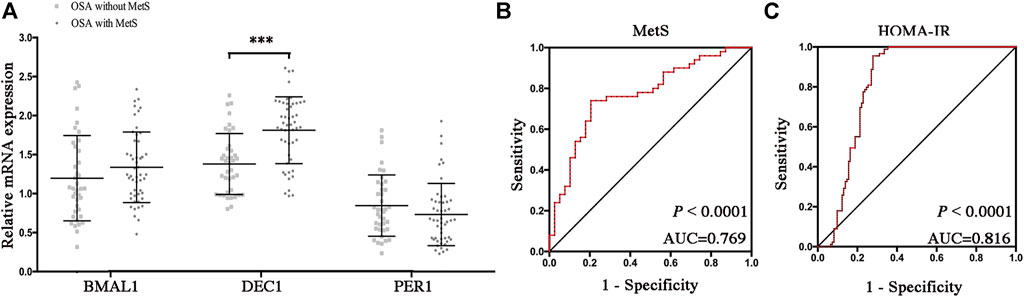
FIGURE 5. Increased DEC1 anticipates MetS and insulin resistance in OSA. (A) Expression levels of the disrupted clock genes (Bmal1, Dec1, and Per1) in the peripheral blood mononuclear cells between OSA-non-MetS and OSA-MetS subgroups (OSA-non-MetS vs. OSA-MetS). (B–C) ROC curve analyses were performed to evaluate the discriminatory power of Dec1 for MetS (B) and HOMA-IR (C). ***, p < 0.001.
IR has been considered as the core mechanism for developing MetS. Therefore, we performed ROC curve analyses of Dec1 (Figures 5B,C) for both MetS and IR. We observed that the areas under the curve (AUCs) for anticipation of MetS and IR were 0.769 and 0.816, respectively (p < 0.0001, for both). These results indicated that Dec1 expression levels in PBMCs may be used as a biomarker to predict MetS and IR in OSA patients.
Disrupted clock genes reflect the inflammatory and oxidant status in OSA
Chronic inflammatory response, one of the major hallmarks of OSA, has been considered to potentially cause MetS (Maniaci et al., 2021). Therefore, we further detected inflammatory markers in the OSA patients and tried to reveal the correlations of these inflammatory factors with circadian clock alternations. As shown in Table 2, 12-cytokines including IL-5, INF-α, IL-2, IL-2, IN-6, IL-1β, IL-10, IL-γ, IL-8, IL-17, IL-4, and IL-12P70, and TNF-α were measured to assess the level of inflammatory responses in OSA and MetS. The results indicated that IL-5 and IL-6 were much higher in the OSA group than those in the control group (p = 0.037). In the OSA group, OSA-MetS patients showed higher levels of IL-6 than the non-MetS patients (p = 0.022). However, no significant differences were found for other cytokines in the study cohort. These data suggest IL-5 and IL-6 are involved in the development of OSA, while IL-6 may be served as a key link between OSA and MetS.
To determine the underlying inflammatory pathways involved in the disruptions of clock genes by OSA, we also performed linear regression analyses to examine the relationship between OSA-upregulated inflammatory cytokines (IL-5 and IL-6) and disrupted clock genes (BMAL1, Dec1, and Per1) in OSA patients. As shown in Figure 6, no significant correlations between disrupted clocks (BMAL1, Dec1, and Per1) and IL-5 (Figures 6A–C) were found in the present study cohort. Although no significant correlations were observed between the disrupted clocks (BMAL1 and Per1) and IL-6, we found a positive correlation between Dec1 mRNA expressions and IL-6 levels in OSA patients (r = 0.275, p = 0.009, Figures 6D–F). These data indicate connections between Dec1 and IL-6, which regulate chronic low-grade inflammation, may be considered as an important pathological mechanism in OSA-induced MetS.
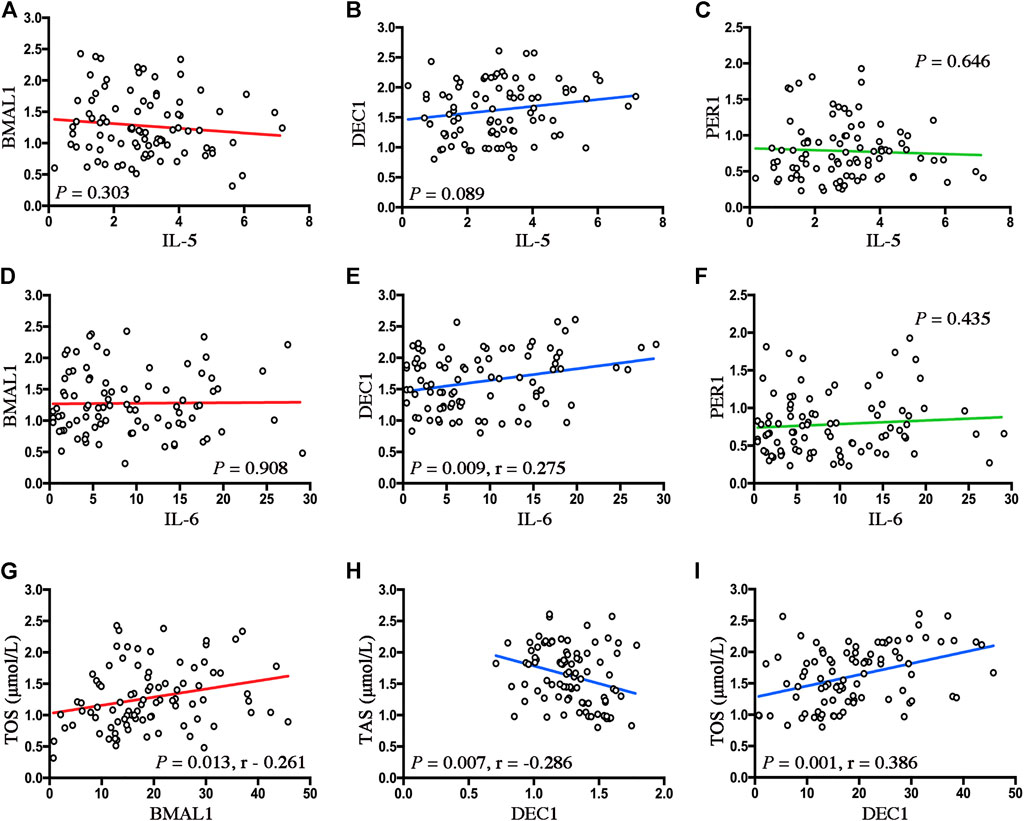
FIGURE 6. Disrupted clock genes reflect the inflammatory and oxidant status in OSA. (A–C) Linear regression between expression levels of Baml1 (A), Dec1 (B), Per1 (C), and IL-5. (D–F) Linear regression among expression levels of Baml1 (D), Dec1 (E), Per1 (F), and IL-6. (G–I) Linear regression between expression levels of Baml1 and TOS (G); Dec1 and TSA (H); Dec1 and TOS (I).
It is well known that oxidative stress is closely associated with the components of MetS, such as obesity, hypertension, hyperlipidemia, and diabetes (Martins et al., 2021). Therefore, we next explored whether these associations between OSA and oxidant status could be impacted by the disrupted circadian clocks in OSA patients. As shown in Table 3, although no significant differences for TAS were found between the control and OSA groups, TOS was significantly upregulated, and OSI was strongly downregulated in the OSA group compared to the control group (p < 0.001 for both). In addition, TAS and OSI were notably decreased (p = 0.039 and 0.024, respectively), while TOS was markedly increased (p < 0.001) in the OSA-MetS group compared with the OSA-non-MetS group. These data suggest that enhanced oxidative stress was involved in both OSA and MetS development.
We next explored the correlations between circadian clock expressions and oxidative levels by performing linear regression analyses. As shown in Figures 6A,G, positive correlation between levels of Bmal1 and TOS was found in OSA patients (r = −0.261, p = 0.013). In addition, Dec1 expressions were not only negatively correlated with the TAS levels (r = −0.286, p = 0.007, Figure 6H) but also positively correlated with the TOS levels in OSA patients (r = 0.386, p = 0.001, Figure 6I). These data suggest that alternations of the clock genes are closely associated with levels of oxidative stress in OSA.
Discussion
Epidemiologic evidence supports the interrelationships of OSA and Mets components that influence and promote each other. Recent research works showed that the biological clock was disrupted in OSA. In addition, circadian rhythms also have a profound impact on metabolic and cardiovascular disorders. Although these underlying connections between OSA and MetS were multifaceted and complicated, insulin resistance has emerged as a common pathophysiological mechanism and circadian clocks might be involved in the molecular changes. Indeed, whether which and how clock genes link OSA with Mets warrants further investigation. This study provides definitive evidence that circadian clock disruptions (Bmal1, Dec1, and Per1) are involved in the development of the MetS component. These disrupted clock genes, especially Dec1, are associated with systemic inflammation, oxidative stress, and IR. Circadian clock Dec1 can not only be used as a hypoxic index of OSA severity but also showed a predictive value for the presence of MetS in OSA patients.
Increasing incidence of Mets has been defined as a clinical feature and hallmark of OSA (Gasa et al., 2011; Murphy et al., 2017; Gaines et al., 2018; Hirotsu et al., 2018). A previous prospective cohort study has reported that OSA is an independent risk factor for incidence of MetS, but not vice versa. Although the presence of Mets did not lead to a growing incidence of OSA in the general population, moderate-to-severe OSA led to a 2-fold increase in the incidence of MetS, which was closely associated with nocturnal hypoxemia (Hirotsu et al., 2018). Another cross-sectional study also described the interrelationships between OSA and metabolic abnormalities, and showed definitive proof of the higher BP, and poorer lipid and glucose control that linked OSA to MetS (Gasa et al., 2011). Consistent with these studies, we also found that MetS components showed a higher prevalence among OSA patients than the non-OSA subjects (Table 1). In addition, we further evaluated levels of HOMA-IR in all participants and found that the well-known index of MetS was significantly correlated with the presence of OSA, which suggested tangled and complex associations among OSA, MetS, and IR. Indeed, mounting evidence has been pointed out with regard to insulin resistance and impaired glucose control among OSA patients, which are also linked to OSA severity and the presence of MetS (Murphy et al., 2017; Gaines et al., 2018; Mokhlesi et al., 2021). However, the specific molecular mechanism in connections of OSA-MetS has not yet been elaborated.
IR is wildly considered as the core mechanism in regulating MetS development. The predisposing effect of hypoxia on IR has been generally studied in both clinical cohorts and molecular mechanisms in the past decades (Gasa et al., 2011; Takikawa et al., 2016; Murphy et al., 2017; Thomas et al., 2017; Gaines et al., 2018; Hirotsu et al., 2018; Tang et al., 2020; Mokhlesi et al., 2021). For instance, endothelial-specific knockout of Argonaute 1, a key regulator in endothelial hypoxic response, protected from high-fat and high-sucrose induced obesity and IR in mice models (Tang et al., 2020). Another in vivo study also indicated that CIH-induced systemic and tissue-specific IR was independent of hypoxia-inducible factor 1 (HIF-1), but through activating the AMPK pathway. In addition, myeloid cell-HIF-1α-knockout mice improve the glucose metabolism in an anti-high-fat-diet-induced inflammatory manner (Takikawa et al., 2016). These investigations may provide some insight into how IR is activated during OSA-induced Mets. However, another possible candidate mechanism may be the dysfunction of the circadian clock network.
Cross-sectional studies have demonstrated that the circadian rhythm of clock genes was impaired in OSA (Yang et al., 2019; Gaspar et al., 2021). Yang et al. (2019) reported that oscillating reduction of Cry1 and Per3 occurred in a time-dependent manner (mainly at midnight) and served as a specific hallmark of severe OSA patients. Laetitia et al. found that OSA altered circadian rhythms of biological clocks, which could be re-established by continuous positive airway pressure (CPAP) (Gaspar et al., 2021). All these results support the hypothesis that disruption of the clock genes could be triggered under hypoxic events. Therefore, it is not surprising that the abnormal circadian rhythm of PER1 in OSA patients could be recovered by CPAP treatment (Burioka et al., 2008). Another study demonstrated that expression levels of HIF-1α were significantly upregulated and strongly associated with the altered circadian clock proteins in OSA patients (Gabryelska et al., 2020). This finding provides direct evidence for the dysregulation of circadian clocks via the hypoxia pathways. Indeed, some of the clock genes, such as Bmal1, Cry1/2, Dec1, and Per1, have been identified as hypoxia-responsive genes that contain HIF binding sites and play key roles in mediating oxygen adaptation (Miyazaki et al., 2002; Adamovich et al., 2017; Wu et al., 2017). Consistently, the present study confirms a similar finding that alternations of increased Baml1 and Dec1, but decreased Per1 exist in OSA patients (Figure 3). All the abovementioned observations revealed that some disrupted clocks could be not only served as an efficient hypoxic biomarker but also used for potential candidate targets in OSA treatment.
On the other hand, disrupted circadian clocks are also closely correlated with MetS components and glucose homeostasis. Recent in vivo studies indicate that peripheral clocks dysregulations in the liver, adipose, kidney, and smooth muscles are involved in the development of hypertension, diabetes, and obesity (Chang et al., 2018; Nakashima et al., 2018; Hou et al., 2019; Murata et al., 2020; Pati et al., 2021). In Dec1-deficient mice models, expression of Atp1b1, a negative transcriptional target of Dec1 and anti‐phase regulator of blood pressure rhythm, was upregulated in the kidney, aorta, and heart tissues, and in turn reduced blood pressure (Nakashima et al., 2018). Yu et al. (2019) have indicated that mRNA levels of BMAL1, Clock, Cry1, Cry2, and Per1 were significantly decreased and interrelated with pro-inflammatory biomarkers of IL-6 and TNF in diabetic patients. Another diabetic ob/ob mice model showed that some of the clock-controlled genes (Bmal1, Clock, Cry1, Dbp, Per1, and Per2) were substantially dysregulated in the liver and adipose tissues, which was prior to metabolic abnormalities (Ando et al., 2011). Either way, most of the studies have confirmed that the rhythm of the clock genes can be generally impaired by OSA and this disruption plays a key role in metabolic processes. It is possible that circadian clocks serve as a regulating link between OSA and metabolic dysfunction. Consistent with the above studies, we found that expressions of Bmal1, Dec1, and Per1 were not only dysregulated in OSA but also have a significant impact on the presence of MetS components, such as hyperglycemia (Dec1 and Per1, p < 0.05 and 0.001, respectively), hypertension (Bmal1 and Dec1, p < 0.001 and 0.01, respectively), hyperlipidemia (Dec1, p < 0.01), and obesity (Dec1, p < 0.05). To the best of our knowledge, the present study is the first to explore roles of clock genes in connections between OSA and MetS.
Oxidative stress and systemic inflammation are known major hallmarks of OSA and involve in a spectrum of cardiovascular and metabolic pathophysiological progression, as reviewed by Maniaci et al. (2021). Recently, Yamauchi et al. (2005) found that urinary 8-hydroxy-2-deoxyguanosine (8-OHdG), an oxidative index in vivo, was significantly increased in severe OSA patients and closely correlated with the hypoxia-related indicators following PSG. Hypoxia conditions impair the oxidant–antioxidant balance and break the redox homeostasis toward the oxidative side (Bozkurt et al., 2015). Therefore, it is clear that with the severity of intermittent hypoxia, a trend of a progressive decrease of TAS, and a significant increase of thiobarbituric acid-reacting substances (TBARS, an index of lipid peroxides) were found from non-to-mild, moderate, and severe OSA patients (Cofta et al., 2019). Consistent with these studies, we found the oxidative indicator TOS and the anti-oxidative indicator OSI were significantly correlated between the control and OSA groups. Furthermore, levels of TAS, TOS, and OSI were all significantly correlated between the OSA-non-MetS and OSA-MetS groups (Table 3). Collectively, all these results indicated that oxidative stress serves as another important hallmark and link in OSA with MetS.
In view of the increased ROS production by CIH-reoxygenation cycles, another critical consequence is presented with inflammation. Likewise, enhanced systemic inflammation had been repeatedly reported in the pathogenesis of both OSA and MetS. Actually, other previous and present studies have implicated the upregulation of pro-inflammatory cytokines in the progression of OSA and its complications through hypoxia-responsive and insulin signaling (Kritikou et al., 2014; Murphy et al., 2017). For instance, the findings of Murphy et al. (2017) demonstrated that intermittent hypoxia promoted the inflammation of visceral adipose tissue via M1 macrophage polarization and reduced the glucose uptake in 3T3-L1 adipocytes in an insulin-signaling pathway. Another study further confirmed OSA was closely associated with IR and inflammation even independent of obesity. They found apnoeic males showed higher hsCRP, IL-6, leptin, and insulin resistance than controls, while no significant influence can be observed in these biomarkers after CPAP treatment (Kritikou et al., 2014). In our study, we found that levels of IL-5 and IL-6 in OSA subjects were much higher than those of the controls, while IL-6 levels showed significant correlations between OSA-non-MetS and OSA-MetS groups (Table 2). Likewise, increased evidence has also been pointed out the role of IL-6, a biomarker of OSA, in mediating connections among OSA, IR or Mets (Kim et al., 2009; Maeder et al., 2015; Akbari and Hassan-Zadeh, 2018; Motamedi et al., 2018; Ko et al., 2019; Imani et al., 2020; Campos-Rodriguez et al., 2021), Although the molecular mechanisms are not clear, our findings indicate that these clocks / oxidative stress / inflammation / IR interactions play a key role in the linkage between OSA and MetS. Among the disrupted circadian clocks, Dec1 should be supposed to involve in these interactions, in view of its correlations with HOMA-IR, presence of Mets, TAS, TOS, and IL-6 (Figures 5, 6). Indeed, several studies provided evidence for this notion. First, among all the OSA-disrupted clock genes, Dec1 shows the most prominent change and the most specific OSA effect in OSA patients during the morning (Gaspar et al., 2021). Second, as a hypoxia-responsive and stress-associated protein, others and we have been found that Dec1 transcriptionally suppresses another transcriptional factor peroxisome proliferative activated receptor-γ (PPARγ), a key mediator in regulating insulin sensitivity (Yun et al., 2002; Li et al., 2021). Third, interactions of both Dec1/PPARγ and Dec1/IL-6 occur under inflammatory or oxidative burden and involved in cellular energy metabolism (Yun et al., 2002; Ning et al., 2017; Noshiro et al., 2020; Luan et al., 2021). Fourth, Dec1 has been reported to modulate the process of occurrence and development of MetS components, including hypertension, diabetes, and obesity (Yun et al., 2002; Yamada et al., 2003; Chen et al., 2015; Nakashima et al., 2018; Noshiro et al., 2018).
The study has some limitations: 1) the lack of a CPAP interventional arm makes it difficult to infer the role of hypoxia intervention in predicting better MetS outcomes in OSA subjects. Further data collection (e.g., clock gene expressions after CPAP treatment in OSA patients) is needed to make this more explicit. 2) Ethically speaking, we can only obtain the morning fasting blood samples of the participants. This precluded us from clarifying the expression difference of tissue-specific clock genes. In addition, circadian oscillation alternations of the clock genes were also unable to assay all day long. 3) Blood samples are limited in size; this study only detected part of the clock genes. There might have been other clock genes that impact MetS development in OSA patients.
In summary, these data suggest a new understanding of circadian clock disruptions in OSA patients and how these genes impact Mets development. Both oxidative stress and systemic inflammation are associated with specific clock gene alternations, and this might cause IR and endothelial dysfunction. Circadian clock disruptions might be the core link of the potential mechanism of OSA promoting cardiovascular metabolic diseases. Furthermore, Bmal1, Dec1, and Per1 may be independent predictors and potential therapeutic targets in OSA patients with MetS.
Data availability statement
The original contribution presented in the study are included in the article/Supplementary Material; further inquiries can be directed to the corresponding authors.
Ethics statement
The studies involving human participants were reviewed and approved by the Ethics Committee of Shandong Provincial Hospital Affiliated to Shandong First Medical University. The patients/participants provided their written informed consent to participate in this study.
Author contributions
Conceptualization: XML and XJL; methodology: XML and XJL; software: XZ and XJL; validation: XML, XZ, HL, and MX; formal analysis: QM; investigation: XW, XB, NG, and XH; resources: BW; data curation: XH; writing—original draft preparation: XML; writing—review and editing: HL and MX; visualization: MX; supervision: MX; project administration: MX; funding acquisition: XML and MX. All authors have read and agreed to the published version of the manuscript.
Funding statement
This work was supported by the Natural Science Foundation of Shandong Province (Nos. ZR2020QH155 and ZR2021MH189).
Conflict of interest
The authors declare that the research was conducted in the absence of any commercial or financial relationships that could be construed as a potential conflict of interest.
Publisher’s note
All claims expressed in this article are solely those of the authors and do not necessarily represent those of their affiliated organizations, or those of the publisher, the editors, and the reviewers. Any product that may be evaluated in this article, or claim that may be made by its manufacturer, is not guaranteed or endorsed by the publisher.
Supplementary material
The Supplementary Material for this article can be found online at: https://www.frontiersin.org/articles/10.3389/fphys.2022.932596/full#supplementary-material
References
Adamovich Y., Ladeuix B., Golik M., Koeners M. P., Asher G. (2017). Rhythmic oxygen levels reset circadian clocks through Hif1α. Cell Metab. 25 (1), 93–101. doi:10.1016/j.cmet.2016.09.014
Akbari M., Hassan-Zadeh V. (2018). Il-6 signalling pathways and the development of type 2 diabetes. Inflammopharmacology 26 (3), 685–698. doi:10.1007/s10787-018-0458-0
Ando H., Kumazaki M., Motosugi Y., Ushijima K., Maekawa T., Ishikawa E., et al. (2011). Impairment of peripheral circadian clocks precedes metabolic abnormalities in Ob/Ob mice. Endocrinology 152 (4), 1347–1354. doi:10.1210/en.2010-1068
Bass J., Lazar M. A. (2016). Circadian time signatures of fitness and disease. Science (New York, NY) 354 (6315), 994–999. doi:10.1126/science.aah4965
Benjafield A. V., Ayas N. T., Eastwood P. R., Heinzer R., Ip M. S. M., Morrell M. J., et al. (2019). Estimation of the global prevalence and burden of obstructive sleep apnoea: a literature-based analysis. Lancet. Respir. Med. 7 (8), 687–698. doi:10.1016/s2213-2600(19)30198-5
Bozkurt H., Neyal A., Geyik S., Taysi S., Anarat R., Bulut M., et al. (2015). Investigation of the plasma nitrite levels and oxidant-antioxidant status in obstructive sleep apnea syndrome. Noro Psikiyatr. Ars. 52 (3), 221–225. doi:10.5152/npa.2015.7607
Burioka N., Koyanagi S., Endo M., Takata M., Fukuoka Y., Miyata M., et al. (2008). Clock gene dysfunction in patients with obstructive sleep apnoea syndrome. Eur. Respir. J. 32 (1), 105–112. doi:10.1183/09031936.00138207
Cai J., Lyu X., Huang P., Li S., Chen R., Chen Z., et al. (2022). Increased levels of Chi3l1 and ha are associated with higher occurrence of liver damage in patients with obstructive sleep apnea. Front. Med. 9, 854570. doi:10.3389/fmed.2022.854570
Campos-Rodriguez F., Cordero-Guevara J., Asensio-Cruz M. I., Sanchez-Armengol A., Sanchez-Lopez V., Arellano-Orden E., et al. (2021). Interleukin 6 as a marker of depression in women with sleep apnea. J. Sleep. Res. 30 (1), e13035. doi:10.1111/jsr.13035
Chang L., Xiong W., Zhao X., Fan Y., Guo Y., Garcia-Barrio M., et al. (2018). Bmal1 in perivascular adipose tissue regulates resting-phase blood pressure through transcriptional regulation of angiotensinogen. Circulation 138 (1), 67–79. doi:10.1161/circulationaha.117.029972
Chen R., Wang Y., Ning R., Hu J., Liu W., Xiong J., et al. (2015). Decreased carboxylesterases expression and hydrolytic activity in type 2 diabetic mice through Akt/Mtor/Hif-1α/Stra13 pathway. Xenobiotica 45 (9), 782–793. doi:10.3109/00498254.2015.1020353
Cofta S., Winiarska H. M., Płóciniczak A., Bielawska L., Brożek A., Piorunek T., et al. (2019). Oxidative stress markers and severity of obstructive sleep apnea. Adv. Exp. Med. Biol. 1222, 27–35. doi:10.1007/5584_2019_433
Gabryelska A., Sochal M., Turkiewicz S., Białasiewicz P. (2020). Relationship between hif-1 and circadian clock proteins in obstructive sleep apnea patients-preliminary study. J. Clin. Med. 9 (5), E1599. doi:10.3390/jcm9051599
Gabryelska A., Turkiewicz S., Karuga F., Sochal M., Strzelecki D., Białasiewicz P. (2022). Disruption of circadian rhythm genes in obstructive sleep apnea patients-possible mechanisms involved and clinical implication. Int. J. Mol. Sci. 23 (2), 709. doi:10.3390/ijms23020709
Gaines J., Vgontzas A. N., Fernandez-Mendoza J., Bixler E. O. (2018). Obstructive sleep apnea and the metabolic syndrome: the road to clinically-meaningful phenotyping, improved prognosis, and personalized treatment. Sleep. Med. Rev. 42, 211–219. doi:10.1016/j.smrv.2018.08.009
Gao C., Guo J., Gong T. T., Lv J. L., Li X. Y., Liu F. H., et al. (2021). Sleep duration/quality with health outcomes: an umbrella review of meta-analyses of prospective studies. Front. Med. 8, 813943. doi:10.3389/fmed.2021.813943
Gasa M., Salord N., Fortuna A. M., Mayos M., Vilarrasa N., Dorca J., et al. (2011). Obstructive sleep apnoea and metabolic impairment in severe obesity. Eur. Respir. J. 38 (5), 1089–1097. doi:10.1183/09031936.00198810
Gaspar L. S., Hesse J., Yalçin M., Santos B., Carvalhas-Almeida C., Ferreira M., et al. (2021). Long-term continuous positive airway pressure treatment ameliorates biological clock disruptions in obstructive sleep apnea. EBioMedicine 65, 103248. doi:10.1016/j.ebiom.2021.103248
Hirotsu C., Haba-Rubio J., Togeiro S. M., Marques-Vidal P., Drager L. F., Vollenweider P., et al. (2018). Obstructive sleep apnoea as a risk factor for incident metabolic syndrome: a joined episono and hypnolaus prospective cohorts study. Eur. Respir. J. 52 (5), 1801150. doi:10.1183/13993003.01150-2018
Hou T., Su W., Guo Z., Gong M. C. (2019). A novel diabetic mouse model for real-time monitoring of clock gene oscillation and blood pressure circadian rhythm. J. Biol. Rhythms 34 (1), 51–68. doi:10.1177/0748730418803719
Imani M. M., Sadeghi M., Khazaie H., Emami M., Sadeghi Bahmani D., Brand S. (2020). Evaluation of serum and plasma interleukin-6 levels in obstructive sleep apnea syndrome: a meta-analysis and meta-regression. Front. Immunol. 11, 1343. doi:10.3389/fimmu.2020.01343
Kim J. H., Bachmann R. A., Chen J. (2009). Interleukin-6 and insulin resistance. Vitam. Horm. 80, 613–633. doi:10.1016/s0083-6729(08)00621-3
Ko C. Y., Liu Q. Q., Su H. Z., Zhang H. P., Fan J. M., Yang J. H., et al. (2019). Gut microbiota in obstructive sleep apnea-hypopnea syndrome: disease-related dysbiosis and metabolic comorbidities. Clin. Sci. 133 (7), 905–917. doi:10.1042/cs20180891
Kritikou I., Basta M., Vgontzas A. N., Pejovic S., Liao D., Tsaoussoglou M., et al. (2014). Sleep apnoea, sleepiness, inflammation and insulin resistance in middle-aged males and females. Eur. Respir. J. 43 (1), 145–155. doi:10.1183/09031936.00126712
Li X., Liu C., Qi W., Meng Q., Zhao H., Teng Z., et al. (2021). Endothelial dec1-pparγ axis impairs proliferation and apoptosis homeostasis under hypoxia in pulmonary arterial hypertension. Front. Cell Dev. Biol. 9, 757168. doi:10.3389/fcell.2021.757168
Lu Y. H., Lu J. M., Wang S. Y., Li C. L., Liu L. S., Zheng R. P., et al. (2006). Comparison of the diagnostic criteria of metabolic syndrome by international diabetes federation and that by Chinese medical association diabetes Branch. Zhonghua Yi Xue Za Zhi 86 (6), 386–389.
Luan X., Zhao Y., Bu N., Chen Y., Chen N. (2021). Dec1 negatively regulates Cyp2b6 expression by binding to the Cyp2b6 promoter region ascribed to il-6-induced downregulation of Cyp2b6 expression in hela cells. Xenobiotica 51, 1343–1351. doi:10.1080/00498254.2021.2004335
Maeder M. T., Strobel W., Christ M., Todd J., Estis J., Wildi K., et al. (2015). Comprehensive biomarker profiling in patients with obstructive sleep apnea. Clin. Biochem. 48 (4-5), 340–346. doi:10.1016/j.clinbiochem.2014.09.005
Makhout S., Vermeiren E., Van De Maele K., Bruyndonckx L., De Winter B. Y., Van Hoorenbeeck K., et al. (2021). The role of brain-derived neurotrophic factor in obstructive sleep apnea and endothelial function in a pediatric population with obesity. Front. Med. 8, 835515. doi:10.3389/fmed.2021.835515
Maniaci A., Iannella G., Cocuzza S., Vicini C., Magliulo G., Ferlito S., et al. (2021). Oxidative stress and inflammation biomarker expression in obstructive sleep apnea patients. J. Clin. Med. 10 (2), E277. doi:10.3390/jcm10020277
Martins C. C., Bagatini M. D., Simões J. L. B., Cardoso A. M., Baldissarelli J., Dalenogare D. P., et al. (2021). Increased oxidative stress and inflammatory markers contrasting with the activation of the cholinergic anti-inflammatory pathway in patients with metabolic syndrome. Clin. Biochem. 89, 63–69. doi:10.1016/j.clinbiochem.2020.12.007
Miyazaki K., Kawamoto T., Tanimoto K., Nishiyama M., Honda H., Kato Y. (2002). Identification of functional hypoxia response elements in the promoter region of the Dec1 and Dec2 genes. J. Biol. Chem. 277 (49), 47014–47021. doi:10.1074/jbc.M204938200
Mokhlesi B., Tjaden A. H., Temple K. A., Edelstein S. L., Sam S., Nadeau K. J., et al. (2021). Obstructive sleep apnea, glucose tolerance, and Β-cell function in adults with prediabetes or untreated type 2 diabetes in the restoring insulin secretion (rise) study. Diabetes Care 44 (4), 993–1001. doi:10.2337/dc20-2127
Motamedi V., Kanefsky R., Matsangas P., Mithani S., Jeromin A., Brock M. S., et al. (2018). Elevated tau and interleukin-6 concentrations in adults with obstructive sleep apnea. Sleep. Med. 43, 71–76. doi:10.1016/j.sleep.2017.11.1121
Murata Y., Ueno T., Tanaka S., Kobayashi H., Okamura M., Hemmi S., et al. (2020). Identification of clock genes related to hypertension in kidney from spontaneously hypertensive rats. Am. J. Hypertens. 33 (12), 1136–1145. doi:10.1093/ajh/hpaa123
Murphy A. M., Thomas A., Crinion S. J., Kent B. D., Tambuwala M. M., Fabre A., et al. (2017). Intermittent hypoxia in obstructive sleep apnoea mediates insulin resistance through adipose tissue inflammation. Eur. Respir. J. 49 (4), 1601731. doi:10.1183/13993003.01731-2016
Nakashima A., Kawamoto T., Noshiro M., Ueno T., Doi S., Honda K., et al. (2018). Dec1 and clock regulate Na(+)/K(+)-Atpase Β1 subunit expression and blood pressure. Hypertension 72 (3), 746–754. doi:10.1161/hypertensionaha.118.11075
Ning R., Zhan Y., He S., Hu J., Zhu Z., Hu G., et al. (2017). Interleukin-6 induces Dec1, promotes Dec1 interaction with Rxrα and suppresses the expression of Pxr, car and their target genes. Front. Pharmacol. 8, 866. doi:10.3389/fphar.2017.00866
Noshiro M., Kawamoto T., Nakashima A., Ozaki N., Saeki M., Honda K., et al. (2020). Dec1 regulates the rhythmic expression of Pparγ target genes involved in lipid metabolism in white adipose tissue. Genes Cells 25 (4), 232–241. doi:10.1111/gtc.12752
Noshiro M., Kawamoto T., Nakashima A., Ozaki N., Ueno T., Saeki M., et al. (2018). Deficiency of the basic helix-loop-helix transcription factor Dec1 prevents obesity induced by a high-fat diet in mice. Genes Cells 23, 658–669. doi:10.1111/gtc.12607
Parameswaran G., Ray D. W. (2022). Sleep, circadian rhythms, and type 2 diabetes mellitus. Clin. Endocrinol. 96 (1), 12–20. doi:10.1111/cen.14607
Pati P., Valcin J. A., Zhang D., Neder T. H., Millender-Swain T., Allan J. M., et al. (2021). Liver circadian clock disruption alters perivascular adipose tissue gene expression and aortic function in mice. Am. J. Physiol. Regul. Integr. Comp. Physiol. 320 (6), R960–R971. doi:10.1152/ajpregu.00128.2020
Ruaro B., Salton F., Braga L., Wade B., Confalonieri P., Volpe M. C., et al. (2021). The history and mystery of alveolar epithelial type ii cells: focus on their physiologic and pathologic role in lung. Int. J. Mol. Sci. 22 (5), 2566. doi:10.3390/ijms22052566
Stenvers D., Scheer F., Schrauwen P., la Fleur S., Kalsbeek A. (2019). Circadian clocks and insulin resistance. Nat. Rev. Endocrinol. 15 (2), 75–89. doi:10.1038/s41574-018-0122-1
Takahashi J. S. (2017). Transcriptional architecture of the mammalian circadian clock. Nat. Rev. Genet. 18 (3), 164–179. doi:10.1038/nrg.2016.150
Takikawa A., Mahmood A., Nawaz A., Kado T., Okabe K., Yamamoto S., et al. (2016). Hif-1α in myeloid cells promotes adipose tissue remodeling toward insulin resistance. Diabetes 65 (12), 3649–3659. doi:10.2337/db16-0012
Tang X., Miao Y., Luo Y., Sriram K., Qi Z., Lin F., et al. (2020). Suppression of endothelial Ago1 promotes adipose tissue browning and improves metabolic dysfunction. Circulation 142 (4), 365–379. doi:10.1161/circulationaha.119.041231
Teng X., Zhang Z., He G., Yang L., Li F. (2012). Validation of reference genes for quantitative expression analysis by real-time rt-pcr in four Lepidopteran insects. J. Insect Sci. 12 (1), 60. doi:10.1673/031.012.6001
Thomas A., Belaidi E., Moulin S., Horman S., van der Zon G., Viollet B., et al. (2017). Chronic intermittent hypoxia impairs insulin sensitivity but improves whole-body glucose tolerance by activating skeletal muscle ampk. Diabetes 66 (12), 2942–2951. doi:10.2337/db17-0186
Wu Y., Tang D., Liu N., Xiong W., Huang H., Li Y., et al. (2017). Reciprocal regulation between the circadian clock and hypoxia signaling at the genome level in mammals. Cell Metab. 25 (1), 73–85. doi:10.1016/j.cmet.2016.09.009
Xiao H., Ding N., Liao H., Yao Z., Cheng X., Zhang J., et al. (2019). Prediction of relapse and prognosis by expression levels of long noncoding rna Peg10 in glioma patients. Medicine 98 (45), e17583. doi:10.1097/md.0000000000017583
Yamada K., Kawata H., Shou Z., Mizutani T., Noguchi T., Miyamoto K. (2003). Insulin induces the expression of the sharp-2/stra13/dec1 gene via a phosphoinositide 3-kinase pathway. J. Biol. Chem. 278 (33), 30719–30724. doi:10.1074/jbc.M301597200
Yamauchi M., Nakano H., Maekawa J., Okamoto Y., Ohnishi Y., Suzuki T., et al. (2005). Oxidative stress in obstructive sleep apnea. Chest 127 (5), 1674–1679. doi:10.1378/chest.127.5.1674
Yang M. Y., Lin P. W., Lin H. C., Lin P. M., Chen I. Y., Friedman M., et al. (2019). Alternations of circadian clock genes expression and oscillation in obstructive sleep apnea. J. Clin. Med. 8 (10), E1634. doi:10.3390/jcm8101634
Yu R., Tian L., Ding Y., Gao Y., Li D., Tang Y. (2019). Correlation between inflammatory markers and impaired circadian clock gene expression in type 2 diabetes mellitus. Diabetes Res. Clin. Pract. 156, 107831. doi:10.1016/j.diabres.2019.107831
Keywords: obstructive sleep apnea, metabolic syndrome, clock genes, insulin resistance, oxidative stress, inflammatory response
Citation: Li X, Liu X, Meng Q, Wu X, Bing X, Guo N, Zhao X, Hou X, Wang B, Xia M and Li H (2022) Circadian clock disruptions link oxidative stress and systemic inflammation to metabolic syndrome in obstructive sleep apnea patients. Front. Physiol. 13:932596. doi: 10.3389/fphys.2022.932596
Received: 16 July 2022; Accepted: 04 August 2022;
Published: 29 August 2022.
Edited by:
Barbara Ruaro, University of Trieste, ItalyReviewed by:
Riccardo Pozzan, University of Trieste, ItalyGloria Maria Citton, Azienda Sanitaria Università Integrata di Trieste, Italy
Copyright © 2022 Li, Liu, Meng, Wu, Bing, Guo, Zhao, Hou, Wang, Xia and Li. This is an open-access article distributed under the terms of the Creative Commons Attribution License (CC BY). The use, distribution or reproduction in other forums is permitted, provided the original author(s) and the copyright owner(s) are credited and that the original publication in this journal is cited, in accordance with accepted academic practice. No use, distribution or reproduction is permitted which does not comply with these terms.
*Correspondence: Hui Li, MTk3N21laWd1aUAxNjMuY29t; Ming Xia, eGlhbWluZ3NkdUBzb2h1LmNvbQ==
†These authors have contributed equally to this work