- 1Department of Neurophysiology and Chronobiology, Institute of Zoology and Biomedical Research, Jagiellonian University, Kraków, Poland
- 2School of Physiology, Pharmacology and Neuroscience, University of Bristol, University Walk, Bristol, United Kingdom
Level of motivation, responsiveness to rewards and punishment, invigoration of exploratory behaviours, and motor performance are subject to daily fluctuations that emerge from circadian rhythms in neuronal activity of the midbrain’s dopaminergic system. While endogenous circadian rhythms are weak in the ventral tegmental area and substantia nigra pars compacta, daily changes in expression of core clock genes, ion channels, neurotransmitter receptors, dopamine-synthesising enzymes, and dopamine transporters, accompanied by changes in electrical activity, are readily observed in these nuclei. These processes cause dopamine levels released in structures innervated by midbrain dopaminergic neurons (e.g., the striatum) to oscillate in a circadian fashion. Additionally, growing evidence show that the master circadian clock located in the suprachiasmatic nucleus of the hypothalamus (SCN) rhythmically influences the activity of the dopaminergic system through various intermediate targets. Thus, circadian changes in the activity of the dopaminergic system and concomitant dopamine release observed on a daily scale are likely to be generated both intrinsically and entrained by the master clock. Previous studies have shown that the information about the value and salience of stimuli perceived by the animal is encoded in the neuronal activity of brain structures innervating midbrain dopaminergic centres. Some of these structures themselves are relatively autonomous oscillators, while others exhibit a weak endogenous circadian rhythm synchronised by the SCN. Here, we place the dopaminergic system as a hub in the extensive network of extra-SCN circadian oscillators and discuss the possible consequences of its daily entrainment for animal physiology and behaviour.
Introduction
Historically, the suprachiasmatic nucleus of the hypothalamus (SCN) has been considered the only, master circadian clock which governs all rhythmic changes in physiology and behaviour occurring at a daily timescale. However, evidence accumulating over the last 2 decades questions the supremacy of the SCN (Yoo et al., 2004; Guilding and Piggins, 2007; Paul et al., 2020). With the discovery of intrinsic clock gene expression in several extra-SCN brain nuclei, from the olfactory bulb (Abraham, 2005), through thalamic (Chrobok et al., 2021a), epithalamic (Guilding et al., 2010), hypothalamic (Guilding et al., 2009) and midbrain regions (Chrobok et al., 2021b), all the way to the hindbrain (Kaneko et al., 2009; Chrobok et al., 2020), it is now believed that at least a part of the rhythmic control of homeostasis must be devolved to such local clocks. These circadian timekeeping centres vary in the degree of their autonomy (Guilding and Piggins, 2007; Paul et al., 2020; Chrobok et al., 2021c). An ‘autonomous oscillator’ displays molecular and electrophysiological rhythms that are strongly synchronised amongst its single cells due to highly-functional connectivity within the structure. These coordinated single-cell oscillations are therefore synchronised in phase what results in a robust endogenous rhythmicity at the whole structure level. Similarly, a ‘semi-autonomous oscillator’ shows intrinsic single-cell rhythmicity. Though, as a cause of a sparse interconnectivity, it requires an entraining input from the autonomous clock, synchronising its components to produce high-amplitude rhythms. Last, a “subordinate oscillator” lacks endogenous mechanisms to express intrinsic oscillations. Rather, its rhythmicity reflects and relies on an input from the autonomous or semi-autonomous clock.
Neurons located in the ventral tegmental area (VTA) and substantia nigra pars compacta (SNc) have been suggested to possess such circadian clock properties (Becker-Krail et al., 2022). These are exhibited through circadian variation in clock gene expression, electrical activity, and the concomitant dopamine release in the targeted brain areas. It is still not clear whether these day-night oscillations result from intrinsic properties of dopaminergic neurons, extrinsic sources (such as SCN), or a combination of both. Different evidence points to either of the scenarios, but it is most plausible that dopaminergic system serves as a semi-autonomous oscillator heavily entrained by other circadian clocks. Most importantly, though, these day-to-night variations, despite their origin, influence animal behaviour in a circadian fashion, as dopamine is well positioned to control e.g., the locomotor activity, goal-directed learning, and motivation. Since most rodents are nocturnal, their locomotor activity is significantly higher during dark phase and it decreases during the behaviourally quiescent light phase. In line with this, animal motivation for food seeking, water and food consumption increases during the active phase (Boulos et al., 1980; Ding et al., 2018; Acosta et al., 2020). Similarly, mating behaviour predominately occurs at night, exhibiting clear circadian rhythmicity (Antle et al., 2005). Additionally, drug self-administration has been found to undergo similar daily changes (Roberts et al., 2002; Trujillo et al., 2009). All these daily-regulated behaviours are under control of brain reward system (Cools, 1986; Pfaus et al., 1990; Volkow et al., 2011a; Volkow et al., 2011b; Berridge and Robinson, 2016).
In this perspective, we aim to explore possible sources of daily and circadian rhythmicity in the dopaminergic system. We first focus on abilities of dopaminergic neurons to express molecular and electrophysiological rhythms around 24 h. Then, we describe possible daily patterning of input to the dopaminergic system from other extra-SCN clocks. Finally, we aim to position the midbrain dopaminergic system as one of the hubs in the complex network of brain circadian oscillators.
Circadian and Daily Rhythms in the Dopaminergic System
The dopaminergic system of the ventral midbrain exhibits circadian variation in the expression of core clock genes, such as Per1, Per2, Per3, Bmal1 and Cry (Bussi et al., 2014; Wang et al., 2019; Koch et al., 2020). Some evidence points out that oscillations in clock genes expression are present within the isolated substantia nigra (Natsubori et al., 2014) and VTA (Landgraf et al., 2016) explant cultures. Other reports, however, using similar methodology, show no or very weak oscillations in the VTA (Abe et al., 2002; Myung et al., 2018) and SNc (Hiler et al., 2008). Thus, even if the VTA/SNc can sustain intrinsic rhythms in clock gene expression, their endogenous timekeeping properties are not overtly robust.
It has also been shown that the electrical activity of VTA neurons changes in the circadian cycle (Luo et al., 2008; Domínguez-López et al., 2014; Fifel et al., 2018). The firing rate of dopaminergic neurons in vivo was reported to oscillate in a 12 h cycle (with peaks at the beginning of phases) and the number of spontaneously active dopaminergic neurons to change in a 24 h cycle (with the peak at the end of the dark phase) (Domínguez-López et al., 2014). Additionally, a new population of fast-firing non-dopaminergic and non-GABAergic cells was reported within the VTA, which are active only during the active phase (Luo et al., 2008). The activity of the VTA is also elevated during the night in freely moving mice; in case of the SNc only a non-significant trend was observed (Fifel et al., 2018). Importantly, multiunit recordings were used for data collection, thus distinguishing between cell types contributing to the daily firing alterations was not possible. Nonetheless, night-time increase in firing agrees with reports showing more cFos-immunoreactive dopaminergic cells within the VTA during the night (Baltazar et al., 2013) as well as increased night-time glucose utilization within the SNc (Room and Tielemans, 1989). The disruption in the molecular clock (Clock knockout) elevated both firing rate and bursting of dopaminergic neurons in vivo (Mcclung et al., 2005). Overall, these data suggest that the electrical activity of dopaminergic neurons undergoes circadian changes, but to what extent this variability depends on changes in their clock genes expression, and to what extent it reflects rhythmic changes in the inputs to the dopaminergic system, remains unclear.
In line with that, studies congruently demonstrate that dopamine concentration is elevated in the striatum during the active phase (Owasoyo et al., 1979; Smith et al., 1992; Paulson and Robinson, 1994; Castañeda et al., 2004; Hampp et al., 2008; Bussi et al., 2014; Ferris et al., 2014; Koch et al., 2020). However, the level and pattern of electrical activity of midbrain dopaminergic neurons does not exhibit as pronounced circadian rhythmicity as it would be expected from the dopamine release rhythm. Thus, it may suggest that other mechanisms of dopamine release regulation may additionally contribute to this phenomenon. Indeed, the expression of genes responsible for synthesis and turnover of dopamine show clear day-night variation. The expression of monoamine oxydase (MAO), which increases dopamine turnover and decay, is lower during the night in both the striatum and VTA (Hampp et al., 2008). Similarly, dopamine transporter (DAT) was shown to be crucial for daily variation in dopamine release (Ferris et al., 2014). Accordingly, the expression of tyrosine hydroxylase (TH), a limiting enzyme in dopamine biosynthesis, is higher during the night in the striatum (Sleipness et al., 2007; Webb et al., 2009; Bussi et al., 2014; Ferris et al., 2014; Koch et al., 2020). Overall, these studies demonstrate that dopamine release increases during night-time, which may be due to daily changes in the activity of dopaminergic neurons and/or the mechanisms that control synaptic dopamine release. In any of these scenarios, the enhanced dopaminergic drive during the dark phase has clear behavioural consequences.
Indeed, it has been shown that the period of locomotor activity in VTA-lesioned rats is significantly decreased (Isobe and Nishino, 2001). Moreover, a lack of D1 dopamine receptor (D1R) in mice attenuates the rate of circadian entrainment (Grippo et al., 2017) and impairs food anticipatory activity (Gallardo et al., 2014). On the other hand, disturbances in the molecular clock influence dopamine-related behaviours, e.g., selective VTA Clock-knockouts exhibit increased locomotor activity in the novel environment (Mukherjee et al., 2010). Moreover, drug abuse studies have confirmed that mice lacking Clock or Per2 genes were more strongly rewarded by cocaine administration (Abarca et al., 2002; Mcclung et al., 2005). Conversely, mice with Per1 knockdown experience no reward after cocaine injection (Abarca et al., 2002). Altogether, these studies reveal intrinsic clock properties of the dopaminergic system that influence daily and circadian rhythms in animal behaviour.
Besides intrinsic circadian timekeeping properties of the VTA, it receives an indirect input from the master clock. It has been shown that the median preoptic nucleus (MPON) serves as a relay between the SCN and the VTA (Luo and Aston-Jones, 2009; Mendoza and Challet, 2014). In return, the VTA directly innervates the master clock, with dopamine able to regulate the rate of photoentrainment of the SCN (Isobe and Nishino, 2001). It has been also shown that this connection plays a key role in brain development, as it synchronises the circadian rhythmicity of the mother and her offspring (Grippo et al., 2020) and, by disruption of food intake timing, can induce overconsumption (Weaver et al., 1992; Viswanathan et al., 1994). Moreover, it is worth mentioning that dopaminergic signalling may be driving the expression of some extra-SCN circadian rhythms, for example in the synthesis of clock protein PERIOD2 in the dorsal striatum (Hood et al., 2010). Importantly, dopaminergic system was shown to be important for maintaining ultradian (∼4 h) locomotor rhythm as either DAT knockout, metamphetamine treatment or chemogenetic activation of dopaminergic neurons lengthened its period (Blum et al., 2014; Bourguignon and Storch, 2017). Moreover, haloperidol had an opposite effect and striatal dopamine levels were shown to correlate strongly with this rhythm. Congruently, dopaminergic system is posed to be important in the control of anticipatory activity rhythms (Steele and Mistlberger, 2015; de Lartigue and McDougle, 2019; Mistlberger, 2020). Thus, we hypothesise that the indirect SCN input to the VTA may synchronise its semi-autonomous rhythms and entrain them to the changing light-dark cycle, but we also highlight the reciprocal character of this connection.
Circadian Rhythmicity in the Input Pathways to the Dopaminergic System
As mentioned above, the last 2 decades have brought discoveries of several extra-SCN neuronal populations of circadian oscillators with varying degrees of autonomy, which participate in shaping daily changes in physiology, behaviour, and cognitive processes. Unsurprisingly, at least some of these extra-SCN clocks are among the building blocks of the incredibly extensive, monosynaptic inputome of the ventral midbrain dopaminergic neurons (Watabe-Uchida et al., 2012; Ogawa et al., 2014). This may have a twofold effect on the functioning of the dopaminergic system.
First, the circadian rhythmicity in the electrical activity present in these input structures may translate into changes of similar, hourly dynamics in the level of dopaminergic neuron firing and concomitant dopamine release in target brain regions as described above. Indeed, such circadian changes have been observed in multiple brain nuclei innervating dopaminergic neurons; with these neuronal centres being the source of those inputs spread throughout the whole cerebrum and carrying information of various kinds. Some of them are involved in maintaining homeostasis of the organism (e.g., dorsomedial hypothalamus; LH, lateral hypothalamus; NTS, nucleus of the solitary tract; Figure 1) (Kirouac and Ciriello, 1997; Leinninger et al., 2009; Mejías-Aponte et al., 2009; Leinninger et al., 2011; Tyree and de Lecea, 2017; Alhadeff et al., 2019) processing of sensory information (e.g., SC, superior colliculus) (Peter and Kevin, 2006; Redgrave et al., 2010; Chrobok et al., 2021b; Pradel et al., 2021), controlling general states of arousal (e.g., LH; tuberomammillary nucleus) (Azeez et al., 2018; Heiss et al., 2018), and carrying out cognitive processes (e.g., PFC, prefrontal cortex; Figure 1) (Carr and Sesack, 2000; Chun et al., 2015; Calabrese et al., 2016; Chen et al., 2016; Seney et al., 2019).
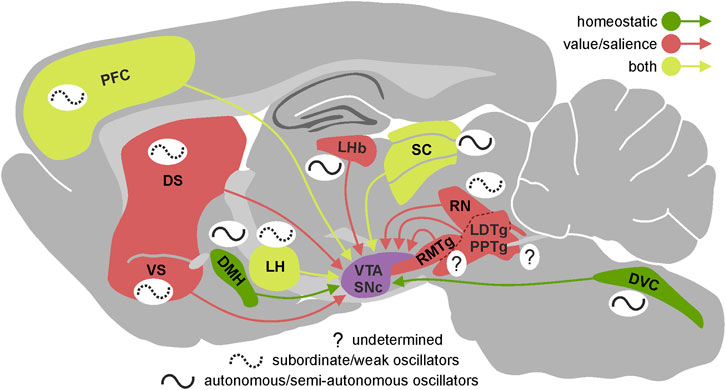
FIGURE 1. Circadian properties of the VTA/SNc inputs. Presence and autonomy of circadian rhythmicity in the most important brain regions innervating VTA/SNc presented on the sagittal section of rat brain. Brain regions are divided according to the type of information they provide dopaminergic system with—green: homeostatic, red: value/salience, yellow: both. The schematic wave next to each brain region represents its circadian rhythmicity. Solid line indicates high level of autonomy (autonomous/semi-autonomous oscillator) and dotted line indicates low level of autonomy (subordinate/weak oscillator) while question mark shows that we currently lack the knowledge about circadian properties of a given brain region. VTA, ventral tegmental area; SNc, substantia nigra pars compacta; DS, dorsal striatum; VS, ventral striatum; LHb, lateral habenula; RMTg, rostromedial tegmental nucleus; LDTg, laterodorsal tegmental nucleus; PPTg, pedunculopontine tegmental nucleus; RN, raphe nucleus; SC, superior colliculus; LH, lateral hypothalamus; DMH, dorsomedial hypothalamic nucleus; DVC, dorsal vagal complex; PFC, prefrontal cortex.
Second, it has been shown that the motivational aspects of stimuli perceived by the animal are encoded in the electrical activity of dopaminergic neurons, based on information from other regions of the brain, which often carry a partially or fully encoded value or salience of the stimulus (Tian et al., 2016; de Jong et al., 2019). Circadian changes in electrophysiological properties of the elements building the internal neural networks of such structures may affect the information passed on to the dopaminergic neurons. In fact, daily changes in gene expression (including clock genes) and/or electrical activity have been observed in many structures and neuronal pathways involved in encoding the motivational and salient features of stimuli, including the medial prefrontal cortex (Chun et al., 2015; Calabrese et al., 2016), dorsal and ventral striatum (Masubuchi et al., 2000; Ángeles-Castellanos et al., 2007; Wang et al., 2019), lateral habenula–rostromedial tegmental nucleus pathway (LHb-RMTg) (Zhao and Rusak, 2005; Guilding et al., 2010; Sakhi et al., 2014; Baño-Otálora and Piggins, 2017), LH (Marston et al., 2008; Azeez et al., 2018), dorsal vagal complex (Kaneko et al., 2009; Chrobok et al., 2020; Chrobok et al., 2021d; Chrobok et al., 2021e; Chrobok et al., 2021f) and dorsal raphe nucleus (Abe et al., 2002; Paul et al., 2020) (Figure 1). It is noteworthy, that in case of some important inputs to the dopaminergic system we lack even basic information about their potential circadian rhythmicity (e.g., pedunculopontine tegmental nucleus, laterodorsal tegmental nucleus, RMTg).
Therefore, it seems that the circadian changes in the electrophysiological properties of the structures innervating dopaminergic neurons may translate into long-scale (occurring in hours) changes in the basal activity of the dopaminergic system, as well as changes in the short-scale (occurring in milliseconds or seconds) phasic responses of DA neurons that encode value and/or salience of the perceived stimuli. Likewise, circadian changes in long-scale and short-scale dopaminergic activity should be apparent at the level of the animal’s behavioural states and behaviours.
Importantly, daily changes in the dopaminergic system happening on the long timescale seem to depend predominantly on the intrinsic brain mechanisms (i.e., clock genes expression along with multi-clock network activity); notably, these are shaped by the long-lasting environmental conditions (i.e., light/dark cycle) (Mendoza and Challet, 2014; Becker-Krail et al., 2022). On the other hand, the dopaminergic system functioning on the short timescale is extremely sensitive to brief cues appearing in the environment (e.g., reward-associated stimuli) (Schultz, 2016). Additionally, these short responses may also be modulated by the current electrophysiological state of dopaminergic neurons, which is a manifestation of long timescale circadian oscillations.
Open Questions and Further Research Directions
Circadian Changes in Fast and Slow Dynamics of the Dopaminergic System
The pattern of electrical activity that a dopaminergic neuron exhibits at a given moment can be positioned on a spectrum ranging between two extremes: tonic, pacemaker-like firing of action potentials and, on the other end, generation of action potentials in purely bursting manner (Grace and Bunney, 1984). These two firing modes are controlled by different input signals and their contribution to the basal activity of dopaminergic neurons differs amongst behavioural states (Robinson et al., 2002; Lodge and Grace, 2006; Geisler et al., 2007; Tsai et al., 2009; Zweifel et al., 2009; Watabe-Uchida et al., 2012). Relatively slow changes in dopaminergic neuron excitability occurring on a scale much longer than seconds or minutes may affect the functioning of the dopaminergic systems in two ways. First, by alerting the propensity to generate action potential bursts and thus setting the ratio of tonic to burst firing, it determines the basal dopamine release in target structures. This in turn affects some aspects of the general behavioural state of an animal (e.g., level of arousal, behavioural invigoration, tendency to effort, and energy expenditure) (Parsons and Justice, 1993; Floresco et al., 2003; Cagniard et al., 2006; Niv et al., 2007; Wang et al., 2021). Second, changes in excitability may affect how short synaptic events translate into momentary changes in dopaminergic neuron firing and thus into phasic increases and/or decreases in dopamine release. Such brief changes in dopamine release, usually lasting less than a second, encode the value and/or salience of stimuli perceived by the animal at a given moment and are the basis of many cognitive processes such as experience-based learning, decision-making, and directing attention (Schultz et al., 1979; Stuber et al., 2005; Day et al., 2007; Stuber et al., 2008; Sunsay and Rebec, 2008; Dreyer et al., 2010; Jastrzebska et al., 2016; Stojakovic et al., 2018; Budygin et al., 2020). Although slow and fast dynamic changes in the dopaminergic system have been extensively described in the literature, the open question remains and how they are affected by the circadian clock.
Our current knowledge sheds light on the contribution of the circadian clock machinery to some of the slow changes observed in the activity of the dopaminergic system. Mostly, it describes the diurnal variation in the clock gene expression, overall electrical activity, concomitant dopamine release, as well as daily changes in the animal behaviour. These observations are consistent with the fact that in many neuronal populations circadian clock gene expression is accompanied, often causally, by changes in the expression of genes determining the membrane properties, neuron responsiveness to neurotransmitters, and the effectiveness of further signal transmission (Paul et al., 2020). In fact, it has been shown that the expression of genes coding some potassium channels, subunits of glutamate and GABA receptors, and proteins related to dopamine release in dopaminergic neurons depend on molecular clock (Mcclung et al., 2005). Such changes in the electrophysiological properties of neurons should directly translate into circadian alterations of both slow and fast dynamic phenomena occurring in the dopaminergic system and related behaviours. Still, there are many gaping holes in our current knowledge on the subject. We still know very little about whether and how the circadian, slow changes in the properties of neurons affect the fast processes taking place in the dopaminergic system, such as encoding value or salience stimuli. Moreover, as it will be discussed in more detail in the next section, it is likely that the circadian changes in VTA/SNc input structures involved in the calculation of the reward prediction error are also reflected at the level of dopaminergic system.
Circadian Changes in Dopaminergic System Upstream and Downstream Structures
As already described above, dopaminergic system receives inputs from multiple regions distributed throughout the whole brain. Since presumably both the baseline activity and responsiveness to environmental cues of these inputs alter across day/night cycle, magnitude of dopaminergic system excitation/inhibition evoked by those inputs should be affected as well. More precisely, it is important to establish how the parameters (such as amplitude, duration, latency, or even polarity) of responses of dopaminergic neurons to value/salience information provided by different inputs (e.g., PFC, SC, LHb, LH; Figure 1) change at different times of a daily cycle. Assuming that the dopaminergic system acts as a hub that computes and sums incoming information, and the circadian oscillation of VTA/SNc inputs change the weight of information they carry, the output information from the dopaminergic system should be appropriately adjusted. The question posed above needs an urgent answer because previous experiments on the responses of dopaminergic neurons to manipulations of its inputs were not designed to measure differences between light and dark phases of the circadian cycle. Additionally, it should be delineated to what extent the dopaminergic signalling is affected by its endogenous circadian rhythmicity (and concomitant changes in electrophysiological properties), or by external circadian rhythmicity within individual VTA/SNc input structures. Notably, almost none of the inputs that reach dopaminergic system are homogeneous biochemically and structurally. Thus, understanding how daily oscillations within particular brain region as a whole might be insufficient to explain its real impact on the neuronal activity of the dopaminergic system across 24 h; different cellular populations within one brain region which often fulfil different functions and convey different information might undergo dissimilar circadian changes.
It is expected that both basal dopamine levels as well as value/salience encoding should differ in active and inactive phases as they pose different behavioural challenges to animals. For example, the impact of the ambient light level and environmental cues on the activity of the dopaminergic system during both phases should differ; indeed, it was shown that the activity of superficial layers of the SC is lower during the night (Chrobok et al., 2021b). The same should apply for the homeostatic information provided by various hypothalamic regions as well as the NTS (Chrobok et al., 2021d; Chrobok et al., 2021f), as the food intake along with the motivational drive to eat varies strongly between active and inactive phases. Some of these hypothalamic structures also regulate the general arousal state so the daily variation in their activity is also anticipated to affect the activity of the dopaminergic system to a great extent. The way dopaminergic neurons respond to aversive and threatening stimuli in active and inactive phase most likely differ as distinct rodents’ predators lurk at different times of a day. Moreover, alertness to such information is different while the animals are resting. For that reason, daily changes in the LHb and RMTg responsiveness (and baseline activity) should not come as a surprise. Indeed, it was shown that the activity of the LHb varies across 24 h cycle (Baño-Otálora and Piggins, 2017). As a result of the multi-input circadian oscillations, the change in the activity of the dopaminergic system in both short and long timescales should translate into daily dopamine release alterations in the target brain areas, such as the striatum. This relation, however, does not have to be linear as various daily changing factors at the level of the striatum itself might influence the dopamine release (e.g., activity of cortical inputs or dopamine level controlling enzymes expression).
Concluding Remarks
The current development in understanding central circadian timing mechanisms, shifting from the uni-clock concept of the omnipotent SCN towards the more complex multi-clock theory, provides new insights in the regulation of discrete neuronal systems. The dopaminergic system serves as an interesting example. The combination of intrinsic circadian timekeeping in the dopaminergic system itself with the circadian fluctuation of multiple inputs to the VTA/SNc from local brain clocks (exhibiting a range of autonomy from the SCN), ensures much more flexibility than a single, circadian input from the master clock. This assumption can be further strengthened by the fact, that these different “ticking” inputs are entrained by different environmental stimuli. With the SCN being a light-entrainable oscillator poorly entrained by feeding, the input from other extra-SCN clocks may provide information e.g., on the circadian patterning of food intake, valuable for the dopaminergic system functioning. Thus, considering the dopaminergic system as a hub in the intricate network of circadian oscillators, drawn in our perspective article, offers novel research opportunities in both reward and circadian neuroscience.
Data Availability Statement
The original contributions presented in the study are included in the article/Supplementary Material, further inquiries can be directed to the corresponding authors.
Author Contributions
KP, GD, LC and TB performed the literature research, wrote parts of the manuscript and revised the manuscript. KP prepared the figure.
Funding
LC was funded by sir Henry Wellcome Postdoctoral Fellowship (224116/Z/21/Z) awarded by Wellcome Trust, United Kingdom KP was funded by Preludium (2017/27/N/NZ4/00785) and Etiuda (2020/36/T/NZ4/00341) grants awarded by National Science Center, Poland. TB was funded by Opus grant (2020/36/T/NZ4/00341) awarded by National Science Center, Poland.
Conflict of Interest
The authors declare that the research was conducted in the absence of any commercial or financial relationships that could be construed as a potential conflict of interest.
Publisher’s Note
All claims expressed in this article are solely those of the authors and do not necessarily represent those of their affiliated organizations, or those of the publisher, the editors and the reviewers. Any product that may be evaluated in this article, or claim that may be made by its manufacturer, is not guaranteed or endorsed by the publisher.
References
Abarca C., Albrecht U., Spanagel R. (2002). Cocaine Sensitization and Reward Are under the Influence of Circadian Genes and Rhythm. Proc. Natl. Acad. Sci. U.S.A. 99 (13), 9026–9030. doi:10.1073/pnas.142039099
Abe M., Herzog E. D., Yamazaki S., Straume M., Tei H., Sakaki Y., et al. (2002). Circadian Rhythms in Isolated Brain Regions. J. Neurosci. 22 (1), 350–356. doi:10.1523/jneurosci.22-01-00350.2002
Abraham U. (2005). Independent Circadian Oscillations of Period1 in Specific Brain Areas In Vivo and In Vitro. J. Neurosci. 25 (38), 8620–8626. doi:10.1523/jneurosci.2225-05.2005
Acosta J., Bussi I. L., Esquivel M., Höcht C., Golombek D. A., Agostino P. V. (2020). Circadian Modulation of Motivation in Mice. Behav. Brain Res. 382, 112471. doi:10.1016/j.bbr.2020.112471
Alhadeff A. L., Goldstein N., Park O., Klima M. L., Vargas A., Betley J. N. (2019). Natural and Drug Rewards Engage Distinct Pathways that Converge on Coordinated Hypothalamic and Reward Circuits. Neuron 103 (5), 891–908. e6. doi:10.1016/j.neuron.2019.05.050
Ángeles-Castellanos M., Mendoza J., Escobar C. (2007). Restricted Feeding Schedules Phase Shift Daily Rhythms of C-Fos and Protein Per1 Immunoreactivity in Corticolimbic Regions in Rats. Neuroscience 144 (1), 344–355. doi:10.1016/j.neuroscience.2006.08.064
Antle M. C., Mistlberger R. E. (2005). “Circadian Rhythms,” in The Behavior of the Laboratory Rat: A Handbook with Tests. Editors I. Q. Whishaw, and B. Kolb (Oxford: Oxford University Press), 193–194.
Azeez I. A., del Gallo F., Cristino L., Bentivoglio M. (2018). Daily Fluctuation of Orexin Neuron Activity and Wiring: The Challenge of "Chronoconnectivity". Front. Pharmacol. 9, 1061. doi:10.3389/fphar.2018.01061
Baltazar R. M., Coolen L. M., Webb I. C. (2013). Diurnal Rhythms in Neural Activation in the Mesolimbic Reward System: Critical Role of the Medial Prefrontal Cortex. Eur. J. Neurosci. 38 (2), 2319–2327. doi:10.1111/ejn.12224
Baño-Otálora B., Piggins H. D. (2017). Contributions of the Lateral Habenula to Circadian Timekeeping. Pharmacol. Biochem. Behav. 162, 46–54. doi:10.1016/j.pbb.2017.06.007
Becker-Krail D. D., Walker W. H., Nelson R. J. (2022). The Ventral Tegmental Area and Nucleus Accumbens as Circadian Oscillators: Implications for Drug Abuse and Substance Use Disorders. Front. Physiol. 13, 886704. doi:10.3389/fphys.2022.886704
Berridge K. C., Robinson T. E. (2016). Liking, Wanting, and the Incentive-Sensitization Theory of Addiction. Am. Psychol. 71 (8), 670–679. doi:10.1037/amp0000059
Blum I. D., Zhu L., Moquin L., Kokoeva M. V., Gratton A., Giros B., et al. (2014). A Highly Tunable Dopaminergic Oscillator Generates Ultradian Rhythms of Behavioral Arousal. elife 3, 1–23. doi:10.7554/eLife.05105
Boulos Z., Rosenwasser A. M., Terman M. (1980). Feeding Schedules and the Circadian Organization of Behavior in the Rat. Behav. Brain Res. 1, 39–65. doi:10.1016/0166-4328(80)90045-5
Bourguignon C., Storch K. F. (2017). Control of Rest:Activity by a Dopaminergic Ultradian Oscillator and the Circadian Clock. Front. Neurol. 8, 614–618. doi:10.3389/fneur.2017.00614
Budygin E. A., Bass C. E., Grinevich V. P., Deal A. L., Bonin K. D., Weiner J. L. (2020). Opposite Consequences of Tonic and Phasic Increases in Accumbal Dopamine on Alcohol-Seeking Behavior. iScience 23 (3), 100877. doi:10.1016/j.isci.2020.100877
Bussi I. L., Levín G., Golombek D. A., Agostino P. V. (2014). Involvement of Dopamine Signaling in the Circadian Modulation of Interval Timing. Eur. J. Neurosci. 40 (1), 2299–2310. doi:10.1111/ejn.12569
Cagniard B., Balsam P. D., Brunner D., Zhuang X. (2006). Mice with Chronically Elevated Dopamine Exhibit Enhanced Motivation, but Not Learning, for a Food Reward. Neuropsychopharmacol 31 (7), 1362–1370. doi:10.1038/sj.npp.1300966
Calabrese F., Savino E., Papp M., Molteni R., Riva M. A. (2016). Chronic Mild Stress-Induced Alterations of Clock Gene Expression in Rat Prefrontal Cortex: Modulatory Effects of Prolonged Lurasidone Treatment. Pharmacol. Res. 104, 140–150. doi:10.1016/j.phrs.2015.12.023
Carr D. B., Sesack S. R. (2000). Projections from the Rat Prefrontal Cortex to the Ventral Tegmental Area: Target Specificity in the Synaptic Associations with Mesoaccumbens and Mesocortical Neurons. J. Neurosci. 20 (10), 3864–3873. doi:10.1523/jneurosci.20-10-03864.2000
Castañeda T. R., Prado B. M., Prieto D., Mora F. (2004). Circadian Rhythms of Dopamine, Glutamate and GABA in the Striatum and Nucleus Accumbens of the Awake Rat: Modulation by Light. J. Pineal Res. 36 (3), 177–185. doi:10.1046/j.1600-079x.2003.00114.x
Chen W., Xia X., Song N., Wang Y., Zhu H., Deng W., et al. (2016). Cross-species Analysis of Gene Expression and Function in Prefrontal Cortex, hippocampus and Striatum. PLoS ONE 11 (10), e0164295–18. doi:10.1371/journal.pone.0164295
Chrobok L., Belle M. D. C., Myung J. (2021). From Fast Oscillations to Circadian Rhythms: Coupling at Multiscale Frequency Bands in the Rodent Subcortical Visual System. Front. Physiology 12, 1–8. doi:10.3389/fphys.2021.738229
Chrobok L., Jeczmien-Lazur J. S., Bubka M., Pradel K., Klekocinska A., Klich J. D., et al. (2021). Daily Coordination of Orexinergic Gating in the Rat Superior Colliculus—Implications for Intrinsic Clock Activities in the Visual System. FASEB J. 35 (10), 1–19. doi:10.1096/fj.202100779rr
Chrobok L., Northeast R. C., Myung J., Cunningham P. S., Petit C., Piggins H. D. (2020). Timekeeping in the Hindbrain: a Multi-Oscillatory Circadian Centre in the Mouse Dorsal Vagal Complex. Commun. Biol. 3 (1), 225. doi:10.1038/s42003-020-0960-y
Chrobok L., Pradel K., Janik M. E., Sanetra A. M., Bubka M., Myung J., et al. (2021). Intrinsic Circadian Timekeeping Properties of the Thalamic Lateral Geniculate Nucleus. J. Neurosci. Res. 99, 3306–3324. doi:10.1002/jnr.24973
Chrobok L., Wojcik M., Klich J. D., Pradel K., Lewandowski M. H., Piggins H. D. (2021). Phasic Neuronal Firing in the Rodent Nucleus of the Solitary Tract Ex Vivo. Front. Physiology 12, 638695. doi:10.3389/fphys.2021.638695
Chrobok L., Klich J. D., Jeczmien‐Lazur J. S., Pradel K., Palus‐Chramiec K., Sanetra A. M., et al. (2021). Daily Changes in Neuronal Activities of the Dorsal Motor Nucleus of the Vagus under Standard and High‐fat Diet. J. Physiology 600 (4), 733–749. doi:10.1113/jp281596
Chrobok L., Klich J. D., Sanetra A. M., Jeczmien‐Lazur J. S., Pradel K., Palus‐Chramiec K., et al. (2021). Rhythmic Neuronal Activities of the Rat Nucleus of the Solitary Tract Are Impaired by High‐fat Diet - Implications for Daily Control of Satiety. J. Physiology 600 (4), 751–767. doi:10.1113/jp281838
Chun L. E., Woodruff E. R., Morton S., Hinds L. R., Spencer R. L. (2015). Variations in Phase and Amplitude of Rhythmic Clock Gene Expression across Prefrontal Cortex, hippocampus, Amygdala, and Hypothalamic Paraventricular and Suprachiasmatic Nuclei of Male and Female Rats. J. Biol. Rhythms 30 (5), 417–436. doi:10.1177/0748730415598608
Cools A. R. (1986). Mesolimbic Dopamine and its Control of Locomotor Activity in Rats: Differences in Pharmacology and Light/dark Periodicity between the Olfactory Tubercle and the Nucleus Accumbens. Psychopharmacol. Berl. 88, 451–459. doi:10.1007/BF00178506
Day J. J., Roitman M. F., Wightman R. M., Carelli R. M. (2007). Associative Learning Mediates Dynamic Shifts in Dopamine Signaling in the Nucleus Accumbens. Nat. Neurosci. 10 (8), 1020–1028. doi:10.1038/nn1923
de Jong J. W., Afjei S. A., Pollak Dorocic I., Peck J. R., Liu C., Kim C. K., et al. (2019). A Neural Circuit Mechanism for Encoding Aversive Stimuli in the Mesolimbic Dopamine System. Neuron 101 (1), 133–151. e7. doi:10.1016/j.neuron.2018.11.005
de Lartigue G., McDougle M. (2019). Dorsal Striatum Dopamine Oscillations: Setting the Pace of Food Anticipatory Activity. Acta Physiol. 225, 1–23. doi:10.1111/apha.13152
Ding G., Gong Y., Eckel-Mahan K. L., Sun Z. (2018). Central Circadian Clock Regulates Energy Metabolism. Adv. Exp. Med. Biol. 1090, 79–103. doi:10.1007/978-981-13-1286-1_5
Domínguez-López S., Howell R. D., López-Canúl M. G., Leyton M., Gobbi G. (2014). Electrophysiological Characterization of Dopamine Neuronal Activity in the Ventral Tegmental Area across the Light-Dark Cycle. Synapse 68 (10), 454–467. doi:10.1002/syn.21757
Dreyer J. K., Herrik K. F., Berg R. W., Hounsgaard J. D. (2010). Influence of Phasic and Tonic Dopamine Release on Receptor Activation. J. Neurosci. 30 (42), 14273–14283. doi:10.1523/jneurosci.1894-10.2010
Ferris M. J., España R. A., Locke J. L., Konstantopoulos J. K., Rose J. H., Chen R., et al. (2014). Dopamine Transporters Govern Diurnal Variation in Extracellular Dopamine Tone. Proc. Natl. Acad. Sci. U. S. A. 111 (26), E2751–E2759. doi:10.1073/pnas.1407935111
Fifel K., Meijer J. H., Deboer T. (2018). Circadian and Homeostatic Modulation of Multi-Unit Activity in Midbrain Dopaminergic Structures. Sci. Rep. 8 (1), 7765. doi:10.1038/s41598-018-25770-5
Floresco S. B., West A. R., Ash B., Moore H., Grace A. A. (2003). Afferent Modulation of Dopamine Neuron Firing Differentially Regulates Tonic and Phasic Dopamine Transmission. Nat. Neurosci. 6 (9), 968–973. doi:10.1038/nn1103
Gallardo C. M., Darvas M., Oviatt M., Chang C. H., Michalik M., Huddy T. F., et al. (2014). Dopamine Receptor 1 Neurons in the Dorsal Striatum Regulate Food Anticipatory Circadian Activity Rhythms in Mice. Elife 3, e03781. doi:10.7554/eLife.03781
Geisler S., Derst C., Veh R. W., Zahm D. S. (2007). Glutamatergic Afferents of the Ventral Tegmental Area in the Rat. J. Neurosci. 27 (21), 5730–5743. doi:10.1523/jneurosci.0012-07.2007
Grace A., Bunney B. (1984). The Control of Firing Pattern in Nigral Dopamine Neurons: Single Spike Firing. J. Neurosci. 4 (11), 2866–2876. doi:10.1523/jneurosci.04-11-02866.1984
Grippo R. M., Purohit A. M., Zhang Q., Zweifel L. S., Güler A. D. (2017). Direct Midbrain Dopamine Input to the Suprachiasmatic Nucleus Accelerates Circadian Entrainment. Curr. Biol. 27 (16), 2465–2475. doi:10.1016/j.cub.2017.06.084
Grippo R. M., Tang Q., Zhang Q., Chadwick S. R., Gao Y., Altherr E. B., et al. (2020). Dopamine Signaling in the Suprachiasmatic Nucleus Enables Weight Gain Associated with Hedonic Feeding. Curr. Biol. 30 (2), 196–208. doi:10.1016/j.cub.2019.11.029
Guilding C., Hughes A. T., Brown T. M., Namvar S., Piggins H. D. (2009). A Riot of Rhythms: Neuronal and Glial Circadian Oscillators in the Mediobasal Hypothalamus. Mol. Brain 2, 28. doi:10.1186/1756-6606-2-28
Guilding C., Hughes A. T. L., Piggins H. D. (2010). Circadian Oscillators in the Epithalamus. Neuroscience 169 (4), 1630–1639. doi:10.1016/j.neuroscience.2010.06.015
Guilding C., Piggins H. D. (2007). Challenging the Omnipotence of the Suprachiasmatic Timekeeper: Are Circadian Oscillators Present throughout the Mammalian Brain? Eur. J. Neurosci. 25 (11), 3195–3216. doi:10.1111/j.1460-9568.2007.05581.x
Hampp G., Ripperger J. A., Houben T., Schmutz I., Blex C., Perreau-Lenz S., et al. (2008). Regulation of Monoamine Oxidase A by Circadian-Clock Components Implies Clock Influence on Mood. Curr. Biol. 18 (9), 678–683. doi:10.1016/j.cub.2008.04.012
Heiss J. E., Yamanaka A., Kilduff T. S. (2018). Parallel Arousal Pathways in the Lateral Hypothalamus. eNeuro 5 (4), 1–18. doi:10.1523/ENEURO.0228-18.2018
Hiler D. J., Bhattacherjee A., Yamazaki S., Tei H., Geusz M. E. (2008). Circadian mPer1 Gene Expression in Mesencephalic Trigeminal Nucleus Cultures. Brain Res. 1214, 84–93. doi:10.1016/j.brainres.2008.03.041
Hood S., Cassidy P., Cossette M.-P., Weigl Y., Verwey M., Robinson B., et al. (2010). Endogenous Dopamine Regulates the Rhythm of Expression of the Clock Protein PER2 in the Rat Dorsal Striatum via Daily Activation of D2 Dopamine Receptors. J. Neurosci. 30 (42), 14046–14058. doi:10.1523/jneurosci.2128-10.2010
Isobe Y., Nishino H. (2001). Circadian Rhythm of Drinking and Running-Wheel Activity in Rats with 6-hydroxydopamine Lesions of the Ventral Tegmental Area. Brain Res. 899, 187–192. doi:10.1016/s0006-8993(01)02223-5
Jastrzebska K., Walczak M., Cieslak P. E., Szumiec L., Turbasa M., Engblom D., et al. (2016). Loss of NMDA Receptors in Dopamine Neurons Leads to the Development of Affective Disorder-like Symptoms in Mice. Sci. Rep. 6 (1), 1–11.
Kaneko K., Yamada T., Tsukita S., Takahashi K., Ishigaki Y., Oka Y., et al. (2009). Obesity Alters Circadian Expressions of Molecular Clock Genes in the Brainstem. Brain Res. 1263, 58–68. doi:10.1016/j.brainres.2008.12.071
Kirouac G. J., Ciriello J. (1997). Medullary Inputs to Nucleus Accumbens Neurons. Am. J. Physiology-Regulatory, Integr. Comp. Physiology 273 (6), R2080–R2088. doi:10.1152/ajpregu.1997.273.6.r2080
Koch C. E., Begemann K., Kiehn J. T., Griewahn L., Mauer J., M E Hess M. E., et al. (2020). Circadian Regulation of Hedonic Appetite in Mice by Clocks in Dopaminergic Neurons of the VTA. Nat. Commun. 11 (1), 3071. doi:10.1038/s41467-020-16882-6
Landgraf D., Long J. E., Welsh D. K. (2016). Depression-like Behaviour in Mice Is Associated with Disrupted Circadian Rhythms in Nucleus Accumbens and Periaqueductal Grey. Eur. J. Neurosci. 43 (10), 1309–1320. doi:10.1111/ejn.13085
Leinninger G. M., Jo Y.-H., Leshan R. L., Louis G. W., Yang H., Barrera J. G., et al. (2009). Leptin Acts via Leptin Receptor-Expressing Lateral Hypothalamic Neurons to Modulate the Mesolimbic Dopamine System and Suppress Feeding. Cell. Metab. 10 (2), 89–98. doi:10.1016/j.cmet.2009.06.011
Leinninger G. M., Opland D. M., Jo Y.-H., Faouzi M., Christensen L., Cappellucci L. A., et al. (2011). Leptin Action via Neurotensin Neurons Controls Orexin, the Mesolimbic Dopamine System and Energy Balance. Cell. Metab. 14 (3), 313–323. doi:10.1016/j.cmet.2011.06.016
Lodge D. J., Grace A. A. (2006). The hippocampus Modulates Dopamine Neuron Responsivity by Regulating the Intensity of Phasic Neuron Activation. Neuropsychopharmacol 31 (7), 1356–1361. doi:10.1038/sj.npp.1300963
Luo A. H., Aston-Jones G. (2009). Circuit Projection from Suprachiasmatic Nucleus to Ventral Tegmental Area: A Novel Circadian Output Pathway. Eur. J. Neurosci. 29 (4), 748–760. doi:10.1111/j.1460-9568.2008.06606.x
Luo A. H., Georges F. E., Aston-Jones G. S. (2008). Novel Neurons in Ventral Tegmental Area Fire Selectively during the Active Phase of the Diurnal Cycle. Eur. J. Neurosci. 27 (2), 408–422. doi:10.1111/j.1460-9568.2007.05985.x
Marston O. J., Williams R. H., Canal M. M., Samuels R. E., Upton N., Piggins H. D. (2008). Circadian and Dark-Pulse Activation of Orexin/hypocretin Neurons. Mol. Brain 1 (1), 19. doi:10.1186/1756-6606-1-19
Masubuchi S., Honma S., Abe H., Ishizaki K., Namihira M., Ikeda M., et al. (2000). Clock Genes outside the Suprachiasmatic Nucleus Involved in Manifestation of Locomotor Activity Rhythm in Rats. Eur. J. Neurosci. 12 (12), 4206–4214. doi:10.1111/j.1460-9568.2000.01313.x
Mcclung C. A., Sidiropoulou K., Vitaterna M., Takahashi J. S., White F. J., Cooper D. C., et al. (2005). Regulation of Dopaminergic Transmission and Cocaine Reward by the Clock Gene. Proc. Natl. Acad. Sci. U.S.A. 102 (26), 9377–9381. doi:10.1073/pnas.0503584102
Mejías-Aponte C. A., Drouin C., Aston-Jones G. (2009). Adrenergic and Noradrenergic Innervation of the Midbrain Ventral Tegmental Area and Retrorubral Field: Prominent Inputs from Medullary Homeostatic Centers. J. Neurosci. 29 (11), 3613–3626. doi:10.1523/jneurosci.4632-08.2009
Mendoza J., Challet E. (2014). Circadian Insights into Dopamine Mechanisms. Neuroscience 282, 230–242. doi:10.1016/j.neuroscience.2014.07.081
Mistlberger R. E. (2020). Food as Circadian Time Cue for Appetitive Behavior. F1000Research 9, 1–12. doi:10.12688/f1000research.20829.1
Mukherjee S., Coque L., Cao J.-L., Kumar J., Chakravarty S., Asaithamby A., et al. (2010). Knockdown of Clock in the Ventral Tegmental Area through RNA Interference Results in a Mixed State of Mania and Depression-like Behavior. Biol. Psychiatry 68 (6), 503–511. doi:10.1016/j.biopsych.2010.04.031
Myung J., Schmal C., Hong S., Tsukizawa Y., Rose P., Zhang Y., et al. (2018). The Choroid Plexus Is an Important Circadian Clock Component. Nat. Commun. 9 (1), 1062. doi:10.1038/s41467-018-03507-2
Natsubori A., Honma K.-i., Honma S. (2014). Dual Regulation of Clock genePer2expression in Discrete Brain Areas by the Circadian Pacemaker and Methamphetamine-Induced Oscillator in Rats. Eur. J. Neurosci. 39 (2), 229–240. doi:10.1111/ejn.12400
Niv Y., Daw N. D., Joel D., Dayan P. (2007). Tonic Dopamine: Opportunity Costs and the Control of Response Vigor. Psychopharmacology 191 (3), 507–520. doi:10.1007/s00213-006-0502-4
Ogawa S. K., Cohen J. Y., Hwang D., Uchida N., Watabe-Uchida M. (2014). Organization of Monosynaptic Inputs to the Serotonin and Dopamine Neuromodulatory Systems. Cell. Rep. 8 (4), 1105–1118. doi:10.1016/j.celrep.2014.06.042
Owasoyo J. O., Walker C. A., Whitworth U. G. (1979). Diurnal Variation in the Dopamine Level of Rat Brain Areas: Effect of Sodium Phenobarbital. Life Sci. 25 (2), 119–122. doi:10.1016/0024-3205(79)90382-5
Parsons L. H., Justice J. B. (1993). Perfusate Serotonin Increases Extracellular Dopamine in the Nucleus Accumbens as Measured by In Vivo Microdialysis. Brain Res. 606 (2), 195–199. doi:10.1016/0006-8993(93)90984-u
Paul J. R., Davis J. A., Goode L. K., Becker B. K., Fusilier A., Meador‐Woodruff A., et al. (2020). Circadian Regulation of Membrane Physiology in Neural Oscillators throughout the Brain. Eur. J. Neurosci. 51 (1), 109–138. doi:10.1111/ejn.14343
Paulson P. E., Robinson T. E. (1994). Relationship between Circadian Changes in Spontaneous Motor Activity and Dorsal versus Ventral Striatal Dopamine Neurotransmission Assessed with On-Line Microdialysis. Behav. Neurosci. 108 (3), 624–635. doi:10.1037/0735-7044.108.3.624
Peter Redgrave, Kevin Gurney (2006). Superior Colliculus Visual Stimulus. Nat. Rev. Neurosci. 7 (12), 967–975.
Pfaus J. G., Damsma G., Nomikos G. G., Wenkstern D. G., Blaha C. D., Phillips A. G., et al. (1990). Sexual Behavior Enhances Central Dopamine Transmission in the Male Rat. Brain Res. 530, 345–348. doi:10.1016/0006-8993(90)91309-5
Pradel K., Drwiȩga G., Błasiak T. (2021). Superior Colliculus Controls the Activity of the Rostromedial Tegmental Nuclei in an Asymmetrical Manner. J. Neurosci. 41 (18), 4006–4022. doi:10.1523/jneurosci.1556-20.2021
Redgrave P., Coizet V., Comoli E., McHaffie J. G., Leriche M., Vautrelle N., et al. (2010). Interactions between the Midbrain Superior Colliculus and the Basal Ganglia. Front. Neuroanat. 4, 132. doi:10.3389/fnana.2010.00132
Roberts D. C. S., Brebner K., Vincler M., Lynch W. J. (2002). Patterns of Cocaine Self-Administration in Rats Produced by Various Access Conditions under a Discrete Trials Procedure. Drug Alcohol Dependence 67 (3), 291–299. doi:10.1016/s0376-8716(02)00083-2
Robinson D. L., Heien M. L. A. V., Wightman R. M. (2002). Frequency of Dopamine Concentration Transients Increases in Dorsal and Ventral Striatum of Male Rats during Introduction of Conspecifics. J. Neurosci. 22 (23), 10477–10486. doi:10.1523/jneurosci.22-23-10477.2002
Room P., Tielemans A. J. P. C. (1989). Circadian Variations in Local Cerebral Glucose Utilization in Freely Moving Rats. Brain Res. 505, 321–325. doi:10.1016/0006-8993(89)91460-1
Sakhi K., Wegner S., Belle M. D. C., Howarth M., Delagrange P., Brown T. M., et al. (2014). Intrinsic and Extrinsic Cues Regulate the Daily Profile of Mouse Lateral Habenula Neuronal Activity. J. Physiol. 592 (22), 5025–5045. doi:10.1113/jphysiol.2014.280065
Schultz W., Dayan P., Montague P. R. (1979). A Neural Substrate of Prediction and Reward. Science 275 (5306), 1593–1599. doi:10.1126/science.275.5306.1593
Schultz W. (2016). Dopamine Reward Prediction-Error Signalling: A Two-Component Response. Nat. Rev. Neurosci. 17, 183–195. Nature Publishing Group. doi:10.1038/nrn.2015.26
Seney M. L., Cahill K., Enwright J. F., Logan R. W., Huo Z., Zong W., et al. (2019). Diurnal Rhythms in Gene Expression in the Prefrontal Cortex in Schizophrenia. Nat. Commun. 10 (1), 3355–3365. doi:10.1038/s41467-019-11335-1
Sleipness E. P., Sorg B. A., Jansen H. T. (2007). Diurnal Differences in Dopamine Transporter and Tyrosine Hydroxylase Levels in Rat Brain: Dependence on the Suprachiasmatic Nucleus. Brain Res. 1129 (1), 34–42. doi:10.1016/j.brainres.2006.10.063
Smith A. D., Olson R. J., Justice J. B. (1992). Quantitative Microdialysis of Dopamine in the Striatum: Effect of Circadian Variation. J. Neurosci. Methods 44, 33–41. doi:10.1016/0165-0270(92)90111-p
Steele A. D., Mistlberger R. E. (2015). Activity Is a Slave to Many Masters. Elife 4, e06351–3. doi:10.7554/eLife.06351
Stojakovic A., Walczak M., Cieślak P. E., Trenk A., Sköld J., Zajdel J., et al. (2018). Several Behavioral Traits Relevant for Alcoholism Are Controlled by Ɣ2 Subunit Containing GABAA Receptors on Dopamine Neurons in Mice. Neuropsychopharmacol 43 (7), 1548–1556. doi:10.1038/s41386-018-0022-z
Stuber G. D., Klanker M., de Ridder B., Bowers M. S., Joosten R. N., Feenstra M. G., et al. (2008). Reward-predictive Cues Enhance Excitatory Synaptic Strength onto Midbrain Dopamine Neurons. Science 321 (5896), 1690–1692. doi:10.1126/science.1160873
Stuber G. D., Roitman M. F., Phillips P. E. M., Carelli R. M., Wightman R. M. (2005). Rapid Dopamine Signaling in the Nucleus Accumbens during Contingent and Noncontingent Cocaine Administration. Neuropsychopharmacol 30 (5), 853–863. doi:10.1038/sj.npp.1300619
Sunsay C., Rebec G. V. (2008). Real-time Dopamine Efflux in the Nucleus Accumbens Core during Pavlovian Conditioning. Behav. Neurosci. 122 (2), 358–367. doi:10.1037/0735-7044.122.2.358
Tian J., Huang R., Cohen J. Y., Osakada F., Kobak D., Machens C. K., et al. (2016). Distributed and Mixed Information in Monosynaptic Inputs to Dopamine Neurons. Neuron 91 (6), 1374–1389. doi:10.1016/j.neuron.2016.08.018
Trujillo J. L., Roberts A. J., Gorman M. R. (2009). Circadian Timing of Ethanol Exposure Exerts Enduring Effects on Subsequent ad libitum Consumption in C57 Mice. Alcohol. Clin. Exp. Res. 33 (7), 1286–1293. doi:10.1111/j.1530-0277.2009.00954.x
Tsai H.-C., Zhang F., Adamantidis A., Stuber G. D., Bonci A., de Lecea L., et al. (2009). Phasic Firing in Dopaminergic Neurons Is Sufficient for Behavioral Conditioning. Science 324 (5930), 1080–1084. doi:10.1126/science.1168878
Tyree S. M., de Lecea L. (2017). Lateral Hypothalamic Control of the Ventral Tegmental Area: Reward Evaluation and the Driving of Motivated Behavior. Front. Syst. Neurosci. 11, 50. doi:10.3389/fnsys.2017.00050
Viswanathan N., Weaver D., Reppert S., Davis F. (1994). Entrainment of the Fetal Hamster Circadian Pacemaker by Prenatal Injections of the Dopamine Agonist SKF 38393. J. Neurosci. 14 (9), 5393–5398. doi:10.1523/jneurosci.14-09-05393.1994
Volkow N. D., Wang G.-J., Baler R. D. (2011). Reward, Dopamine and the Control of Food Intake: Implications for Obesity. Trends Cognitive Sci. 15 (1), 37–46. doi:10.1016/j.tics.2010.11.001
Volkow N. D., Wang G.-J., Fowler J. S., Tomasi D., Telang F. (2011). Addiction: Beyond Dopamine Reward Circuitry. Proc. Natl. Acad. Sci. U.S.A. 108 (37), 15037–15042. doi:10.1073/pnas.1010654108
Wang D.-Q., Wang X.-L., Wang C.-Y., Wang Y., Li S.-X., Liu K.-Z. (2019). Effects of Chronic Cocaine Exposure on the Circadian Rhythmic Expression of the Clock Genes in Reward-Related Brain Areas in Rats. Behav. Brain Res. 363, 61–69. doi:10.1016/j.bbr.2019.01.035
Wang Y., Toyoshima O., Kunimatsu J., Yamada H., Matsumoto M. (2021). Tonic Firing Mode of Midbrain Dopamine Neurons Continuously Tracks Reward Values Changing Moment-By-Moment. Elife 10, e63166. doi:10.7554/elife.63166
Watabe-Uchida M., Zhu L., Ogawa S. K., Vamanrao A., Uchida N. (2012). Whole-Brain Mapping of Direct Inputs to Midbrain Dopamine Neurons. Neuron 74 (5), 858–873. doi:10.1016/j.neuron.2012.03.017
Weaver D. R., Rivkees S. A., Reppert S. M. (1992). D1-dopamine Receptors Activate C-Fos Expression in the Fetal Suprachiasmatic Nuclei. Proc. Natl. Acad. Sci. U.S.A. 89, 9201–9204. doi:10.1073/pnas.89.19.9201
Webb I. C., Baltazar R. M., Wang X., Pitchers K. K., Coolen L. M., Lehman M. N. (2009). Diurnal Variations in Natural and Drug Reward, Mesolimbic Tyrosine Hydroxylase, and Clock Gene Expression in the Male Rat. J. Biol. Rhythms 24 (6), 465–476. doi:10.1177/0748730409346657
Yoo S.-H., Yamazaki S., Lowrey P. L., Shimomura K., Ko C. H., Buhr E. D., et al. (2004). PERIOD2::LUCIFERASE Real-Time Reporting of Circadian Dynamics Reveals Persistent Circadian Oscillations in Mouse Peripheral Tissues. Proc. Natl. Acad. Sci. U.S.A. 101 (15), 5339–5346. doi:10.1073/pnas.0308709101
Zhao H., Rusak B. (2005). Circadian Firing-Rate Rhythms and Light Responses of Rat Habenular Nucleus Neurons In Vivo and In Vitro. Neuroscience 132 (2), 519–528. doi:10.1016/j.neuroscience.2005.01.012
Zweifel L. S., Parker J. G., Lobb C. J., Rainwater A., Wall V. Z., Fadok J. P., et al. (2009). Disruption of NMDAR-dependent Burst Firing by Dopamine Neurons Provides Selective Assessment of Phasic Dopamine-dependent Behavior. Proc. Natl. Acad. Sci. U.S.A. 106 (18), 7281–7288. doi:10.1073/pnas.0813415106
Keywords: dopamine, extra-SCN oscillators, circadian clock, ventral tegmental area, substantia nigra pars compacta, timescale, dopaminergic system, multi-clock model
Citation: Pradel K, Drwięga G, Chrobok L and Błasiak T (2022) Racing and Pacing in the Reward System: A Multi-Clock Circadian Control Over Dopaminergic Signalling. Front. Physiol. 13:932378. doi: 10.3389/fphys.2022.932378
Received: 29 April 2022; Accepted: 07 June 2022;
Published: 23 June 2022.
Edited by:
Etienne Challet, Université de Strasbourg, FranceReviewed by:
Andrew Steele, California State Polytechnic University, Pomona, United StatesRalph E Mistlberger, Simon Fraser University, Canada
Copyright © 2022 Pradel, Drwięga, Chrobok and Błasiak. This is an open-access article distributed under the terms of the Creative Commons Attribution License (CC BY). The use, distribution or reproduction in other forums is permitted, provided the original author(s) and the copyright owner(s) are credited and that the original publication in this journal is cited, in accordance with accepted academic practice. No use, distribution or reproduction is permitted which does not comply with these terms.
*Correspondence: Lukasz Chrobok, bHVrYXN6LmNocm9ib2tAYnJpc3RvbC5hYy51aw==; Tomasz Błasiak, dG9tYXN6LmJsYXNpYWtAdWouZWR1LnBs
†These authors have contributed equally to this work