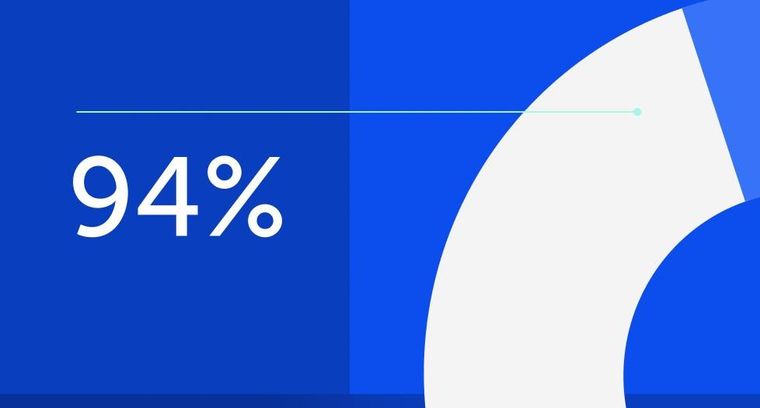
94% of researchers rate our articles as excellent or good
Learn more about the work of our research integrity team to safeguard the quality of each article we publish.
Find out more
REVIEW article
Front. Physiol., 09 September 2022
Sec. Invertebrate Physiology
Volume 13 - 2022 | https://doi.org/10.3389/fphys.2022.928013
This article is part of the Research TopicMethods and Applications in Invertebrate PhysiologyView all 12 articles
Current understanding of cephalopod digestive tract physiology is based on relatively “old” literature and a “mosaic of data” from multiple species. To provide a background to the discussion of methodologies for investigating physiology we first review the anatomy of the cephalopod digestive tract with a focus on Octopus vulgaris, highlighting structure-function relationships and species differences with potential functional consequences (e.g., absence of a crop in cuttlefish and squid; presence of a caecal sac in squid). We caution about extrapolation of data on the digestive system physiology from one cephalopod species to another because of the anatomical differences. The contribution of anatomical and histological techniques (e.g., digestive enzyme histochemistry and neurotransmitter immunohistochemistry) to understanding physiological processes is discussed. For each major digestive tract function we briefly review current knowledge, and then discuss techniques and their limitations for the following parameters: 1) Measuring motility in vitro (e.g., spatiotemporal mapping, tension and pressure), in vivo (labelled food, high resolution ultrasound) and aspects of pharmacology; 2) Measuring food ingestion and the time course of digestion with an emphasis on understanding enzyme function in each gut region with respect to time; 3) Assessing transepithelial transport of nutrients; 4) Measuring the energetic cost of food processing, impact of environmental temperature and metabolic rate (flow-through/intermittent respirometry); 4) Investigating neural (brain, gastric ganglion, enteric) and endocrine control processes with an emphasis on application of molecular techniques to identify receptors and their ligands. A number of major knowledge lacunae are identified where available techniques need to be applied to cephalopods, these include: 1) What is the physiological function of the caecal leaflets and intestinal typhlosoles in octopus? 2) What role does the transepithelial transport in the caecum and intestine play in ion, water and nutrient transport? 3) What information is signalled from the digestive tract to the brain regarding the food ingested and the progress of digestion? It is hoped that by combining discussion of the physiology of the cephalopod digestive system with an overview of techniques and identification of key knowledge gaps that this will encourage a more systematic approach to research in this area.
The digestive system (DS) in cephalopods includes an epithelium-lined muscular tract (DT), and glands (salivary glands and digestive gland [DG]± appendages). The DS physically and chemically degrades food, absorbs nutrients and processes them for metabolism. The DG has a major role in detoxification of ingested potential toxins (e.g., domoic acid, metals) but the “barrier function” of the epithelium also contributes to defence of the organism. The DS is also responsible for excretion of waste products (faeces, including mucus and sloughed epithelial cells) and voiding of undigested and indigestible matter (i.e. faeces, vomit).
Studying the physiology of the cephalopod DS can be justified from a pure science perspective, particularly contributing to comparative and evolutionary studies. For example, comparison with fish as the main predators of cephalopods in the same ecological niches has been considered mainly from a brain evolution perspective (e.g., Ponte et al., 2021), but this should be broadened to include the DS which processes food to “fuel” brain tissue. In “Cephalopods and fish: The limits of convergence,” Packard (1972) commented on similarities in relation to the caecum but other comparisons between fish and cephalopod digestive tracts are unexplored. There are also applied research justifications to consider: 1) Optimising diets at all life stages in aquaculture; 2) Understanding the consequences of dietary change in the wild; 3) Understanding adaptations to environmental change; 4) Assessing the impact of ingestion of plastics and other contaminants on the digestive tract, health and welfare; 5) Animal welfare in the laboratory, public display and aquaculture (see Sykes et al., 2017, for detailed discussion).
This review considers techniques used to study the physiological processes underlying key functions of the digestive system in cephalopods with a particular focus on octopuses as these have been the subject of most recent studies.
To provide a background we briefly describe the range of food eaten by cephalopods and then the anatomy of the cephalopod DT highlighting major species differences.
Villanueva et al. (2017) commented that food sources ranged from “detritus to birds.” All cephalopods are carnivorous with cephalopods, fish, gastropods and crustacea amongst the most common foods depending upon the species and habitat. Chemically, the diet is dominated by proteins and lipids. However, field studies have widened the range of food types to include for example, gelatinous fauna by the giant deep-sea octopus (Haliphron atlanticus, Hoving and Haddock, 2017) and various types of detritus (e.g., faecal pellets, gelatinous zooplankton) by vampire squid (Vampyroteuthis infernalis, Hoving and Robison, 2012).
The digestive system also deals with non-prey items. Examples include, microplastics in the water, adherent to, or ingested by the prey. Evidence for microplastic ingestion has been published for wild and cultured cuttlefish (Sepia officinalis, Oliveira et al., 2020), common octopus (Octopus vulgaris, Pedà et al., 2022), jumbo squid (Dosidicus gigas: Braid et al., 2012; Rosas-Luis, 2016), vampire and mid-water squid (V. infernalis and Abralia veranyi, Ferreira et al., 2022). Although no adverse effects are reported, the potential for harm, particularly from obstruction of the ducts linking the caecum and digestive gland is considerable (for discussion see Oliveira et al., 2020). Plant matter (e.g., seaweed, bull kelp, eelgrass) has been found in the cephalopod digestive tract (Boucaud-Camou and Boucher-Rodoni, 1983; Braid et al., 2012). In theory, non-prey items could be digested by enzymes, ejected unchanged by defaecation or vomiting/regurgitation or remain in the tract where, if accumulated, they could cause an obstruction.
Food ingestion will be accompanied by seawater, potentially containing dissolved or suspended chemical contaminants. The first report of metals in the cephalopod DG was in 1975 (Martin and Flegal, 1975; Bustamante et al., 1998) with high levels of cadmium, copper, iron, silver and zinc in Loligo opalescens, Ommastrephes bartrami, and Sthenoteuthis oualaniensis. The metal concentrations were considered sufficient to be toxic. Bustamante et al. (2006) reviewed the DG and muscle mercury content in 20 cephalopod species concluding that the DG did not store mercury, but demethylated it allowing accumulation in organic form in muscle. Rodrigo and Costa (2017) identified the DG as the main metal accumulation organ and concluded that metallothionines were not the only detoxification mechanism with spherulae (high MWt. protein and metal association) chelating metals (see also, Bustamante et al., 2006). B-esterases have recently been implicated in metabolism of plastic additives in the DG of O. vulgaris and S. officinalis (Omedes et al., 2022).
The biochemical pathways involved in processing metals and other potentially toxic chemicals (e.g., domoic acid) in the DG, their subsequent distribution to other tissues (e.g., brain, muscle) and the functional consequences (e.g., impaired metabolism, modified neuronal function) require further study, especially considering the important role of cephalopods in the marine ecosystems.
Sea water drinking occurs in marine teleosts and elasmobranchs and is an important component of fluid and ionic homeostasis (for review see: Grosell, 2010; Takei, 2021). Haemolymph osmolarity measured in O. vulgaris, O. insularis and O. ocellatus ranged from ∼940 to ∼1170 mOsm/kg (Amado et al., 2015; Sakamoto et al., 2015) so is slightly hypo-osmotic or iso-osmotic with sea water. The values are comparable to marine osmoconforming fish but considerably higher than the osmoregulating teleosts (Takei, 2021). Wells and Wells (1989; 1993) investigated water uptake in O. vulgaris and concluded that the digestive gland appendage (“pancreas”) is one site of fluid and ion transport. Of relevance to this is the finding in squid (L. vulgaris) and cuttlefish (S. officinalis) hatchlings (Hu et al., 2010) that the appendage shows Na+/K+ ATPase immunoreactivity; this is significant as this ion exchange pump is responsible for generating the local osmotic gradient in the lateral intercellular spaces responses for transepithelial water transport but there are no physiological studies of fluid/ionic transport in cephalopod gut. Wells and Wells (1989, p. 219) describe rectal ingestion of sea water in O. vulgaris and Mangold and Young (1998) include “intake of seawater” in their list of intestinal functions in cephalopods. Furthermore, Bidder (1950) reports that water is drawn into the intestine of “larval” L. vulgaris and that this may occur in adults monitored by the processes of specialised rectal cells and involving the anal leaflets. These latter observations require investigation using modern physiological techniques.
The structure and function of organs is related. As a background, we briefly describe the anatomy of the digestive tract in O. vulgaris, highlighting poorly understood aspects in relation to function and then discuss the main anatomical differences from other cephalopods to emphasise the necessity to study a variety of species.
Table 1 summarises publications describing the gross anatomy of the digestive system in a range of cephalopod species and also provides references to specific topics of relevance to understanding physiology.
TABLE 1. Selected key publications that include detailed descriptions of the anatomy and histology of the digestive system and its innervation in a range of cephalopod species.
The buccal mass comprising the beak, radula and associated muscles is the point at which food enters the body with the size of the bolus determined by the gape of the beak and the bite force. The food is mixed with secretions from the anterior and posterior salivary glands which contain digestive enzymes but also in the case of the posterior glands toxins used to subdue or kill prey (see below for details and Ponte and Modica, 2017).
From the buccal mass, pieces of food are propelled along the cuticle lined oesophagus which passes between the sub- and supra-oesophageal lobes of the brain linked by the connectives. The distensibility of the oesophagus and the encircling brain tissue will limit the bolus size together with the compressibility of the food by the oesophageal muscle which in its proximal part has functional characteristics of striated muscle (Andrews and Tansey, 1983a).
Following passage along the oesophagus, the bolus enters the crop with very limited evidence for a sphincter between the two structures (for details see Sykes et al., 2020). Diagrams of the digestive tract in O. vulgaris (see Figure 1) sometimes show a bridge of tissue linking each posterior salivary gland to the anterior crop and this can also be seen in published pictures (for O. vulgaris see fig. 1 in Baldascino et al., 2017; for O. americanus see fig. 3 in Avendaño et al., 2020). Although this structure appears like a duct transporting salivary secretions into the crop, this has not been demonstrated (G. Ponte and P.L.R. Andrews, unpublished observations). Studies of this structure should be undertaken in paralarvae to investigate patency as the structure in adults may be vestigial. Another possibility is that the connexion serves to hold the salivary glands in position as the crop fills.
FIGURE 1. The diagram on the left shows the digestive tract of Octopus vulgaris. Note that the posterior salivary glands are connected to the buccal mass by a duct which delivers saliva containing digestive enzymes and toxins for injection into prey and each gland is also connected to the crop by a duct-like structure (see text for discussion). The labelling of the original is retained with the numbers indicating sites at which the tract was lesioned/ligated for studies of water uptake. Reproduced from Wells and Wells, 1989, p.217 with permission from The Company of Biologists, Cambridge, United Kingdom. The photograph on the right is taken from Baldascino et al., 2017 (Figure 1) and shows the posterior salivary glands (psg) in Octopus vulgaris and the connections (arrowed only on the left) to the anterior crop (Cr). The oesophagus (oes) detached from the buccal mass is also visible.
When distended with food, the crop can be seen to have a more distensible anterior half (see Figure 2) which accommodates ∼75% of the content and a narrower posterior part connecting to the gastric vestibule (Andrews and Tansey, 1983a) with no strong evidence for a sphincter. The gastric vestibule is one of two thin layers of muscle holding the two thick muscle blocks closely apposed and responsible for the movement of one thick block against the other. The stomach has a relatively thick cuticle lining. The anatomical similarity of the stomach in octopus (and to a lesser extent cuttlefish) to that of the koilen-lined “gizzard” present in some species of bird was first noted by Aristotle (1910). The gastric vestibule connects with both the spiral caecum and the intestine with evidence for sphincters between these structures lacking. The major enzymes responsible for digestion (see below) originate in the digestive gland, are passed into the caecum (DG ducts) from where they can enter the gastric vestibule to contact the food. The capacity of the stomach in octopus is small in relation to the crop so only relatively small boluses of food can enter for trituration while enzymes continue to act and then the partially digested food progresses to the either caecum or intestine. Alternatively, the crop and stomach may act as a single functional unit with food interchanged between the “hopper” (crop) and “mill” (stomach) until it is physically and chemically degraded and is passed to the caecum or intestine. Currently, there is no definitive evidence to decide between the two, not incompatible, options. The common cavity between the crop, stomach, caecum and intestine means that contents can readily interchange depending upon the intraluminal pressure gradient generated by the muscles in each region.
FIGURE 2. The digestive tract removed from an Octopus vulgaris weighing 900 g. The main anatomical regions are labelled including the gastric ganglion (GG). Double headed white arrows indicate the potential for bi-directional exchange of material due the existence of a common cavity. For the anal opening although only unidirectional flow (defaecation) is indicated there is some evidence for the entry of sea water via this route (see text for discussion). Note the “teardrop” shape of the crop distended with food (primarily an octopus arm), the relatively thin and thick parts of the stomach, the caecal lamellae visible through the thin wall, the presence of aggregata in the intestine, and the location of the GG with nerve radiating to adjacent structures.
In O. vulgaris, the oesophagus, crop and particularly the stomach are all lined with a cuticle. The cuticle protects the underlying epithelium against abrasion by ingested prey (e.g., crab carapace and limbs, fish bones) and in the stomach provides a surface for trituration. The presence of a continuous, variable thickness, layer of cuticle makes it highly unlikely that the oesophagus, crop or stomach has an absorptive function. However, it should be noted that limited evidence has been provided for uptake of radio-labelled glycine/leucine by the crop in Nautilus pompilius (Westermann and Schipp, 1998a) and O. vulgaris (Wells, 1978) but uptake into the epithelium does not provide evidence for transepithelial transport.
The caecum is a thin-walled muscular tube, coiled into about two and a half turns forming a cone and lined with lamellae which will increase the surface area. It is stated that the caecum filters food from the stomach “discarding gross particles that pass directly to the intestine” (Anadón, 2019, p. 4). Whilst this is a likely function, together with the addition of mucus to begin formation of faecal ropes, there are no direct studies in octopus demonstrating this but studies of the caecum in squid (Bidder, 1950) are supportive. Additionally, cilia have been to proposed to be responsible for the movement of “fine particles in suspension” from the caecum into the digestive gland (Fernández-Gago et al., 2019, p. 331) but no studies have investigated the relative roles of the cilia, caecal muscle constricting the cone or DG duct peristalsis in this process. The large surface area of the caecum is also consistent with an absorptive function, but Fernández-Gago et al. (2019, p. 331) concluded that in O. vulgaris that there was “no clear evidence for absorption in the caecum” although Boucaud-Camou et al. (1976) considered it was involved in absorption as is also the case in N. pompilius (Westermann and Schipp, 1998b). In the squid the caecum/caecal sac is proposed to have a major role in absorption (Boucher-Rodoni and Mangold, 1977; Boucaud-Camou and Boucher-Rodoni, 1983). Published studies used uptake of radiolabelled molecules by the epithelium or the presence of lipid containing vesicles in the epithelium but have not demonstrated transepithelial transport into the haemolymph from the caecum and this is therefore a major knowledge gap.
The control of the directionality of movement of contents between the common cavities of the crop, stomach and vestibule, caecum (and hence the DG duct) and proximal intestine has not been investigated. Evidence for the presence of sphincters is inconclusive or absent in octopus although there may be a flap-valve between the caecum and the digestive gland duct at the tip of the columella (Budelmann et al., 1997; see figure 64A, p. 197) and a thickening of circular muscle at the exit of the ink duct and anus is consistent with it acting as a sphincter (Young, 1967).
The tubular intestine shows little external variation in octopus throughout its course from the stomach vestibule/caecal junction to the anal opening, but turns anteriorly in the middle of its course. Internally, the mucosa has longitudinal folds, two of which on opposite sides are larger forming a “typhlosole” in the proximal intestine. It is assumed that the function of the typhlosole is to increase surface area and whilst this is a logical assumption the question of the reason why a surface area increase is required has not been answered; usually this is to facilitate absorption but it could also be related to secretory capacity (e.g., mucus). It is reported that the epithelium of the typhlosoles is ciliated but that of adjacent intestinal epithelial tissue has a luminal border with microvilli (Anadón, 2019; Fernández-Gago et al., 2019) suggesting a differentiation of function. Ciliary motion would be capable of moving mucus with trapped particles so investigation of the direction of ciliary beating in fresh specimens would be of particular interest. The function of the typhlosoles is not established, but constriction of the circular muscle would lead to the division of the lumen into two channels by apposition of the typhlosoles. A further issue regarding intestinal function in octopus is the extent to which it is involved in transepithelial transport of nutrients, ions and water which has not been investigated directly. In Loligo limited evidence was presented by Bidder (1950) for some absorption in the intestine while caecal absorption is in progress noting that the intestine may have a more major role when the gonads enlarge, limiting filling of the caecal sac. The capacity to absorb nutrients from contents bypassing the caecum/digestive gland but which have been exposed to digestive enzymes would be an advantage, as would involvement in water and ion homeostasis.
The anal opening in O. vulgaris has paired muscular anal flaps or wings. Their absence in species lacking an ink sac suggests involvement in inking rather than a digestive tract function but further investigation is required. The flaps are innervated by the atrio-rectal nerve branch of the visceral nerve and Young (1967, figure 18, p. 9) noted the presence of a plexus of fine fibres in the flap commenting “perhaps afferent.”
The faeces are formed as mucus coated ropes but their composition has not been studied in any detail and the mechanisms, presumed to be neural, controlling of defaecation are unknown (see Ponte et al., 2017 for discussion).
The toxicity of the exocrine secretions from the posterior salivary glands of O. vulgaris was first noted by Lo Bianco working at the Stazione Zoologica Naples, in the early C19th but the toxic fraction was not identified until 1959 (Ghiretti, 1959). Two main constituents were identified with differing biological effects on crabs; amines inducing hyperexcitability and cephalotoxin, lethality. Cephalotoxin is a glycoprotein now known to occur in α and β forms (Cariello and Zanetti, 1977). The current view is that the saliva is a chemical cocktail including tachykinins (i.e. Eledoisin from Eledone, Erspamer and Anastasi, 1962), OctTK 1, phospholipase A2, other proteins, and enzymes (e.g., peptidase, hyaluronidase, chitinase) that also play key roles in toxicity of coleoid venom, supplemented by other small organic molecules, peptides, and non-enzymatic proteins (for review see: Ruder et al., 2013; Gonçalves and Costa, 2021).
Analysis of the phylogenetic history and molecular evolution of coleoid venom shows comparable diversity and complexity with those found in snakes (Ruder et al., 2013) with evidence of active evolution of some constituents (e.g., cysteine-rich pacifastin and kallikrein). Characterization of the molecular structure and composition of cephalotoxins is still incomplete (maybe except for Sepia esculenta cephalotoxin, Ueda et al., 2008). Apart from the glycosylation of the protein, characterization allowed the identification of conserved domains: EGF-like, Sushi, TSP type-1 and LDL-receptor class A (review in Gonçalves and Costa, 2021). Of particular interest is the epidermal growth factor (EGF) domain known to paralyse crustaceans via Nav block; cephalotoxins, should be described as EGF neurotoxins. The increasing availability of cephalopod genomes has provided insights about tissue-specific genes expressed in the posterior salivary gland of three cephalopod species (non-tetrodotoxin-bearing octopods vs. tetrodotoxin in Hapalochlaena maculosa; Whitelaw et al., 2020).
Although cephalopod salivary gland research is focused on the nature of the toxins and their biological effects little is known about the physiological mechanisms involved in their secretion, how the ratio of the various constituents is determined, and the neural control of secretion together with the transport of saliva along the ducts. The discrete nature of the salivary glands makes them suitable for in vitro studies of intact glands and subsequently isolated cells comparable to those applied to mammalian salivary glands.
Knowledge of the physiological consequences of structural similarities and differences in the digestive tract between species (“functional morphology,” Mangold and Young, 1998) is essential to understanding how the animal adapts to its ecological niche. Mangold and Young (1998) and Voss (1988) utilised digestive tract morphology for systematics and revealing phylogenetic relationships. A recent review of the structure and function of the digestive system in molluscs makes more general comparisons (Lobo-da-Cunha, 2019).
The crop is present in Nautiloidea, Vampyromorpha and in most Octopoda but may be reduced or absent in some cirrates (e.g., Cirrothauma, Aldred et al., 1983) with Voss (1988) proposing that this is related to a diet of small, soft-bodied prey. Naef (1928) includes a crop in their diagram of a “primitive hypothetical cephalopod.” The crop is absent in Sepiodea and Teuthoidea; molecular techniques should be able to identify the gene(s) responsible. As the primary function of the crop is storage, its absence in Sepiodea and Teuthoidea arguably limits their ability to optimise feeding opportunities unless the stomach is adapted to compensate by being more sacculated and distensible than the stomach in Octopoda.
A caecum of variable complexity is present in Nautiloidea and Coleoidea but a caecal sac is only present in Teuthoidea and even then, not all Oegopsida (Mangold and Young, 1998) although the functional implications are unknown. The complexity of lamellae in the caecum is less in cuttlefish compared to octopus and squid (Anadón, 2019).
In contrast to the poor evidence for structures (sphincters or valves) in the octopus DT there are more detailed descriptions in L. vulgaris. Williams (1909) describes a “very remarkable five-way valve” (p. 36) between the caecum and neighbouring cavities of the gut and also sphincters at the openings from the oesophagus and the stomach. Attached to the caecal wall, Bidder (1950) describes a solid valve structure reinforced by cartilage with an innervated “sphincter-like” muscle surrounding its edge and capable of regulating the passage of fluid into, or out of, the caecum at various phases of digestion. Additionally, the sac-like posterior caecum can be separated from the more complex anterior portion (appendix, ciliated organ accessible by the digestive gland and intestine) by a “diaphragm-like” sphincter (Bidder, 1950).
Regarding the intestine, the principle difference visible externally is the presence of coils or loops (Mangold and Young, 1998) occurring approximately half-way between the junction with the caecum and anal opening. These are present in Sepioidea, Nautiloidea and Octopoda although variably in the Cirrata (e.g., absent in Cirrothauma; Aldred et al., 1983) and absent in Teuthoidea and Vampyromorpha. In species with loops and coils the overall intestinal length will be relatively longer in relation to body size but we do not know if the proportions of intestine, rectum and anal regions differs. Loops and coils in the intestine increase the overall luminal surface area for absorption so may indicate differing absorptive capacity. Coiling also increases the relative length of the intestine in relation to body size and potentially the time that digesta spends in the digestive tract, but formal studies supporting this hypothesis are lacking. There have been no comparisons of the coiled area with adjacent regions to investigate structural specialisations and there is insufficient data to identify potential relationships between food type, morphological differences in other structures (e.g., ± crop, ±caecal sac) and intestinal complexity. Internal differences in the intestine have not been reported systematically but transverse septa are present in the proximal intestine of Nautilus (Budelmann et al., 1997) but not octopus and the typhlosole is more complex in Nautilus.
The above brief descriptions are from a relatively limited number of species but demonstrate that that it would be unwise to extrapolate data on physiology of the digestive tract from one species to another.
Post mortem dissection of fresh specimens is the classical method for describing the gross anatomy. In fresh specimens, it is possible to view and measure the distribution of contents and classify their nature, i.e. liquid, solid and colour (Figure 2); note a description of changes in squid stomach contents with time in Wallace et al. (1981) and Boucher-Rodoni (1976) for caecal contents in Eledone cirrhosa. Care should be taken in interpretation as the method of killing may modify digestive tract motility (e.g., brain destruction will stimulate neurones; magnesium chloride relaxes smooth muscle; Bacq, 1934) and contractions may occur post mortem redistributing contents, particularly liquids. Guidance on dissection techniques is given in Øresand and Oxby’s (2021) illustrated guide to the dissection of the bobtail squid, Sepietta oweniana and by Guerra (2019) for several species.
Useful data can also be obtained from dissection of fixed specimens but distortion can occur and fixation of entire (larger) animals is a challenge. Freezing intact animals immediately post mortem preserves the tissue and maintains anatomical relationships.
Gross dissection provides largely descriptive, qualitative information on the morphology of the digestive system enabling comparison between species (see above). However, quantification of the wet weight of identified tissues provides data enabling further differentiation of species, assessment of the impact of different diets (e.g., natural vs. prepared/synthetic/elaborated) and investigation of potential correlations with environmental changes.
Most DS organ weight data is available for the DG; for example: six decapodiform cephalopods (Omura and Endo, 2016), O. vulgaris (García-Garrido et al., 2010; Bastos et al., 2020), Octopus maya and Octopus mimus (Linares et al., 2015; Pascual et al., 2019) with more limited data for the stomach and caecum (e.g., Omura and Endo, 2016). Detailed analysis of digestive gland weights has shown changes in the weight of the digestive gland in O. maya with season and location (Pascual et al., 2019).
Ideally, data on dry weight should also be obtained to differentiate between increase tissue mass compared to increased water content which may be pathophysiological (e.g., oedema).
Dissection is a destructive process so should only be used when there are no other methods available. For example, high-field magnetic resonance imaging and micro-computed tomography have been used for external and internal taxonomic description of a preserved specimen of a novel species of dumbo octopus (Grimpoteuthis imperator, Ziegler and Sagorny, 2021). The resolution of the digestive system was sufficient to identify all key features (e.g., presence of crop and “pancreatic” portion of the DG, paired hepatic ducts) and also to note that there was only a single posterior salivary gland and the anal flaps were absent (as was the ink sac). Importantly, collection of this type of imaging data enables construction of 3D models of the viscera so that the relationships of structures can be readily examined. High resolution ultrasound can also be used to image tissue in preserved animals but it has particular utility for anatomical and physiological studies in living animals so is discussed below.
The following are selected examples to illustrate the potential contribution of histological techniques to understanding physiology.
The muscle layers and the presence of connective tissue has mainly been investigated using haematoxylin and eosin (H&E) and Masson’s trichrome (e.g., Anadón, 2019) with a few studies using transmission electron microscopy (reviewed by Budelmann et al., 1997). Histology enables identification of the muscle type (e.g., smooth or striated), the orientation (e.g., circular, longitudinal, oblique) and thickness of the layers in the various regions. This knowledge has functional implications as smooth and striated muscles have different physiological properties. When the muscle is relatively thin it is suggestive of areas of storage and when thicker, indicative of more powerful contractions or sphincter areas. As noted above, evidence for the presence of sphincters in the digestive tract is limited and is an area where histological studies can inform understanding of control processes. The complex valves involving both muscle and mucosal modifications reported in squid (Williams, 1909; Bidder, 1950) require histological investigation particularly to investigate the claim (Bidder, 1950, p. 18) that they are “reinforced by cartilage.”
The basic organization of the muscle in the cephalopod digestive tract is an inner layer of longitudinal muscle and an outer layer of circular muscle with some regions having an additional outer longitudinal layer (e.g., O. vulgaris oesophagus, Fernández-Gago et al., 2019) or a circular layer mixed with oblique muscles (e.g., thick stomach muscle in E. megalocyathus, Garri and Lauria de Cidre, 2013). Significantly, this contrasts with the vertebrate digestive tract where broadly the organisation is an inner circular muscle layer and an outer longitudinal one (Furness, 2006); the functional consequences particularly for the mechanisms underlying peristalsis require investigation.
Using H&E, Fernandez-Gago et al. (2019, p. 324, table 1) surveyed the epithelial cells secreting mucus or a granular secretion throughout the digestive tract of O. vulgaris and similar data is available for E. megalocyathus (Garri and Lauria de Cidre, 2013). Mucus secretions were classified using alcian blue (acidic/sulphated/carboxylated glycoconjugates) and periodic acid Schiff (neutral glycoconjugates) alone or in combination. Of particular physiological relevance is the differential distribution of the various types of mucus secreting cells in the lamellae and first and second coils of the caecum (Fernández-Gago et al., 2019) in O. vulgaris.
Further insights into secretions are gained from studies of ultrastructure (e.g., electron dense granules, rough endoplasmic reticulum) and enzyme immunohistochemistry (Westermann and Schipp, 1998a). In N. pompilius digestive tract the most prominent enzymes were acid and alkaline phosphatase and β-glucuronidase, the latter implicated in mucus catabolism but chymotrypsin and trypsin-like enzymes implicated in nutrient digestion were also present (Westermann and Schipp, 1998a).
In the DG marked quantifiable histological changes occur during the course of digestion. DGs showing large numbers of “boules” are considered to be active as these contain digestive enzymes ready for exocytosis (Bidder, 1957). The number of “boules” decreases with time after feeding and are absent in one to 3 days without food in O. vulgaris (Bidder, 1957). Brown and grey bodies associated with the excretion of waste products are seen during the excretory phase (Bidder, 1957; Rodrigo and Costa, 2017).
The innervation of the digestive tract in cephalopods has been relatively neglected since the detailed anatomical and histological studies by, for example, Alexandrowicz (1928), Bogoraze and Cazal (1946) and Young (1967; 1971) with recent studies only commenting in passing on the presence of neurones (e.g., Anadón, 2019; Fernández-Gago et al., 2019). Here we use studies of the gastric ganglion to exemplify the contribution of histological techniques. The gastric ganglion is a prominent oval structure (∼3 mm long in a 500 g – body weight - O. vulgaris) located on the external surface of the digestive tract at the junction of the stomach, caecum and intestine (Figure 2). It is composed of a cortical layer of cells with an inner neuropil from which nerve fibres emerge to supply all adjacent regions of the DT including the digestive gland ducts. The neuronal organisation of the ganglion has been investigated using cresyl violet (Andrews and Tansey, 1983a), Picro-Ponceau (Baldascino et al., 2017), Golgi-Cox (Spasiano, 2020) various modifications of Cajal’s silver stain methods (Young, 1971) and antibodies for neurofilament 200, neuronal marker acetylated alpha-tubulin and neuronal nuclear antigen (NeuN, Baldascino et al., 2017). The overall organisation of the cells and the connectivity of axons and dendrites closely resembles the organisation of the central nervous system, supporting the view that it is a major peripheral control centre for the digestive tract. Conventional, fluorescence and immuno-histochemistry (including for the presence of synthetic or destructive enzymes) have identified the presence (usually by “like-immunoreactivity”) of potential neurotransmitters including acetylcholine, corticotrophin-releasing factor, dopamine, FMRF-amide, gamma amino butyric acid, 5-hydroxytryptamine, nor-adrenaline, octopamine (see Baldascino et al., 2017 for methodological details and references); the functions of these substances now need investigating using the physiological techniques discussed below. Although we have focused on the tract itself there is evidence that the vasculature supplying the tract is innervated (Andrews and Tansey, 1983b) which is of importance in matching blood flow changes to the post prandial metabolic demands.
The majority of the in vivo techniques will be regulated by national legislation (e.g., Directive 2010/63/EU) and/or institutional review. Collection of animals from the wild may also require specific authorisation. Removal of digestive tract tissue for study in vitro requires a humane method of killing (see Andrews et al., 2013; Fiorito et al., 2015) and although the EU legislation does not currently specify methods for cephalopods, unlike the situation for vertebrates, in Annexe IV the principles to be followed are specified. For all studies involving animals, researchers should carefully consider application of the 3Rs (Fiorito et al., 2014), experimental design, for example by using the Experimental Design Assistant, and consult animal experimentation reporting guidelines such as ARRIVE (Kilkenny et al., 2010; Percie du Sert et al., 2020). Some research on the cephalopod digestive system is of direct relevance to aquaculture so note should be taken of the growing concerns regarding welfare (Jacquet et al., 2019; Birch et al., 2021).
Motility describes the way the contraction and relaxation of the muscles of the digestive tract move the secretions (including salivary and DG ducts), the food whilst it is digested and absorbed, and any indigestible components, waste products, adherent mucus and shed epithelial cells.
Whole DT transit time is usually taken as the time from when food enters the gut to when the undigested constituents of that meal leave the DT as faeces but it can also be applied to the time taken for an artificial marker to pass through the DT. Although conceptually it is a simple measurement, the value obtained depends upon the physical and chemical nature of the food, the physiology of the DT which may be influenced by external stressors, ambient temperature in poikilothermic species and any conscious control over motility such as deferral of defaecation. Although whole digestive tract transit time provides some information about the physiology of digestion it gives little insight into each region’s contribution to the overall time and hence its function (e.g., how long do different foods spend in the stomach?). Measurements of overall and regional times are required when investigating control of the digestive tract as well as quantifying the impact of different diets and environmental changes (e.g., temperature). Two main methods have been used.
The movement of shrimps (Crangon crangon) labelled with barium sulphate was monitored in juvenile N. pompilius by taking timed X-ray photographs (Westermann et al., 2002). Although this technique allows non-invasive monitoring of food movement once the shrimps lose their integrity the barium will disperse into the liquid contents of the tract so may move at a different rate from the solid components. Indigestible food entered the rectum 4 h after feeding but spent a further 8 h in the rectum before defaecation; the overall duration of digestion is assessed at 12 h (at 18–19°C). This technique has not been used in other cephalopods but is applicable (particularly to cuttlefish) provided humane methods of restraint during imaging can be developed. X-rays can also be used to monitor the passage of solid radio-opaque markers of different size incorporated into the food.
This usually involves feeding matched (e.g., weight, sex) groups of animals a known amount of food after a period of food deprivation, e.g., 24 h for O. vulgaris or S. officinalis (see Sykes et al., 2017 for assessment of welfare impact), killing groups at fixed times after feeding and measuring the amount and composition (e.g., pH, enzyme activity, lipid and protein content) of contents in the lumen of each region of the tract; some studies also include biochemical analysis of the digestive gland and haemolymph sampling. The change in the amount and composition of digesta in each region of the gut (and potentially the faeces) can be used to assess the overall time taken to digest food and the contribution of each region. This approach has been used in Octopus maya, O. mimus and O. vulgaris type II from Brazil (Martínez et al., 2012; Linares et al., 2015; Gallardo et al., 2017; Bastos et al., 2020).
Using standardised food intake this method can also be used to estimate the rate at which food leaves the stomach as shown in cuttlefish (Quintela and Andrade, 2002a; b). With a shrimp meal gastric emptying in S. officinalis was shown to be faster at higher ambient temperatures; the time for 50% of the meal to be emptied from the stomach was estimated to be 3.5 h at 15.5°C, but 1.6 h at 23°C (Quintela and Andrade, 2002a) but this method does not allow the relative contributions of the effects of higher temperature on enzyme activity and motility to be identified. Techniques need to be developed to measure the emptying of the solid and liquid components separately as liquid components are likely to empty more quickly; in cephalopods this may be important as in octopus soluble nutrients enter the digestive gland and haemolymph ∼ 40 min post ingestion (Rosa et al., 2004; Linares et al., 2015). This food distribution method is readlily applicable to meaure emptying from the stomach in cuttlefish and squid where there is no crop, but in octopus where the crop and stomach act in concert other techniques will need to be developed to measure exchange between the two regions as well as net emptying into the caecum/intestine. Finally, a key reason why measurement of gastric emptying is needed is to understand how the process is regulated to ensure that only suitable processed food enters the caecum and the relationship between gastric emptying and satiety/hunger signals.
The main disadvantages of this technique are that it requires the death of the animal, uses a large number of animals depending on the temporal resolution studied, groups need careful matching so data can be combined to produce a single plot of the timing of various processes, contents may move after death because of the interconnectivity of the gut regions and post mortem continuation of motility. However, currently it is the only technique allowing sampling of gut contents (except faeces) enabling detailed biochemical analysis to follow the course of food digestion and also the analysis of exocrine secretions contributing to digestion. If an animal is killed, we urge that optimal use is made of the all the tissues by making them available to other local researchers or making a bank of fixed or frozen tissue; the latter is particularly important for rarer species.
In vitro here refers to studies in which either the entire digestive tract, a region (e.g., the crop, the rectum) or a strip of tissue cut from a region is removed from an animal killed humanely is placed in a tissue bath. Functionality is maintained by immersion in a physiological solution (modified Ringer’s solution), gassed (to maintain oxygen and the pH of any buffers) and kept within normal body temperature range while measurements are made of muscle contractile activity (e.g., tension or intra-luminal pressure). Here we focus on techniques appliable to either segments of digestive tract or the entire digestive tract but do not discuss In vitro techniques applied to studying the working of the buccal mass (Boyle et al., 1979).
With appropriate experimental design and controls the techniques described below can be used to investigate the effects of a range of interventions and conditions applied before the in vitro study but which are likely to have prolonged effects. Examples include: 1) Life stage dependent changes; 2) Effect of protracted exposure to expected changes in environmental conditions associated with climate change (e.g., sea water acidification); 3) Comparison of diets with differing composition, energy density and digestibility; 4) Investigation of functional effects of endogenous agents and/or their receptors which may be identified from genomic studies (e.g., cephalopod G-protein receptors, Ritschard et al., 2019), neuropeptidome analysis (e.g., Zatylny-Gaudin et al., 2016), molecular and immunohistochemical analysis of the gastric ganglion (e.g., Baldascino et al., 2017).
In vitro recordings of tension from various regions of the digestive tract have been undertaken in O. vulgaris (e.g., Andrews and Tansey, 1983a; Takuwa-Kuroda et al., 2003), S. officinalis (e.g., Bacq, 1934; Zatylny-Gaudin et al., 2010) and Doryteuthis pealeii (e.g., Wood, 1969). However, the paucity of studies shows that this is an under-utilized technique in cephalopods. It is also possible to measure pressure in closed isolated segments of digestive tract (Andrews and Ponte, personal observations).
Two insights into the physiology of digestive tract motility emerge from published studies:
All regions of the digestive tract show varying degrees of spontaneous (i.e., in the absence of overt stimuli) contractile activity with contractions of differing amplitudes and frequencies (Figure 3). Myogenic activity (i.e. not requiring neurones) is particularly important to investigate as in vertebrates, including fish (e.g., Myoxocephalus scorpius Brijs et al., 2017b) and humans (Hwang et al., 2009), this is due to activity of the Interstitial Cells of Cajal (ICC) which act as pacemaker cells periodically depolarizing to initiate contraction of adjacent smooth muscle cells (Sanders, 2019). Wood (1969) reported that contractile response of the squid stomach to stretch continued in the presence of tetrodotoxin as did the associated electrical activity providing very preliminary evidence for “myogenic activity.” Functional studies, combined with molecular and immunohistochemical studies (e.g., calcium activated chloride channel ANO-1 positive cells) to investigate the presence of ICCs are required to identify whether the fundamental mechanism (ICC-smooth muscle cell) underlying digestive tract contractile activity in vertebrates occurs in cephalopods.
FIGURE 3. Spontaneous contractile activity recorded in vitro from longitudinal muscle strips of the main regions of the digestive tract removed immediately post mortem from Octopus vulgaris. Note that all regions show some spontaneous contractile activity but that the magnitude and frequency differs. Abbreviations: caec = caecum; cro = crop; gan. gast = gastric ganglion; intest = intestine; rect = rectum. Modified from Figures 1 and 4 in Andrews and Tansey, 1983a, pages 111 and 115. Reproduced with the application of an author permissions waiver request from Cambridge University Press.
Figure 4 shows a stimulatory effect (increased tone, contraction amplitude and frequency) of nor-adrenaline/adrenaline on the crop, thin part of the stomach and intestine while acetylcholine and nicotine inhibited activity which recovered above baseline levels on washing (Andrews and Tansey, 1983a). This provides preliminary evidence for reciprocal control of motility analogous to divisions of the autonomic nervous system in vertebrates (Olsson, 2009). An inhibitory effect of acetylcholine followed by post-washing excitation has also been reported in squid stomach as has the excitatory effect of adrenaline (Bacq, 1934; Wood, 1969). Although responses to the cholinergic and adrenergic receptor agonists are clear, defining receptor types/subtypes involved requires the use of selective or specific receptor antagonists (see IUPHRA/BPS Guide to Pharmacology; www.guidetophamacology.org). Whilst many receptors are relatively well characterized in mammalian tissues very little information is available regarding the pharmacology of receptors in cephalopods defined either by agonists or antagonists.
FIGURE 4. The effects of adreno- and cholino-receptor ligands on the in vitro contractile activity of main regions of the Octopus vulgaris digestive tract. The crop, stomach and intestine all show an increase in contraction amplitude and tone to noradrenaline (1 µg/ml–10 μg/ml) with the response to adrenaline (2 μg/ml) in the crop also shown. Both acetylcholine (ACH; 20 μg/ml) and nicotine (20 μg/ml) cause transient inhibition of ongoing contractile activity in the crop followed by rebound excitation following washing. The upper right -hand panel shows the excitatory effect of gastric ganglion (GG) stimulation (20 V, 0.5 ms, 20 Hz) on the crop and the stimulation of crop motility by fluid distension (2 ml); these two recordings are from in situ preparations. Abbreviations: caec, caecum; cro, crop; gan. gast, gastric ganglion; intest, intestine; rect, rectum. Details of methods are in Andrews and Tansey, 1983a. This Figure is modified from Figures 1, 8, 9 and 10 in Andrews and Tansey, 1983a, pages 111, 119, 121 and 122. Reproduced with the application of an author permissions waiver request from Cambridge University Press.
The external profile of the digestive tract changes with the progression of contractions and also as the degree of distension of a particular segment is changed by bolus progression. High resolution video recording of the digestive tract followed by automated computer analysis of the apparent diameter enables the generation of “spatiotemporal maps” showing the contractile activity, its magnitude, direction (oro-anal or ano-oral) and speed of travel (see Lentle and Hulls, 2018 for review of the method). Spatiotemporal mapping frequently used in mammals to investigate the effects of drugs (e.g., in mouse stomach, Worth et al., 2015) has also been utilised to study motility in the digestive tract of the fish Oncorhynchus mykiss (Brijs et al., 2017a) and Myoxocephalus scorpius (Brijs et al., 2014; Brijs et al., 2017b). However, it has not been applied to cephalopods, although using cursor measurement of individual video frames at timed intervals Sykes et al. (2020; Figure 3B) demonstrated its applicability to analysis of crop contractions using an in vitro preparation in O. vulgaris.
Other techniques also applicable in vitro to investigate the effect of treatments in vivo (e.g., acute food deprivation, adaptation to different diets, pharmacological or surgical interventions to investigate control) on motility measured in vitro include the passage of artificial boluses (e.g., Le et al., 2021), electrophysiological studies of muscle using extracellular (e.g., suction electrodes as in squid stomach, Wood, 1969) and intracellular (adapting techniques used in octopus arm muscle, Rokni and Hochner, 2002) recordings.
Imaging the digestive tract in situ in the living animal (in vivo) allows measurement of the movement of food, changes in the physical nature of the contents through the tract and characterisation of the contractile activity. However, methods used currently require some form of restraint or possibly sedation which may themselves affect motility, although in some species it may be possible to train the animal to remain quiescent during monitoring. Whilst X-rays have been used to monitor the progress of food mixed with radio-opaque barium sulphate in N. pompilius (Westermann et al., 2002) and S. officinalis (Ponte et al., 2017, see also Figure 1H therein) the low temporal resolution does not provide information about individual contractions. Additionally, movement of the contrast medium may not accurately reflect the bolus movement.
The technique most applicable to cephalopods is the use of high-resolution ultrasound (e.g., VEVO - Visualsonics). In a pilot study of unrestrained O. vulgaris, Ponte et al. (2017) were able to show propulsive contractions of the crop in both longitudinal and transverse planes, and constriction of the caecum together with the motion of the complex spirally organised lamellae (see figure 1 in Ponte et al., 2017). The method also provides information about the physical characteristics of the lumen contents. This technique should be adaptable to cuttlefish, although the locomotor activity of squid may prevent use without undue restraint. Combination of in vivo ultrasound with the in vitro techniques outlined above will identify if motility is modified by diets of different composition, and the impact of environmental changes.
The transparency of O. vulgaris paralarvae post-hatching, enables direct observation of the digestive tract during feeding allowing observation of the distribution of food in the digestive tract. Analysis of high-resolution video recordings enabled quantification of crop volume, contraction frequency in crop, stomach and intestine, and insights into the overall timing of digestion (Nande et al., 2017). Juvenile and mature individuals of several species of cephalopod are sufficiently transparent for direct observation of the passage of material through parts of the digestive tract and also to see changes in the colour of the digestive gland. Bidder (1950) utilised the transparency of juvenile squid to make observations on the movement ingested pieces of fish (marked with iron saccharate, carmine and Nile blue) in the digestive tract commenting that “the only parts of the digestive system not visible in the living animal are the buccal glands and poison glands and the “pancreas” (Bidder, 1950, p. 8). Direct observation is not an option for cuttlefish species because of the cuttlebone but there are several examples of transparent/semi-transparent squid (e.g., Cranchiide family) and octopus (e.g., Vitreledonellidae family).
Ingestion rate can be measured in experimental settings using live or frozen organisms, pieces of natural or processed (elaborated/synthetic) food and is calculated as the difference between the delivered food and that remaining for at least 2 h after being offered to the animals. The latter time can be established according to the feeding time regularly used in the laboratory depending on the species feeding habits. The percentage of leached nutrients must be measured and depends on the type of the diet. When processed food is used, leaching is measured using the shaking method (Obaldo et al., 2002). For this, 10 samples of 2 g of each diet are placed in 250-ml flasks placed in a horizontal shaker for 2 h (or the time that is estimated in each experimental condition to feed the animals) at the same temperature and water quality used experimentally. After that time, the water is filtered (pre-weighed, Whatman 1441-090 is recommended) to separate the remaining food from the leached water. Original food (N = 10) and leached samples of each diet are dried in a convection oven at 60°C for 48 h until constant weight, and then cooled in a desiccator. Dried feed samples are weighed and analysed for dry matter retention. Ingestion rate is calculated according to the following equation:
where offered and recovered food are expressed as dry weight (g) (60°C, 48 h), and unconsumed food as % of the nutrients lost during the shaking procedure (Obaldo et al., 2002). Ingestion rate (I) is expressed as g. ingested food (wet weight) considering the water content obtained after dry sampling at 60°C for 48 h. In the case of fresh or frozen food the same method is used. A review of the stomach contents and ingestion rate in cephalopods by Ibáñez et al. (Ibáñez et al., 2021) gives recommendations for obtaining data of ecological importance.
Digestion time differs between species, with temperature, dissolved oxygen and type of food, among other variables. For that reason, when evaluating the digestive processes, preliminary observations are required to determine the overall duration of digestion and hence sampling frequency. In O. vulgaris sensu stricto, O. vulgaris type II from Brazil, O. maya, and O. mimus fed fresh crab at 5% of the body weight it took ∼ 400 min to complete the digestion process (Martínez et al., 2012; Linares et al., 2015; Gallardo et al., 2017; Bastos et al., 2020). Depending on the number of animals available, it is possible to take timed samples during the process, but how many animals should be sampled to maintain a balance between 3Rs considerations and data quality? The answer depends on the type of analysis required, but usually a minimum of three animals will be necessary to obtain data that can be statistically analysed (Table 2). Also, it is essential to remember that digestive juice enzyme activity can be highly variable, especially with a mixed diet. For that reason, in this type of study it is highly recommended to use a single type of food to evaluate digestion timing (crustacea are arguably the best diet because their importance in trophic ecology of cephalopods).
TABLE 2. Number of animals sampled in three octopus species when the timing of the digestive process was studied.
Table 3 shows the variety of digestive enzymes in octopus species. Although there are probably more than a dozen types of enzyme with different roles, there is evidence suggesting that the acidic enzymes have higher activity during digestion (e.g., Ibarra-García et al., 2018). Based on Boucaud-Camou and Boucher-Rodoni (1983), Martínez et al. (2012) studied the pH of the “gastric juice” (i.e., the fluid found in the stomach but not secreted by the stomach) of O. maya during digestion of crab. They found that pH varied between 5.2 and 6, demonstrating, as was previously observed in O. sinensis (?) by Morisita (1972 a, b and c, cited by Boucaud-Camou and Boucher-Rodoni, 1983) that the main enzymes in the digestive tract of those octopus species were acidic proteinases. Until now, besides O. sinensis(?) and O. vulgaris sp. (Table 3) the presence of acidic proteinases has been demonstrated in the gastric juice of O. mimus, O. maya (Linares et al., 2015) and O. vulgaris type II (Bastos et al., 2020), and in the digestive gland of O. bimaculoides (Ibarra-García et al., 2018), Robsonella fontaniana (Pereda et al., 2009) and Enteroctopus megalociathus (Farías et al., 2010) indicating that the acidic proteinases may be the main type of digestive enzyme in octopus species. It is interesting to note that in some studies high activities of amylase are reported suggesting that some species (O. bimaculoides; O. vulgaris sensu stricto) may have the ability to digest complex carbohydrates (Table 3). Although interesting, these studies require duplication (Table 3) and the sources of complex carbohydrate identified.
TABLE 3. Enzyme activities detected with different methods in digestive gland (DG), anterior (ASG), and posterior (PSG) salivary gland, gastric juice (GJ) or entire paralarvae (All) of several octopus species.
It is important to evaluate the pH, temperature, substrate concentration and divalent ions that could specifically regulate digestive enzymes activity in each region of the tract (Martínez et al., 2011; Ibarra-García et al., 2018). Sampling requires the animal to be anaesthetized and killed (e.g., Roumbedakis et al., 2020). As soon as possible the digestive tract should be separated immediately into the crop, stomach, caecum and digestive gland using surgical clamps to avoid mixing the luminal contents. Once separated, the volume and weight of the chyme (the mixture of secretions [e.g., mucus and enzymes], partially digested food and ingested fluid) contained in each region should be measured, immediately frozen in liquid nitrogen, and stored until analysis (depending on the time until analysis, samples can be stored at −20, −40 or −80°C). A wealth of information on the digestive physiology of octopus can be obtained from this type of study particularly when combined with other techniques (e.g., in vitro studies of motility or preservation of tissue for IHC; see above); measurements are summarised in Figure 5.
FIGURE 5. Summary of measurements which can be made in octopus to quantify the movement of food through the digestive tract, the digestive processes and the metabolic consequences of digestion. See text for details and references. Abbreviations: ca, caecum; cr, crop; DG, digestive gland; DT, digestive tract; st, stomach. Diagram of octopus and digestive tract modified from Gallardo et al., 2017.
In O. maya, O. mimus and O. vulgaris juveniles and adults, soluble nutrients (e.g., soluble protein, small peptides and amino acids) in the food move quickly to the DG and pass to the circulation becoming available for tissue metabolism, only 40–60 min after ingestion (Rosa et al., 2004; Linares et al., 2015).
The digestive physiology of octopus species and probably of other cephalopods can be divided into two phases: 1)soluble nutrients pre-digested in the prey (or elaborated food) quickly pass-through the crop, stomach and caecum to the DG and then the haemolymph to be transported to tissues; 2) more complex nutrients (e.g., myofibrillar proteins, complex lipids) are processed by the simultaneous action of the enzymes (from salivary glands and DG) when food is in the crop with mechanical degradation by concerted action of the crop and stomach, filtration in the caecum and finally entering the DG via the ducts as a suspension.
A recent histochemical study of the digestive tract of O. vulgaris detected no digestive enzyme presence in the digestive tract epithelium (Fernández-Gago et al., 2019) consistent with the view that chyme formation during the digestive process is predominantly by digestive enzymes secreted from DG with a minor contribution from salivary enzymes. This means that the changes occurring as food passes along the digestive tract are mainly because of the pulses of enzyme production in DG during the digestion phases previously described in cephalopods (Semmens, 2002; Martínez et al., 2011; Linares et al., 2015). The time to digest a meal depends on the species and ambient temperature. For example, in tropical and sub-tropical pre-adults of O. maya (26°C; 0.500 kg), O. mimus (14°C; 1.2 kg) (Linares et al., 2015) and O. vulgaris type II from Brazil (20.8°C) (Bastos et al., 2020) the complete digestive process takes 6–7 h, while in Octopus cyanea the entire digestive process was takes around 12 h at 30°C from the beginning of the meal (Boucher-Rodoni, 1973). Although digestion appears is a fast process in octopus species it is necessary to evaluate how temperature is modulating all the physiological, endocrinological and enzymatic mechanisms involved in the process to predict possible consequences in warming scenarios; a meta-analysis would be a useful first step.
Once the chyme is in the DG, biochemical process involving absorption and further digestion are activated (Boucaud-Camou and Boucher-Rodoni, 1983; Budelmann et al., 1997). In the DG of O. maya fasted for 24 h in laboratory conditions, “resting cells” characterised by few or no heterolysosomes or residual bodies are observed (Martínez et al., 2011). Additionally, an apocrine secretion and cell debris can be observed in the tubular lumen, probably resulting from catabolism in response to food deprivation for 24 h. During the first 1 and 2 h of the postprandial period (PP), digestive cells show nuclei forming a belt at the basal region of each columnar cell as the typical characteristic. Some heterolysosomes appeared inside the digestive cells. Acidophilic chyme in the tubule lumen indicates soluble nutrients in the DG. Once the digestive system in the DG is activated, heterophagosomes in the acinar cells transport amino acids to the haemolymph and then muscle and other tissues. In O. maya, O. mimus and O. vulgaris type II studies suggest that soluble protein is accumulated in muscle tissues initiating the glycosynthetic process (Gallardo et al., 2017; Bastos et al., 2020). After 4 h PP, the digestive cells store heterolysosomes (nutrients). Additionally, heterophagosomes were observed only near the apical zone of the cell, revealing the beginning of complex nutrient transport because of the mechanical digestion of more complex nutrients. Digestive cells showed a brush border (i.e., microvilli) on the apical membrane contacting the lumen. Acidophilic chyme was observed in the centre of the lumen. Peaks of glucose, cholesterol, acylglycerols and soluble protein can be observed with high digestive activity in DG cells. All these processes indicate that nutrients were transformed and transported to the tissues for energy and anabolic processes. At 6 h PP, the digestive cells have a conspicuous brush border and an empty lumen. Digestive cells appear filled with heterolysosomes, without heterophagosomes. At 8 h PP, a reduction in heterolysosomes was be observed, together with residual bodies, cell debris and apocrine secretion in the lumen. The proliferation of nuclei indicated the presence of replacement cells located in the basal lamina of the tubules signalling the end of the process (Boucaud-Camou and Boucher-Rodoni, 1983; Martínez et al., 2011; Gallardo et al., 2017; Fernández-Gago et al., 2019; Bastos et al., 2020).
Before describing the measurement of metabolic rate we first highlight one of the important parameters derived from its measurement. Many years ago it was recognized that the apparent heat increment (AHI) also termed specific dynamic action, (SDA) could be used as an indicator of the cost of mechanical and biochemical processes associated with the ingestion and assimilation of food (Nixon, 1969). Although muscular tissue is responsible for the mechanical activity, and the epithelium of the tract synthesises and secretes chitin and mucus requiring energy, the digestive gland is the main site of metabolic functions (Boucaud-Camou and Boucher-Rodoni, 1983). Hence, the AHI may result from addition of the energy used in the above processes; depending on the diet and temperature this constitutes a considerable percentage of the daily energy budget in aquatic organisms (Katsanevakis et al., 2005; Aguila et al., 2007; Noyola et al., 2013). In O. vulgaris values between 25 and 55% of food conversion efficiency were calculated. When different temperatures were compared it was observed that the peak value of AHI (SDA) of O. vulgaris was 64% at 20°C and 42% at 28°C (Katsanevakis et al., 2005) and in O. cyanea AHI was 60% of the ingested food (Nixon, 1969; Boucaud-Camou and Boucher-Rodoni, 1983). In O. maya it was also demonstrated that the AHI values are dependent on the type of diet with values that oscillate between 9 and 14% of the ingested energy when animals were fed fresh crabs or mixed diets made from fresh crab meat and other ingredients (Aguila et al., 2007; Rosas et al., 2007; Rosas et al., 2008). When temperature was investigated, the AHI values of O. maya were relatively higher in animals maintained in optimal temperature ranges (30–48% of routine metabolic rate; 22–26°C) than observed in animals maintained at 30°C (21%) suggesting that in higher temperatures the energy invested in mechanical and biochemical transformation of food is decreased due to additional costs associated with maintaining a higher metabolic rate at higher temperatures (Noyola et al., 2013). That means that depending on the species and its thermal tolerance temperatures beyond the optimal thermal range could affect the general physiological condition of the animals reducing the energy available to be invested in processing food. In larvae of coral reef, tropical fish it was observed that a temperature of 31.5°C does not affect the AHI (SDA) magnitude and duration suggesting that this species (Amphiprion percula) is well adapted to temperatures expected even in warming scenarios (McLeod and Clark, 2016).
There has been considerable debate about measurement of respiratory metabolism in aquatic animals in the light of new hypotheses related to global warming and physiological adaptation of ectotherms (Sokolova et al., 2012a; Sokolova et al., 2012b; Norin and Clark, 2016; Pörtner et al., 2017). Although the debate has focused on fish (Chabot et al., 2016a), there is a broad consensus on measurement of metabolism in aquatic organisms (Steffensen, 1989; Chabot et al., 2016b; Svendsen et al., 2016). Two types of respirometer have been used:
i) Flow-through respirometry. This measures oxygen consumption by quantifying the difference between inlet and outlet oxygen concentration and adjusting the flow of water through the respirometer to maintain a pre-set oxygen content difference. Oxygen consumption is then calculated as the product of water flow through the respirometer per unit time and the difference in oxygen concentration of the water entering and exiting the respirometer. In this respirometer the constant inflow of clean water reduces or eliminates the hypoxia, hypercapnia and nitrogenous waste issues associated with closed-system respirometry, but it introduces mixing and equilibration (washout) problems (Steffensen, 1989), although this can be corrected (Niimi, 1978).
ii) Intermittent-flow respirometry. This combines short measurement periods in a recirculating, but closed, respirometer. After some time (determined by the oxygen level in the respirometric chamber: not lower than dissolved oxygen saturation of 80%) the chamber is flushed with clean water to ensure that the water in the respirometer has been thoroughly exchanged to eliminate potential hypoxia, hypercapnia and nitrogenous waste build-up in the chamber (Steffensen, 1989). While this oxygen consumption method is the most popular amongst fish physiologists, it does require more equipment and a slightly more complex experimental setup than the flow-through respirometer (Svendsen et al., 2016). Although both methods have pros and cons, the most important aspect is the accuracy and validity of the measurements.
The development of oxygen sensors to measure dissolved oxygen facilitated experimental work in this area. Although polarographic oxygen probes are still used, optical sensors are considered the best to use in physiological evaluations of metabolic rate of aquatic animals including cephalopods. Much of the data obtained in the last 50 years on octopus species used the home tank as a respirometric chamber and in the many cases as closed respirometers (Wells et al., 1983; O'Dor and Wells, 1987; Wells et al., 1996; Cerezo Valverde and Garcia Garcia, 2005; Katsanevakis et al., 2005). Although those data give useful information about the respiratory physiology of several octopus species, using optical sensors and improving the systems (i.e., Intermittent-flow or flow-through respirometry) will give more precise data as shown in O. vulgaris and O. maya (Cerezo Valverde and Garcia Garcia, 2004; Aguila et al., 2007; Noyola et al., 2013; Martínez et al., 2014; Meza-Buendía et al., 2021).
Data on oxygen consumption can be used to evaluate the energetic costs of different types of food (natural and elaborated) if the AHI is measured but also, if ingested food (I), faeces production (F), nitrogen excretion (N) and growth (P) are measured (all expressed in energy units; J day−1 g−1 or kg−1), these values can be integrated into an energetic flow equation (Wells et al., 1996):
where assimilated energy (As) can be calculated as:
In this equation R = RAHI + Rrut, with Rrut the metabolic rate of animals before feeding in respirometric chamber. Studies made in O. maya show stable Rrut values can be obtained in animals conditioned to respirometric chambers for 12–18 h, depending on temperature and the animal size (Roumbedakis et al., 2020; Meza-Buendía et al., 2021). When this type of data is obtained in animals fed different types of food it is possible to know how the nutritional characteristics of the food modulate the quantity of energy that is channelled to growth in comparison to mechanical and biochemical transformation of the ingested food. When pre-adult O. maya were fed Callinectes sapidus crab, R was 30% of As, but animals fed with elaborated food made from fish meal the R/As, % was between 80 and 90% indicating that a significantly proportion of the ingested energy was used to transform that diet in physiologically useful energy reducing the energy available for growth (Aguila et al., 2007). When an elaborated paste made from crab and squid was used to feed early juveniles of this species, a R/As value of 27%, was obtained. Analysis of R/A% and growth shows that lower values (∼30%) are associated with higher growth rates but diets with high R/A% (80–95%) are associated with lower growth as almost all the ingested energy is channelled to maintenance but not growth (Van Heukelem, 1976; Martínez et al., 2014).
The utility of molecular studies is well illustrated by studies investigating effects of the gastrointestinal parasite Aggregata octopiana. Changes in gene expression in tissue from discrete regions of the DS provides insights into physiology. For example, in the octopus gastric ganglion expression of selected genes correlated with increased relative expression of six genes in conditions of high infection (i.e., Sn, Nfkb2, Cckar, SCPRP, Serpinb10, Tlr3) while reduced expression was reported for others (e.g., Litaf, Mirp, Sod1, Prdx6, Gpx1, Rph3al) (Baldascino et al., 2017). The study demonstrated effects of the parasite on a neural structure at a site distant from the locus of infection in the intestinal wall. Transcriptomic analysis of haemocytes identified >500 differentially expressed transcripts in Aggregata infected octopus infected with Aggregata including genes involved in pathways such as Nuclear Factor-kB, Toll-like receptor, and Complement (e.g., Castellanos-Martínez et al., 2014) or transcriptional variations of diet, temperature, and growth-related genes in paralarvae (e.g., García-Fernández et al., 2019; Garcia De La Serrana et al., 2020).
Proteomics further characterised salivary glands and their secretions. For example, the Southern blue-ringed octopus (Hapalochlaena maculosa) is a remarkable exception with loss of proteinaceous toxins due to the presence of tetrodotoxin (Whitelaw et al., 2020). The gland has >600 genes exclusive to H. maculosa when compared to O. bimaculoides and Callistoctopus minor) providing an overall scenario of fewer and more specialized genes expressed by other octopod species, when compared to Hapalochlaena.
Although omics and molecular studies provide novel insights it is important that findings are translated where possible into understanding the physiology of the digestive system.
Relatively little is known about how the individual functions described above are controlled, coordinated with each other (e.g., the relationship between the crop, stomach and caecum and the secretory and absorptive phases of DG activity) and with the regulation of food intake. Here we briefly review selected studies to illustrate some of the applicable techniques.
a) The enteric nervous system (ENS). The ENS comprises the neurones within the wall of the digestive tract which may act as relays between the gastric ganglion and the muscle/epithelium to modulate secretion and motility but which also form independent reflex circuits comprising intrinsic afferent (the subepithelial plexus is reported to consist mainly of sensory cells in cuttlefish, Alexandrowicz, 1928) and efferent neurones (Furness and Stebbing, 2018). Although there is good histological evidence for the presence of intramural neurones throughout the digestive tract in cephalopods (e.g., Alexandrowicz, 1928; Young, 1967) only a limited number of species have been studied, with limited techniques (e.g., no tract tracing studies to define the exact relationship to the GG or immunohistochemistry) and there are no specific functional studies. Involvement of ENS in coordinated peristalsis and mucus secretion is highly probable by analogy with other species (Costa and Furness, 1976; Furness, 2006) as is modulation by extrinsic nerves (e.g., from the gastric ganglion) but these speculations await experimental confirmation using for example in vitro pharmacological studies (see above), and neurophysiological recordings from enteric neurones as have been performed extensively in mammals (Furness, 2006).
b) The gastric ganglion (GG). The structure and neurochemical complexity of the GG is consistent with its proposed role as a peripheral integrative centre but limited studies have only been undertaken in O. vulgaris. Electrical stimulation of the GG in O. vulgaris increased contractile activity in the crop, stomach, caecum (spiral tightening) and proximal intestine (Andrews and Tansey, 1983a) and there is a single report (Falloise, 1906) of accelerated flow from the digestive gland which could be due to capsule contraction, expulsion of stored secretion or de novo synthesised secretions. However, there have been no neurophysiological studies demonstrating the integrative abilities of the GG, the properties of its neurones or how it interfaces with the ENS, although its compact nature and structure (cortical layer of cells) make it ideally suited for these types of study. Neurophysiological studies of the stomatogastric ganglion in other molluscs (e.g., Jing et al., 2007; Daur et al., 2016) provide examples of the techniques and types of study which need to be undertaken.
Although surgical removal of the GG would be a technically simple procedure, it is unlikely to yield results which would be readily interpretable because of its extensive influence. In vitro studies of the entire digestive tract in O. vulgaris showed that GG removal/local anaesthetic application disrupted coordination of contractions between the crop and stomach and also contracted the crop suggesting removal of a tonic inhibitory effect of the GG on the crop (Andrews and Tansey, 1983a).
Molecular and immunohistochemical studies of the O. vulgaris GG (Baldascino et al., 2017) have identified a number of putative peptide neurotransmitters (e.g., cephalotocin, corticotrophin releasing factor, FMRF-amide, octopressin, small cardioactive peptide-related peptide, tachykinin related peptide) and receptors (e.g., cholecystokinin A,B, octopressin, orexin receptor2). However, functional studies are limited to showing contraction of the radula muscle (small cardioactive peptide-related peptide tachykinin related peptide, Kanda and Minakata, 2006) and contraction of the crop and stomach (tachykinin related peptide, Kanda et al., 2007) or rectum (octopressin, Takuwa-Kuroda et al., 2003). Detailed studies of the effect of putative peptide neurotransmitters on digestive tract motility and secretions are needed both by investigating their direct effects (in vitro studies) and also indirect effects by acting on the GG where they could induce motor program switching (e.g., Kirby and Nusbaum, 2007).
The role of the GG in regulating individual regions of the digestive tract could also be investigated by selective surgical transection (under general anaesthesia, with recovery and including a sham lesion group) of nerves radiating from the ganglion to adjacent structures. Following recovery, urge to eat, food intake, growth, transit time, faecal composition, haemolymph composition and post-prandial metabolism (O2 consumption) could be measured to identify the impact of the lesion. Such a study would need careful justification, with clearly defined outcomes. Interpretation of the results may be complicated as the sectioned nerves may contain both afferent and efferent fibres and digestion of food is a sequential process with interdependent components.
c) The central nervous system (CNS), interchange of information with the digestive system, and control of food intake.
Communication (neural and/or endocrine) between the digestive system and the CNS is essential for the regulation of food intake which requires behaviour change to locate, capture and ingest food at a time when digestion of the previous meal permits. Limited evidence from changes in attack behaviour in O. vulgaris at different times after feeding has been used to conclude that the crop sends information on its degree of distension to the CNS (directly or via the GG; see: Young, 1960; Nixon, 1965). Additionally, as not all nerve fibres degenerate when the sympathetic nerves are cut between the inferior buccal ganglion and oesophagus it is concluded that the nerve contains some afferent fibres (Young, 1965). Neurophysiological studies are required to record from the sympathetic nerves to investigate whether they convey information about crop distension to the CNS in octopus or in the case of cuttlefish and squid from the stomach. If mechanosensitive afferents are present, we hypothesise by analogy with mechanoreceptors in hollow visceral organs of vertebrates, that they will be in-series with the muscle and hence capable of signalling both overall level of distension and contractions (see for example Andrews et al., 1980).
A detailed neurophysiological survey of the information carried in the nerves connecting the GG to the DT, the visceral nerves, and the sympathetic nerves connecting with the inferior buccal ganglion and superior buccal lobe is required to understand the relative role(s) played by the CNS, GG and ENS.
The effects of surgical transection of the nerves connecting the CNS and digestive system was investigated by Best and Wells (1983) in O. vulgaris but the observations have not been pursued. In food deprived animals the sight of a crab in a sealed glass jar stimulated secretion in the DG, an effect prevented by section of the sympathetic nerves either above or below the crop implying a response driven by the CNS. This is the equivalent of the “cephalic phase” of digestive tract secretions first reported by Pavlov in dogs (Pavlov, 1897). However, the majority of animals (16/18) with sympathetic nerves cut were unable to clean crabs after successfully attacking complicating interpretation. Section of the abdominal and atrio-rectal branches of the visceral nerve was without apparent effect on the ability to clean a crab, the DG response, post-prandial rise in O2 consumption or growth. Although representing unique observations the results should be treated with caution until replicated using more refined surgical techniques.
The control of the DS by substances acting via the haemolymph has not been investigated in any detail especially in comparison to the reproductive system in cephalopods (e.g., Zatylny-Gaudin et al., 2016). Hormones synthesised outside the digestive system could regulate any of the DT functions directly or via the GG but also the potential for hormones released from the digestive tract to act on the GG or on the CNS for example to regulate food intake should not be overlooked. With the possible exception of the posterior salivary glands (Ponte and Modica, 2017) histological studies have not identified cells with characteristics typical of endocrine or paracrine cells in the digestive system but there have not been any systematic studies. Measurements of haemolymph during food deprivation and post-prandially would be the most obvious approach to identifying hormones linked to digestion with candidates identified from analysis of the genome. Based particularly on molecular studies, the following families are of particular interest: 1) secretin (Cardoso et al., 2010; Mirabeau and Joly, 2013; Tam et al., 2014). A secretin-like substance was isolated from the caecum in O. vulgaris and shown to stimulate secretion in the digestive gland (Ledrut and Ungar, 1937). This study should be followed up as a major function of secretin is stimulation of fluid secretion in the vertebrate exocrine pancreas (Bayliss and Starling, 1902); 2) vasopressin/oxytocin (Odekunle et al., 2019). Evidence for the octopressin receptor in the GG has been obtained (Baldascino et al., 2017) and Odekunle et al. (2019) noted evidence implicating the vasopressin/oxytocin -type signalling from a number of studies of protostomes; 3) gastrin/cholecystokinin (Zatylny-Gaudin and Favrel, 2014) as there is evidence for cholecystokinin A,B receptors in the GG (Baldascino et al., 2017); 5) orexin (Ritschard et al., 2019). There is limited evidence for the orexin receptor2 in the GG but the ligand in cephalopods is not known although members of the orexin family have been implicated in regulation of food intake in vertebrates (Wong et al., 2011).
Molecular and haemolymph profile studies need to be complemented by studies showing a functional effect of any hormones on the digestive tract. In addition, the effect of reproductive hormones on the digestive tract needs to be investigated as they may be involved in the suppression of food intake which accompanies egg laying and care in several octopus species (e.g., O. maya).
We have reviewed diverse techniques applied to understanding the physiology of the digestive tract which enable it to perform its primary function of processing food into a form which can be utilized. Three themes emerge: 1) Many of the pivotal studies were published more than 50 years ago and should be replicated using more modern techniques to both confirm and extend the initial findings; 2) There are a number of major knowledge gaps (Figure 6) (e.g., transepithelial transport in the caecum/intestine) which can be resolved using established physiological techniques (e.g., Ussing chamber, molecular identification of transporters) and assumptions about function are made based primarily upon anatomy (e.g., typhlosole function); 3) Our understanding of the physiology of the digestive tract in cephalopods is a “mosaic of knowledge” with data on individual DT functions coming from a range of species in different Orders rather than a detailed understanding of the entire digestive tract in representative species (e.g., O. vulgaris and S. officinalis). As Bidder (1950, p. 41) noted 70 years ago “Until more information is available it is clearly dangerous to combine, as has too often been done, one observation from Octopus with another from Sepia under the generalisation “In the Cephalopods.””
FIGURE 6. Diagram summarising a number of the key issues discussed in the review. The left -hand panel shows some of the diverse range of materials (food and non-food items) which can enter the digestive tract of cephalopods and which it will need to digest/detoxify/metabolise or eject (vomiting or defaecation). From left to right in each row the symbols indicate: water (may also contain dispersed oils), ions, biohazardous material, fish (or pieces), fish bones, fish scales, crustacea, jellyfish, plastic (entire items or fragments depending on animal size), toxins (may be adsorbed to plastics), mussels and other shellfish, domoic acid (may be present in mussels), shrimps, cephalopods (including conspecifics), seaweed and seagrasses (probably incidentally ingested with other food). The middle panel shows gross anatomical features of the digestive tract of an exemplar octopus, cuttlefish and squid to highlight the major gross anatomical differences. The horizontal red line represents the transit time-the time taken from when food enters the digestive tract via the beak to when the remains of that meal leave in the faeces (black oval). Abbreviations: af, anal flaps; bc, buccal complex; ca, caecum; cr, crop; DG, digestive gland; dga, digestive gland appendage; int, intestine; oe, oesophagus; psgs, posterior salivary glands; st, stomach. The right -hand panel list 10 key questions which need to be answered about the physiology of the cephalopod digestive tract and which will require the application of some new techniques and development of other to investigate in cephalopods. See text for detailed discussion references. The diagram in the middle panel is modified from Lobo-da-Cunha, 2019.
It is hoped that this review by combining discussion of the physiology of the cephalopod DS with an overview of techniques and identification of key knowledge gaps will stimulate a more systematic approach to research in this area.
All authors made an equivalent contribution to the mansucript.
CR thanks the PAPIIT program at the National Autonomous University of Mexico for the economic support given to study the digestive physiology of Octopus maya (IN 203022). GP and PLRA have been supported by the Stazione Zoologica Anton Dohrn intramural fund.
PA wishes to acknowledge that this review was written during the tenure of an honorary Research Fellowship at Stazione Zoologica Anton Dohrn Naples, Italy and would like to thank G. Fiorito (Head of the Department of Biology and Evolution of Marine Organisms, SZN) and the SZN President R. Danovaro. PA and GP wish to thank Dieter Fuchs and Visualsonics, and the Association for Cephalopod Research for their support and advice regarding the use of high-resolution ultrasound to image the octopus digestive system.
The authors declare that the research was conducted in the absence of any commercial or financial relationships that could be construed as a potential conflict of interest.
All claims expressed in this article are solely those of the authors and do not necessarily represent those of their affiliated organizations, or those of the publisher, the editors, and the reviewers. Any product that may be evaluated in this article, or claim that may be made by its manufacturer, is not guaranteed or endorsed by the publisher.
Aguila J., Cuzon G., Pascual C., Domingues P. M., Gaxiola G., Sánchez A., et al. (2007). The effects of fish hydrolysate (CPSP) level on Octopus maya (Voss and Solis) diet: Digestive enzyme activity, blood metabolites, and energy balance. Aquaculture 273, 641–655. doi:10.1016/j.aquaculture.2007.07.010
Aldred R. G., Nixon M., Young J. Z. (1983). Cirrothauma murrayi Chun, a finned octopod. Philosophical Trans. R. Soc. Lond. B, Biol. Sci. 301, 1–54.
Alexandrowicz J. S. (1928). Notes sur l'innervation du tube digestif cephalopodes. Archives de zoologie Exp. Gen. 67, 69–90.
Amado E. M., Souza-Bastos L. R., Vidal E. a. G., Leite T. S., Freire C. A. (2015). Different abilities to regulate tissue hydration upon osmotic challenge in vitro, in the cephalopods Octopus vulgaris and O. insularis. Mar. Freshw. Behav. Physiology 48, 205–211. doi:10.1080/10236244.2015.1024078
Anadón R. (2019). “Functional histology: The tissues of common coleoid cephalopods,” in Handbook of pathogens and diseases in cephalopods (Springer), 39–85.
Andrews P. L., Grundy D., Scratcherd T. (1980). Vagal afferent discharge from mechanoreceptors in different regions of the ferret stomach. J. Physiol. 298, 513–524. doi:10.1113/jphysiol.1980.sp013098
Andrews P. L. R., Darmaillacq A. S., Dennison N., Gleadall I. G., Hawkins P., Messenger J. B., et al. (2013). The identification and management of pain, suffering and distress in cephalopods, including anaesthesia, analgesia and humane killing. J. Exp. Mar. Biol. Ecol. 447, 46–64. doi:10.1016/j.jembe.2013.02.010
Andrews P. L. R., Tansey E. M. (1983a). The digestive tract of Octopus vulgaris: The anatomy, physiology and pharmacology of the upper tract. J. Mar. Biol. Assoc. U. K. 63, 109–134. doi:10.1017/s0025315400049845
Andrews P. L. R., Tansey E. M. (1983b). Aminergic innervation of the blood vessels of Octopus vulgaris. Cell Tissue Res. 230, 229–232. doi:10.1007/BF00216043
Aristotle (1910). Historia animalium, English translation by D'arcy wenthworth thompson. Oxford: Clarendon Press.
Arvy L. (1960). Histoenzymological data on the digestive tract of Octopus vulgaris lamarck (cephalopoda). Ann. N. Y. Acad. Sci. 90, 929–949. doi:10.1111/j.1749-6632.1960.tb26440.x
Avendaño O., Roura Á., Cedillo-Robles C. E., González Á. F., Rodríguez-Canul R., Velázquez-Abunader I., et al. (2020). Octopus americanus: A cryptic species of the O. vulgaris species complex redescribed from the caribbean. Aquat. Ecol. 54, 909–925. doi:10.1007/s10452-020-09778-6
Bacq Z. M. (1934). Recherches Sur La Physiologie Du Systéme Nerveux Autonome. V. – Réactions Du Ventricule Médian, Des Chromatophores Et De Divers Organes Isolés d'Un Mollusque Céphalopode (Loligo Pealii) A L'Adrénaline, L'Acétylcholine, L'Ergotamine, L'Atropine Et Aux Ions K, Ca et Mg. Arch. Int. Physiol. 38, 138–159. doi:10.3109/13813453309145138
Baldascino E., Di Cristina G., Tedesco P., Hobbs C., Shaw T. J., Ponte G., et al. (2017). The gastric ganglion of Octopus vulgaris: Preliminary characterization of gene- and putative neurochemical-complexity, and the effect of Aggregata octopiana digestive tract infection on gene expression. Front. Physiol. 8, 1001. doi:10.3389/fphys.2017.01001
Bastos P., Fracalossi D. M., Chimal M. E., Sánchez A., Rosas C. (2020). Digestive enzymes and timing of digestion in Octopus vulgaris type II. Aquac. Rep. 16, 100262. doi:10.1016/j.aqrep.2019.100262
Bastos P., Gallardo P., Rosas C., Vieira F. D. N., Silva C. P., Oliveira G. B., et al. (2021). Pelleted diet with thermal treatment of ingredients for Octopus americanus: Growth performance and enzymatic activity. Aquac. Res. 52, 1106–1117. doi:10.1111/are.14968
Bayliss W. M., Starling E. H. (1902). The mechanism of pancreatic secretion. J. Physiol. 28, 325–353. doi:10.1113/jphysiol.1902.sp000920
Best E., Wells M. (1983). The control of digestion in Octopus I: The anticipatory response and the effects of severing the nerves to the gut. Vie Milieu/Life Environ. 33, 135–142.
Bidder A. M. (1957). Evidence for an absorptive function in the liver of Octopus vulgaris. Pubblicazioni della Stazione Zool. Napoli 29, 139–150.
Bidder A. M. (1950). The digestive mechanism of the European squids Loligo vulgaris, Loligo forbesii, Alloteuthis media and Alloteutihis subulata. J. Cell Sci. 9, 1–43. doi:10.1242/jcs.s3-91.13.1
Birch J., Burn C., Schnell A., Browning H., Crump A. (2021). Review of the evidence of sentience in cephalopod molluscs and decapod Crustaceans. London: London School of Economics and Political Science.
Bogoraze D., Cazal P. (1946). Remarques sur le système stomatogastrique du Poulpe (Octopus vulgaris lamarck): Le complexe retro-buccal. Archives de zoologie expérimentale générale 84, 115–131.
Boucaud-Camou E., Boucher-Rodoni R., Mangold K. (1976). Digestive absorption in Octopus vulgaris (cephalopoda: Octopoda). J. Zoology 179, 261–271. doi:10.1111/j.1469-7998.1976.tb02295.x
Boucaud-Camou E. V. E., Boucher-Rodoni R. (1983). “Feeding and digestion in cephalopods,” in The Mollusca. Editors A. S. M. Saleuddin, and K. M. Wilbur (San Diego: Academic Press), 149–187.
Boucher-Rodoni R. (1976). Étude histologique du tube digestif de deux Cephalopodes Eledone cirrosa (Octopoda) et Illex illecebrosus (Teuthoidea), au cours de la digestion. Cah. Biol. Mar. 17, 245–260.
Boucher-Rodoni R. (1982). La glande digestive des céphalopodes, organe de synthèse et de sécrétion d’enzymes digestives. Cah. Biol. Mar. 23, 299–318.
Boucher-Rodoni R., Mangold K. (1977). Experimental study of digestion in Octopus vulgaris (cephalopoda: Octopoda). J. Zoology 183, 505–515. doi:10.1111/j.1469-7998.1977.tb04202.x
Boucher-Rodoni R. (1973). Vitesse de Digestion d'Octopus cyanea (cephalopoda: Octopoda). Mar. Biol. 18, 237–242. doi:10.1007/bf00367990
Boyle P. R., Mangold1 K., Froesch* D. (1979). The mandibular movements of Octopus vulgaris. J. Zoology 188, 53–67. doi:10.1111/j.1469-7998.1979.tb03392.x
Braid H. E., Deeds J., Degrasse S. L., Wilson J. J., Osborne J., Hanner R. H. (2012). Preying on commercial fisheries and accumulating paralytic shellfish toxins: A dietary analysis of invasive Dosidicus gigas (cephalopoda ommastrephidae) stranded in pacific Canada. Mar. Biol. 159, 25–31. doi:10.1007/s00227-011-1786-4
Brijs J., Hennig G. W., Axelsson M., Olsson C. (2014). Effects of feeding on in vivo motility patterns in the proximal intestine of shorthorn sculpin (Myoxocephalus scorpius). J. Exp. Biol. 217, 3015–3027. doi:10.1242/jeb.101741
Brijs J., Hennig G. W., Gräns A., Dekens E., Axelsson M., Olsson C. (2017a). Exposure to seawater increases intestinal motility in euryhaline rainbow trout (Oncorhynchus mykiss). J. Exp. Biol. 220, 2397–2408. doi:10.1242/jeb.156000
Brijs J., Hennig G. W., Kellermann A.-M., Axelsson M., Olsson C. (2017b). The presence and role of interstitial cells of Cajal in the proximal intestine of shorthorn sculpin (Myoxocephalus scorpius). J. Exp. Biol. 220, 347–357. doi:10.1242/jeb.141523
Budelmann B. U., Schipp R., Boletzky S. V. (1997). “Cephalopoda,” in Microscopic anatomy of invertebrates. Editors F. W. Harrison, and A. J. Kohn (New York: Wiley-Liss, Inc.), 119–414.
Bustamante P., Caurant F., Fowler S. W., Miramand P. (1998). Cephalopods as a vector for the transfer of cadmium to top marine predators in the north-east Atlantic Ocean. Sci. Total Environ. 220, 71–80. doi:10.1016/s0048-9697(98)00250-2
Bustamante P., Lahaye V., Durnez C., Churlaud C., Caurant F. (2006). Total and organic Hg concentrations in cephalopods from the North Eastern Atlantic waters: Influence of geographical origin and feeding ecology. Sci. Total Environ. 368, 585–596. doi:10.1016/j.scitotenv.2006.01.038
Cardoso J. C. R., Vieira F. A., Gomes A. S., Power D. M. (2010). The serendipitous origin of chordate secretin peptide family members. BMC Evol. Biol. 10, 135. doi:10.1186/1471-2148-10-135
Cariello L., Zanetti L. (1977). Alpha- and beta-cephalotoxin: Two paralysing proteins from posterior salivary glands of Octopus vulgaris. Comp. Biochem. Physiol. C. Comp. Pharmacol. 57, 169–173. doi:10.1016/0306-4492(77)90066-1
Castellanos-Martínez S., Arteta D., Catarino S., Gestal C. (2014). De novo transcriptome sequencing of the Octopus vulgaris hemocytes using illumina RNA-seq Technology: Response to the infection by the gastrointestinal parasite aggregata octopiana. PLOS ONE 9, e107873. doi:10.1371/journal.pone.0107873
Cerezo Valverde J., Garcia Garcia B. N. (2004). Influence of body weight and temperature on post-prandial oxygen consumption of common octopus (Octopus vulgaris). Aquaculture 233, 599–613. doi:10.1016/j.aquaculture.2003.11.025
Cerezo Valverde J., Garcia Garcia B. (2005). Suitable dissolved oxygen levels for common octopus (Octopus vulgaris cuvier, 1797) at different weights and temperatures: Analysis of respiratory behaviour. Aquaculture 244, 303–314. doi:10.1016/j.aquaculture.2004.09.036
Chabot D., Mckenzie D. J., Craig J. F. (2016a). Metabolic rate in fishes: Definitions, methods and significance for conservation physiology. J. Fish. Biol. 88, 1–9. doi:10.1111/jfb.12873
Chabot D., Steffensen J. F., Farrell A. P. (2016b). The determination of standard metabolic rate in fishes. J. Fish. Biol. 88, 81–121. doi:10.1111/jfb.12845
Costa M., Furness J. B. (1976). The peristaltic reflex: An analysis of the nerve pathways and their pharmacology. Naunyn. Schmiedeb. Arch. Pharmacol. 294, 47–60. doi:10.1007/BF00692784
Costa P. M., Rodrigo A. P., Costa M. H. (2014). Microstructural and histochemical advances on the digestive gland of the common cuttlefish, Sepia officinalis L. Zoomorphology 133, 59–69. doi:10.1007/s00435-013-0201-8
Daur N., Nadim F., Bucher D. (2016). The complexity of small circuits: The stomatogastric nervous system. Curr. Opin. Neurobiol. 41, 1–7. doi:10.1016/j.conb.2016.07.005
Emam W., Ibrahim A. a. M., Ghareb T. (2016). Macro and microscopic structure of the digestive system of Octopus vulgaris from Alexandria water on the Mediterranean Sea. Int. J. Dev. 5, 27–45. doi:10.21608/idj.2016.146734
Erspamer V., Anastasi A. (1962). Structure and pharmacological actions of eledoisin, the active endecapeptide of the posterior salivary glands of eledone. Experientia 18, 58–59. doi:10.1007/BF02138250
Falloise A. (1906). Contribution à la physiologie comparée de la digestion. La digestion chez les Céphalopodes. Arch. Intern. Physiol. 3, 282–296.
Farías A., Pereda S. V., Uriarte I., Dörner J., Cuzon G., Rosas C. (2010). Evaluating the effects of formulated moist diets on juveniles of patagonian Octopus Enteroctopus megalocyathus (gould 1852). J. Shellfish Res. 29, 793796–793798. doi:10.2983/035.029.0412
Fernández-Gago R., Heß M., Gensler H., Rocha F. (2017). 3D reconstruction of the digestive system in Octopus vulgaris cuvier, 1797 embryos and paralarvae during the first month of life. Front. Physiol. 8, 462. doi:10.3389/fphys.2017.00462
Fernández-Gago R., Molist P., Rocha F. (2019). Anatomical and histochemical features of the digestive system of Octopus vulgaris Cuvier, 1797 with a special focus on secretory cells. Acta Zool. 100, 320–335. doi:10.1111/azo.12257
Ferreira G. V. B., Justino A. K. S., Eduardo L. N., Lenoble V., Fauvelle V., Schmidt N., et al. (2022). Plastic in the inferno: Microplastic contamination in deep-sea cephalopods (Vampyroteuthis infernalis and Abralia veranyi) from the southwestern Atlantic. Mar. Pollut. Bull. 174, 113309. doi:10.1016/j.marpolbul.2021.113309
Fiorito G., Affuso A., Anderson D. B., Basil J., Bonnaud L., Botta G., et al. (2014). Cephalopods in neuroscience: Regulations, research and the 3Rs. Invert. Neurosci. 14, 13–36. doi:10.1007/s10158-013-0165-x
Fiorito G., Affuso A., Basil J., Cole A., De Girolamo P., D'angelo L., et al. (2015). Guidelines for the care and welfare of cephalopods in research - a consensus based on an initiative by CephRes, FELASA and the Boyd Group. Lab. Anim. 49, 1–90. doi:10.1177/0023677215580006
Furness J. B., Stebbing M. J. (2018). The first brain: Species comparisons and evolutionary implications for the enteric and central nervous systems. Neurogastroenterol. Motil. 30, e13234. doi:10.1111/nmo.13234
Gallardo P., Olivares A., Martínez-Yáñez R., Caamal-Monsreal C., Domingues P. M., Mascaró M., et al. (2017). Digestive physiology of Octopus maya and O. mimus: Temporality of digestion and assimilation processes. Front. Physiol. 8, 355. doi:10.3389/fphys.2017.00355
Garcia De La Serrana D., Pérez M., Nande M., Hernández-Urcera J., Pérez E., Coll-Lladó C., et al. (2020). Regulation of growth-related genes by nutrition in paralarvae of the common octopus (Octopus vulgaris). Gene 747, 144670. doi:10.1016/j.gene.2020.144670
García-Fernández P., Prado-Alvarez M., Nande M., Garcia De La Serrana D., Perales-Raya C., Almansa E., et al. (2019). Global impact of diet and temperature over aquaculture of Octopus vulgaris paralarvae from a transcriptomic approach. Sci. Rep. 9, 10312. doi:10.1038/s41598-019-46492-2
García-Garrido S., Hachero-Cruzado I., Garrido D., Rosas C., Domingues P. (2010). Lipid composition of the mantle and digestive gland of Octopus vulgaris juveniles (Cuvier, 1797) exposed to prolonged starvation. Aquac. Int. 18, 1223–1241. doi:10.1007/s10499-010-9335-6
Garri G. R., Lauria de Cidre L. (2013). Microanatomy of the digestive system of Enteroctopus megalocyathus (cephalopoda, Octopoda) of the southwest atlantic. Bol. Invest. Mar. Cost. 42, 255–274. doi:10.25268/bimc.invemar.2013.42.2.49
Gestal C., Páez De La Cadena M., Pascual S. (2002). Malabsorption syndrome observed in the common octopus Octopus vulgaris infected with Aggregata octopiana (Protista: Apicomplexa). Dis. Aquat. Organ. 51, 61–65. doi:10.3354/dao051061
Gestal C., Pascual S., Guerra Á., Fiorito G., Vieites J. M. (2019). Handbook of pathogens and diseases in cephalopods. Springer International Publishing.
Ghiretti F. (1959). Cephalotoxin: The crab-paralysing agent of the posterior salivary glands of cephalopods. Nature 183, 1192–1193. doi:10.1038/1831192b0
Gonçalves C., Costa P. M. (2021). Cephalotoxins: A hotspot for marine bioprospecting? Front. Mar. Sci. 8. doi:10.3389/fmars.2021.647344
Grisley M., Boyle P. (1987). Bioassay and proteolytic activity of digestive enzymes from octopus saliva. Comp. Biochem. Physiology Part B Comp. Biochem. 88, 1117–1123. doi:10.1016/0305-0491(87)90014-9
Grisley M. S., Boyle P. R. (1990). Chitinase, a new enzyme in octopus saliva. Comp. Biochem. Physiology Part B Comp. Biochem. 95, 311–316. doi:10.1016/0305-0491(90)90081-4
Grosell M. (2010). “4 - the role of the gastrointestinal tract in salt and water balance,” in Fish physiology. Editors M. Grosell, A. P. Farrell, and C. J. Brauner (Academic Press), 135–164.
Guerra Á. (2019). “Functional anatomy: Macroscopic anatomy and post-mortem examination,” in Handbook of pathogens and diseases in cephalopods (Springer), 11–38.
Hoving H. J. T., Haddock S. H. D. (2017). The giant deep-sea octopus Haliphron atlanticus forages on gelatinous fauna. Sci. Rep. 7, 44952. doi:10.1038/srep44952
Hoving H. J. T., Robison B. H. (2012). Vampire squid: Detritivores in the oxygen minimum zone. Proc. Biol. Sci. 279, 4559–4567. doi:10.1098/rspb.2012.1357
Hu M. Y., Sucré E., Charmantier-Daures M., Charmantier G., Lucassen M., Himmerkus N., et al. (2010). Localization of ion-regulatory epithelia in embryos and hatchlings of two cephalopods. Cell Tissue Res. 339, 571–583. doi:10.1007/s00441-009-0921-8
Hwang S. J., Blair P. J. A., Britton F. C., O’driscoll K. E., Hennig G., Bayguinov Y. R., et al. (2009). Expression of anoctamin 1/TMEM16A by interstitial cells of Cajal is fundamental for slow wave activity in gastrointestinal muscles. J. Physiol. 587, 4887–4904. doi:10.1113/jphysiol.2009.176198
Ibáñez C. M., Riera R., Leite T., Díaz-Santana-Iturrios M., Rosa R., Pardo-Gandarillas M. C. (2021). Stomach content analysis in cephalopods: Past research, current challenges, and future directions. Rev. Fish. Biol. Fish. 31, 505–522. doi:10.1007/s11160-021-09653-z
Ibarra-García L. E., Tovar-Ramírez D., Rosas C., Campa-Córdova Á. I., Mazón-Suástegui J. M. (2018). Digestive enzymes of the californian two-spot octopus, Octopus bimaculoides (pickford and McConnaughey, 1949). Comp. Biochem. Physiol. B Biochem. Mol. Biol. 215, 10–18. doi:10.1016/j.cbpb.2017.10.001
Jacquet J., Franks B., Godfrey-Smith P., Sánchez-Suárez W. (2019). The case against Octopus farming. Issues Sci. Technol. 35, 37–44.
Jing J., Vilim F. S., Horn C. C., Alexeeva V., Hatcher N. G., Sasaki K., et al. (2007). From hunger to satiety: Reconfiguration of a feeding network by Aplysia neuropeptide Y. J. Neurosci. 27, 3490–3502. doi:10.1523/JNEUROSCI.0334-07.2007
Kanda A., Minakata H. (2006). Isolation and characterization of a novel small cardioactive peptide-related peptide from the brain of Octopus vulgaris. Peptides 27, 1755–1761. doi:10.1016/j.peptides.2005.12.006
Kanda A., Takuwa-Kuroda K., Aoyama M., Satake H. (2007). A novel tachykinin-related peptide receptor of Octopus vulgaris– evolutionary aspects of invertebrate tachykinin and tachykinin-related peptide. FEBS J. 274, 2229–2239. doi:10.1111/j.1742-4658.2007.05760.x
Katsanevakis S., Protopapas N., Miliou H., Verriopoulos G. (2005). Effect of temperature on specific dynamic action in the common octopus, Octopus vulgaris (Cephalopoda). Mar. Biol. 146, 733–738. doi:10.1007/s00227-004-1476-6
Kilkenny C., Browne W. J., Cuthill I. C., Emerson M., Altman D. G. (2010). Improving bioscience research reporting: The ARRIVE guidelines for reporting animal research. PLoS Biol. 8, e1000412. doi:10.1371/journal.pbio.1000412
Kirby M. S., Nusbaum M. P. (2007). Peptide hormone modulation of a neuronally modulated motor circuit. J. Neurophysiol. 98, 3206–3220. doi:10.1152/jn.00795.2006
Le H. T. M. D., Lie K. K., Etayo A., Rønnestad I., Sæle Ø. (2021). Physical and nutrient stimuli differentially modulate gut motility patterns, gut transit rate, and transcriptome in an agastric fish, the ballan wrasse. PLOS ONE 16, e0247076. doi:10.1371/journal.pone.0247076
Ledrut J., Ungar G. (1937). Action de la Sécrétine chez L'Octopus vulgaris. Arch. Int. Physiol. 44, 205–211. doi:10.3109/13813453709145203
Lentle R. G., Hulls C. M. (2018). Quantifying patterns of smooth muscle motility in the gut and other organs with new techniques of video spatiotemporal mapping. Front. Physiol. 9, 338. doi:10.3389/fphys.2018.00338
Linares M., Caamal-Monsreal C., Olivares A., Sánchez A., Rodríguez S., Zúñiga O., et al. (2015). Timing of digestion, absorption and assimilation in octopus species from tropical (Octopus maya) and subtropical-temperate (O. mimus) ecosystems. Aquat. Biol. 24, 127–140. doi:10.3354/ab00642
Lobo-da-Cunha A. (2019). Structure and function of the digestive system in molluscs. Cell Tissue Res. 377, 475–503. doi:10.1007/s00441-019-03085-9
Mancuso M., Giordano D., Genovese L., Denaro M. G., Caruso G. (2014). Study of digestive enzymes in wild specimens of Sepia officinalis (Linnaeus, 1758) and Octopus vulgaris (Cuvier, 1797). Cah. Biol. Mar. 55, 445–452.
Mangold K. M., Young R. E. (1998). “The systematic value of the digestive organs,” in Systematics and biogeography of cephalopods. Editors N. A. Voss, M. Vecchione, R. B. Toll, and M. J. Sweeney (Washington, DC: Smithsonian Institution), 21–30.
Martin J. H., Flegal A. R. (1975). High copper concentrations in squid livers in association with elevated levels of silver, cadmium, and zinc. Mar. Biol. 30, 51–55. doi:10.1007/bf00393752
Martínez R., Gallardo P., Pascual C., Navarro J., Sánchez A., Caamal-Monsreal C., et al. (2014). Growth, survival and physiological condition of Octopus maya when fed a successful formulated diet. Aquaculture 426-427, 310–317. doi:10.1016/j.aquaculture.2014.02.005
Martínez R., Sántos R., Álvarez A., Cuzón G., Arena L., Mascaró M., et al. (2011). Partial characterization of hepatopancreatic and extracellular digestive proteinases of wild and cultivated Octopus maya. Aquacult. Int. 19, 445–457. doi:10.1007/s10499-010-9360-5
Martínez R., Santos R., Mascaró M., Canseco L., Caamal-Monsreal C., Rosas C. (2012). Digestive dynamics during chyme formation of Octopus maya (Mollusca, Cephalopoda). Aquac. Res. 43, 1119–1126. doi:10.1111/j.1365-2109.2011.02915.x
Martínez-Montaño E., Uriarte I., Rosas C., Amthauer R., Romero A., Farías A. (2018). Replacing live feed with formulated diets in juvenile Patagonian red octopus (Enteroctopus megalocyathus). Aquac. Nutr. 24, 633–643. doi:10.1111/anu.12589
McLeod I. M., Clark T. D. (2016). Limited capacity for faster digestion in larval coral reef fish at an elevated temperature. PLOS ONE 11, e0155360. doi:10.1371/journal.pone.0155360
Meza-Buendía A. K., Trejo-Escamilla I., Piu M., Caamal-Monsreal C., Rodríguez-Fuentes G., Diaz F., et al. (2021). Why high temperatures limit reproduction in cephalopods? The case of Octopus maya. Aquac. Res. 52, 5111–5123. doi:10.1111/are.15387
Mirabeau O., Joly J.-S. (2013). Molecular evolution of peptidergic signaling systems in bilaterians. Proc. Natl. Acad. Sci. U. S. A. 110, E2028–E2037. doi:10.1073/pnas.1219956110
Moltschaniwskyj N., Johnston D. (2006). Evidence that lipid can be digested by the dumpling squid Euprymna tasmanica, but is not stored in the digestive gland. Mar. Biol. 149, 565–572. doi:10.1007/s00227-006-0246-z
Morote E., Rodríguez M., Mancera J. M., Moyano F. J., Muñoz J. (2011). Las enzimas digestivas como indicadores del estado nutricional en paralarvas de pulpo Octopus vulgaris Cuvier, 1797. Bol. Inst. Español Oceanogr. 21, 177–186.
Naef A. (1928). Die Cephalopoden (Embryologie). Fauna und Flora des Golfes von Neapel. Monographie 35. Roma, Italy; Berlin, Germany: Bardi; R. Friedländer & Sohn.
Nande M., Presa P., Roura Á., Andrews P. L. R., Pérez M. (2017). Prey capture, ingestion, and digestion dynamics of Octopus vulgaris paralarvae fed live zooplankton. Front. Physiol. 8, 573. doi:10.3389/fphys.2017.00573
Niimi A. J. (1978). Lag adjustment between estimated and actual physiological responses conducted in flow-through systems. J. Fish. Res. Bd. Can. 35, 1265–1269. doi:10.1139/f78-197
Nixon M. (1965). Some observations on the food intake and learning in Octopus vulgaris. Pubbl. Staz. Zool. Napoli 34, 329–339.
Nixon M. (1969). The time and frequency of responses by Octopus vulgaris to an automatic food dispenser. J. Zoology 158, 475–483. doi:10.1111/j.1469-7998.1969.tb02163.x
Norin T., Clark T. D. (2016). Measurement and relevance of maximum metabolic rate in fishes. J. Fish. Biol. 88, 122–151. doi:10.1111/jfb.12796
Noyola J., Mascaró M., Caamal-Monsreal C., Noreña-Barroso E., Díaz F., Re D., et al. (2013). Effect of temperature on energetic balance and fatty acid composition of early juveniles of Octopus maya. J. Exp. Mar. Biol. Ecol. 445, 156–165. doi:10.1016/j.jembe.2013.04.008
O'Dor R. K., Wells M. J. (1987). “Energy and nutrient flow,” in Cephalopod life cycles. Editors R. K. O'dor, and M. J. Wells (London: Academic Press), 109–131.
Obaldo L. G., Divakaran S., Tacon A. G. (2002). Method for determining the physical stability of shrimp feeds in water. Aquac. Res. 33, 369–377. doi:10.1046/j.1365-2109.2002.00681.x
Odekunle E. A., Semmens D. C., Martynyuk N., Tinoco A. B., Garewal A. K., Patel R. R., et al. (2019). Ancient role of vasopressin/oxytocin-type neuropeptides as regulators of feeding revealed in an echinoderm. BMC Biol. 17, 60. doi:10.1186/s12915-019-0680-2
Okutani K., Kimata M. (1964). Studies on chitinolytic enzyme present in aquatic animals: III distribution of chitinase in digestive organs of a few kinds of aquatic animals. Bull Jpn. Soc. Sci. Fish. 30, 574–576. doi:10.2331/suisan.30.574
Oliveira A. R., Sardinha-Silva A., Andrews P. L. R., Green D., Cooke G. M., Hall S., et al. (2020). Microplastics presence in cultured and wild-caught cuttlefish, Sepia officinalis. Mar. Pollut. Bull. 160, 111553. doi:10.1016/j.marpolbul.2020.111553
Olsson C. (2009). Autonomic innervation of the fish gut. Acta Histochem. 111, 185–195. doi:10.1016/j.acthis.2008.11.014
Omedes S., Andrade M., Escolar O., Villanueva R., Freitas R., Solé M. (2022). B-esterases characterisation in the digestive tract of the common octopus and the European cuttlefish and their in vitro responses to contaminants of environmental concern. Environ. Res. 210, 112961. doi:10.1016/j.envres.2022.112961
Omura A., Endo H. (2016). The functional-morphological adaptive strategy of digestive organs of decapodiform cephalopods. J. Vet. Med. Sci. 78, 43–47. doi:10.1292/jvms.15-0185
Øresland R., Oxby G. (2021). A photo-illustrated dissection guide for bobtail squids. Sweden: Divers and scientists West Coast Sweden.
Packard A. (1972). Cephalopods and fish: The limits of convergence. Biol. Rev. 47, 241–307. doi:10.1111/j.1469-185x.1972.tb00975.x
Pascual C., Mascaro M., Rodríguez-Canul R., Gallardo P., Sánchez A. A., Rosas C., et al. (2019). Sea surface temperature modulates physiological and immunological condition of Octopus maya. Front. Physiol. 10, 739. doi:10.3389/fphys.2019.00739
Pavlov I. P. (1897). The work of the digestive glands. London, UK: C. Griffin & Company Limited. Translated by W.H. Thompson, 1902.
Pedà C., Longo F., Berti C., Laface F., De Domenico F., Consoli P., et al. (2022). The waste collector: Information from a pilot study on the interaction between the common octopus (Octopus vulgaris, cuvier, 1797) and marine litter in bottom traps fishing and first evidence of plastic ingestion. Mar. Pollut. Bull. 174, 113185. doi:10.1016/j.marpolbul.2021.113185
Percie du Sert N., Ahluwalia A., Alam S., Avey M. T., Baker M., Browne W. J., et al. (2020). Reporting animal research: Explanation and elaboration for the ARRIVE guidelines 2.0. PLoS Biol. 18, e3000411. doi:10.1371/journal.pbio.3000411
Pereda S. V., Uriarte I., Cabrera J. C. (2009). Effect of diet and paralarval development on digestive enzyme activity in the cephalopod Robsonella fontaniana. Mar. Biol. 156, 2121–2128. doi:10.1007/s00227-009-1242-x
Ponte G., Modica M. V. (2017). Salivary glands in predatory mollusks: Evolutionary considerations. Front. Physiol. 8, 580. doi:10.3389/fphys.2017.00580
Ponte G., Sykes A. V., Cooke G. M., Almansa E., Andrews P. L. R. (2017). The digestive tract of cephalopods: Toward non-invasive in vivo monitoring of its physiology. Front. Physiol. 8, 403. doi:10.3389/fphys.2017.00403
Ponte G., Taite M., Borrelli L., Tarallo A., Allcock A. L., Fiorito G. (2021). Cerebrotypes in cephalopods: Brain diversity and its correlation with species habits, life history, and physiological adaptations. Front. Neuroanat. 14, 565109. doi:10.3389/fnana.2020.565109
Pörtner H.-O., Bock C., Mark F. C. (2017). Oxygen- and capacity-limited thermal tolerance: Bridging ecology and physiology. J. Exp. Biol. 220, 2685–2696. doi:10.1242/jeb.134585
Quintela J., Andrade J. P. (2002a). Diel feeding rhythms, daily ration and gastric evacuation rates of Sepia officinalis in the Ria Formosa Lagoon (South Portugal). Bull. Mar. Sci. 71, 665–680.
Quintela J., Andrade J. P. (2002b). Effects of temperature on gastric evacuation rates in Sepia officinalis (Linnaeus, 1758) in laboratory conditions. Bull. Mar. Sci. 71, 681–689.
Ritschard E. A., Fitak R. R., Simakov O., Johnsen S. (2019). Genomic signatures of G-protein-coupled receptor expansions reveal functional transitions in the evolution of cephalopod signal transduction. Proc. Biol. Sci. 286, 20182929. doi:10.1098/rspb.2018.2929
Rodrigo A. P., Costa P. M. (2017). The role of the cephalopod digestive gland in the storage and detoxification of marine pollutants. Front. Physiol. 8, 232. doi:10.3389/fphys.2017.00232
Rokni D., Hochner B. (2002). Ionic currents underlying fast action potentials in the obliquely striated muscle cells of the Octopus arm. J. Neurophysiol. 88, 3386–3397. doi:10.1152/jn.00383.2002
Rosa R., Costa P. R., Nunes M. L. (2004). Effect of sexual maturation on the tissue biochemical composition of Octopus vulgaris and O. defilippi (Mollusca: Cephalopoda). Mar. Biol. 145, 563–574. doi:10.1007/s00227-004-1340-8
Rosas C., Cuzon G., Pascual C., Gaxiola G., Chay D., López N., et al. (2007). Energy balance of Octopus maya fed crab or an artificial diet. Mar. Biol. 152, 371–381. doi:10.1007/s00227-007-0692-2
Rosas C., Tut J., Baeza J., Sánchez A., Sosa V., Pascual C., et al. (2008). Effect of type of binder on growth, digestibility, and energetic balance of Octopus maya. Aquaculture 275, 291–297. doi:10.1016/j.aquaculture.2008.01.015
Rosas-Luis R. (2016). Description of plastic remains found in the stomach contents of the jumbo squid Dosidicus gigas landed in Ecuador during 2014. Mar. Pollut. Bull. 113, 302–305. doi:10.1016/j.marpolbul.2016.09.060
Roumbedakis K., Alexandre M. N., Puch J. A., Martins M. L., Pascual C., Rosas C. (2020). Short and long-term effects of anesthesia in Octopus maya (cephalopoda, octopodidae) juveniles. Front. Physiol. 11, 697. doi:10.3389/fphys.2020.00697
Ruder T., Sunagar K., Undheim E. a. B., Ali S. A., Wai T.-C., Low D. H. W., et al. (2013). Molecular phylogeny and evolution of the proteins encoded by coleoid (cuttlefish, Octopus, and squid) posterior venom glands. J. Mol. Evol. 76, 192–204. doi:10.1007/s00239-013-9552-5
Ruth P., Schipp R., Arnold J. M. (1999). Organization and function of the midgut glands of Nautilus pompilius L. and Nautilus macromphalus SOW. (Cephalopoda, Tetrabranchiata). Zoology 102, 196–211.
Sakamoto T., Ogawa S., Nishiyama Y., Akada C., Takahashi H., Watanabe T., et al. (2015). Osmotic/ionic status of body fluids in the euryhaline cephalopod suggest possible parallel evolution of osmoregulation. Sci. Rep. 5, 14469. doi:10.1038/srep14469
Sanders K. M. (2019). “Spontaneous electrical activity and rhythmicity in gastrointestinal smooth muscles,” in Smooth muscle spontaneous activity: Physiological and pathological modulation. Editors H. Hashitani, and R. J. Lang (Singapore: Springer), 3–46.
Semmens J. M. (2002). Changes in the digestive gland of the loliginid squid Sepioteuthis lessoniana (Lesson 1830) associated with feeding. J. Exp. Mar. Biol. Ecol. 274, 19–39. doi:10.1016/s0022-0981(02)00165-x
Sokolova I. M., Frederich M., Bagwe R., Lannig G., Sukhotin A. A. (2012a). Energy homeostasis as an integrative tool for assessing limits of environmental stress tolerance in aquatic invertebrates. Mar. Environ. Res. 79, 1–15. doi:10.1016/j.marenvres.2012.04.003
Sokolova I. M., Sukhotin A. A., Lannig G. (2012b). “Stress effects on metabolism and energy budgets in mollusks,” in Oxidative stress in aquatic ecosystems. Editors D. Abele, J. P. Vazquez-Medina, and T. Zenteno-Savin (Boston: Wiley-Blackwell), 263–280.
Spasiano E. (2020). Characterization of the “little brain” of Octopus vulgaris (Mollusca, Cephalopoda). Naples: Università degli Studi di Napoli "Federico II". MSc Master Degree.
Steffensen J. F. (1989). Some errors in respirometry of aquatic breathers: How to avoid and correct for them. Fish. Physiol. Biochem. 6, 49–59. doi:10.1007/BF02995809
Svendsen M. B. S., Bushnell P. G., Steffensen J. F. (2016). Design and setup of intermittent-flow respirometry system for aquatic organisms. J. Fish. Biol. 88, 26–50. doi:10.1111/jfb.12797
Sykes A. V., Almansa E., Cooke G. M., Ponte G., Andrews P. L. R. (2017). The digestive tract of cephalopods: A neglected topic of relevance to animal welfare in the laboratory and aquaculture. Front. Physiol. 8, 492. doi:10.3389/fphys.2017.00492
Sykes A. V., Almansa E., Ponte G., Cooke G. M., Andrews P. L. R. (2020). Can cephalopods vomit? Hypothesis based on a review of circumstantial evidence and preliminary experimental observations. Front. Physiol. 11, 765. doi:10.3389/fphys.2020.00765
Takei Y. (2021). The digestive tract as an essential organ for water acquisition in marine teleosts: Lessons from euryhaline eels. Zool. Lett. 7, 10. doi:10.1186/s40851-021-00175-x
Takuwa-Kuroda K., Iwakoshi-Ukena E., Kanda A., Minakata H. (2003). Octopus, which owns the most advanced brain in invertebrates, has two members of vasopressin/oxytocin superfamily as in vertebrates. Regul. Pept. 115, 139–149. doi:10.1016/s0167-0115(03)00151-4
Tam J. K. V., Lee L. T. O., Jin J., Chow B. K. C. (2014). Molecular evolution of GPCRS: Secretin/secretin receptors. J. Mol. Endocrinol. 52, T1–T14. doi:10.1530/JME-13-0259
Tedeschi G., Negri A., Ceciliani F., Ronchi S., Vetere A., D'aniello G., et al. (1994). Properties of the flavoenzyme d-aspartate oxidase from Octopus vulgaris. Biochim. Biophys. Acta 1207, 217–222. doi:10.1016/0167-4838(94)00071-9
Ueda A., Nagai H., Ishida M., Nagashima Y., Shiomi K. (2008). Purification and molecular cloning of SE-cephalotoxin, a novel proteinaceous toxin from the posterior salivary gland of cuttlefish Sepia esculenta. Toxicon. 52, 574–581. doi:10.1016/j.toxicon.2008.07.007
Van Heukelem W. F. (1976). Growth, bioenergetics and life-span of Octopus cyanea and Octopus maya. Manoa: University of Hawai'i at Manoa.
Villanueva R., Koueta N., Riba J., Boucaud-Camou E. (2002). Growth and proteolytic activity of Octopus vulgaris paralarvae with different food rations during first feeding, using Artemia nauplii and compound diets. Aquaculture 205, 269–286. doi:10.1016/s0044-8486(01)00678-0
Villanueva R., Perricone V., Fiorito G. (2017). Cephalopods as predators: A short journey among behavioral flexibilities, adaptions, and feeding habits. Front. Physiol. 8, 1–12. doi:10.3389/fphys.2017.00598
Voss G. L. (1988). “Evolution and phylogenetic relationships of deep-sea octopods (Cirrata and Incirrata),” in The Mollusca. Paleontology and neontology of cephalopods. Editors M. R. Clarke, and E. R. Trueman (San Diego: Academic Press), 253–276.
Wallace I., O'dor R., Amaratunga T. (1981). Sequential observations on the digestive process in the squid Illex illecebrosus. NAFO Sci. Counc. Stud. 1, 65–69.
Wells M. J., Clarke A., Clarke M. R. (1996). Energetics: The costs of living and reproducing for an individual cephalopod. Philosophical Trans. R. Soc. Lond. Ser. B Biol. Sci. 351, 1083–1104.
Wells M. J., O'dor R. K., Mangold K., Wells J. (1983). Diurnal changes in activity and metabolic rate in Octopus vulgaris. Mar. Behav. Physiology 9, 275–287. doi:10.1080/10236248309378598
Wells M. J. (1978). Octopus: Physiology and behaviour of an advanced invertebrate. Springer Science & Business Media.
Wells M. J., Wells J. (1993). Fluid uptake and the maintenance of blood volume in octopus. J. Exp. Biol. 175, 211–218. doi:10.1242/jeb.175.1.211
Wells M. J., Wells J. (1989). Water uptake in a cephalopod and the function of the so-called ‘pancreas. J. Exp. Biol. 145, 215–226. doi:10.1242/jeb.145.1.215
Westermann B., Ruth P., Litzlbauer H. D., Beck I., Beuerlein K., Schmidtberg H., et al. (2002). The digestive tract of Nautilus pompilius (cephalopoda, tetrabranchiata): An X-ray analytical and computational tomography study on the living animal. J. Exp. Biol. 205, 1617–1624. doi:10.1242/jeb.205.11.1617
Westermann B., Schipp R. (1998a). Cytological and enzyme-histochemical investigations on the digestive organs of Nautilus pompilius (Cephalopoda, Tetrabranchiata). Cell Tissue Res. 293, 327–336. doi:10.1007/s004410051124
Westermann B., Schipp R. (1998b). Morphology and histology of the digestive tract of Nautilus pompilius and Nautilus macromphalus (Cephalopoda, Tetrabranchiata). Zoomorphology 117, 237–245. doi:10.1007/s004350050048
Whitelaw B. L., Cooke I. R., Finn J., Da fonseca R. R., Ritschard E. A., Gilbert M. T. P., et al. (2020). Adaptive venom evolution and toxicity in octopods is driven by extensive novel gene formation, expansion, and loss. GigaScience 9, giaa120. doi:10.1093/gigascience/giaa120
Williams L. W. (1909). The anatomy of the common squid Loligo pealii, Lesueur. Leiden, Holland: E.J. Brill.
Wong K. K. Y., Ng S. Y. L., Lee L. T. O., Ng H. K. H., Chow B. K. C. (2011). Orexins and their receptors from fish to mammals: A comparative approach. General Comp. Endocrinol. 171, 124–130. doi:10.1016/j.ygcen.2011.01.001
Wood J. D. (1969). Electrophysiological and pharmacological properties of the stomach of the squid Loligo pealii (Lesueur). Comp. Biochem. Physiol. 30, 813–824. doi:10.1016/0010-406x(69)90036-x
Worth A. A., Forrest A. S., Peri L. E., Ward S. M., Hennig G. W., Sanders K. M. (2015). Regulation of gastric electrical and mechanical activity by cholinesterases in mice. J. Neurogastroenterol. Motil. 21, 200–216. doi:10.5056/jnm14120
Young J. Z. (1971). The anatomy of the nervous system of Octopus vulgaris. London, UK: Oxford University Press.
Young J. Z. (1965). The buccal nervous system of Octopus. Philosophical Trans. R. Soc. Lond. Ser. B, Biol. Sci. 249, 27–44.
Young J. Z. (1967). The visceral nerves of Octopus. Philosophical Trans. R. Soc. Lond. Ser. B, Biol. Sci. 253, 1–22.
Young J. Z. (1960). Unit processes in the formation of representations in the memory of Octopus. Proc. R. Soc. Lond. Ser. B. Biol. Sci. 153, 1–17.
Zatylny-Gaudin C., Bernay B., Zanuttini B., Leprince J., Vaudry H., Henry J. (2010). Characterization of a novel LFRFamide neuropeptide in the cephalopod Sepia officinalis. Peptides 31, 207–214. doi:10.1016/j.peptides.2009.11.021
Zatylny-Gaudin C., Cornet V., Leduc A., Zanuttini B., Corre E., Le Corguillé G., et al. (2016). Neuropeptidome of the cephalopod Sepia officinalis: Identification, tissue mapping, and expression pattern of neuropeptides and neurohormones during egg laying. J. Proteome Res. 15, 48–67. doi:10.1021/acs.jproteome.5b00463
Zatylny-Gaudin C., Favrel P. (2014). Diversity of the RFamide peptide family in mollusks. Front. Endocrinol. 5, 178. doi:10.3389/fendo.2014.00178
Keywords: octopus, digestive tract, digestive gland, motility, digestion, secretion, welfare, Directive 2010/63/EU
Citation: Andrews PLR, Ponte G and Rosas C (2022) Methodological considerations in studying digestive system physiology in octopus: limitations, lacunae and lessons learnt. Front. Physiol. 13:928013. doi: 10.3389/fphys.2022.928013
Received: 25 April 2022; Accepted: 01 August 2022;
Published: 09 September 2022.
Edited by:
Fernando Ariel Genta, Oswaldo Cruz Foundation (Fiocruz), BrazilReviewed by:
Pedro M. Costa, New University of Lisbon, PortugalCopyright © 2022 Andrews, Ponte and Rosas. This is an open-access article distributed under the terms of the Creative Commons Attribution License (CC BY). The use, distribution or reproduction in other forums is permitted, provided the original author(s) and the copyright owner(s) are credited and that the original publication in this journal is cited, in accordance with accepted academic practice. No use, distribution or reproduction is permitted which does not comply with these terms.
*Correspondence: Paul L. R. Andrews, cGFuZHJld3NAc2d1bC5hYy51aw==
Disclaimer: All claims expressed in this article are solely those of the authors and do not necessarily represent those of their affiliated organizations, or those of the publisher, the editors and the reviewers. Any product that may be evaluated in this article or claim that may be made by its manufacturer is not guaranteed or endorsed by the publisher.
Research integrity at Frontiers
Learn more about the work of our research integrity team to safeguard the quality of each article we publish.