- 1Centro Universitario de Investigaciones Biomédicas, CONACYT-Universidad de Colima, Colima, Mexico
- 2Departamento de Bioquímica, Centro de Investigación y de Estudios Avanzados, Mexico City, Mexico
PKC inhibitors stimulate Ca2+ release from internal stores in diverse cell types. Our data indicate that this action cannot be explained by an increased agonist-induced IP3 production or an overloaded SR Ca2+ pool in smooth muscle cells from guinea pig urinary bladder. The incubation of these cells with three different PKC inhibitors, such as Go6976, Go6983, and BIM 1, resulted in a higher SR Ca2+ leak revealed by inhibition of the SERCA pump with thapsigargin. This SR Ca2+ leakage was sensitive to protein translocation inhibitors such as emetine and anisomycin. Since this increased SR Ca2+ leak did not result in a depleted SR Ca2+ store, we have inferred there was a compensatory increase in SERCA pump activity, resulting in a higher steady-state. This new steady-state increased the frequency of Spontaneous Transient Outward Currents (STOCs), which reflect the activation of high conductance, Ca2+-sensitive potassium channels in response to RyR-mediated Ca2+ sparks. This increased STOC frequency triggered by PKC inhibition was restored to normal by inhibiting translocon-mediated Ca2+ leak with emetine. These results suggest a critical role of PKC-mediated translocon phosphorylation in regulating SR Ca2+ steady-state, which, in turn, alters SR Ca2+ releasing activity.
Introduction
A transient elevation of cytoplasmic Ca2+ ([Ca2+]i) induces changes in cell behavior such as muscle contraction, secretion, and neurotransmission, among others (Berridge et al., 2003). It is known that chemical, electrical and physical stimuli generate calcium influx at the plasma membrane and Ca2+ release from internal stores, producing an increase in the [Ca2+]i, which results in the activation of specific cellular mechanisms. Since the Ca2+ ion is a second messenger with a broad-spectrum role in cell signaling, the Ca2+ information is coded in the amplitude, location, and frequency of Ca2+ signals (Dolmetsch et al., 1997) to generate different behaviors in the same cell. The main internal calcium store is located in the endoplasmic reticulum (ER) or sarcoplasmic reticulum (SR) in muscle cells. This internal Ca2+ store is refilled by the action of Sarco/endoplasmic reticulum Ca2+ ATPase (SERCA), which in turn hydrolyzes one ATP and accumulates two Ca2+ ions in the ER/SR. This action results in a chemical gradient that provides a driving force for Ca2+ discharge upon activation of Ca2+ release channels (Toyoshima and Nomura, 2002). In smooth muscle cells, the two main Ca2+ release channels are the 1,4,5 inositol trisphosphate receptor (IP3R) and ryanodine receptor (RyR) (McGeown, 2004; Sanders, 2008). The leak Ca2+ channel is the third element involved in determining the amount of Ca2+ contained in the SR. The relevance of leak channels arises from establishing the ER/SR steady-state luminal [Ca2+] together with the SERCA pump (Gómez-Viquez et al., 2005). These changes in steady-state imply the existence of SERCA pump reserve to cope with conditions associated with increased ER/SR Ca2+ leak. Accordingly, this new steady-state level will increase the cellular energy burden. However, this futile cycle cannot be seen as a waste of energy since it participates in cell events such as thermoregulation (Bal and Periasamy, 2020). Despite the importance that Ca2+ leak channels play in establishing the luminal SR Ca2+ level, the molecular nature of this leak channel has not been identified yet in smooth muscle. A small fraction of RyRs is open in resting condition serving as Ca2+ leak channels (Gómez-Viquez et al., 2003; Dagnino-Acosta and Guerrero-Hernández, 2009). In other cells, ER Ca2+ leak channels appear to be different from IP3Rs and RyRs, being the translocon one of those ER Ca2+ leak channels (Camello et al., 2002; Lomax et al., 2002). Translocon is a pore complex that allows peptide movement in and out of the ER (Rapoport et al., 2017). This channel has a large pore to accommodate proteins, which, unregulated, will rapidly dissipate the ER/SR Ca2+ gradient. Indeed, a small peptide coded within the translocon functions as a plug for this channel (Itskanov et al., 2021), reducing its Ca2+ leakiness; additionally, bound ribosomes decrease Ca2+ release via translocon (Crowley et al., 1994; Roy and Wonderlin, 2003; van Coppenolle et al., 2004). Moreover, calmodulin and BiP proteins also inhibit the Ca2+ leak activity of translocon (Erdmann et al., 2011; Schäuble et al., 2012).
The question is whether the translocon normally functions as ER/SR leak channel (Lemos et al., 2021), particularly in smooth muscle cells (Amer et al., 2009). Translocon in immortalized cell lines appears to be part of the ER Ca2+ leak (Flourakis et al., 2006; Schäuble et al., 2012; Linxweiler et al., 2017). However, freshly isolated smooth muscle cells do not present an open translocon, based on the observation that SERCA pump inhibition does not reduce luminal SR Ca2+ levels even after several minutes (Gómez-Viquez et al., 2003, 2005).
In smooth muscle cells, Ca2+ release from the SR is triggered by IP3-producing agonists and by conditions that activate RyRs (Dagnino-Acosta and Guerrero-Hernández, 2009). Localized activation of clusters of RyRs produces Ca2+ sparks in smooth muscle cells, those located near the plasma membrane activate a cluster of Ca2+-dependent K+ channels generating what is known as spontaneous transient outward currents (STOCs) (Muñoz et al., 1998). Agonists that activate PLC via G proteins induce the hydrolysis of PIP2 phospholipid in stoichiometric amounts of IP3 and DAG, while the former activates IP3Rs causing the transient elevation of the [Ca2+]i; the latter activates PKC and phosphorylates different proteins. Active PKC decreases Ca2+ release by phosphorylating membrane receptors (Ishida et al., 2021) or PLCβ (Yue et al., 2000), resulting in a substantial reduction in the agonist-mediated IP3 production and, in turn, Ca2+ release.
We have studied the mechanism used by inhibitors of PKC to facilitate agonist-mediated Ca2+ release in smooth muscle cells, particularly Go6976 because this inhibitor stimulates Ca2+ release while inhibiting the carbachol-induced IP3 response. This apparent paradox was clarified by showing that PKC inhibitors trigger SR Ca2+ leak that seems to be occurring via translocon, and SERCA pumps compensate for this leak. This new steady-state in the luminal SR Ca2+ level facilitates agonist-induced Ca2+ release. Our results suggest that PKC activity might be modulating translocon-mediated SR Ca2+ leak, which modifies SR Ca2+ releasing activity.
Materials and Methods
Ethics Statement on Animal Use
All animal care and experimental procedures were performed according to the Mexican Official Norm for the Use and Care of Laboratory Animals (NOM-062-ZOO-1999). The protocol was reviewed and approved by the local Ethics Committee on Animal Experimentation (CICUAL, Cinvestav) with 0306–06 and renewed with the reference number 0131–15. Animals were bred and housed in the Cinvestav Animal facility with clean air and controlled light and temperature. Food and water were given ad libitum. Precautions were implemented to minimize animal use and reduce pain and distress.
Isolation of Single Smooth Muscle Cells From Guinea Pig Urinary Bladder
Single smooth muscle cells were isolated using the previously described method (Dagnino-Acosta and Guerrero-Hernández, 2009). The urinary bladder was surgically removed from male guinea pigs, followed by urothelium detachment. The cleaned detrusor muscle (200 mg) was minced in 20 mg pieces; these pieces were digested with preactivated collagenase and papain. Contaminating DNA was minimized with two cycles of incubation with DNAse I for 15 min each in dissection solution. Single smooth muscle cells were obtained by gentle mechanical dispersion with a plastic pipette, and the quantity and quality of cells for each preparation were verified with an optical microscope. Cells were then resuspended in fresh dissection solution and loaded for 1 h in the dark with Mag-Fluo4/AM, followed by two centrifugations at 500 x g with new recording saline solution, and the cell pellet was resuspended in recording saline solution. Smooth muscle cells were stored at 4°C for a minimum of 2 hours before being used the same day.
Calcium Measurements in Intact Smooth Muscle Cells Loaded With Fura-2/AM
Smooth muscle cells were loaded with 2 μM Fura-2/AM in the dark for 1 h at room temperature. Fura-2 loaded smooth muscle cells were placed on the stage of an inverted microscope and dually excited with a PTI DeltaRamV attached to a Nikon Diaphot TMD inverted microscope. Fluorescence excited at 340 and 380 nm were recorded at 510 nm in response to the addition of 20 mM caffeine, 100 μM carbachol, or 10 μM thapsigargin dissolved in recording saline solution and applied using a puffer pipette placed 10 μm away from the cell. These compounds were applied to the cell with a WPI PV830 pico pump (Aguilar-Maldonado et al., 2003).
Simultaneous Recording of Both the Cytoplasmic (Fura-2) and the SR Luminal [Ca2+] (Mag-fluo4) in Single Patch-Clamped Dialyzed Smooth Muscle Cells
Smooth muscle cells were loaded with 5 μM Mag-Fluo4/AM in the dark at room temperature for 1 h. Mag-Fluo4 loaded smooth muscle cells were placed on the stage of an inverted microscope connected to a PTI microfluorometer as described before. Single smooth muscle cells were patch clamped in the whole-cell configuration, with a holding membrane potential of 0 mV, and dialyzed with a pipette solution containing 100 μM Fura-2 free acid for at least 10 min to ensure that Mag-Fluo4 was removed from the cytoplasm and at the same time loading cells with enough Fura-2 to reliably measure changes in the [Ca2+]i (Dagnino-Acosta and Guerrero-Hernández, 2009).
Spontaneous Transient Outward Currents (STOCs) Recording With the Patch-Clamp Technique
To record spontaneous transient outward currents (STOCs), single muscle cells were patched in the whole-cell configuration and held at 0 mV. The frequency of STOCs was determined for 1 min. Digitized ion current data was stored to be analyzed offline. The frequency of STOCs was determined using the peak detector tool of the Origin program using a threshold ≥20 pA (Gómez-Viquez et al., 2003).
Quantification of Inositol 1,4,5-Trisphosphate Production (IP3) with the Mass Assay
To determine IP3 production, we followed a procedure previously described (Rueda et al., 2002b). Briefly, the cerebella of 12 male rats were homogenized in a solution containing Tris-HCl (50 mM), EDTA (1 mM), and mercaptoethanol (1 mM) at pH 8.0 using 15 ml of solution per gr of tissue. Residual tissue debris and nuclei were eliminated by centrifugation at 20,000 x g for 15 min. The resulting pellet was resuspended using a ratio of 2.5 mg of protein per ml of solution, and the IP3R-enriched microsomes were kept at -20°C for 2 days before being used for IP3 binding assays. Competitive binding between myo-[3H]inositol 1,4,5-trisphosphate radioactive and nonradioactive IP3 gave us an IC50 of approximately 10 nM with a Hill coefficient close to 1. The nonspecific binding was obtained using 4 µM of nonradioactive IP3.
The amount of 1,4,5-trisphosphate IP3 produced in 25 mg of smooth muscle detrusor preincubated in Li solution with or without PKC inhibitors and thapsigargin for 30 min was determined with and without 100 μM carbachol stimulation for 5 s. The IP3 production was stopped with 24% perchloric acid solution and kept at four°C. The IP3 containing solution was adjusted to a pH 8 with a solution containing TRIS (100 mM), EDTA (50 mM), and KOH (1.5 M). The amount of IP3 produced was calculated using the fraction of labeled IP3 released from cerebellar IP3R microsomes with the equation: IP3 = k (1-b)/b, where the k value was obtained for each determination using 75 and 150 pmol of IP3 standards. The b value was obtained with the equation b = (sample-NS)/(Bo-NS), where Bo was one of the standards, and NS was the nonspecific binding signal (Rueda et al., 2002b).
Solutions and Chemicals
The dissociation solution contained (in mM): 55 NaCl, 6 KCl, 5 MgCl2, 10 glucose, 80 NaOH, 80 glutamic acid, and 10 HEPES, pH 7.4 (NaOH). The recording saline solution contained (in mM): 137 NaCl, 5 KCl, 4 NaHCO3, 2 CaCl2, 2 MgCl2, 0.42 KH2PO4, 10 glucose, and 10 HEPES, pH 7.4 (NaOH). The pipette internal solution contained (in mM): 80 glutamic acid, 80 KOH, 5 NaCl, 40 KCl, 2 MgCl2, 2 Na2ATP, 0.1 GTP, 20 HEPES, pH 7.2 (KOH). The internal solution also contained 100 μM of Fura-2 acid in experiments where SR and cytosolic calcium were measured simultaneously. The Li solution contained (in mM): 120 NaCl, 10 LiCl, 4 KCl, 2 NaHCO3, 2 CaCl2, 1 MgSO4, 10 glucose, and 10 HEPES, pH 7.4 (NaOH).
Mag-Fluo4/AM, Fura-2/AM, and Fura-2-free acid were purchased from Molecular Probes (Eugene, OR). Thapsigargin was purchased from RBI. All other chemicals were from Sigma-Aldrich. Fura-2/AM, Mag-Fluo4/AM, Go6976, Go6983, BIM1, and Thapsigargin were dissolved in a DMSO 1000x stock solution and stored before use. Fura-2-free acid and carbachol were dissolved in water, and aliquots were stored separately at -20°C before being used. Daily freshly dissolved caffeine was prepared in the recording saline solution. All experiments were performed at room temperature (24°C).
Data and Statistical Analysis
Data for IP3 [Ca2+]i, luminal SR Ca2+ level, and STOCs are reported as mean ± SEM, where n represents either the number of cells studied or the number of independent experiments to determine IP3 production. Statistical analysis was carried out with Student`s t-test or ANOVA using Dunnett or SNK posthoc analysis. The differences were considered significant when p < 0.05.
Results
Inhibition of PKC With Go6976 Inhibits Carbachol-Induced IP3 Response
In smooth muscle cells from guinea pig urinary bladder, the interaction of carbachol with muscarinic receptors leads to PLCβ activation, resulting in the production of both inositol 1,4,5 trisphosphate (IP3) and diacylglycerol (DAG). The incubation with carbachol (100 μM) for only 5 s significantly increased more than double the IP3 production (Figure 1, left green bar). It is known that PMA-activated PKC inhibits IP3 production in smooth muscle (Brock et al., 1985; Aguilar-Maldonado et al., 2003). It is plausible then that incubation with inhibitors of PKC might increase IP3 production. If the PKC-feedback loop is absent in this cell type, these inhibitors should not affect agonist-induced IP3 production. Unexpectedly, the preincubation of muscle tissue with 200 nM Go6976, a potent inhibitor of classic isoforms of PKC (Gordge and Jonathan Ryves, 1994), for 30 min, totally inhibited the carbachol-induced IP3 response (Figure 1, middle green bar. n = 6). Although the presence of lithium should be inhibiting 1IP phosphatase in our assay conditions (Hallcher and Sherman, 1980), which is one of the IP3 degradation pathways, it is feasible that the agonist-induced Ca2+ increase might stimulate a Ca2+-dependent IP3 kinase limiting the rise in IP3 levels. Therefore, we incubated smooth muscle with 100 nM thapsigargin (to deplete SR Ca2+ stores) and 200 nM Go6976 for 30 min before the stimulation of IP3 production with carbachol (Figure 1, right bars). However, the inhibition of the [Ca2+]i response with thapsigargin did not restore the agonist-induced IP3 production. This analysis suggests that Go6976 strongly inhibits IP3 production.
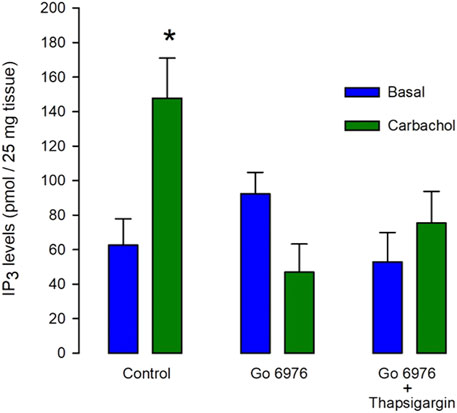
FIGURE 1. The carbachol-induced IP3 production is decreased by Go6976, a specific inhibitor of PKC. Guinea pig urinary bladder smooth muscle fragments were incubated with carbachol (100 μM) for 5 s (left green bar) or vehicle (left blue bar), and IP3 levels were measured using the mass assay. Different tissue fragments were preincubated with Go6976 (200 nM) for 30 min (central bars) or with the combination of Go6976 (200 nM) and thapsigargin (100 nM) before the addition of carbachol (right bars). IP3 levels are expressed as average pmols produced in 25 mg of tissue ±standard error, n = 6. ANOVA, significance was determined with Newman_Keuls post-hoc test with *p = 0.0041.
The Incubation With Go6976 Potentiates Agonist-Induced [Ca2+]i Responses
Despite the strong reduction in the carbachol-induced IP3 response, the carbachol-induced [Ca2+]i response was significantly elevated (Figure 2, green trace vs. blue trace). All the parameters examined were significantly modified by the incubation with 200 nM Go6978. Indeed, the amplitude of the carbachol-induced [Ca2+]i response (Figure 2B) went from 494 ± 52 nM (n = 11) to 718 ± 67 nM (n = 19), the peak rate of rise (Figure 2C) increased from 570 ± 91 nM/s (n = 11) to 919 ± 105 nM/s (n = 19) while the rising time (Figure 2D) was significantly decreased since it went from 2.81 ± 0.29 s (n = 11) to 1.96 ± 0.18 s (n = 19) by the inhibition of PKC with Go6976. Since Go6976 did not promote a larger carbachol-induced IP3 production, we studied the possibility that the incubation with Go6976 could result in an overloaded SR Ca2+ store. The smooth muscle SR is endowed with the IP3 receptor and Ryanodine Receptor (RyR); these receptors share the same SR Ca2+ store in this cell type (Rueda et al., 2002a). The application of low levels of caffeine (2 mM) displayed a faster and increased [Ca2+]i response (Figure 3A, solid line) that went from 149 ± 7 nM (n = 8, blue trace) to 233 ± 25 nM (n = 8, green trace) in cells incubated with 200 nM Go6976. A saturating concentration of caffeine (20 mM) that activates all RyR at once can be used as an indirect indicator of the size of the SR Ca2+ store. In this case, caffeine-induced [Ca2+]i response did not display any difference between cells incubated with Go6976 (Figure 3B, green trace, 211 ± 26 nM, n = 8) and control (Figure 3B, blue trace, 166 ± 8 nM, n = 8). These data show that inhibition of PKC potentiates both the carbachol- and caffeine-induced [Ca2+]i responses, but it seems unlikely that this was due to an overloaded SR Ca2+ store.
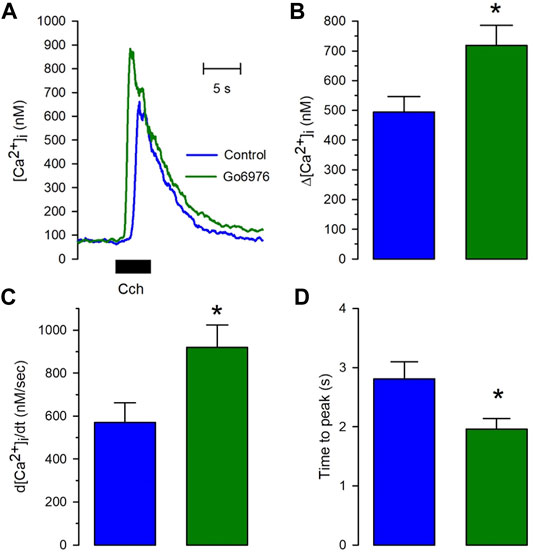
FIGURE 2. The calcium release induced by carbachol is potentiated in cells pretreated with Go6976. Single smooth muscle cells were loaded with Fura-2/AM and incubated with or without 200 nM Go6976 for 30 min before the exposure to 100 μM carbachol for 5 s using a puffer pipette placed next to the cell. (A) Representative carbachol-induced [Ca2+]i response in cells incubated with (green trace) and without Go6976 (blue trace). (B) The peak amplitude with (green bar) and without Go6976 (blue trace). (C) The peak rate of [Ca2+]i rise with (green bar) and without Go6976 (blue trace). (D) Time to the peak of the carbachol-induced [Ca2+]i response with (green bar) and without Go6976 (blue bar). The bars show the average ±standard error for control (n = 11) and Go6976 (n = 19). Significance was assessed using Student´s t-test. *p < 0.05.
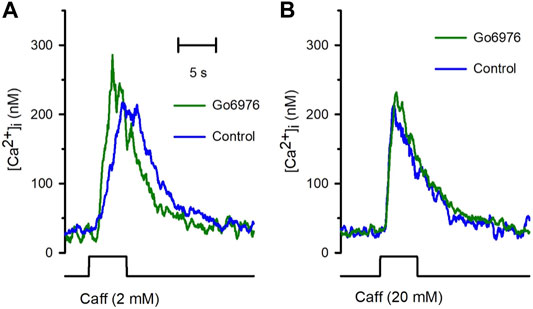
FIGURE 3. The incubation with Go6976 potentiates caffeine-induced [Ca2+]i responses. Single smooth muscle cells loaded with Fura-2/AM were stimulated with caffeine using a puffer pipette placed next to the cell for the time indicated in the bottom black trace (5 s). (A) Representative traces of the [Ca2+]i response for cells preincubated with (green trace) and without (blue trace) Go6976 and stimulated with 2 mM caffeine at the time indicated (bottom black trace). (B) Representative traces of the [Ca2+]i responses stimulated with 20 mM of caffeine for cells preincubated with (green trace) and without (blue trace) Go6976 (n = 8).
The Inhibition of PKC Increases the Ca2+ Leak From the SR Store
To study the effect of Go6976-induced PKC inhibition on the luminal SR Ca2+ level, we simultaneously recorded changes in Mag-fluo4 and Fura-2 fluorescence to measure modifications in the luminal SR (Figure 4, blue trace) and the [Ca2+]i (Figure 4, brown trace), respectively. We have previously shown that the SR Ca2+ store does not present an evident Ca2+ leak under our recording conditions (Gómez-Viquez et al., 2003, 2005; Dagnino-Acosta and Guerrero-Hernández, 2009). Indeed, applying 10 μM thapsigargin for 5 s to inhibit the SERCA pump resulted in a small increase in the [Ca2+]i because this pump no longer buffers the plasma membrane Ca2+ entry (Figure 4, dotted line). Still, there was no discernible SR Ca2+ leak for the recorded time (Figure 4, dashed line). We have previously shown that inhibition of SERCA pumps produces a large reduction in the Ca2+ availability to release channels in smooth muscle cells (Gómez-Viquez et al., 2003; Dagnino-Acosta and Guerrero-Hernández, 2009). Accordingly, the agonist-induced [Ca2+]i response was significantly diminished (Figure 5A, second application of carbachol) since the [Ca2+]i response was 159 ± 15 nM (n = 7). However, the same protocol but in cells incubated with 200 nM Go6976 resulted in a clear reduction in the SR Ca2+ level in response to the inhibition of SERCA pumps with thapsigargin (Figure 5B, red dashed line). This effect was associated with a transient elevation of the [Ca2+]i suggesting an increased SR Ca2+ leak and a faster reduction of the SR Ca2+ content upon thapsigargin application. Indeed, a second stimulation with carbachol induced a significantly smaller [Ca2+]i response (78 ± 17 nM, n = 5). The third application of carbachol-induced no further reduction of the luminal SR Ca2+ level nor any increase in the [Ca2+]i (Figure 5). These observations support the contention that the 5-s application of 10 μM thapsigargin irreversibly inhibited SERCA pumps. Moreover, although the luminal SR Ca2+ level was not calibrated as an absolute value of [Ca2+]SR, the luminal SR Ca2+ nadir (-0.06 ± 0.02 ΔF/Fo, n = 7) was not different (-0.07 ± 0.02 DF/Fo, n = 5) when cells were incubated with 200 nM Go6976. If Go6976 had induced a Ca2+ overloaded SR, a smaller Ca2+ leak should be expected since a less leaky SR would be easier to overload with Ca2+. Nevertheless, Go6976 increased the SR Ca2+ leak.
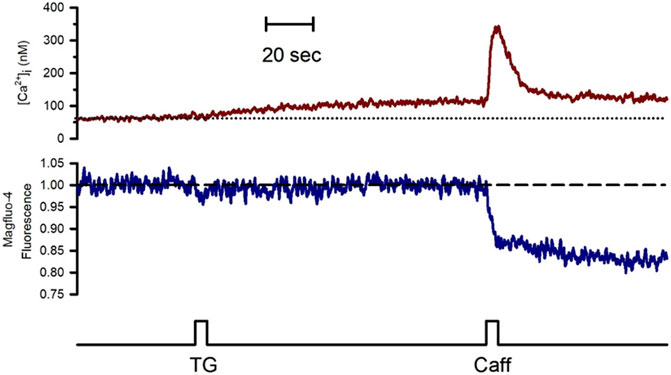
FIGURE 4. The inhibition of the SERCA pump with thapsigargin revealed a weak SR Ca2+ leak in the freshly isolated guinea pig urinary bladder. Single smooth muscle cells previously loaded with Mag-fluo-4/AM were dialyzed with the whole configuration of the patch-clamp technique (to remove the excess of cytoplasmic Mag-fluo-4) with a pipette solution containing Fura-2 free acid and kept at 0 mV to record the [Ca2+]i (red trace) and the luminal SR Ca2+ level (blue trace). The application of 10 μM thapsigargin with a puffer pipette placed next to the cell at the time indicated by the bottom black trace resulted in a slow and slight elevation of the [Ca2+]i with no reduction in the luminal SR Ca2+ level. Representative trace of n = 10.
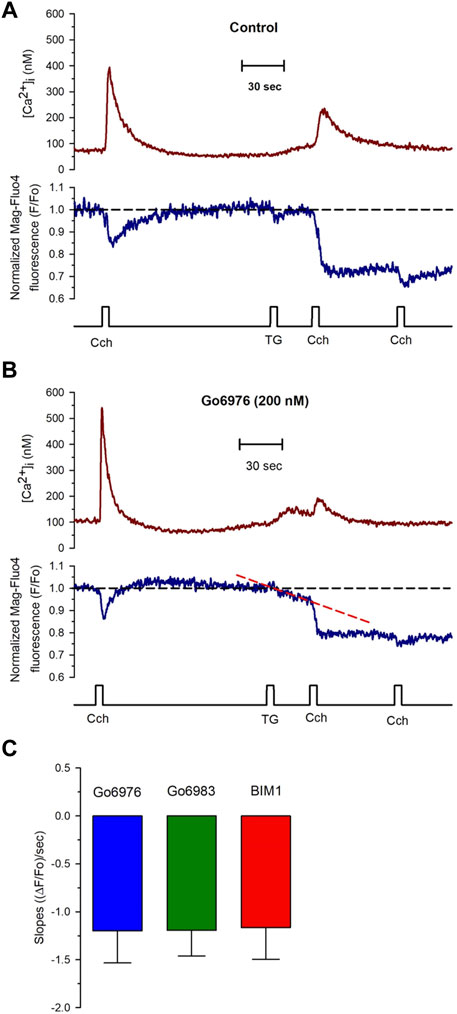
FIGURE 5. PKC inhibitors generate a compensated SR calcium leak in smooth muscle cells revealed with the application of thapsigargin. Single smooth muscle cells previously loaded with Mag-fluo-4/AM were dialyzed with the whole configuration of the patch-clamp technique (to remove the excess of cytoplasmic Mag-fluo-4) with a pipette solution containing Fura-2 free acid and kept at 0 mV to record the [Ca2+]i (red trace) and the luminal SR Ca2+ level (blue trace). (A) Representative traces of control cells exposed to 100 μM carbachol at the time indicated, followed by the exposure to 10 μM thapsigargin and another application of carbachol 30 s after thapsigargin. The application of thapsigargin did not reduce the luminal SR Ca2+ level. (B) Representative traces were obtained from smooth muscle cells incubated with Go6976. The application of thapsigargin revealed an apparent SR Ca2+ leak, as indicated by the red dashed line. (C) Smooth muscle cells were incubated with different PKC inhibitors such as Go6976 (blue bars, n = 8), Go6983 (green bar, n = 6), and BIM1 (red bar, n = 7), and the thapsigargin-induced SR Ca2+ leak slope was determined as the mean ± error standard for the number of indicated cells.
Go6976 inhibits classic PKCs and PKD (DAVIES et al., 2000). To determine whether PKD was participating in the process, we used Go6983, another inhibitor of PKC that does not inhibit PKD. Incubation of cells with 200 nM of Go6983 induced an SR Ca2+ leak similar to the one caused by Go6976 (Figure 5C, green bar). These data suggest that inhibition of PKCs was responsible for the induction of the SR Ca2+ leak. Indeed, incubation of smooth muscle cells with 5 μM BIM 1 also produced the same magnitude of SR Ca2+ leak based on the slope of SR Ca2+ reduction (Figure 5C, red bar). It has been reported that Go6976 increases the activity of EGF receptors (Shah et al., 2005). We decided to incubate cells with Go6976 and AG1478, an inhibitor of the EGF receptor (Shah et al., 2005). The slope of the thapsigargin-induced reduction in the luminal SR Ca2+ level (1.17 × 10−3 ± 0.34 × 10−3 ((ΔF/Fo)/sec), n = 4) was similar to the one obtained with only Go6976. These data imply that the effect of Go6976 was due to the inhibition of PKCs.
Inhibition of PKC Increases SR Ca2+ Leak via the Translocon in Smooth Muscle Cells
All these data show that PKC inhibition activated an SR Ca2+ leak, implying the activation of release channels (IP3Rs or RyRs) or some other type of Ca2+-permeable channel in the SR. 2 mM MgCl2 inside the pipette solution inhibits the RyR-mediated SR Ca2+ leak. Yet, this condition did not interfere with the Go6976-induced SR Ca2+ leak (Figures 5B, C). Additionally, the IP3Rs do not appear to participate in the enhanced SR Ca2+ leak based on the observation that the presence of heparin in the pipette solution did not decrease the enhanced STOCs frequency induced by Go6976 (Figure 7C). Translocon is a pore that allows the nascent peptide to reach the ER lumen while functioning as a Ca2+ leak in the ER (van Coppenolle et al., 2004; Linxweiler et al., 2017). We decided to study whether PKC inhibition might be activating the translocon. We used two previously reported translocon-mediated Ca2+ leak inhibitors, emetine and anisomycin. They inhibit protein translation by stalling the nascent peptide in the ribosome (van Coppenolle et al., 2004; Ong et al., 2007; Amer et al., 2009). The presence of 10 μM emetine inhibited the effect of 200 nM Go6976 on the stimulation of Ca2+ leak (Figures 6A,B). Emetine has decreased the leakiness of the SR Ca2+ store, so the second stimulation displayed a larger [Ca2+]i response than with only Go6976 vs. Figures 6A,B. The presence of anisomycin (200 μM, Figure 6C) or emetine (10 μM, Figure 6C) significantly reduced the slope of thapsigargin-induced SR Ca2+ leak (compare the slopes of the red dashed lines in vs. Figures 6A,B).
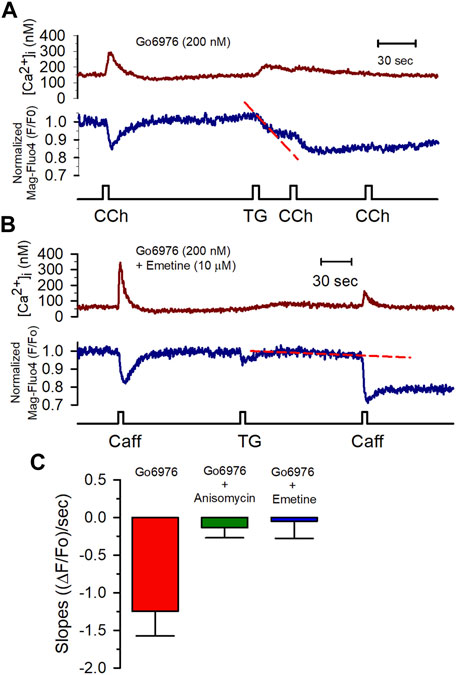
FIGURE 6. Anisomycin and emetine blocked the Go6976-induced SR Ca2+ leak. (A) [Ca2+]i (red trace) and [Ca2+]SR (blue trace) changes in response to CCh (100 μM) from a smooth muscle cell incubated with Go6976 (200 nM). The application of thapsigargin resulted in a rapid and partial depletion of the [Ca2+]SR (red dashed line) with strong inhibition of the CCh-induced responses. (B) Caffeine-induced changes in the [Ca2+]i (red trace) and the [Ca2+]SR (blue trace) from a smooth muscle cell incubated with both Go6976 (200 nM) and emetine (10 μM). The application of TG resulted in a much smaller SR Ca2+ leak (red dashed line). (C) The slope of the SR signal obtained after blocking the SERCA pump with thapsigargin was evaluated in cells incubated with Go6976 and vehicle (red bar, n = 5), 200 μM anisomycin (green bar, n = 4), and 10 μM emetine (blue bar, n = 6) and plotted as the average ±standard error. Dunnett post-hoc with *p < 0.05.
A New Steady State in the SR Ca2+ Content Stimulates RyR-Mediated Ca2+ Release
Spontaneous transient outward currents (STOCs) are potassium currents carried out by Ca2+-dependent large-conductance K+ channels activated by a Ca2+ spark due to the coordinated opening of a small cluster of RyRs (ZhuGe et al., 1999; Cheranov and Jaggar, 2002). Figure 7A shows that Ca2+ release by IP3R (carbachol application) did not increase the BKCa current, while caffeine activated the BKCa current with the same increase in the [Ca2+]i. The frequency of STOCs increases with a higher RyR activity (ZhuGe et al., 1999; Cheranov and Jaggar, 2002). The incubation of cells with 200 nM Go6976 significantly increased the frequency of STOCs (Figure 7C, green bar, n = 5), suggesting that the inhibition of PKC increased the spontaneous activation of RyRs. Moreover, the inhibition of IP3Rs with 5 mg/ml of heparin in the pipette solution did not modify the effect of Go6976 (Figure 7C, red bar, n = 5). However, the presence of emetine (10 μM) fully inhibited the Go6976-induced increased STOCs frequency (Figure 7C, cyan bar, n = 6) without interfering with the STOCs amplitude. Collectively, these data suggest that the inhibition of PKC in smooth muscle cells induces a translocon-mediated SR Ca2+ leak that appears to be compensated by an increase in the SERCA pump activity. This new steady-state seems to facilitate the spontaneous activation of RyRs.
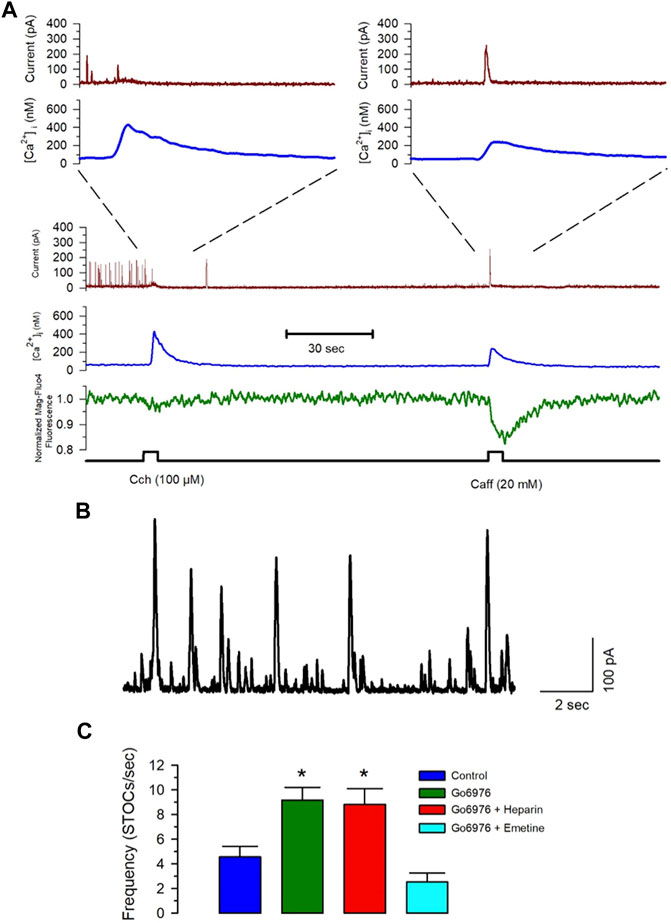
FIGURE 7. Emetine blocks the Go6976-induced increased STOC frequency. Freshly isolated smooth muscle cells were loaded with Mag-fluo-4/AM and dialyzed with Fura-2 free acid in the pipette solution while holding membrane potential at 0 mV. (A) The spontaneous and calcium-sensitive potassium currents were recorded (brown trace) together with the [Ca2+]i (blue trace) and the luminal SR Ca2+ level (green trace) during the application of carbachol or caffeine at the time indicated (bottom black trace). Both stimuli induced a significant elevation of the [Ca2+]i, with the main difference that carbachol. However, inhibited STOCs, did not cause a generalized activation of STOCs, while caffeine clearly stimulated transient outward currents. (B) Representative trace of STOCs in a control smooth muscle cell. (C) Frequency of STOCs per second plotted as the average ±standard error for control (blue bar), with 200 nM Go6976 (green bar), with 200 nM Go6976 with 5 mg/ml heparin in the pipette solution (red bar), and with 200 nM Go6976 and 10 μM emetine (cyan bar). ANOVA, Dunnett post-hoc test, *p < 0.05.
Discussion
We have found that PKC inhibition stimulated Ca2+ release from the SR in response to carbachol and caffeine in smooth muscle cells isolated from guinea pig urinary bladder. However, we could not find evidence of an overloaded Ca2+ store to explain this effect. Moreover, PKC inhibition reveals a translocon-mediated SR Ca2+ leak without reducing the agonist-induced [Ca2+]i responses. We have inferred that this situation reflects increased compensatory SERCA pump activity. This new steady-state facilitates Ca2+ release by both IP3Rs and RyRs. The latter resulted in a higher STOCs frequency. Overall, these data point to the SR Ca2+ leak role in determining the steady-state luminal SR Ca2+ level, particularly the participation of translocon in defining the SR Ca2+ leakage.
The Translocon Plays a Role in the SR Ca2+ Leak in Smooth Muscle Cells
The inhibition of PKC with different inhibitors such as Go6976, Go6983, and BIM1 resulted in a reduction of the luminal SR Ca2+ level upon inhibition of the SERCA pump. This SR Ca2+ leak was not inhibited by MgCl2 or heparin in the pipette solution, arguing against RyRs or IP3Rs being the SR Ca2+ leak channels responding to the inhibitors of PKC. However, emetine and anisomycin inhibited this SR Ca2+ leak supporting the idea that Sec61 translocon participates in this SR Ca2+ leak.
The translocon from eukaryotic cells is a protein complex formed by the three subunits of SEC61 (α, β, γ), Sec62, and Sec63 proteins, oligosaccharyltransferase complex (ribophorin I, ribophorin II, and OST48), TRAM, and the TRAP protein complex (TRAP α, β, γ, and δ) (Potter and Nicchitta, 2002). Puromycin, an inhibitor of the protein synthesis that releases the nascent peptides leaving the ribosome attached to the open conformation of the translocon, has been critical for demonstrating the participation of translocon as an ER Ca2+ leak in different cell types (Camello et al., 2002; Lomax et al., 2002; Giunti et al., 2007; Ong et al., 2007). However, results obtained in proliferating vascular smooth muscle cells show that translocon could work as an SR Ca2+ leak since it is activated by puromycin and inhibited with anisomycin and emetine. Still, it does not participate in the thapsigargin-revealed SR Ca2+ leak (Amer et al., 2009). A result we have corroborated here as well. Our data suggest that constitutive PKC activity inhibits smooth muscle translocon SR Ca2+ leak activity. However, this effect of PKC is not exclusive to smooth muscle cells since we have data that PKC is also blocking the HeLa cell translocon Ca2+ leak activity.
The translocon contains a large pore of 50 Å in diameter, making it difficult for the SERCA pump to avoid Ca2+ pool depletion. Accordingly, an extensive account of molecular mechanisms reduces translocon Ca2+ leak activity (Lang et al., 2017). The pore ring of SEC61α involves hydrophobic amino acid residues that block the central pore (Voorhees and Hegde, 2016; Itskanov et al., 2021). The plug peptide, calmodulin, and BiP block the translocon Ca2+ leak activity (Erdmann et al., 2011; Schäuble et al., 2012; Lang et al., 2017; Rapoport et al., 2017). Mass spectrometry and biochemical studies have shown that several proteins of the translocon complex are phosphorylated in the absence of any stimulation. This phosphorylation is present in SEC61α, β, and γ, Sec62, Sec63, the two subunits of TRAM and the five subunits of TRAP (Gruss et al., 1999; Villén et al., 2007; Daub et al., 2008; Dephoure et al., 2008; Oppermann et al., 2009). Interestingly, SEC61β phosphorylation significantly improves peptide translation efficiency to the luminal part of the SR (Gruss et al., 1999), suggesting a better coupling of translocon and ribosomes. Additional target candidates for PKC phosphorylation under basal activity cannot be excluded (i.e., phosphorylation of the signal recognition particle and its receptor). However, we do not know how and which phosphorylated subunit or related protein reduces translocon Ca2+ leak activity.
Although staurosporine is a generalized kinase inhibitor, paradoxically, it can also activate kinases. This is the case, particularly with p38 MAPK (Xiao et al., 1999; Yamaki et al., 2010; Ramiro-Cortés et al., 2011). Moreover, both emetine (Kim et al., 2015) and anisomycin (Xiong et al., 2006; Sampieri et al., 2008) activate p38 MAPK. Additionally, the effect of protein synthesis inhibitors on P38 MAPK activity requires hours of incubation, while the Ca2+ leak inhibition occurs much faster. Since these two protein synthesis inhibitors blocked staurosporine-induced ER Ca2+ leak and all three inhibitors have the same effect on p38 MAPK, we think it is unlikely that modifications in p38 MAPK activity explain the effect of staurosporine seen in the present study.
Role of the SR Ca2+ Leak in Establishing the New Steady-State
A constant [Ca2+]SR results from the balance between Ca2+ leak and SERCA pump activity (Berridge et al., 2003; Friel and Chiel, 2008). One way to assess the size of this Ca2+ leakage is the fast inhibition of the SERCA pump with thapsigargin and to determine the rate of luminal SR Ca2+ reduction. Under our recording conditions, thapsigargin did not produce any rapid decrease in the [Ca2+]SR. This result cannot be explained by thapsigargin partially inhibiting the SERCA pump. These data indicate a comparable small SR Ca2+ leak and a small SERCA pump activity. However, PKC inhibitors increased translocon-mediated SR Ca2+ leak, implying that translocon phosphorylation reduces its Ca2+ leak activity in resting conditions. Because the PKC inhibitors did not deplete the SR Ca2+ pool, there must be a compensatory increase in SERCA pump activity and, arguably, higher ATP consumption.
Role of SERCA Pump Activity in the Facilitation of Ca2+ Release
Currently, the role of SERCA pumps in refilling the ER/SR Ca2+ pool is clearly established. However, data suggest that the SERCA pump sensitizes the propagating Ca2+ wave-front in secretory cells, facilitating an efficient calcium release (Huang et al., 2006). This conclusion was reached by observing that SERCA pump inhibition reduces the Ca2+ wave velocity without affecting the [Ca2+]i amplitude or decreasing the luminal calcium content (Keller et al., 2007). Moreover, RGS2 knockout stimulates agonist-induced Ca2+ release without evidence of an increased agonist-induced IP3 production or higher sensitivity of IP3Rs (Wang et al., 2004, 2005).
We have previously shown that SERCA pumps potentiate Ca2+ release in smooth muscle cells by a mechanism independent of its SR Ca2+ refilling activity (Gómez-Viquez et al., 2003). Blocking the SERCA pump produced a decreased amplitude and velocity of Ca2+ release with IP3R in HeLa cells (Aguilar-Maldonado et al., 2003) or with IP3Rs and RyRs in smooth muscle cells (Dagnino-Acosta and Guerrero-Hernández, 2009). The activation of β adrenergic receptors in heart cells stimulates Ca2+ release by increasing the activity of SERCA pumps without any evidence of an overloaded SR Ca2+ pool (Zhou et al., 1999; Maxwell and Blatter, 2012). This occurs because there is a compensatory higher RyR2 leak activity (Reinhardt et al., 2021; Nolla-Colomer et al., 2022). Therefore, examples abound showing SERCA pump increased activity facilitates Ca2+ release by generating a new steady-state.
The Role of Leak Channels in Establishing the Steady-State [Ca2+] and Their Effect on the SR Physiology
The evidence indicates that translocon presents significant participation in the ER Ca2+ leak in pancreatic acinar cells (Lomax et al., 2002), human salivary glands (Ong et al., 2007), liver microsomes from rats (Giunti et al., 2007), and LNCaP cells (van Coppenolle et al., 2004), suggesting that Ca2+ leak activity of Sec61α has a critical role in establishing the steady-state ER Ca2+ level. However, translocon does not seem to be operating as an SR Ca2+ leak channel under basal conditions in smooth muscle cells. Our data suggest that this is due to the constitutive PKC activity that results in a reduced SR Ca2+ leak. Nevertheless, not only translocon but other channels function as ER Ca2+ leak channels; for instance, the RyRs (Bellinger et al., 2008; Lehnart et al., 2008), the IP3Rs (Oakes et al., 2005), TRPV1(Lotteau et al., 2013), Orai2 (Bandara et al., 2013), and Orai3 (Leon-Aparicio et al., 2017), among others. This large variety of ion channels operating as ER/SR Ca2+ leak channels argues for the relevance of ER/SR Ca2+ leak in cell physiology.
The SR Functions as an Intelligent Compartment Handling Free Calcium
The calcium release from internal stores is organized in discrete units (Baddeley et al., 2011), resulting in quantal Ca2+ release events such as sparks for RyR or puffs for IP3Rs (Steenbergen and Fay, 1996; Hajnóczky and Thomas, 1997). The ER/SR is a network of interconnected tubules and cisternae without diffusion barriers; therefore, it is expected that activation of release channels leads to complete depletion of the ER/SR. However, ER/SR shows quantal Ca2+ release behavior (Wu and Bers, 2006; McCarron and Olson, 2008). There are several explanations for this phenomenon; one of them is that Ca2+ release channels (IP3Rs and RyRs) have preferential access to Ca2+ trapped in luminal proteins (Guerrero-Hernandez et al., 2010; Guerrero-Hernández et al., 2020), making an efficient Ca2+ release event. While the leak channels control SERCA pump activity by modifying the free luminal ER/SR [Ca2+], implying much smaller Ca2+ fluxes than when the total Ca2+ capacity of the ER/SR is involved. This separation between Ca2+ release channels and leak channels allows the ER/SR to function as a Ca2+ source without triggering ER stress by Ca2+ depletion or other activities that require an elevated free luminal [Ca2+]ER.
Altogether, our results indicate that PKC inhibitors increase the Ca2+ leak activity of translocon, which appears to be compensated by a higher SERCA pump activity to avoid SR Ca2+ depletion. This increased steady-state results in accelerated Ca2+ release in response to activation of either IP3Rs or RyRs. These results point to the scenario where the translocon Ca2+ leak modulates the Ca2+-releasing activity of the SR Ca2+ store.
Data Availability Statement
The original contributions presented in the study are included in the article/supplementary material, further inquiries can be directed to the corresponding author.
Ethics Statement
The animal study was reviewed and approved by the Ethics Committee on Animal Experimentation (CICUAL, Cinvestav) with the reference number 0306–06 and renewed with the reference number 0131–15.
Authors Contributions
AD-A: Performed research and analyzed data; AG-H: Designed research, analyzed data, wrote the original manuscript draft, supervised study, and acquired financial support. All authors read, corrected, and approved the final version of the manuscript.
Funding
This work was partially supported by CONACYT grant FC 2016-2803 to AG-H.
Conflict of Interest
The authors declare that the research was conducted in the absence of any commercial or financial relationships that could be construed as a potential conflict of interest.
Publisher’s Note
All claims expressed in this article are solely those of the authors and do not necessarily represent those of their affiliated organizations, or those of the publisher, the editors and the reviewers. Any product that may be evaluated in this article, or claim that may be made by its manufacturer, is not guaranteed or endorsed by the publisher.
Acknowledgments
The authors are especially thankful for the technical help provided by Lucía García and the animal facility personnel at CINVESTAV.
References
Aguilar-Maldonado B., Gómez-Viquez L., Garcı́a L., Del Angel R. M., Arias-Montaño J. A., Guerrero-Hernández A. (2003). Histamine Potentiates IP3-Mediated Ca2+ Release via Thapsigargin-Sensitive Ca2+Pumps. Cell. Signal. 15, 689–697. doi:10.1016/S0898-6568(03)00012-3
Amer M. S., Li J., O'Regan D. J., Steele D. S., Porter K. E., Sivaprasadarao A., et al. (2009). Translocon Closure to Ca2+ Leak in Proliferating Vascular Smooth Muscle Cells. Am. J. Physiol. Heart Circ. Physiol. 296, H910–H916. doi:10.1152/AJPHEART.00984.2008/SUPPL_FILE/SUPPLEMENTAL
Baddeley D., Crossman D., Rossberger S., Cheyne J. E., Montgomery J. M., Jayasinghe I. D., et al. (2011). 4D Super-resolution Microscopy with Conventional Fluorophores and Single Wavelength Excitation in Optically Thick Cells and Tissues. PLoS ONE 6, e20645. doi:10.1371/journal.pone.0020645
Bal N. C., Periasamy M. (2020). Uncoupling of Sarcoendoplasmic Reticulum Calcium ATPase Pump Activity by Sarcolipin as the Basis for Muscle Non-shivering Thermogenesis. Phil. Trans. R. Soc. B 375, 20190135. doi:10.1098/rstb.2019.0135
Bandara S., Malmersjö S., Meyer T. (2013). Regulators of Calcium Homeostasis Identified by Inference of Kinetic Model Parameters from Live Single Cells Perturbed by siRNA. Sci. Signal. 6. ra56. doi:10.1126/scisignal.2003649
Bellinger A. M., Reiken S., Dura M., Murphy P. W., Deng S.-X., Landry D. W., et al. (2008). Remodeling of Ryanodine Receptor Complex Causes "leaky" Channels: a Molecular Mechanism for Decreased Exercise Capacity. Proc. Natl. Acad. Sci. U. S. A. 105, 2198–2202. doi:10.1073/pnas.0711074105
Berridge M. J., Bootman M. D., Roderick H. L. (2003). Calcium Signalling: Dynamics, Homeostasis and Remodelling. Nat. Rev. Mol. Cell Biol. 4, 517–529. doi:10.1038/nrm1155
Brock T. A., Rittenhouse S. E., Powers C. W., Ekstein L. S., Gimbrone M. A., Alexander R. W. (1985). Phorbol Ester and 1-Oleoyl-2-Acetylglycerol Inhibit Angiotensin Activation of Phospholipase C in Cultured Vascular Smooth Muscle Cells. J. Biol. Chem. 260, 14158–14162. doi:10.1016/S0021-9258(17)38697-0
Camello C., Lomax R., Petersen O. H., Tepikin A. v. (2002). Calcium Leak from Intracellular Stores-The Enigma of Calcium Signalling. Cell Calcium 32, 355–361. doi:10.1016/S0143416002001926
Cheranov S. Y., Jaggar J. H. (2002). Sarcoplasmic Reticulum Calcium Load Regulates Rat Arterial Smooth Muscle Calcium Sparks and Transient KCacurrents. J. Physiology 544, 71–84. doi:10.1113/JPHYSIOL.2002.025197
Crowley K. S., Liao S., Worrell V. E., Reinhart G. D., Johnson A. E. (1994). Secretory Proteins Move through the Endoplasmic Reticulum Membrane via an Aqueous, Gated Pore. Cell 78, 461–471. doi:10.1016/0092-8674(94)90424-3
Dagnino-Acosta A., Guerrero-Hernández A. (2009). Variable Luminal Sarcoplasmic Reticulum Ca2+ Buffer Capacity in Smooth Muscle Cells. Cell Calcium 46, 188–196. doi:10.1016/j.ceca.2009.07.005
Daub H., Olsen J. V., Bairlein M., Gnad F., Oppermann F. S., Körner R., et al. (2008). Kinase-selective Enrichment Enables Quantitative Phosphoproteomics of the Kinome across the Cell Cycle. Mol. Cell 31, 438–448. doi:10.1016/J.MOLCEL.2008.07.007
Davies S. P., Reddy H., Caivano M., Cohen P. (2000). Specificity and Mechanism of Action of Some Commonly Used Protein Kinase Inhibitors. Biochem. J. 351, 95–105. doi:10.1042/bj3510095
Dephoure N., Zhou C., Villén J., Beausoleil S. A., Bakalarski C. E., Elledge S. J., et al. (2008). A Quantitative Atlas of Mitotic Phosphorylation. Proc. Natl. Acad. Sci. U.S.A. 105, 10762–10767. doi:10.1073/PNAS.0805139105
Dolmetsch R. E., Lewis R. S., Goodnow C. C., Healy J. I. (1997). Differential Activation of Transcription Factors Induced by Ca2+ Response Amplitude and Duration. Nature 386, 855–858. doi:10.1038/386855a0
Erdmann F., Schäuble N., Lang S., Jung M., Honigmann A., Ahmad M., et al. (2011). Interaction of Calmodulin with Sec61α Limits Ca2+leakage from the Endoplasmic Reticulum. EMBO J. 30, 17–31. doi:10.1038/EMBOJ.2010.284
Flourakis M., Van Coppenolle F., Lehen'Kyi V. Y., Beck B., Skryma R., Prevarskaya N., et al. (2006). Passive Calcium Leak via Translocon Is a First Step for iPLA2-pathway Regulated Store Operated Channels Activation. FASEB J. 20, 1215–1217. doi:10.1096/FJ.05-5254FJE
Friel D. D., Chiel H. J. (2008). Calcium Dynamics: Analyzing the Ca2+ Regulatory Network in Intact Cells. Trends Neurosci. 31, 8–19. doi:10.1016/J.TINS.2007.11.004
Giunti R., Gamberucci A., Fulceri R., Bánhegyi G., Benedetti A. (2007). Both translocon and a Cation Channel Are Involved in the Passive Ca2+ Leak from the Endoplasmic Reticulum: a Mechanistic Study on Rat Liver Microsomes. Archives Biochem. Biophysics 462, 115–121. doi:10.1016/J.ABB.2007.03.039
Gómez-Viquez L., Guerrero-Serna G., García U., Guerrero-Hernández A. (2003). SERCA Pump Optimizes Ca2+ Release by a Mechanism Independent of Store Filling in Smooth Muscle Cells. Biophysical J. 85, 370–380. doi:10.1016/S0006-3495(03)74481-6
Gómez-Viquez L., Rueda A., García U., Guerrero-Hernández A. (2005). Complex Effects of Ryanodine on the Sarcoplasmic Reticulum Ca2+ Levels in Smooth Muscle Cells. Cell Calcium 38, 121–130. doi:10.1016/j.ceca.2005.06.002
Gordge P. C., Jonathan Ryves W. (1994). Inhibitors of Protein Kinase C. Cell. Signal. 6, 871–882. doi:10.1016/0898-6568(94)90020-5
Gruss O. J., Feick P., Frank R., Dobberstein B. (1999). Phosphorylation of Components of the ER Translocation Site. Eur. J. Biochem. 260, 785–793. doi:10.1046/J.1432-1327.1999.00215.X
Guerrero-Hernandez A., Dagnino-Acosta A., Verkhratsky A. (2010). An Intelligent Sarco-Endoplasmic Reticulum Ca2+ Store: Release and Leak Channels Have Differential Access to a Concealed Ca2+ Pool. Cell Calcium 48, 143–149. doi:10.1016/j.ceca.2010.08.001
Guerrero-Hernández A., Sánchez-Vázquez V. H., Martínez-Martínez E., Sandoval-Vázquez L., Perez-Rosas N. C., Lopez-Farias R., et al. (2020). Sarco-Endoplasmic Reticulum Calcium Release Model Based on Changes in the Luminal Calcium Content. Adv. Exp. Med. Biol. 1131. 337, 370. doi:10.1007/978-3-030-12457-1_14
Hajnóczky G., Thomas A. P. (1997). Minimal Requirements for Calcium Oscillations Driven by the IP3 Receptor. EMBO J. 16, 3533–3543. doi:10.1093/EMBOJ/16.12.3533
Hallcher L. M., Sherman W. R. (1980). The Effects of Lithium Ion and Other Agents on the Activity of Myo-Inositol-1-Phosphatase from Bovine Brain. J. Biol. Chem. 255, 10896–10901. doi:10.1016/S0021-9258(19)70391-3
Huang G., Yao J., Zeng W., Mizuno Y., Kamm K. E., Stull J. T., et al. (2006). ER Stress Disrupts Ca2+-Signaling Complexes and Ca2+ Regulation in Secretory and Muscle Cells from PERK-Knockout Mice. J. Cell Sci. 119, 153–161. doi:10.1242/JCS.02731
Ishida Y., Kitayama K., Hanada K., Shibutani S., Nishizaki K., Kinjo T., et al. (2021). Diltiazem Inhibits Coronary Spasm via Inhibition of Cav1.2Phosphorylation and Protein Kinase C Activation in a Mouse Model of Coronary Spastic Angina. Int. Heart J. 62, 910–918. doi:10.1536/IHJ.20-366
Itskanov S., Kuo K. M., Gumbart J. C., Park E. (2021). Stepwise Gating of the Sec61 Protein-Conducting Channel by Sec63 and Sec62. Nat. Struct. Mol. Biol. 28, 162–172. doi:10.1038/s41594-020-00541-x
Keller M., Kao J., Egger M., Niggli E. (2007). Calcium Waves Driven by "sensitization" Wave-Fronts. Cardiovasc. Res. 74, 39–45. doi:10.1016/J.CARDIORES.2007.02.006
Kim J. H., Cho E. B., Lee J., Jung O., Ryu B. J., Kim S. H., et al. (2015). Emetine Inhibits Migration and Invasion of Human Non-small-cell Lung Cancer Cells via Regulation of ERK and P38 Signaling Pathways. Chemico-Biological Interact. 242, 25–33. doi:10.1016/J.CBI.2015.08.014
Lang S., Pfeffer S., Lee P.-H., Cavalié A., Helms V., Förster F., et al. (2017). An Update on Sec61 Channel Functions, Mechanisms, and Related Diseases. Front. Physiol. 8, 887. doi:10.3389/fphys.2017.00887
Lehnart S. E., Mongillo M., Bellinger A., Lindegger N., Chen B.-X., Hsueh W., et al. (2008). Leaky Ca2+ Release Channel/ryanodine Receptor 2 Causes Seizures and Sudden Cardiac Death in Mice. J. Clin. Invest. 118, 2230. doi:10.1172/JCI35346
Lemos F. O., Bultynck G., Parys J. B. (2021). A Comprehensive Overview of the Complex World of the Endo- and Sarcoplasmic Reticulum Ca2+-Leak Channels. Biochimica Biophysica Acta (BBA) - Mol. Cell Res. 1868, 119020. doi:10.1016/j.bbamcr.2021.119020
Leon-Aparicio D., Pacheco J., Chavez-Reyes J., Galindo J. M., Valdes J., Vaca L., et al. (2017). Orai3 Channel Is the 2-APB-Induced Endoplasmic Reticulum Calcium Leak. Cell Calcium 65, 91–101. doi:10.1016/j.ceca.2017.01.012
Linxweiler M., Schick B., Zimmermann R. (2017). Let's Talk about Secs: Sec61, Sec62 and Sec63 in Signal Transduction, Oncology and Personalized Medicine. Sig Transduct. Target Ther. 2, 1–10. doi:10.1038/sigtrans.2017.2
Lomax R. B., Camello C., van Coppenolle F., Petersen O. H., Tepikin A. v. (2002). Basal and Physiological Ca2+ Leak from the Endoplasmic Reticulum of Pancreatic Acinar Cells. J. Biol. Chem. 277, 26479–26485. doi:10.1074/JBC.M201845200
Lotteau S., Ducreux S., Romestaing C., Legrand C., Van Coppenolle F. (2013). Characterization of Functional TRPV1 Channels in the Sarcoplasmic Reticulum of Mouse Skeletal Muscle. PLoS ONE 8, e58673. doi:10.1371/journal.pone.0058673
Maxwell J. T., Blatter L. A. (2012). Facilitation of Cytosolic Calcium Wave Propagation by Local Calcium Uptake into the Sarcoplasmic Reticulum in Cardiac Myocytes. J. Physiol. 590, 6037–6045. doi:10.1113/JPHYSIOL.2012.239434
McCarron J. G., Olson M. L. (2008). A Single Luminally Continuous Sarcoplasmic Reticulum with Apparently Separate Ca2+ Stores in Smooth Muscle. J. Biol. Chem. 283, 7206–7218. doi:10.1074/JBC.M708923200
McGeown J. G. (2004). Interactions between Inositol 1,4,5-trisphosphate Receptors and Ryanodine Receptors in Smooth Muscle: One Store or Two? Cell Calcium 35, 613–619. doi:10.1016/j.ceca.2004.01.016
Muñoz A., García L., Guerrero-Hernández A. (1998). In Situ characterization of the Ca2+ Sensitivity of Large Conductance Ca2+-Activated K+ Channels: Implications for Their Use as Near-Membrane Ca2+ Indicators in Smooth Muscle Cells. Biophysical J. 75, 1774–1782. doi:10.1016/S0006-3495(98)77619-2
Nolla-Colomer C., Casabella-Ramon S., Jimenez-Sabado V., Vallmitjana A., Tarifa C., Herraiz-Martínez A., et al. (2022). β2-adrenergic Stimulation Potentiates Spontaneous Calcium Release by Increasing Signal Mass and Co-activation of Ryanodine Receptor Clusters. Acta Physiol. 234, e13736. doi:10.1111/APHA.13736
Oakes S. A., Scorrano L., Opferman J. T., Bassik M. C., Nishino M., Pozzan T., et al. (2005). Proapoptotic BAX and BAK Regulate the Type 1 Inositol Trisphosphate Receptor and Calcium Leak from the Endoplasmic Reticulum. Proc. Natl. Acad. Sci. U.S.A. 102, 105–110. doi:10.1073/PNAS.0408352102
Ong H. L., Liu X., Sharma A., Hegde R. S., Ambudkar I. S. (2007). Intracellular Ca2+ Release via the ER Translocon Activates Store-Operated Calcium Entry. Pflugers Arch. - Eur. J. Physiol. 453, 797–808. doi:10.1007/S00424-006-0163-5
Oppermann F. S., Gnad F., Olsen J. V., Hornberger R., Greff Z., Kéri G., et al. (2009). Large-scale Proteomics Analysis of the Human Kinome. Mol. Cell. Proteomics 8, 1751–1764. doi:10.1074/MCP.M800588-MCP200
Potter M. D., Nicchitta C. V. (2002). Endoplasmic Reticulum-Bound Ribosomes Reside in Stable Association with the Translocon Following Termination of Protein Synthesis. J. Biol. Chem. 277, 23314–23320. doi:10.1074/jbc.M202559200
Ramiro-Cortés Y., Guemez-Gamboa A., Morán J. (2011). Reactive Oxygen Species Participate in the P38-Mediated Apoptosis Induced by Potassium Deprivation and Staurosporine in Cerebellar Granule Neurons. Int. J. Biochem. Cell Biol. 43, 1373–1382. doi:10.1016/j.biocel.2011.06.001
Rapoport T. A., Li L., Park E. (2017). Structural and Mechanistic Insights into Protein Translocation. Annu. Rev. Cell Dev. Biol. 33, 369–390. doi:10.1146/annurev-cellbio-100616-060439
Reinhardt F., Beneke K., Pavlidou N. G., Conradi L., Reichenspurner H., Hove-Madsen L., et al. (2021). Abnormal Calcium Handling in Atrial Fibrillation Is Linked to Changes in Cyclic AMP Dependent Signaling. Cells 10, 3042. doi:10.3390/CELLS10113042
Roy A., Wonderlin W. F. (2003). The Permeability of the Endoplasmic Reticulum Is Dynamically Coupled to Protein Synthesis. J. Biol. Chem. 278, 4397–4403. doi:10.1074/JBC.M207295200
Rueda A., Garcı́a L., Guerrero-Hernández A. (2002a). Luminal Ca2+ and the Activity of Sarcoplasmic Reticulum Ca2+ Pumps Modulate Histamine-Induced All-Or-None Ca2+ Release in Smooth Muscle Cells. Cell. Signal. 14, 517–527. doi:10.1016/S0898-6568(01)00284-4
Rueda A., Garcı́a L., Soria-Jasso L.-E., Arias-Montaño J.-A., Guerrero-Hernández A. (2002b). The Initial Inositol 1,4,5-trisphosphate Response Induced by Histamine Is Strongly Amplified by Ca2+ Release from Internal Stores in Smooth Muscle. Cell Calcium 31, 161–173. doi:10.1054/CECA.2002.0270
Sampieri C. L., Nuttall R. K., Young D. A., Goldspink D., Clark I. M., Edwards D. R. (2008). Activation of P38 and JNK MAPK Pathways Abrogates Requirement for New Protein Synthesis for Phorbol Ester Mediated Induction of Select MMP and TIMP Genes. Matrix Biol. 27, 128–138. doi:10.1016/j.matbio.2007.09.004
Sanders K. M. (2008). Regulation of Smooth Muscle Excitation and Contraction. Neurogastroenterol. Motil. 20, 39–53. doi:10.1111/j.1365-2982.2008.01108.x
Schäuble N., Lang S., Jung M., Cappel S., Schorr S., Ulucan Ö., et al. (2012). BiP-mediated Closing of the Sec61 Channel Limits Ca2+leakage from the ER. EMBO J. 31, 3282–3296. doi:10.1038/emboj.2012.189
Shah B. H., Olivares-Reyes J. A., Catt K. J. (2005). The Protein Kinase C Inhibitor Go6976 [12-(2-Cyanoethyl)-6,7,12,13-Tetrahydro-13-Methyl-5-Oxo-5h-Indolo(2,3-A)pyrrolo(3,4-C)-Carbazole] Potentiates Agonist-Induced Mitogen-Activated Protein Kinase Activation through Tyrosine Phosphorylation of the Epidermal Growth Factor Receptor. Mol. Pharmacol. 67, 184–194. doi:10.1124/MOL.104.003533
Steenbergen J. M., Fay F. S. (1996). The Quantal Nature of Calcium Release to Caffeine in Single Smooth Muscle Cells Results from Activation of the Sarcoplasmic Reticulum Ca2+-ATPase. J. Biol. Chem. 271, 1821–1824. doi:10.1074/jbc.271.4.1821
Toyoshima C., Nomura H. (2002). Structural Changes in the Calcium Pump Accompanying the Dissociation of Calcium. Nature 418, 605–611. doi:10.1038/nature00944
van Coppenolle F., vanden Abeele F., Slomianny C., Flourakis M., Hesketh J., Dewailly E., et al. (2004). Ribosome-translocon Complex Mediates Calcium Leakage from Endoplasmic Reticulum Stores. J. Cell Sci. 117, 4135–4142. doi:10.1242/jcs.01274
Villén J., Beausoleil S. A., Gerber S. A., Gygi S. P. (2007). Large-scale Phosphorylation Analysis of Mouse Liver. Proc. Natl. Acad. Sci. U.S.A. 104, 1488–1493. doi:10.1073/PNAS.0609836104
Voorhees R. M., Hegde R. S. (2016). Structure of the Sec61 Channel Opened by a Signal Sequence. Science 351, 88–91. doi:10.1126/SCIENCE.AAD4992
Wang X., Huang G., Luo X., Penninger J. M., Muallem S. (2004). Role of Regulator of G Protein Signaling 2 (RGS2) in Ca2+ Oscillations and Adaptation of Ca2+ Signaling to Reduce Excitability of RGS2-/- Cells. J. Biol. Chem. 279, 41642–41649. doi:10.1074/JBC.M406450200
Wang X., Zeng W., Soyombo A. A., Tang W., Ross E. M., Barnes A. P., et al. (2005). Spinophilin Regulates Ca2+ Signalling by Binding the N-Terminal Domain of RGS2 and the Third Intracellular Loop of G-Protein-Coupled Receptors. Nat. Cell Biol. 7, 405–411. doi:10.1038/NCB1237
Wu X., Bers D. M. (2006). Sarcoplasmic Reticulum and Nuclear Envelope Are One Highly Interconnected Ca 2+ Store Throughout Cardiac Myocyte. Circulation Res. 99, 283–291. doi:10.1161/01.RES.0000233386.02708.72
Xiao Y.-Q., Someya K.-i., Morita H., Takahashi K., Ohuchi K. (1999). Involvement of P38 MAPK and ERK/MAPK Pathways in Staurosporine-Induced Production of Macrophage Inflammatory Protein-2 in Rat Peritoneal Neutrophils. Biochimica Biophysica Acta (BBA) - Mol. Cell Res. 1450, 155–163. doi:10.1016/S0167-4889(99)00042-7
Xiong W., Kojic L. Z., Zhang L., Prasad S. S., Douglas R., Wang Y., et al. (2006). Anisomycin Activates P38 MAP Kinase to Induce LTD in Mouse Primary Visual Cortex. Brain Res. 1085, 68–76. doi:10.1016/j.brainres.2006.02.015
Yamaki K., Hong J., Hiraizumi K., Ahn J. W., Zee O., Ohuchi K. (2010). Participation of Various Kinases in Staurosporine-Induced Apoptosis of RAW 264.7 Cells. J. Pharm. Pharmacol. 54, 1535–1544. doi:10.1211/002235702144
Yue C., Ku C.-Y., Liu M., Simon M. I., Sanborn B. M. (2000). Molecular Mechanism of the Inhibition of Phospholipase C β3 by Protein Kinase C. J. Biol. Chem. 275, 30220–30225. doi:10.1074/JBC.M004276200
Zhou Y. Y., Song L. S., Lakatta E. G., Xiao R. P., Cheng H. (1999). Constitutive β 2-adrenergic Signalling Enhances Sarcoplasmic Reticulum Ca 2+ Cycling to Augment Contraction in Mouse Heart. J. Physiology 521, 351–361. doi:10.1111/j.1469-7793.1999.00351.x
Keywords: translocon, PKC, sarcoplasmic reticulum, IP3, smooth muscle, SERCA, calcium leak
Citation: Dagnino-Acosta A and Guerrero-Hernandez A (2022) PKC Inhibits Sec61 Translocon-Mediated Sarcoplasmic Reticulum Ca2+ Leak in Smooth Muscle Cells. Front. Physiol. 13:925023. doi: 10.3389/fphys.2022.925023
Received: 21 April 2022; Accepted: 03 June 2022;
Published: 28 June 2022.
Edited by:
Richard Zimmermann, Saarland University, GermanyReviewed by:
Volkhard Helms, Saarland University, GermanyDavid Thomas, The University of the Pacific, United States
Copyright © 2022 Dagnino-Acosta and Guerrero-Hernandez. This is an open-access article distributed under the terms of the Creative Commons Attribution License (CC BY). The use, distribution or reproduction in other forums is permitted, provided the original author(s) and the copyright owner(s) are credited and that the original publication in this journal is cited, in accordance with accepted academic practice. No use, distribution or reproduction is permitted which does not comply with these terms.
*Correspondence: Agustín Guerrero-Hernandez, YWd1ZXJyZXJvQGNpbnZlc3Rhdi5teA==