- 1School of Human Kinetics, University of Ottawa, Ottawa, ON, Canada
- 2Department of Cellular and Molecular Medicine, Centre for Neuromuscular Disease, University of Ottawa, Ottawa, ON, Canada
Human skeletal muscle is a remarkedly plastic tissue that has a high capacity to adapt in response to various stimuli. These adaptations are due in part to the function of muscle-resident stem/progenitor cells. Skeletal muscle regeneration and adaptation is facilitated by the activation and expansion of muscle stem cells (MuSCs). MuSC fate is regulated by signals released from cells in their niche, such as fibro-adipogenic progenitors (FAPs), as well as a variety of non-cellular niche components. Sufficient dietary protein consumption is critical for maximizing skeletal muscle adaptation to exercise and maintaining skeletal muscle in disease; however, the role of dietary protein in altering MuSC and FAP responses to exercise in healthy populations and skeletal muscle disease states requires more research. The present review provides an overview of this emerging field and suggestions for future directions. The current literature suggests that in response to resistance exercise, protein supplementation has been shown to increase MuSC content and the MuSC response to acute exercise. Similarly, protein supplementation augments the increase in MuSC content following resistance training. Endurance exercise, conversely, is an area of research that is sparse with respect to the interaction of protein supplementation and exercise on muscle stem/progenitor cell fate. Initial evidence suggests that protein supplementation augments the early myogenic response to acute endurance exercise but does not enhance the MuSC response to endurance training. Resistance training increases the number of proliferating FAPs with no additional effect of protein supplementation. Future research should continue to focus on the nutritional regulation of skeletal muscle stem/progenitor cell fate paired with studies examining the effects of exercise on a variety of human populations.
Introduction
Skeletal muscle is a remarkably plastic tissue that has a high capacity to adapt in response to different stimuli (Baldwin and Haddad, 2002). In response to resistance exercise, skeletal muscle undergoes hypertrophy and results in an increased muscle mass (Haun et al., 2019). Conversely, muscle disuse and catabolic conditions can cause muscle wasting and degeneration resulting in atrophy (Fanzani et al., 2012). Atrophy can be the result of malnutrition, inactivity, aging, and skeletal muscle disease (Fanzani et al., 2012; Rudrappa et al., 2016). Skeletal muscle mass accounts for roughly 30% of body weight in females and approximately 38% in males (Janssen et al., 2000), and it has an important role in nutrient storage and regulation of metabolism (Wolfe, 2006). Indeed, skeletal muscle contributes significantly to post-prandial glucose disposal, lipid oxidation, resting metabolic rate, and whole-body protein metabolism (Wolfe, 2006; Morton et al., 2015). Further, the role of skeletal muscle as an endocrine organ regulating multiple body systems is emerging (Severinsen and Pedersen, 2020). Therefore, the maintenance and regeneration of muscle mass is critical to ensure health across the lifespan.
Skeletal muscle regeneration is facilitated by the activation and expansion of myogenic muscle stem cells (MuSCs), also known as satellite cells (Wang and Rudnicki, 2011; Wang et al., 2014). MuSCs reside in skeletal muscle, adjacent to myofibers and beneath the basal lamina, in a state of quiescence (Katz, 1961; Mauro, 1961). Muscle damage and signals from cells in their niche, including fibro-adipogenic progenitors (FAPs), cause MuSCs to exit quiescence, proliferate, and differentiate to facilitate muscle repair and adaptation (Feige et al., 2018). To form a mature muscle fibre, MuSCs undergo multiple rounds of proliferation to generate sufficient myonuclei (Bischoff, 1990). Muscle regeneration occurs with several rounds of myoblast fusion and muscle fibre maturation (Knudsen and Horwitz, 1977). Additionally, while the majority of MuSCs go through proliferation and differentiation for skeletal muscle regeneration, a sub-group of MuSCs will undergo cell division to maintain the MuSC pool for future injuries (Collins et al., 2005; Sacco et al., 2008; Dumont et al., 2015). FAPs are muscle resident multipotent stromal cells that are directly involved in muscle adaptation through their promotion of MuSC proliferation and differentiation (Joe et al., 2010; Uezumi et al., 2010; Mozzetta et al., 2013; Uezumi et al., 2021). FAPs have adipogenic, fibrogenic, and osteogenic differentiation potential in vivo (Contreras et al., 2021) and are phenotypically identified primarily by their expression of PDGFRα and/or CD90 in humans (Uezumi et al., 2014a; Farup et al., 2021) as well as several recent markers that may distinguish FAP subpopulations (Contreras et al., 2021). FAPs are critical during muscle regeneration to sustain MuSC differentiation and to maintain the MuSC pool during normal development (Wosczyna et al., 2019) and aging (Lukjanenko et al., 2019). However, FAPs have a physiological dichotomy such that in pathological conditions FAP expansion results in an overproduction of fibro/fatty infiltration which leads to impaired myogenesis (Joe et al., 2010; Uezumi et al., 2010; Mozzetta et al., 2013; Contreras et al., 2016; Dong et al., 2017; Gonzalez et al., 2017; Madaro et al., 2018; Stumm et al., 2018).
Adequate dietary protein consumption throughout the lifespan is critical for maintaining optimal skeletal muscle health. Protein consumption plays a substantial role in the attenuation of many skeletal muscle disorders, including those caused by diabetes and age-related loss of muscle mass quantity and function, termed sarcopenia (Beaudry and Devries, 2019). Dietary protein consumption and dietary-derived amino acids have proven to be an effective approach to slow muscle protein catabolism in older adults (Paddon-Jones et al., 2004; Paddon-Jones, 2006). Additionally, protein consumption from a wide variety of sources such as whey supplementation (Park et al., 2019), yogurt (Bridge et al., 2019), whole egg (Van Vliet et al., 2017), and amino acids (Rieu et al., 2006), has a beneficial effect on muscle growth and strength. While a large body of literature and several excellent reviews (Atherton and Smith, 2012; Phillips, 2016; Burd et al., 2019) have described the interaction between dietary protein consumption, exercise, and muscle protein synthesis, the role of dietary protein consumption on MuSC and FAP responses to exercise have been largely unexplored. Therefore, the purpose of the current review is to highlight recent studies examining the role of dietary protein in altering MuSC and FAP responses to exercise, particularly hypertrophy-inducing resistance exercise in healthy populations and skeletal muscle disease states.
Role of Protein on Skeletal Muscle Mass and the MuSC Niche
Muscle mass is determined, in part, by the relative rates of both muscle protein synthesis (MPS) and muscle protein breakdown (MPB). In the fasted state, rates of MPB exceed MPS which results in a negative protein balance (Biolo et al., 1995). In response to protein consumption and an increase in plasma amino acid availability there will be a subsequent increase in MPS and decrease in MPB which will result in a net positive protein balance (Biolo et al., 1995; Glynn et al., 2010). Exercise also acts synergistically to the effects of protein consumption and augments the muscle protein synthetic response (McLeod et al., 2016; Burd and De Lisio, 2017). This response has been demonstrated following both resistance exercise (Burd et al., 2010; Burd et al., 2011; Reitelseder et al., 2011; Agergaard et al., 2017) and endurance exercise (Pikosky et al., 2006; Harber et al., 2010; Di Donato et al., 2014; Churchward-Venne et al., 2020). This increase anabolic response following exercise is thought to be due to an increased muscle sensitivity to hyperaminoacidemia, resulting in a greater muscle protein synthetic response to lower concentrations of protein intake (Churchward-Venne et al., 2012). In contrast, catabolic conditions such as physical inactivity shifts net protein balance to favour degradation, which, if sustained for an extended period can result in muscle atrophy (Vainshtein and Sandri, 2020). The fine-tune balance between protein synthesis and degradation in skeletal muscle is related to physical activity and nutritional status and can positively or negatively impact the cells of the muscle niche (Atherton and Smith, 2012; Shamim et al., 2018).
Dietary protein consumption is critical for providing the substrates responsible for facilitating skeletal muscle repair and regeneration (Shamim et al., 2018). Amino acids from dietary protein consumption have previously been demonstrated to promote myotube formation in vitro and increased Myod and Myogenin expression in rat skeletal muscle (Dai et al., 2015). Similarly, the leucine metabolite β-hydroxy-β-methyl butyrate (HMB) has been demonstrated to enhance proliferation, differentiation, and accelerate fusion in primary myoblasts (Kornasio et al., 2009) and increase MuSC proliferation in neonatal pigs (Kao et al., 2016). These effects may be due to leucine’s role in stimulating mammalian target of rapamycin (mTOR) activity (Sancak et al., 2008). mTOR has long been known for its key role in diverse cellular processes including cell growth, differentiation, and protein synthesis (Laplante and Sabatini, 2012). As such, mTOR is essential for MuSC function and skeletal muscle regeneration through its role in regulating the expression of myogenic genes (Zhang et al., 2015). Rodgers et al. (2014) have previously demonstrated that mTORC1 activity is necessary for MuSCs to transition during quiescence to an alert phase to enhance their regenerative capacity. Additionally, leucine can promote myoblast proliferation and differentiation in vitro through an mTORC1-MyoD cascade (Dai et al., 2015). Other essential amino acids (EAA’s) such as methionine has also been identified as a regulator of cell proliferation (Walvekar et al., 2018). Similarly, glutamine is the second most consumed nutrient apart from glucose during the proliferation phase of C2C12 myoblasts (Hosios et al., 2016), suggesting an important role in cell proliferation and its deprivation can lead to an incomplete cell cycle (Gaglio et al., 2009). Thus, in vitro and preclinical evidence suggests that dietary amino acids may play a direct role in regulating MuSC fate.
The Effects of Resistance Exercise and Training With Dietary Protein Manipulation on MuSCs
Responses to Acute Resistance Exercise
A large body of evidence has detailed the response of MuSCs after acute resistance exercise in untrained adults. These studies have been excellently reviewed elsewhere (Kadi et al., 2005; Snijders et al., 2015; Murach et al., 2021; Roman and Muñoz-Cánoves, 2022). Resistance exercise is the primary mode of exercise used to elicit positive changes in muscle mass (Schoenfeld et al., 2015) and is composed of contracting the muscles against an external resistance. In general, a single-bout of resistance exercise induces MuSC activation as early as 4-h post-exercise (McKay et al., 2009), with peak activation occurring at 72-h (Bellamy et al., 2014). This has been confirmed in both young and older adults in which increases in MuSC activation were observed between 6 and 24-h post-exercise (Walker et al., 2012; Luk et al., 2019), although the MuSC response in older adults is delayed (Snijders et al., 2014b).
A few recent studies have examined the synergistic effects of protein consumption and acute resistance exercise on MuSC content and activation (Hulmi et al., 2008; Roberts et al., 2010; Farup et al., 2014a; Snijders et al., 2014a; Reidy PT. et al., 2017). Gene expression of the myogenic regulatory factors, Pax7 and MyoD, was similar in whole muscle homogenates between whey protein and placebo groups between 1–6 h after a single bout of resistance exercise in both young (Roberts et al., 2010) and older (Hulmi et al., 2008) adults, suggesting no added benefit of whey protein intake. However, with respect to MuSC content, whey protein intake significantly increased MuSC content in type II fibres 48-h post-unilateral knee extension exercises (Farup et al., 2014a). Type II fibres are commonly referred to as “fast twitch” fibre types and are characterized as having lower oxidative capacity, greater glycolytic capacity, and greater force producing capacity compared to “slow-twitch” type I fibres (Henriksson and Reitman, 1976). Basal concentrations of MuSC’s have been shown to vary between muscle fibre types in healthy and diseased populations (Verdijk et al., 2007; Verney et al., 2008; Verdijk et al., 2009; Snijders et al., 2011; McKay et al., 2012; Suetta et al., 2012; Verdijk et al., 2012; Bankolé et al., 2013; Snijders et al., 2014b) and respond differentially following exercise depending on the type of fibre on which the MuSC is located (McKay et al., 2012; Cermak et al., 2013; Joanisse et al., 2013; Snijders et al., 2014b; Fry et al., 2014). In older adults, ingestion of an EAA supplement resulted in a trend for more Pax7+MuSCs 24-h after acute resistance exercise compared to the placebo condition, indicating that there was a trend for an increase in MuSC content (Reidy PT. et al., 2017). Further, MyoD+MuSCs and Ki67+MuSCs were also significantly increased in the group receiving EAAs versus placebo at 24-h, both indicating that there was an increase in proliferative cells (Reidy PT. et al., 2017). Together, these data suggest that protein supplementation in the form of whey or EAAs augments the MuSC response to acute resistance exercise in both young and older males.
To examine the effects of reduced protein consumption on the MuSC response to acute resistance exercise, Snijders et al. (2014a) restricted dietary protein to 0.1 g/kg/d, which is below the recommended daily allowance (RDA) (0.8 g/kg/d), for 4-days prior to an acute bout of resistance exercise in young males. Interestingly, no differences in MuSC content following resistance exercise were observed in the protein restricted group compared to the group that consumed adequate amounts of protein (1.2 g/kg/d) (Snijders et al., 2014a). However, the proportion of MuSCs expressing myostatin, an inhibitor of myogenesis (Langley et al., 2002), remained low in the protein restricted group compared to the normal protein group. A prolonged reduction in Myostatin+MuSCs may predict a prolonged MuSC response to training when protein is restricted; however, later time points were not examined in this study.
The general findings of the acute exercise studies suggest that protein intake in the form of whey or EAA augments the MuSC response to resistance exercise in both young and older males. Conversely, protein restriction does not impair the MuSC response to training and may prolong the response; however, future studies with longer protein restriction and later post-exercise timepoints are necessary to confirm this speculation.
Response to Resistance Training
Most studies suggest that resistance training increases MuSC content, primarily in type II fibres. Verdijk et al. (2014), found that 12-weeks of lower-body resistance training significantly increased type II fibre CD56+MuSC content in older adults. Similarly, a 12-weeks lower-body training program found that NCAM+MuSC content increased robustly following resistance training in extreme responders (Petrella et al., 2008). In addition, 16-weeks of lower-body resistance training in males and females aged 20–35 years increased NCAM+MuSC content (Petrella et al., 2006). Similarly, another 16-week study demonstrated Pax7+MuSC content was significantly increased in both type I and II fibres in young males (Bellamy et al., 2014). The consensus is that chronic resistance training increases MuSC content in adults of all ages.
Several resistance training studies have examined the effects of whey protein on Pax7+MuSC content. Some studies have shown that protein supplementation during a resistance exercise intervention did not increase MuSC content from what was seen with exercise alone (Farup et al., 2014b; Reidy P. T. et al., 2017; Snijders et al., 2018). However, Mobley et al. (2017) observed an increase in MuSC number with protein supplementation compared to placebo post-training. These inconsistent findings could be explained by the amount of protein consumed by participants in the different studies. Participants in the study detecting differences in MuSC content (Mobley et al., 2017) consumed higher (∼1.8–2.0 g/kg/d) amounts of protein compared to placebo (∼1.3 g/kg/d). However, in all studies, the placebo groups were consuming adequate amounts of protein to support muscle hypertrophy ∼1.3 g/kg/d of protein.
A particularly novel study examined the role of protein supplementation on markers of muscle regeneration during bedrest and recovery (Brooks et al., 2010). Participants were confined to bedrest for 28-days followed by 14-days of active recovery and were randomized to receive 15 g of EAA without resistance training, EAA 3-h after resistance training or 5 min before resistance training (Brooks et al., 2010). During the 14-days of active recovery, all participants completed mild endurance training, while the participants originally assigned to the resistance training group during bedrest continued their resistance training program. Only the participants that consumed the EAA supplement 3-h after resistance exercise significantly increased myonuclei per fibre, while a significant reduction in myonuclei per fibre was detected in both the group that received EAAs and remained sedentary, and the group that received EAAs 5-min before exercise compared to baseline (Brooks et al., 2010). These results suggest that the timing of amino acid delivery around exercise may be a relevant factor in the MuSC and myonuclei response.
In summary, it appears as though protein supplementation augments the increase in MuSC content following resistance exercise training compared to control diets. The limited studies in this area appear to indicate the effect of protein supplementation is dependent on dose with higher amounts (>2xRDA) and protein restriction below the RDA both potentially augmenting the MuSC response. Interestingly, the single study examining the timing of protein supplementation suggests that consuming protein a few hours after resistance exercise may provide the optimal response, at least in the context of skeletal muscle recovery following bedrest.
The Effects of Endurance Exercise and Training With Dietary Protein Manipulation on MuSCs
Endurance exercise is the most commonly prescribed type of exercise and traditionally involves exercising at 65–70% of VO₂ peak for durations of 30–60 min (Potteiger et al., 2003). Endurance exercise has historically been considered to be non- or minimally hypertrophic (Konopka and Harber, 2014). As a result, the role of MuSCs in facilitating adaptations to endurance exercise was ignored for several years. However, recently it has become appreciated that MuSCs adapt to endurance exercise by increasing their function and potentially content and play a role in non-hypertrophic muscle adaptation to endurance exercise (Joanisse et al., 2013; Nederveen et al., 2015; Abreu et al., 2017; Joanisse et al., 2018). The synergistic effects of endurance exercise paired with protein consumption are not well studied in general, and even less so in the context of MuSCs.
With respect to acute endurance exercise, Rowlands et al. (2016), examined the acute effects of different doses of protein and leucine compared to placebo on myogenic transcripts following intense endurance exercise composed of 100-min of cycling at 70–90% Wmax. In the protein/leucine supplemented condition the regenerative transcriptome was significantly upregulated compared to placebo with no difference between protein/leucine doses (Rowlands et al., 2016). With respect to endurance training, (McKenzie et al., 2016) did not find any additional beneficial effects of protein supplementation compared to placebo on MuSC content following an intervention that included 3-h of cycling for 20-days. Together, initial evidence suggests that protein supplementation augments the early myogenic response to a novel endurance exercise stimulus but does not enhance the MuSC response to endurance training.
The Effects of Dietary Protein Manipulation and Muscle Disuse on MuSCs
Skeletal muscle atrophy can occur following a period of muscle disuse, as a consequence of disease, or in conjunction with aging (McKenna and Fry, 2017). Perturbations in MuSC activity and quantity can exacerbate skeletal muscle atrophy in various conditions (McKenna and Fry, 2017), which may be improved by protein consumption. Muyskens and colleagues (2019) demonstrated that in patients undergoing total knee arthroplasty, those who consumed 20 g of EAAs twice-daily for 7-days prior and 6 weeks following surgery had higher MuSC content on the day of surgery compared with a group consuming a placebo. Further, the group that consumed EAAs had higher Myogenin expression, a marker of later stage myogenesis at 1-week post-surgery, while the placebo group had higher Myod expression, a marker of early myogenic commitment (Ganassi et al., 2020), suggesting EAA supplementation may accelerate myogenesis (Muyskens et al., 2019). When resistance training was combined with whey protein supplementation in patients on dialysis, Molsted et al. (2015) found that the fibre-type specific increase in MuSC content was not augmented by protein supplementation compared to placebo. Lastly, in the context of aging, HMB supplementation has been shown to improve muscle recovery in rats with sarcopenia, in part by increasing MuSC proliferation (Alway et al., 2013). While in a human study, Dirks and colleagues (2017) found no additional benefits of protein supplementation compared to placebo in increasing MuSC content following resistance training in frail elderly adults. As such, protein supplementation does not appear to provide added benefits to resistance exercise in the limited research using clinical populations; however, EAAs may enhance the myogenic response following surgery.
The Effects of Resistance Exercise and Training With Dietary Protein Manipulation on FAPs
MuSC’s contribution to muscle development, maintenance, and regeneration is regulated by a variety of cells that reside in the muscle niche. Among these cells, FAPs are an essential component of the MuSC niche, providing trophic factors that modulate MuSC activation and differentiation (Joe et al., 2010; Uezumi et al., 2010; Lukjanenko et al., 2019; Wosczyna et al., 2019). FAPs are identified by different transmembrane markers such as platelet-derived growth factor receptor alpha (PDGFRα), stem cell antigen-1 (Sca-1) in mice and PDGFRα, cluster of differentiation 90 (CD90) in humans, along with CD201, CD166, CD105, CD73 and CD15 (Joe et al., 2010; Uezumi et al., 2014a; Uezumi et al., 2014b; Arrighi et al., 2015; Uezumi et al., 2016; Kasai et al., 2017; Farup et al., 2020; Goloviznina et al., 2020; Contreras et al., 2021; Farup et al., 2021). These skeletal muscle-resident progenitors reside in the interstitial space between myofibers and sense mechanical/contractile forces (De Lisio et al., 2014). FAPs play an essential role in skeletal muscle maintenance and regeneration (Wosczyna et al., 2019; Uezumi et al., 2021); however, during aging FAPs enhance fibrotic differentiation at the expense of adipogenic differentiation (Lukjanenko et al., 2019) and many muscle diseases are characterized by enhance fibrotic differentiation of FAPs (Contreras et al., 2021; Giuliani et al., 2021). This dual role observed in FAPs during health and disease is highly determined by the muscle niche (Theret et al., 2021), of which amino acids are a crucial component, as well as their metabolism (Nguyen et al., 2019; Collao et al., 2020).
The mechanisms responsible for how exercise and protein consumption regulate FAP function is an area that warrants further investigation. In this context, Farup et al. (2015), demonstrated that 12 weeks of resistance training in humans increased of the number of proliferating PDGFRα+ and CD90+ FAPs, with no additional effect of whey protein supplementation. Interestingly, an acute muscle-damaging eccentric contraction protocol in humans showed a decrease in pericyte number, only in the protein supplemented group (De Lisio et al., 2015). Pericytes are perivascular cells in the muscle interstitium that envelop and form connections with adjacent capillary endothelial cells and line the skeletal muscle vasculature (Bergers and Song, 2005). They have some phenotypic and functional overlap with FAPs in their role in muscle regeneration and MuSC regulation (Joe et al., 2010, Birbrair et al., 2013). A recent paper by Liu et al. (2022) demonstrated transcriptomic changes occur after exercise training in old mice. Their single-cell RNA sequencing analysis indicated an increase in Igf (the gene for insulin growth factor) expression in FAPs from aged and exercise trained mice. IGF signalling is known to stimulate mTOR-Akt signalling to mediate skeletal muscle hypertrophy by promoting myogenesis and protein synthesis (Yoon, 2017, Bodine et al., 2001). These results provide a potential mechanism whereby paracrine factors, produced by FAPs could act synergistically with dietary protein to promote MPS and MuSC activation. The relationship between exercise, protein supplementation and FAP function, and the mechanisms responsible for exercise induced FAP regulation and how changes in circulating amino acid availability could impact the crosstalk between all the cells in the muscle niche, is still poorly understood.
Perspectives and Future Directions
While a large body of literature has been dedicated to delineating the effects of protein supplementation on myofiber adaptations and the protein synthetic response to increased/decreased use and disease; the effects of protein and exercise on skeletal muscle cell populations, including MuSCs and FAPs have been relatively understudied. The few recent studies in this field have provided initial evidence to suggest that protein supplementation enhances the MuSC response to exercise training and that this effect may be dependent upon protein dose and timing of protein consumption relative to exercise (Figure 1). Conversely, in the context of disease, protein supplementation does not appear to provide any additional benefit to the resistance exercise induced MuSC response; however, there is a paucity of work in this area. Further, protein does not appear to enhance the FAP response to training. Given the paucity of work, the occasionally equivocal findings, and the potential for clinical application, more work is needed in this area. As with most studies in exercise physiology and skeletal muscle biology, sex-based differences should receive increased attention as most studies have exclusively or predominantly included only male participants. Further, standardized controls that are isocaloric when examining the effects of protein, or isocaloric and isonitrogenous when examining the effects of different protein sources/timing of protein ingestion should be included. The apparent discrepant response observed with respect to protein dose, with augmentation of the satellite cell response at high and low doses of protein, but no effect of moderate protein doses, requires further attention. It is interesting to speculate that interactions between protein synthesis and MuSC-mediated responses to exercise may be regulated to a certain extent by amino acid availability with sufficient amino acid availability required to maximize the protein synthetic response, while excess amino acids used to enhance the MuSC response and limited amino acids requiring an augmented MuSC response to compensate for incomplete activation of protein synthesis. Lastly, at the molecular level, determining the extent to which amino acids are directly sensed and taken up by MuSCs and other skeletal muscle cell populations via amino acid transporters could provide valuable mechanistic insights into the regulation of muscle stem/progenitor cell fate by amino acids. The field of nutritional regulation of skeletal muscle stem/progenitor cell fate is emerging alongside studies examining the effects of exercise on these same populations. Continued work examining the interaction of both diet and exercise on muscle stem/progenitor cell content and fate is expected to allow for novel and optimized therapeutic interventions for augmenting muscle function and maintaining muscle health in disease.
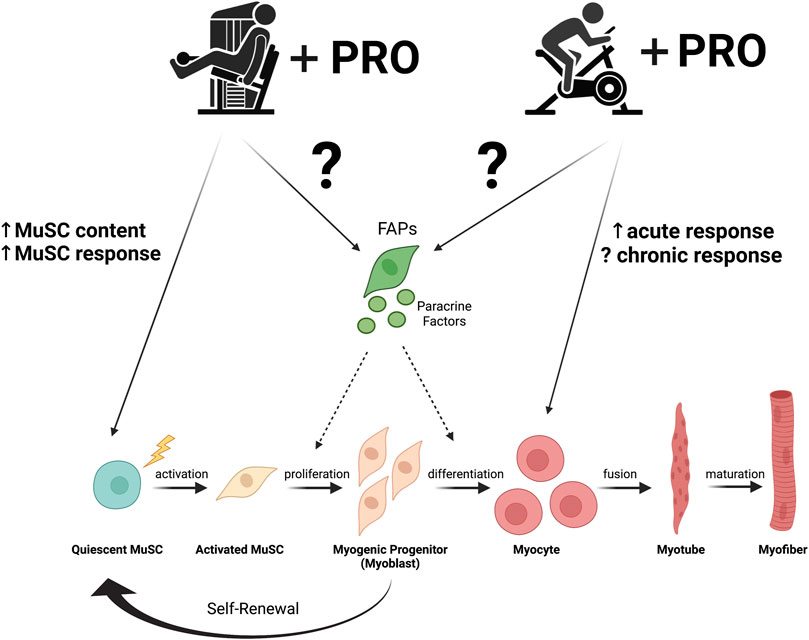
FIGURE 1. The effects of resistance and endurance exercise plus protein consumption (PRO) on muscle stem cells (MuSC) and fibro-adipogenic progenitors (FAPs). In response to skeletal muscle damage and signals from cells in their niche including FAPs, MuSCs exit from quiescence, proliferate, and differentiate to facilitate muscle repair and adaptation. Generally, findings of both acute and chronic resistance exercise suggest that protein intake augments the MuSC response in both young and older adults. Endurance exercise however is less understood. Acute exercise combined with protein consumption appears to upregulate myogenic transcripts with no effects found in chronic endurance exercise. The effects of exercise, both resistance and endurance, with protein consumption on FAP function is poorly understood and requires further investigation. Figure created with BioRender.com.
Author Contributions
MD and KB conceived the topic for review. MD, KB, EB, and NC wrote and edited the manuscript. All authors approved final version of the manuscript.
Funding
This work was supported by grants from the National Sciences and Engineering Research Council (NSERC), and the Egg Farmers of Canada (EFC) to MD. EB was supported by a Canadian Institutes of Health Research (CIHR), CGS-M award. NC is supported by The Chilean National Agency for Research and Development (ANID) and the uOttawa/CHEO Research Institute’s Doctoral Fellowship for Advancement of Biological Perspectives for Exercise Interventions Across the Lifespan. EFC funded the work but had no role in the decision to publish.
Conflict of Interest
The authors declare that the research was conducted in the absence of any commercial or financial relationships that could be construed as a potential conflict of interest.
Publisher’s Note
All claims expressed in this article are solely those of the authors and do not necessarily represent those of their affiliated organizations, or those of the publisher, the editors and the reviewers. Any product that may be evaluated in this article, or claim that may be made by its manufacturer, is not guaranteed or endorsed by the publisher.
References
Abreu P., Mendes S. V. D., Ceccatto V. M., Hirabara S. M. (2017). Satellite Cell Activation Induced by Aerobic Muscle Adaptation in Response to Endurance Exercise in Humans and Rodents. Life Sci. 170, 33–40. doi:10.1016/j.lfs.2016.11.016
Agergaard J., Bülow J., Jensen J. K., Reitelseder S., Drummond M. J., Schjerling P., et al. (2017). Light-Load Resistance Exercise Increases Muscle Protein Synthesis and Hypertrophy Signaling in Elderly Men. Am. J. Physiology-Endocrinology Metabolism 312, E326–E338. doi:10.1152/ajpendo.00164.2016
McKenzie A. I., D'Lugos A. C., Saunders M. J., Gworek K. D., Luden N. D. (2016). Fiber Type-Specific Satellite Cell Content in Cyclists Following Heavy Training with Carbohydrate and Carbohydrate-Protein Supplementation. Front. Physiol. 16 (7), 550.
Alway S. E., Pereira S. L., Edens N. K., Hao Y., Bennett B. T. (2013). β-Hydroxy-β-methylbutyrate (HMB) Enhances the Proliferation of Satellite Cells in Fast Muscles of Aged Rats during Recovery from Disuse Atrophy. Exp. Gerontol. 48, 973–984. doi:10.1016/j.exger.2013.06.005
Arrighi N., Moratal C., Clément N., Giorgetti-Peraldi S., Peraldi P., Loubat A., et al. (2015). Characterization of Adipocytes Derived from Fibro/Adipogenic Progenitors Resident in Human Skeletal Muscle. Cell Death Dis. 6, E1733. doi:10.1038/cddis.2015.79
Atherton P. J., Smith K. (2012). Muscle Protein Synthesis in Response to Nutrition and Exercise. J. Physiol. 590, 1049–1057. doi:10.1113/jphysiol.2011.225003
Baldwin K. M., Haddad F. (2002). Skeletal Muscle Plasticity. Am. J. Phys. Med. Rehabilitation 81, S40–S51. doi:10.1097/00002060-200211001-00006
Bankolé L. C., Feasson L., Ponsot E., Kadi F. (2013). Fibre Type-specific Satellite Cell Content in Two Models of Muscle Disease. Histopathology 63, 826–832. doi:10.1111/his.12231
Beaudry K. M., Devries M. C. (2019). Nutritional Strategies to Combat Type 2 Diabetes in Aging Adults: The Importance of Protein. Front. Nutr. 6, 138. doi:10.3389/fnut.2019.00138
Bellamy L. M., Joanisse S., Grubb A., Mitchell C. J., Mckay B. R., Phillips S. M., et al. (2014). The Acute Satellite Cell Response and Skeletal Muscle Hypertrophy Following Resistance Training. Plos One 9, E109739. doi:10.1371/journal.pone.0109739
Bergers G., Song S. (2005). The Role of Pericytes in Blood-Vessel Formation and Maintenance. Neuro Oncol. 7, 452–464. doi:10.1215/s1152851705000232
Biolo G., Maggi S. P., Williams B. D., Tipton K. D., Wolfe R. R. (1995). Increased Rates of Muscle Protein Turnover and Amino Acid Transport after Resistance Exercise in Humans. Am. J. Physiology-Endocrinology Metabolism 268, E514–E520. doi:10.1152/ajpendo.1995.268.3.e514
Birbrair A., Zhang T., Wang Z.-M., Messi M. L., Enikolopov G. N., Mintz A., et al. (2013). Role of Pericytes in Skeletal Muscle Regeneration and Fat Accumulation. Stem Cells Dev. 22, 2298–2314. doi:10.1089/scd.2012.0647
Bischoff R. (1990). Cell Cycle Commitment of Rat Muscle Satellite Cells. J. Cell Biol. 111, 201–207. doi:10.1083/jcb.111.1.201
Bodine S. C., Stitt T. N., Gonzalez M., Kline W. O., Stover G. L., Bauerlein R., et al. (2001). Akt/Mtor Pathway Is A Crucial Regulator of Skeletal Muscle Hypertrophy and Can Prevent Muscle Atrophy In Vivo. Nat. Cell Biol. 3, 1014–1019. doi:10.1038/ncb1101-1014
Bridge A., Brown J., Snider H., Nasato M., Ward W. E., Roy B. D., et al. (2019). Greek Yogurt and 12 Weeks of Exercise Training on Strength, Muscle Thickness and Body Composition in Lean, Untrained, University-Aged Males. Front. Nutr. 6, 55. doi:10.3389/fnut.2019.00055
Brooks N. E., Cadena S. M., Vannier E., Cloutier G., Carambula S., Myburgh K. H., et al. (2010). Effects of Resistance Exercise Combined with Essential Amino Acid Supplementation and Energy Deficit on Markers of Skeletal Muscle Atrophy and Regeneration during Bed Rest and Active Recovery. Muscle Nerve 42, 927–935. doi:10.1002/mus.21780
Burd N. A., Beals J. W., Martinez I. G., Salvador A. F., Skinner S. K. (2019). Food-First Approach to Enhance the Regulation of Post-Exercise Skeletal Muscle Protein Synthesis and Remodeling. Sports Med. 49, 59–68. doi:10.1007/s40279-018-1009-y
Burd N. A., De Lisio M. (2017). Skeletal Muscle Remodeling: Interconnections between Stem Cells and Protein Turnover. Exerc Sport Sci. Rev. 45, 187–191. doi:10.1249/jes.0000000000000117
Burd N. A., Holwerda A. M., Selby K. C., West D. W. D., Staples A. W., Cain N. E., et al. (2010). Resistance Exercise Volume Affects Myofibrillar Protein Synthesis and Anabolic Signalling Molecule Phosphorylation in Young Men. J. Physiol. 588, 3119–3130. doi:10.1113/jphysiol.2010.192856
Burd N. A., West D. W. D., Moore D. R., Atherton P. J., Staples A. W., Prior T., et al. (2011). Enhanced Amino Acid Sensitivity of Myofibrillar Protein Synthesis Persists for up to 24 H after Resistance Exercise in Young Men. J. Nutr. 141, 568–573. doi:10.3945/jn.110.135038
Cermak N. M., Snijders T., Mckay B. R., Parise G., Verdijk L. B., Tarnopolsky M. A., et al. (2013). Eccentric Exercise Increases Satellite Cell Content in Type Ii Muscle Fibers. Med. Sci. Sports Exerc 45, 230–237. doi:10.1249/mss.0b013e318272cf47
Churchward-Venne T. A., Burd N. A., Phillips S. M. (2012). Nutritional Regulation of Muscle Protein Synthesis with Resistance Exercise: Strategies to Enhance Anabolism. Nutr. Metab. (Lond) 9, 40. doi:10.1186/1743-7075-9-40
Churchward-Venne T. A., Pinckaers P. J. M., Smeets J. S. J., Betz M. W., Senden J. M., Goessens J. P. B., et al. (2020). Dose-Response Effects of Dietary Protein on Muscle Protein Synthesis during Recovery from Endurance Exercise in Young Men: A Double-Blind Randomized Trial. Am. J. Clin. Nutr. 112, 303–317. doi:10.1093/ajcn/nqaa073
Collao N., Farup J., De Lisio M. (2020). Role of Metabolic Stress and Exercise in Regulating Fibro/Adipogenic Progenitors. Front. Cell Dev. Biol. 8, 9. doi:10.3389/fcell.2020.00009
Collins C. A., Olsen I., Zammit P. S., Heslop L., Petrie A., Partridge T. A., et al. (2005). Stem Cell Function, Self-Renewal, and Behavioral Heterogeneity of Cells from the Adult Muscle Satellite Cell Niche. Cell 122, 289–301. doi:10.1016/j.cell.2005.05.010
Contreras O., Rebolledo D. L., Oyarzún J. E., Olguín H. C., Brandan E. (2016). Connective Tissue Cells Expressing Fibro/Adipogenic Progenitor Markers Increase under Chronic Damage: Relevance in Fibroblast-Myofibroblast Differentiation and Skeletal Muscle Fibrosis. Cell Tissue Res. 364, 647–660. doi:10.1007/s00441-015-2343-0
Contreras O., Rossi F. M. V., Theret M. (2021). Origins, Potency, and Heterogeneity of Skeletal Muscle Fibro-Adipogenic Progenitors-Time for New Definitions. Skelet. Muscle 11, 16. doi:10.1186/s13395-021-00265-6
Dai J.-M., Yu M.-X., Shen Z.-Y., Guo C.-Y., Zhuang S.-Q., Qiu X.-S. (2015). Leucine Promotes Proliferation and Differentiation of Primary Preterm Rat Satellite Cells in Part through Mtorc1 Signaling Pathway. Nutrients 7, 3387–3400. doi:10.3390/nu7053387
De Lisio M., Farup J., Sukiennik R. A., Clevenger N., Nallabelli J., Nelson B., et al. (2015). The Acute Response of Pericytes to Muscle-Damaging Eccentric Contraction and Protein Supplementation in Human Skeletal Muscle. J. Appl. Physiology 119, 900–907. doi:10.1152/japplphysiol.01112.2014
De Lisio M., Jensen T., Sukiennik R. A., Huntsman H. D., Boppart M. D. (2014). Substrate and Strain Alter the Muscle-Derived Mesenchymal Stem Cell Secretome to Promote Myogenesis. Stem Cell Res. Ther. 5, 74. doi:10.1186/scrt463
Di Donato D. M., West D. W. D., Churchward-Venne T. A., Breen L., Baker S. K., Phillips S. M. (2014). Influence of Aerobic Exercise Intensity on Myofibrillar and Mitochondrial Protein Synthesis in Young Men during Early and Late Postexercise Recovery. Am. J. Physiology-Endocrinology Metabolism 306, E1025–E1032. doi:10.1152/ajpendo.00487.2013
Dirks M. L., Tieland M., Verdijk L. B., Losen M., Nilwik R., Mensink M. (2017). Protein Supplementation Augments Muscle Fiber Hypertrophy but Does Not Modulate Satellite Cell Content During Prolonged Resistance-Type Exercise Training in Frail Elderly. J. Am. Med. Dir. Assoc. 18 (7), 608–615.
Dong J., Dong Y., Chen Z., Mitch W. E., Zhang L. (2017). The Pathway to Muscle Fibrosis Depends on Myostatin Stimulating the Differentiation of Fibro/Adipogenic Progenitor Cells in Chronic Kidney Disease. Kidney Int. 91, 119–128. doi:10.1016/j.kint.2016.07.029
Dumont N. A., Bentzinger C. F., Sincennes M. C., Rudnicki M. A. (2015). Satellite Cells and Skeletal Muscle Regeneration. Compr. Physiol. 5, 1027–1059. doi:10.1002/cphy.c140068
Fanzani A., Conraads V. M., Penna F., Martinet W. (2012). Molecular and Cellular Mechanisms of Skeletal Muscle Atrophy: An Update. J. Cachexia Sarcopenia Muscle 3, 163–179. doi:10.1007/s13539-012-0074-6
Farup J., De Lisio M., Rahbek S. K., Bjerre J., Vendelbo M. H., Boppart M. D., et al. (2015). Pericyte Response to Contraction Mode-specific Resistance Exercise Training in Human Skeletal Muscle. J. Appl. Physiology 119, 1053–1063. doi:10.1152/japplphysiol.01108.2014
Farup J., Just J., De Paoli F., Lin L., Jensen J. B., Billeskov T., et al. (2021). Human Skeletal Muscle Cd90+ Fibro-Adipogenic Progenitors Are Associated with Muscle Degeneration in Type 2 Diabetic Patients. Cell Metab. 33, 2201–2214. E10. doi:10.1016/j.cmet.2021.10.001
Farup J., Just J., De Paoli F., Lin L., Jensen J. B., Billeskov T., et al. (2020). Human Skeletal Muscle Cd90+ Fibro-Adipogenic Progenitors Are Associated with Muscle Degeneration in Type 2 Diabetic Patients. Biorxiv. doi:10.1242/prelights.24354
Farup J., Rahbek S. K., Knudsen I. S., De Paoli F., Mackey A. L., Vissing K. (2014a). Whey Protein Supplementation Accelerates Satellite Cell Proliferation during Recovery from Eccentric Exercise. Amino Acids 46, 2503–2516. doi:10.1007/s00726-014-1810-3
Farup J., Rahbek S. K., Riis S., Vendelbo M. H., Paoli F. d., Vissing K. (2014b). Influence of Exercise Contraction Mode and Protein Supplementation on Human Skeletal Muscle Satellite Cell Content and Muscle Fiber Growth. J. Appl. Physiology 117, 898–909. doi:10.1152/japplphysiol.00261.2014
Feige P., Brun C. E., Ritso M., Rudnicki M. A. (2018). Orienting Muscle Stem Cells for Regeneration in Homeostasis, Aging, and Disease. Cell Stem Cell 23, 653–664. doi:10.1016/j.stem.2018.10.006
Fry C. S., Noehren B., Mula J., Ubele M. F., Westgate P. M., Kern P. A., et al. (2014). Fibre Type-specific Satellite Cell Response to Aerobic Training in Sedentary Adults. J. Physiol. 592, 2625–2635. doi:10.1113/jphysiol.2014.271288
Gaglio D., Soldati C., Vanoni M., Alberghina L., Chiaradonna F. (2009). Glutamine Deprivation Induces Abortive S-phase Rescued by Deoxyribonucleotides in K-Ras Transformed Fibroblasts. Plos One 4, E4715. doi:10.1371/journal.pone.0004715
Ganassi M., Badodi S., Wanders K., Zammit P. S., Hughes S. M. (2020). Myogenin Is an Essential Regulator of Adult Myofibre Growth and Muscle Stem Cell Homeostasis. Elife 9. doi:10.7554/eLife.60445
Giuliani G., Rosina M., Reggio A. (2021). Signaling Pathways Regulating the Fate of Fibro/Adipogenic Progenitors (Faps) in Skeletal Muscle Regeneration and Disease. Febs J. doi:10.1111/febs.16080
Glynn E. L., Fry C. S., Drummond M. J., Dreyer H. C., Dhanani S., Volpi E., et al. (2010). Muscle Protein Breakdown Has A Minor Role in the Protein Anabolic Response to Essential Amino Acid and Carbohydrate Intake Following Resistance Exercise. Am. J. Physiology-Regulatory, Integr. Comp. Physiology 299, R533–R540. doi:10.1152/ajpregu.00077.2010
Goloviznina N. A., Xie N., Dandapat A., Iaizzo P. A., Kyba M. (2020). Prospective Isolation of Human Fibroadipogenic Progenitors with Cd73. Heliyon 6, E04503. doi:10.1016/j.heliyon.2020.e04503
Gonzalez D., Contreras O., Rebolledo D. L., Espinoza J. P., Van Zundert B., Brandan E. (2017). ALS Skeletal Muscle Shows Enhanced TGF-β Signaling, Fibrosis and Induction of Fibro/adipogenic Progenitor Markers. Plos One 12, E0177649. doi:10.1371/journal.pone.0177649
Harber M. P., Konopka A. R., Jemiolo B., Trappe S. W., Trappe T. A., Reidy P. T. (2010). Muscle Protein Synthesis and Gene Expression during Recovery from Aerobic Exercise in the Fasted and Fed States. Am. J. Physiology-Regulatory, Integr. Comp. Physiology 299, R1254–R1262. doi:10.1152/ajpregu.00348.2010
Haun C. T., Vann C. G., Roberts B. M., Vigotsky A. D., Schoenfeld B. J., Roberts M. D. (2019). A Critical Evaluation of the Biological Construct Skeletal Muscle Hypertrophy: Size Matters but So Does the Measurement. Front. Physiol. 10, 247. doi:10.3389/fphys.2019.00247
Henriksson J., Reitman J. S. (1976). Quantitative Measures of Enzyme Activities in Type I and Type Ii Muscle Fibres of Man after Training. Acta Physiol. Scand. 97, 392–397. doi:10.1111/j.1748-1716.1976.tb10279.x
Hosios A. M., Hecht V. C., Danai L. V., Johnson M. O., Rathmell J. C., Steinhauser M. L., et al. (2016). Amino Acids rather Than Glucose Account for the Majority of Cell Mass in Proliferating Mammalian Cells. Dev. Cell 36, 540–549. doi:10.1016/j.devcel.2016.02.012
Hulmi J. J., Kovanen V., Lisko I., Selänne H., Mero A. A. (2008). The Effects of Whey Protein on Myostatin and Cell Cycle-Related Gene Expression Responses to A Single Heavy Resistance Exercise Bout in Trained Older Men. Eur. J. Appl. Physiol. 102, 205–213. doi:10.1007/s00421-007-0579-4
Janssen I., Heymsfield S. B., Wang Z., Ross R. (2000). Skeletal Muscle Mass and Distribution in 468 Men and Women Aged 18-88 Yr. J. Appl. Physiology 89, 81–88. doi:10.1152/jappl.2000.89.1.81
Joanisse S., Gillen J. B., Bellamy L. M., Mckay B. R., Tarnopolsky M. A., Gibala M. J., et al. (2013). Evidence for the Contribution of Muscle Stem Cells to Nonhypertrophic Skeletal Muscle Remodeling in Humans. FASEB J. 27, 4596–4605. doi:10.1096/fj.13-229799
Joanisse S., Snijders T., Nederveen J. P., Parise G. (2018). The Impact of Aerobic Exercise on the Muscle Stem Cell Response. Exerc Sport Sci. Rev. 46, 180–187. doi:10.1249/jes.0000000000000153
Joe A. W. B., Yi L., Natarajan A., Le Grand F., So L., Wang J., et al. (2010). Muscle Injury Activates Resident Fibro/Adipogenic Progenitors that Facilitate Myogenesis. Nat. Cell Biol. 12, 153–163. doi:10.1038/ncb2015
Kadi F., Charifi N., Denis C., Lexell J., Andersen J. L., Schjerling P., et al. (2005). The Behaviour of Satellite Cells in Response to Exercise: What Have We Learned from Human Studies? Pflugers Arch. - Eur. J. Physiol. 451, 319–327. doi:10.1007/s00424-005-1406-6
Kao M., Columbus D. A., Suryawan A., Steinhoff-Wagner J., Hernandez-Garcia A., Nguyen H. V., et al. (2016). Enteral β-hydroxy-β-methylbutyrate Supplementation Increases Protein Synthesis in Skeletal Muscle of Neonatal Pigs. Am. J. Physiology-Endocrinology Metabolism 310, E1072–E1084. doi:10.1152/ajpendo.00520.2015
Kasai T., Nakatani M., Ishiguro N., Ohno K., Yamamoto N., Morita M., et al. (2017). Promethazine Hydrochloride Inhibits Ectopic Fat Cell Formation in Skeletal Muscle. Am. J. Pathology 187, 2627–2634. doi:10.1016/j.ajpath.2017.08.008
Katz B. (1961). The Termination of the Afferent Nerve Fibre in the Muscle Spindle of the Frog. Philosophical Trans. Of R. Soc. Of Lond. Ser. B, Biol. Sci. 243, 221–240.
Knudsen K. A., Horwitz A. F. (1977). Tandem Events in Myoblast Fusion. Dev. Biol. 58, 328–338. doi:10.1016/0012-1606(77)90095-1
Konopka A. R., Harber M. P. (2014). Skeletal Muscle Hypertrophy after Aerobic Exercise Training. Exerc Sport Sci. Rev. 42, 53–61. doi:10.1249/jes.0000000000000007
Kornasio R., Riederer I., Butler-Browne G., Mouly V., Uni Z., Halevy O. (2009). β-hydroxy-β-methylbutyrate (HMB) Stimulates Myogenic Cell Proliferation, Differentiation and Survival via the MAPK/ERK and PI3K/Akt Pathways. Biochimica Biophysica Acta (BBA) - Mol. Cell Res. 1793, 755–763. doi:10.1016/j.bbamcr.2008.12.017
Langley B., Thomas M., Bishop A., Sharma M., Gilmour S., Kambadur R. (2002). Myostatin Inhibits Myoblast Differentiation by Down-Regulating Myod Expression. J. Biol. Chem. 277, 49831–49840. doi:10.1074/jbc.m204291200
Laplante M., Sabatini D. M. (2012). Mtor Signaling in Growth Control and Disease. Cell 149, 274–293. doi:10.1016/j.cell.2012.03.017
Liu L., Buckley M. T., Reyes J. M., Kim S., Tian L., Wang M., et al. (2022). Exercise Reprograms the Inflammatory Landscape of Multiple Stem Cell Compartments during Mammalian Aging. Biorxiv. doi:10.1101/2022.01.12.475145
Luk H.-Y., Levitt D. E., Boyett J. C., Rojas S., Flader S. M., Mcfarlin B. K., et al. (2019). Resistance Exercise-Induced Hormonal Response Promotes Satellite Cell Proliferation in Untrained Men but Not in Women. Am. J. Physiology-Endocrinology Metabolism 317, E421–E432. doi:10.1152/ajpendo.00473.2018
Lukjanenko L., Karaz S., Stuelsatz P., Gurriaran-Rodriguez U., Michaud J., Dammone G., et al. (2019). Aging Disrupts Muscle Stem Cell Function by Impairing Matricellular Wisp1 Secretion from Fibro-Adipogenic Progenitors. Cell Stem Cell 24, 433–446. E7. doi:10.1016/j.stem.2018.12.014
Madaro L., Passafaro M., Sala D., Etxaniz U., Lugarini F., Proietti D., et al. (2018). Denervation-Activated Stat3-Il-6 Signalling in Fibro-Adipogenic Progenitors Promotes Myofibres Atrophy and Fibrosis. Nat. Cell Biol. 20, 917–927. doi:10.1038/s41556-018-0151-y
Mauro A. (1961). Satellite Cell of Skeletal Muscle Fibers. J. Biophys. Biochem. Cytol. 9, 493–495. doi:10.1083/jcb.9.2.493
Mckay B. R., De Lisio M., Johnston A. P., O'reilly C. E., Phillips S. M., Tarnopolsky M. A., et al. (2009). Association of Interleukin-6 Signalling with the Muscle Stem Cell Response Following Muscle-Lengthening Contractions in Humans. Plos One 4, E6027. doi:10.1371/journal.pone.0006027
Mckay B. R., Ogborn D. I., Bellamy L. M., Tarnopolsky M. A., Parise G. (2012). Myostatin Is Associated with Age-Related Human Muscle Stem Cell Dysfunction. FASEB J. 26, 2509–2521. doi:10.1096/fj.11-198663
Mckenna C. F., Fry C. S. (2017). Altered Satellite Cell Dynamics Accompany Skeletal Muscle Atrophy during Chronic Illness, Disuse, and Aging. Curr. Opin. Clin. Nutr. Of Metabolic Care 20, 447–452. doi:10.1097/mco.0000000000000409
Mcleod M., Breen L., Hamilton D. L., Philp A. (2016). Live Strong and Prosper: The Importance of Skeletal Muscle Strength for Healthy Ageing. Biogerontology 17, 497–510. doi:10.1007/s10522-015-9631-7
Mobley C. B., Haun C. T., Roberson P. A., Mumford P. W., Romero M. A., Kephart W. C., et al. (2017). Effects of Whey, Soy or Leucine Supplementation with 12 Weeks of Resistance Training on Strength, Body Composition, and Skeletal Muscle and Adipose Tissue Histological Attributes in College-Aged Males. Nutrients 9. doi:10.3390/nu9090972
Molsted S., Andersen J. L., Harrison A. P., Eidemak I., Mackey A. L. (2015). Fiber Type-specific Response of Skeletal Muscle Satellite Cells to High-Intensity Resistance Training in Dialysis Patients. Muscle Nerve 52, 736–745. doi:10.1002/mus.24633
Morton R. W., Mcglory C., Phillips S. M. (2015). Nutritional Interventions to Augment Resistance Training-Induced Skeletal Muscle Hypertrophy. Front. Physiol. 6, 245. doi:10.3389/fphys.2015.00245
Mozzetta C., Consalvi S., Saccone V., Tierney M., Diamantini A., Mitchell K. J., et al. (2013). Fibroadipogenic Progenitors Mediate the Ability of Hdac Inhibitors to Promote Regeneration in Dystrophic Muscles of Young, but Not Old Mdx Mice. Embo Mol. Med. 5, 626–639. doi:10.1002/emmm.201202096
Murach K. A., Fry C. S., Dupont-Versteegden E. E., Mccarthy J. J., Peterson C. A. (2021). Fusion and beyond: Satellite Cell Contributions to Loading-Induced Skeletal Muscle Adaptation. Faseb J. 35, E21893. doi:10.1096/fj.202101096R
Muyskens J. B., Foote D. M., Bigot N. J., Strycker L. A., Smolkowski K., Kirkpatrick T. K., et al. (2019). Cellular and Morphological Changes with Eaa Supplementation before and after Total Knee Arthroplasty. J. Appl. Physiology 127, 531–545. doi:10.1152/japplphysiol.00869.2018
Nederveen J. P., Joanisse S., Séguin C. M. L., Bell K. E., Baker S. K., Phillips S. M., et al. (2015). The Effect of Exercise Mode on the Acute Response of Satellite Cells in Old Men. Acta Physiol. 215, 177–190. doi:10.1111/apha.12601
Nguyen J. H., Chung J. D., Lynch G. S., Ryall J. G. (2019). The Microenvironment Is A Critical Regulator of Muscle Stem Cell Activation and Proliferation. Front. Cell Dev. Biol. 7, 254. doi:10.3389/fcell.2019.00254
Paddon-Jones D. (2006). Interplay of Stress and Physical Inactivity on Muscle Loss: Nutritional Countermeasures. J. Nutr. 136, 2123–2126. doi:10.1093/jn/136.8.2123
Paddon-Jones D., Sheffield-Moore M., Urban R. J., Sanford A. P., Aarsland A., Wolfe R. R., et al. (2004). Essential Amino Acid and Carbohydrate Supplementation Ameliorates Muscle Protein Loss in Humans during 28 Days Bedrest. J. Clin. Endocrinol. Metabolism 89, 4351–4358. doi:10.1210/jc.2003-032159
Park Y., Park H.-Y., Kim J., Hwang H., Jung Y., Kreider R., et al. (2019). Effects of Whey Protein Supplementation Prior to, and Following, Resistance Exercise on Body Composition and Training Responses: A Randomized Double-Blind Placebo-Controlled Study. Jenb 23, 34–44. doi:10.20463/jenb.2019.0015
Petrella J. K., Kim J.-s., Cross J. M., Kosek D. J., Bamman M. M. (2006). Efficacy of Myonuclear Addition May Explain Differential Myofiber Growth Among Resistance-Trained Young and Older Men and Women. Am. J. Physiology-Endocrinology Metabolism 291, E937–E946. doi:10.1152/ajpendo.00190.2006
Petrella J. K., Kim J.-s., Mayhew D. L., Cross J. M., Bamman M. M. (2008). Potent Myofiber Hypertrophy during Resistance Training in Humans Is Associated with Satellite Cell-Mediated Myonuclear Addition: A Cluster Analysis. J. Appl. Physiology 104, 1736–1742. doi:10.1152/japplphysiol.01215.2007
Phillips S. M. (2016). The Impact of Protein Quality on the Promotion of Resistance Exercise-Induced Changes in Muscle Mass. Nutr. Metab. (Lond) 13, 64. doi:10.1186/s12986-016-0124-8
Pikosky M. A., Gaine P. C., Martin W. F., Grabarz K. C., Ferrando A. A., Wolfe R. R., et al. (2006). Aerobic Exercise Training Increases Skeletal Muscle Protein Turnover in Healthy Adults at Rest. J. Of Nutr. 136, 379–383. doi:10.1093/jn/136.2.379
Potteiger J. A., Jacobsen D. J., Donnelly J. E., Hill J. O., Midwest Exercise T. (2003). Glucose and Insulin Responses Following 16 Months of Exercise Training in Overweight Adults: The Midwest Exercise Trial. Metabolism 52, 1175–1181. doi:10.1016/s0026-0495(03)00146-x
Reidy P. T., Fry C. S., Dickinson J. M., Drummond M. J., Rasmussen B. B. (2017a). Postexercise Essential Amino Acid Supplementation Amplifies Skeletal Muscle Satellite Cell Proliferation in Older Men 24 hours Postexercise. Physiol. Rep. 5. doi:10.14814/phy2.13269
Reidy P. T., Fry C. S., Igbinigie S., Deer R. R., Jennings K., Cope M. B., et al. (2017b). Protein Supplementation Does Not Affect Myogenic Adaptations to Resistance Training. Med. Sci. Sports Exerc 49, 1197–1208. doi:10.1249/mss.0000000000001224
Reitelseder S., Agergaard J., Doessing S., Helmark I. C., Lund P., Kristensen N. B., et al. (2011). Whey and Casein Labeled withl-[1-13C]leucine and Muscle Protein Synthesis: Effect of Resistance Exercise and Protein Ingestion. Am. J. Physiology-Endocrinology Metabolism 300, E231–E242. doi:10.1152/ajpendo.00513.2010
Rieu I., Balage M., Sornet C., Giraudet C., Pujos E., Grizard J., et al. (2006). Leucine Supplementation Improves Muscle Protein Synthesis in Elderly Men Independently of Hyperaminoacidaemia. J. Physiol. 575, 305–315. doi:10.1113/jphysiol.2006.110742
Roberts M. D., Dalbo V. J., Hassell S. E., Brown R., Kerksick C. M. (2010). Effects of Preexercise Feeding on Markers of Satellite Cell Activation. Med. Sci. Sports Exerc 42, 1861–1869. doi:10.1249/mss.0b013e3181da8a29
Rodgers J. T., King K. Y., Brett J. O., Cromie M. J., Charville G. W., Maguire K. K., et al. (2014). mTORC1 Controls the Adaptive Transition of Quiescent Stem Cells from G0 to GAlert. Nature 510, 393–396. doi:10.1038/nature13255
Roman W., Muñoz-Cánoves P. (2022). Muscle Is A Stage, and Cells and Factors Are Merely Players. Trends Cell Biol. doi:10.1016/j.tcb.2022.03.001
Rowlands D. S., Nelson A. R., Raymond F., Metairon S., Mansourian R., Clarke J., et al. (2016). Protein-Leucine Ingestion Activates A Regenerative Inflammo-Myogenic Transcriptome in Skeletal Muscle Following Intense Endurance Exercise. Physiol. Genomics 48, 21–32. doi:10.1152/physiolgenomics.00068.2015
Rudrappa S. S., Wilkinson D. J., Greenhaff P. L., Smith K., Idris I., Atherton P. J. (2016). Human Skeletal Muscle Disuse Atrophy: Effects on Muscle Protein Synthesis, Breakdown, and Insulin Resistance-A Qualitative Review. Front. Physiol. 7, 361. doi:10.3389/fphys.2016.00361
Sacco A., Doyonnas R., Kraft P., Vitorovic S., Blau H. M. (2008). Self-Renewal and Expansion of Single Transplanted Muscle Stem Cells. Nature 456, 502–506. doi:10.1038/nature07384
Sancak Y., Peterson T. R., Shaul Y. D., Lindquist R. A., Thoreen C. C., Bar-Peled L., et al. (2008). The Rag Gtpases Bind Raptor and Mediate Amino Acid Signaling to Mtorc1. Science 320, 1496–1501. doi:10.1126/science.1157535
Schoenfeld B. J., Peterson M. D., Ogborn D., Contreras B., Sonmez G. T. (2015). Effects of Low- vs. High-Load Resistance Training on Muscle Strength and Hypertrophy in Well-Trained Men. J. Strength Cond. Res. 29, 2954–2963. doi:10.1519/jsc.0000000000000958
Severinsen M. C. K., Pedersen B. K. (2020). Muscle-Organ Crosstalk: The Emerging Roles of Myokines. Endocr. Rev. 41, 594–609. doi:10.1210/endrev/bnaa016
Shamim B., Hawley J. A., Camera D. M. (2018). Protein Availability and Satellite Cell Dynamics in Skeletal Muscle. Sports Med. 48, 1329–1343. doi:10.1007/s40279-018-0883-7
Snijders T., Bell K. E., Nederveen J. P., Saddler N. I., Mazara N., Kumbhare D. A., et al. (2018). Ingestion of A Multi-Ingredient Supplement Does Not Alter Exercise-Induced Satellite Cell Responses in Older Men. J. Nutr. 148, 891–899. doi:10.1093/jn/nxy063
Snijders T., Nederveen J. P., Mckay B. R., Joanisse S., Verdijk L. B., Van Loon L. J. C., et al. (2015). Satellite Cells in Human Skeletal Muscle Plasticity. Front. Physiol. 6, 283. doi:10.3389/fphys.2015.00283
Snijders T., Verdijk L. B., Hansen D., Dendale P., Van Loon L. J. C. (2011). Continuous Endurance-type Exercise Training Does Not Modulate Satellite Cell Content in Obese Type 2 Diabetes Patients. Muscle Nerve 43, 393–401. doi:10.1002/mus.21891
Snijders T., Verdijk L. B., Mckay B. R., Smeets J. S. J., Van Kranenburg J., Groen B. B. B., et al. (2014a). Acute Dietary Protein Intake Restriction Is Associated with Changes in Myostatin Expression after A Single Bout of Resistance Exercise in Healthy Young Men. J. Nutr. 144, 137–145. doi:10.3945/jn.113.183996
Snijders T., Verdijk L. B., Smeets J. S. J., Mckay B. R., Senden J. M. G., Hartgens F., et al. (2014b). The Skeletal Muscle Satellite Cell Response to A Single Bout of Resistance-type Exercise Is Delayed with Aging in Men. Age 36, 9699. doi:10.1007/s11357-014-9699-z
Stumm J., Vallecillo-García P., Vom Hofe-Schneider S., Ollitrault D., Schrewe H., Economides A. N., et al. (2018). Odd Skipped-Related 1 (Osr1) Identifies Muscle-Interstitial Fibro-Adipogenic Progenitors (Faps) Activated by Acute Injury. Stem Cell Res. 32, 8–16. doi:10.1016/j.scr.2018.08.010
Suetta C., Frandsen U., Jensen L., Jensen M. M., Jespersen J. G., Hvid L. G., et al. (2012). Aging Affects the Transcriptional Regulation of Human Skeletal Muscle Disuse Atrophy. Plos One 7, E51238. doi:10.1371/journal.pone.0051238
Theret M., Rossi F. M. V., Contreras O. (2021). Evolving Roles of Muscle-Resident Fibro-Adipogenic Progenitors in Health, Regeneration, Neuromuscular Disorders, and Aging. Front. Physiol. 12, 673404. doi:10.3389/fphys.2021.673404
Uezumi A., Fukada S., Yamamoto N., Ikemoto-Uezumi M., Nakatani M., Morita M., et al. (2014a). Identification and Characterization of PDGFRα+ Mesenchymal Progenitors in Human Skeletal Muscle. Cell Death Dis. 5, E1186. doi:10.1038/cddis.2014.161
Uezumi A., Ikemoto-Uezumi M., Zhou H., Kurosawa T., Yoshimoto Y., Nakatani M., et al. (2021). Mesenchymal Bmp3b Expression Maintains Skeletal Muscle Integrity and Decreases in Age-Related Sarcopenia. J. Clin. Invest. 131. doi:10.1172/JCI139617
Uezumi A., Fukada S.-i., Yamamoto N., Takeda S. i., Tsuchida K. (2010). Mesenchymal Progenitors Distinct from Satellite Cells Contribute to Ectopic Fat Cell Formation in Skeletal Muscle. Nat. Cell Biol. 12, 143–152. doi:10.1038/ncb2014
Uezumi A., Ikemoto-Uezumi M., Tsuchida K. (2014b). Roles of Nonmyogenic Mesenchymal Progenitors in Pathogenesis and Regeneration of Skeletal Muscle. Front. Physiol. 5, 68. doi:10.3389/fphys.2014.00068
Uezumi A., Nakatani M., Ikemoto-Uezumi M., Yamamoto N., Morita M., Yamaguchi A., et al. (2016). Cell-Surface Protein Profiling Identifies Distinctive Markers of Progenitor Cells in Human Skeletal Muscle. Stem Cell Rep. 7, 263–278. doi:10.1016/j.stemcr.2016.07.004
Vainshtein A., Sandri M. (2020). Signaling Pathways that Control Muscle Mass. Int. J. Mol. Sci. 21. doi:10.3390/ijms21134759
Van Vliet S., Shy E. L., Abou Sawan S., Beals J. W., West D. W., Skinner S. K., et al. (2017). Consumption of Whole Eggs Promotes Greater Stimulation of Postexercise Muscle Protein Synthesis Than Consumption of Isonitrogenous Amounts of Egg Whites in Young Men. Am. J. Clin. Nutr. 106, 1401–1412. doi:10.3945/ajcn.117.159855
Verdijk L. B., Dirks M. L., Snijders T., Prompers J. J., Beelen M., Jonkers R. A. M., et al. (2012). Reduced Satellite Cell Numbers with Spinal Cord Injury and Aging in Humans. Med. Sci. Sports Exerc 44, 2322–2330. doi:10.1249/mss.0b013e3182667c2e
Verdijk L. B., Gleeson B. G., Jonkers R. A. M., Meijer K., Savelberg H. H. C. M., Dendale P., et al. (2009). Skeletal Muscle Hypertrophy Following Resistance Training Is Accompanied by A Fiber Type-specific Increase in Satellite Cell Content in Elderly Men. Journals Gerontology Ser. A Biol. Sci. Med. Sci. 64A, 332–339. doi:10.1093/gerona/gln050
Verdijk L. B., Koopman R., Schaart G., Meijer K., Savelberg H. H. C. M., Van Loon L. J. C. (2007). Satellite Cell Content Is Specifically Reduced in Type Ii Skeletal Muscle Fibers in the Elderly. Am. J. Physiology-Endocrinology Metabolism 292, E151–E157. doi:10.1152/ajpendo.00278.2006
Verdijk L. B., Snijders T., Drost M., Delhaas T., Kadi F., Van Loon L. J. C. (2014). Satellite Cells in Human Skeletal Muscle; from Birth to Old Age. Age 36, 545–557. doi:10.1007/s11357-013-9583-2
Verney J., Kadi F., Charifi N., Féasson L., Saafi M. A., Castells J., et al. (2008). Effects of Combined Lower Body Endurance and Upper Body Resistance Training on the Satellite Cell Pool in Elderly Subjects. Muscle Nerve 38, 1147–1154. doi:10.1002/mus.21054
Walker D. K., Fry C. S., Drummond M. J., Dickinson J. M., Timmerman K. L., Gundermann D. M., et al. (2012). Pax7+ Satellite Cells in Young and Older Adults Following Resistance Exercise. Muscle Nerve 46, 51–59. doi:10.1002/mus.23266
Walvekar A. S., Srinivasan R., Gupta R., Laxman S. (2018). Methionine Coordinates A Hierarchically Organized Anabolic Program Enabling Proliferation. MBoC 29, 3183–3200. doi:10.1091/mbc.e18-08-0515
Wang Y. X., Dumont N. A., Rudnicki M. A. (2014). Muscle Stem Cells at A Glance. J. Cell Sci. 127, 4543–4548. doi:10.1242/jcs.151209
Wang Y. X., Rudnicki M. A. (2011). Satellite Cells, the Engines of Muscle Repair. Nat. Rev. Mol. Cell Biol. 13, 127–133. doi:10.1038/nrm3265
Wolfe R. R. (2006). The Underappreciated Role of Muscle in Health and Disease. Am. J. Clin. Nutr. 84, 475–482. doi:10.1093/ajcn/84.3.475
Wosczyna M. N., Konishi C. T., Perez Carbajal E. E., Wang T. T., Walsh R. A., Gan Q., et al. (2019). Mesenchymal Stromal Cells Are Required for Regeneration and Homeostatic Maintenance of Skeletal Muscle. Cell Rep. 27, 2029–2035. E5. doi:10.1016/j.celrep.2019.04.074
Yoon M.-S. (2017). Mtor as A Key Regulator in Maintaining Skeletal Muscle Mass. Front. Physiol. 8, 788. doi:10.3389/fphys.2017.00788
Keywords: satellite cells, fibro/adipogenic progenitor cells, skeletal muscle, nutrition, leucine
Citation: Beaudry KM, Binet ER, Collao N and De Lisio M (2022) Nutritional Regulation of Muscle Stem Cells in Exercise and Disease: The Role of Protein and Amino Acid Dietary Supplementation. Front. Physiol. 13:915390. doi: 10.3389/fphys.2022.915390
Received: 08 April 2022; Accepted: 30 May 2022;
Published: 07 July 2022.
Edited by:
David Cristóbal Andrade, University of Antofagasta, ChileReviewed by:
Osvaldo Contreras, Victor Chang Cardiac Research Institute, AustraliaMauricio Castro-Sepulveda, Universidad Finis Terrae, Chile
Copyright © 2022 Beaudry, Binet, Collao and De Lisio. This is an open-access article distributed under the terms of the Creative Commons Attribution License (CC BY). The use, distribution or reproduction in other forums is permitted, provided the original author(s) and the copyright owner(s) are credited and that the original publication in this journal is cited, in accordance with accepted academic practice. No use, distribution or reproduction is permitted which does not comply with these terms.
*Correspondence: Michael De Lisio, bWRlbGlzaW9AdW90dGF3YS5jYQ==