- 1Department of Gastroenterology, The First Affiliated Hospital of China Medical University, Shenyang, China
- 2Department of Stem Cells and Regenerative Medicine, Key Laboratory of Cell Biology, National Health Commission of China, and Key Laboratory of Medical Cell Biology, Ministry of Education of China, China Medical University, Shenyang, China
Metabolic associated fatty liver disease (MAFLD) is the most common chronic liver disease worldwide due to the sedentary and overeating lifestyle. Yet, the pathophysiology of MAFLD is still unclear and no drug has been approved for MAFLD treatment. Extracellular vesicles (EVs) are heterogenous membrane-bound particles released from almost all types of cells. These nano-sized particles mediate intercellular communication through their bioactive cargos including nucleic acids, proteins, and lipids. The EVs modulate metabolic homeostasis via communication between adipose tissue and liver. The dysregulation of lipid metabolism leads to inflammation in liver and the number and compounds of EVs are changed during MAFLD. The injured hepatocytes secrete EVs to induce the migration of bone marrow-derived monocytes and the activation of macrophages in liver. The EVs secreted by different cells regulate the alteration of hepatic stellate cell (HSC) phenotypes and HSC activation gives rise to liver fibrosis. Based on the participation of EVs in MAFLD progression, we discuss the prospects of EVs as a therapeutic target and their application in drug delivery.
Introduction
Non-alcoholic fatty liver disease (NAFLD), the most common chronic liver disease, affects about 25 percent of the world’s population and causes a heavy economic burden (Younossi et al., 2016). In China, the prevalence of NAFLD is about 30% (Wu et al., 2020a; Wang et al., 2021), and the number of patients is predicted to reach 314.58 million in 2030 (Estes et al., 2018). Previously, NAFLD was mainly characterized by steatosis in >5% hepatocytes, without other fat accumulation reasons such as alcohol consumption, steatogenic medication use and hereditary diseases (Chalasani et al., 2012; European Association for the Study of the Liver (EASL)European Association for the Study of Diabetes (EASD)European Association for the Study of Obesity (EASO), 2016). But, NAFLD can coexist with viral hepatitis, autoimmune liver disease and alcoholic hepatitis. Furthermore, alcohol intake reported by patients is often inaccurate in clinical practice. These issues challenge the definition and diagnosis of NAFLD. Recently, an international consensus panel on fatty liver renamed this disease from NAFLD to metabolic associated fatty liver disease (MAFLD) (Eslam et al., 2020a). The new diagnosis of MAFLD is based on liver steatosis confirmed by liver biopsy, imaging method, or blood markers, with one of the following conditions: overweight/obesity, type 2 diabetes mellitus (T2DM), or metabolic syndrome (Eslam et al., 2020b). Formerly, the spectrum of NAFLD included simple steatosis, NAFLD associated steatohepatitis (NASH) and advanced fibrosis, cirrhosis, or even hepatocellular carcinoma (Younossi et al., 2019). But in new criteria, the spectrum of disease is not divided into “NASH” and “not-NASH.” MAFLD is used as a single overarching term to reflect the continuous disease progression (Eslam et al., 2020b). The pathophysiology underlying MAFLD is highly intricate. It evolved from the “two-hit” hypothesis to the “multiple parallel hits” theory (Tilg and Moschen, 2010), and the alteration of denomination indicated that MAFLD was a feature of metabolic syndrome (Marchesini et al., 2001; Eslam et al., 2020a). Novel regulatory molecules and pathways are being discovered, but associated pharmaceutical therapies have not been approved.
Extracellular vesicles (EVs) are a heterogeneous collection of nano-sized vesicles, involving exosomes, microvesicles and apoptotic bodies. They contain various surface markers and cargos derived from parental cells. The surface molecules on EVs can bind to the receptors on recipient cells, inducing intracellular signaling pathways. Then, EVs are internalized via endocytosis, phagocytosis or directly fusing with the membrane of target cells (Devhare and Ray, 2018; van Niel et al., 2018). In normal conditions, cells release EVs to exchange messages. Pathological conditions change the biogenesis mechanism and contents of EVs. Besides, EVs can be artificially modified by engineering surface molecules or loading therapeutic agents (Tang et al., 2020). The exciting features of EVs intrigue us to study the roles of EVs in the pathophysiology and treatment of MAFLD.
What are Extracellular Vesicles?
Definition and Classification of Extracellular Vesicles
EVs, nanoparticles with lipid bilayers and cytosolic components, are released into extracellular space by virtually all active cells. The cargos of EVs comprise nucleic acids (mRNA, microRNA, long non-coding RNA, genomic DNA, or mitochondrial DNA), proteins and lipids. The lipid bilayers of EVs protect these compounds from degradation in the extracellular environment (Rilla et al., 2019). EVs are ubiquitously present in human body fluids, including plasma, urine, bile, breast milk, saliva, semen, synovial fluid, ascites, cerebrospinal fluid, pleural effusions, bronchoalveolar lavage fluids, and even feces (Witwer et al., 2013). Also, EVs could be collected from culture supernatants of many eukaryotic cells (Giebel and Helmbrecht, 2017). According to their size, surface markers and biogenesis, EVs are classified into three subcategories (Table 1).
Biogenesis and Release of Extracellular Vesicles
In response to various stimuli and stress, cells secrete EVs via different mechanisms (Figure 1). Exosome is a class of EVs studied extensively. During the endocytosis process, the cell membrane invaginates, and then early endosomes are formed. As intraluminal vesicles (ILVs) bud into endosome membrane, the early endosomes mature into late endosomes which are referred as multivesicular bodies (MVBs). Subsequently, MVBs degrade in lysosomes or fuse with the plasma membrane to release ILVs as exosomes (Xu et al., 2018; Rilla et al., 2019).
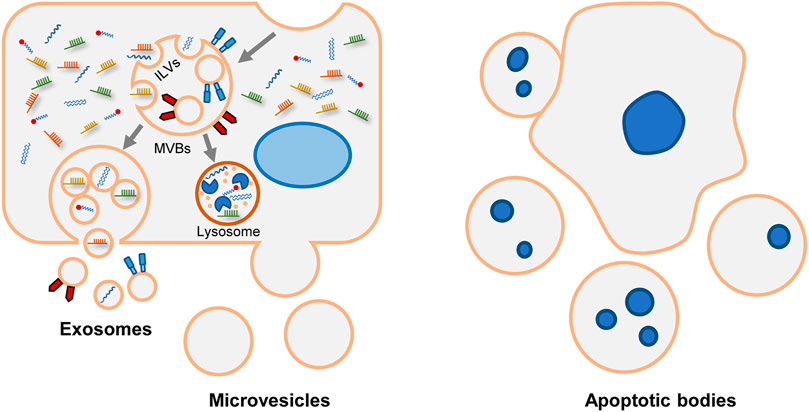
FIGURE 1. Biogenesis and release of various EVs. Generally, EVs are divided into exosomes, microvesicles and apoptotic bodies. Exosomal formation begins with endocytic process. After the endosomal membrane invagination, intraluminal vesicles (ILVs) are formed. The endosomes containing ILVs are referred as multivesicular bodies (MVBs). Subsequently, MVBs have two fates. They can be degraded in lysosomes. Alternatively, MVBs merge with plasma membrane and release ILVs as exosomes. Microvesicles are formed through direct budding process. Apoptotic bodies are formed during apoptosis.
The mechanisms of ILVs assembly and MVBs formation are complicated. The endosomal sorting complex required for transport (ESCRT) is the central machinery that determines cargo sorting, cargo clustering, and the invagination of endosomes (Hirsova et al., 2016a). Alternatively, some ESCRT-independent pathways have been described, including neutral sphingomyelinase (nSMAse-2), ceramide (Trajkovic et al., 2008; Bianco et al., 2009), tetraspanins CD9, and CD63 (Andreu and Yáñez-Mó, 2014). Besides, syntenin modulates the intraluminal budding of endosomal membranes. In time of ILVs formation, syntenin binds to syndecan and recruits ALIX, an accessory protein of ESCRT (Baietti et al., 2012). Heparanase trims the heparan sulfate chains on syndecan and activates the syndecan-syntenin-ALIX pathway (Roucourt et al., 2015). Syntenin-mediated exosomes biogenesis also depends on the small GTPase ADP ribosylation factor 6 (ARF6) and its effector phospholipase D2 (PLD2) (Ghossoub et al., 2014). And tyrosine kinase SRC is the upstream regulator of ARF6/PLD2 (Imjeti et al., 2017). The mechanisms vary among different cell types. For instance, CXCR1 and CXCR2 coordinate the release of exosomes in hepatocytes (Lee et al., 2021); adiponectin increases exosomes formation in MVBs in endothelial cells (Obata et al., 2018).
Different external stimuli also affect the formation of exosomes. In MAFLD state, toxic lipids are often accumulated in hepatocytes and regulate the formation of exosomes (Figure 2). Study showed that saturated free fatty acids activated death receptor 5 (DR5) and recruited caspase 8 and caspase 3 (Cazanave et al., 2011). Then caspase 3 cleaved and activated Rho-associated kinase 1 (ROCK1) (Coleman et al., 2001). And the release of hepatic exosomes was mediated by this signaling pathway: DR5 → caspase 8 → caspase 3 → ROCK1 (Hirsova et al., 2016b). During the early stage of lipid accumulation, geranylgeranyl diphosphate synthase (Ggpps) was upregulated and induced the secretion of exosomes in hepatocytes. In details, Ggpps geranylgeranylated a Rab GTPase-Rab27A and promoted MVBs distribution and exosome formation (Zhao et al., 2020). Besides, mixed lineage kinase 3 (MLK3) activated JNK and aggravated MAFLD progression (Ibrahim et al., 2014). The secretion of exosome during MAFLD was also regulated by MLK3 and activated JNK. Treated with MLK3 inhibitor or JNK inhibitor, hepatocytes released less exosomes. Subsequently, exosomes were loaded with a specific cargo-(C-X-C motif) ligand 10 (CXCL10), which depended on P-STAT1 and P-P38 phosphorylated by MLK3 (Ibrahim et al., 2016). As a sensor of lipotoxic stress, inositol requiring enzyme 1α (IRE1α) could increase the release of exosomes by splicing X-box binding protein-1 (XBP1). Then, IRE1α packaged ceramide via upregulating serine palmitoyltransferase1 (SPT1) (Kakazu et al., 2016a). In addition to ceramide, sphingosine-1-phosphate (S1P) was enclosed in exosomes of hepatocytes (Kakazu et al., 2016a). Another study found sphingosine kinase 1 (SphK1) and sphingosine kinase 2 (SphK2) induced the release of exosomes in lipotoxic hepatocytes by mediating the formation of S1P (Liao et al., 2018). Lysosome can degrade MVBs before MVBs fuse with the cell membrane. In MAFLD patients, damage-regulated autophagy modulator (DRAM) could recruit stomatin (STOM) and increase the lysosomal membrane permeabilization. MVBs were degraded less and released more exosomes (Zhang et al., 2021).
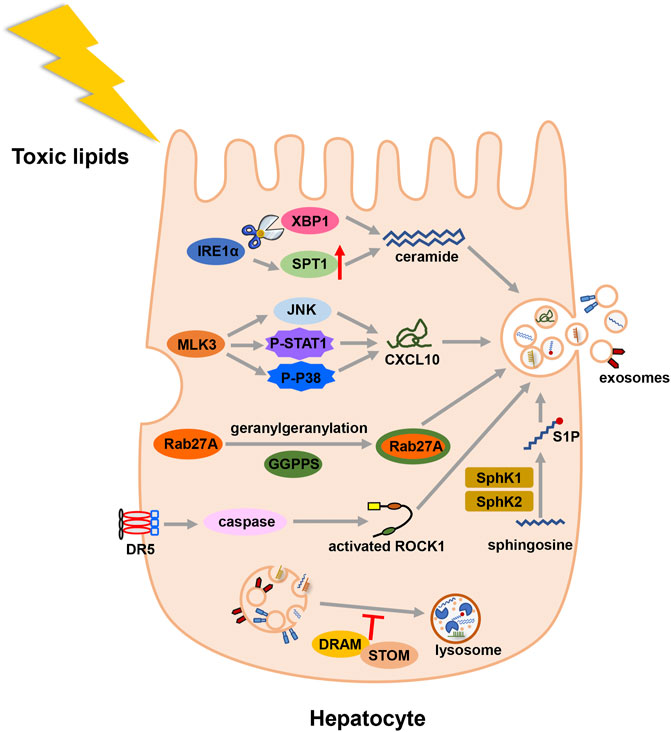
FIGURE 2. Illustration for the biogenesis fo exosomes in MAFLD. Toxic lipid accumulation is the major hit of MAFLD, which causes more secretion of exosomes by multiple mechanisms. Abbreviation: death receptor 5 (DR5), Rho-associated kinase 1 (ROCK1), geranylgeranyl diphosphate synthase (Ggpps), mixed lineage kinase 3 (MLK3), (C-X-C motif) ligand 10 (CXCL10), inositol requiring enzyme 1α (IRE1α), X-box binding protein-1 (XBP1), serine palmitoyltransferase1 (SPT1), sphingosine-1-phosphate (S1P), sphingosine kinase 1 (SphK1), and sphingosine kinase 2 (SphK2), damage-regulated autophagy modulator (DRAM), stomatin (STOM).
Unlike exosomes, microvesicles and apoptotic bodies are formed via direct exocytosis. The initiate process of exocytosis depends on the contraction of actin-myosin. Phosphatidylserine (PS) is usually segregated in the inner layer. Whereas in response to stimulation, PS is externalized via the activation of flippases and floppases, and causing MVs release. So, it allows PS to be a characteristic lipoprotein of microvesicles (Hugel et al., 2005). But not all microvesicles express PS. For example, partial platelet-derived microvesicles are PS-negative (Arraud et al., 2014; Lundström et al., 2020). The overlapped size and the limitation of purification technology make it challenging to categorize exosomes or microvesicles accurately (Xu et al., 2015; Giebel and Helmbrecht, 2017; Chuo et al., 2018; Nolan and Duggan, 2018). In this review, EVs are used as a general term to represent exosomes and microvesicles. Apoptotic bodies are not the research focus of EVs, so they are not discussed.
Extracellular Vesicles and Metabolic Associated Fatty Liver Disease Pathogenesis
With the in-depth study, the “two-hit” hypothesis of MAFLD is challenged. To supplement the deficiency of previous theory, the “multiple parallel hits” theory has been proposed, which involves lipotoxicity, adipokines, insulin resistance (IR), intestinal dysfunction, endoplasmic reticulum (ER) stress, diet, and genetic factors (Tilg and Moschen, 2010; Noureddin and Sanyal, 2018; Tilg et al., 2021). Recent studies confirmed that primary hepatocytes (Conde-Vancells et al., 2008; Ogese et al., 2019), hepatocellular carcinoma cells (Matilainen et al., 2020), hepatic stellate cells (Kostallari et al., 2018), adipocytes (Crewe and Scherer, 2021) and liver sinusoidal endothelial cells (Wang et al., 2015) secreted EVs. A proteomic analysis of nontumoral hepatocytes-derived EVs identified 244 proteins in EVs. Gene ontology (GO) analysis revealed that these proteins had relation to intracellular transport, lipid metabolism, catabolic process and the generation of precursor metabolites and energy (Conde-Vancells et al., 2008). In MAFLD mice models, Povero et al. observed early increased release of EVs in the liver and blood (Povero et al., 2014). And there was a correlation between the elevated level of EVs and hepatocyte cell death, fibrosis, and pathological angiogenesis. In human bodies, Kornek et al. found circulating EVs derived from immune cells were elevated in patients with MAFLD versus healthy individuals. And EVs number also correlated with the severity of MAFLD (Kornek et al., 2012). Here, we reviewed recent findings and discussed how EVs participated in the different stages of MAFLD.
Extracellular Vesicle: A Mediator of Lipid Metabolic Dysfunction
MAFLD can be considered as the hepatic manifestation of systematic metabolic disorder. Insulin pathways involve closely in metabolism homeostasis. When insulin response is diminished, or the insulin pathway is impaired, IR occurs, which contributes to the onset and progression of MAFLD (Khan et al., 2019; Gerges et al., 2021; Rinaldi et al., 2021). In a rat model of MAFLD, insulin associated PI3K/Akt/mTOR pathway was inhibited (Sztolsztener et al., 2021). In obese people preexisting T2DM, the hyperinsulinemia and lower insulin sensitivity was related to the presence of MAFLD (Lomonaco et al., 2016). Compared with low IR people, high IR people had higher hepatic steatosis, and inflammation (Luukkonen et al., 2016). Besides, insulin resistance index (HOMA-IR) could be a diagnostic indicator of MAFLD to some degree (Isokuortti et al., 2017). Adipose tissue plays important roles in the development of MAFLD (Azzu et al., 2020). Several studies have shown that adipose tissue can function as an endocrine organ and secrete EVs to regulate IR of hepatocytes. In obesity mice, adipose tissue-derived EVs contained less miR-141-3p and impeded insulin response of hepatocytes (Dang et al., 2019). Furthermore, a study of human adipose tissue showed that most of EVs inhibited insulin signaling. And many adipokines existed in EVs, such as monocyte chemoattractant protein-1 (MCP-1), migration inhibitory factor (MIF) and interleukin-6 (IL-6) (Kranendonk et al., 2014). Obesity also results in the accumulation of proinflammatory macrophages in adipose (Weisberg et al., 2003). These macrophages secreted EVs, which contained miR-155 and miR-29a, and depressed insulin sensitivity of hepatocytes (Ying et al., 2017; Liu et al., 2019).
In MAFLD patients, IR stimulated de novo lipogenesis of hepatocytes (Smith et al., 2020). So beyond impairing insulin signaling, EVs from adipocytes also induced following fatty accumulation. Li et al. reported that adipocytes-secreted EVs could deliver miR-199a-5p and foster hepatic lipid deposition and steatosis (Li et al., 2020). The function of miR-199a-5p was achieved via targeting mammalian sterile 20-like kinase 1 (MST1) and the downstream SREBP-1c and AMPK pathway. In adipocytes, AMPKα1 was found to impede EVs secretion (Yan et al., 2021). EVs shed from AMPKα1 deficient adipose tissue elevated the level of triglycerides (TGs), cholesterol in liver. In these EVs, CD36, a fatty acid transporter (Rada et al., 2020), had high level among 43 different protein cargos. CD36 promoted the internalization of EVs and aggravated the lipid accumulation and apoptosis of HepG2 cells. Metformin, which relieved diabetes via the activation of AMPK, was also found to suppress the secretion of EVs and alleviate high fat diet (HFD)-induced fatty liver (Yan et al., 2021). Under ER stress, adipocytes secreted more EVs, which encapsulated aldo-keto-reductase 1B7 (Akr1b7). After targeting hepatocytes, Akr1b7 could augment the accumulation of glycerol and TGs and elevated inflammation factors and fibrosis indicators (Gu et al., 2021). Another molecule involved in adipose tissue-liver crosstalk was resistin, which was rich in adipocytes-generated EVs (Rong et al., 2019). Injecting resistin-enriched EVs elevated the serum TGs and hepatic lipid accumulation of mice. But interestingly, the markers associated with inflammation, fibrosis and cell apoptosis were not obviously altered in hepatocytes. This meant resistin-enriched EVs might trigger hepatic steatosis without eliciting hepatic inflammation. Melatonin was found to reduce the level of resistin in EVs and alleviate the HFD-induced hepatic steatosis.
Above studies suggested that adipose tissue-derived EVs probably were “harmful,” especially in the obese state. Liver was the major target of adipose tissue-derived EVs. And the final effect included IR, lipid accumulation, inflammatory response, and fibrosis progression (Figure 3). Classical drugs, metformin and melatonin might relieve MAFLD via inhibiting the release of “harmful” EVs or reducing the “harmful” cargos levels in adipocyte-derived exosomes.
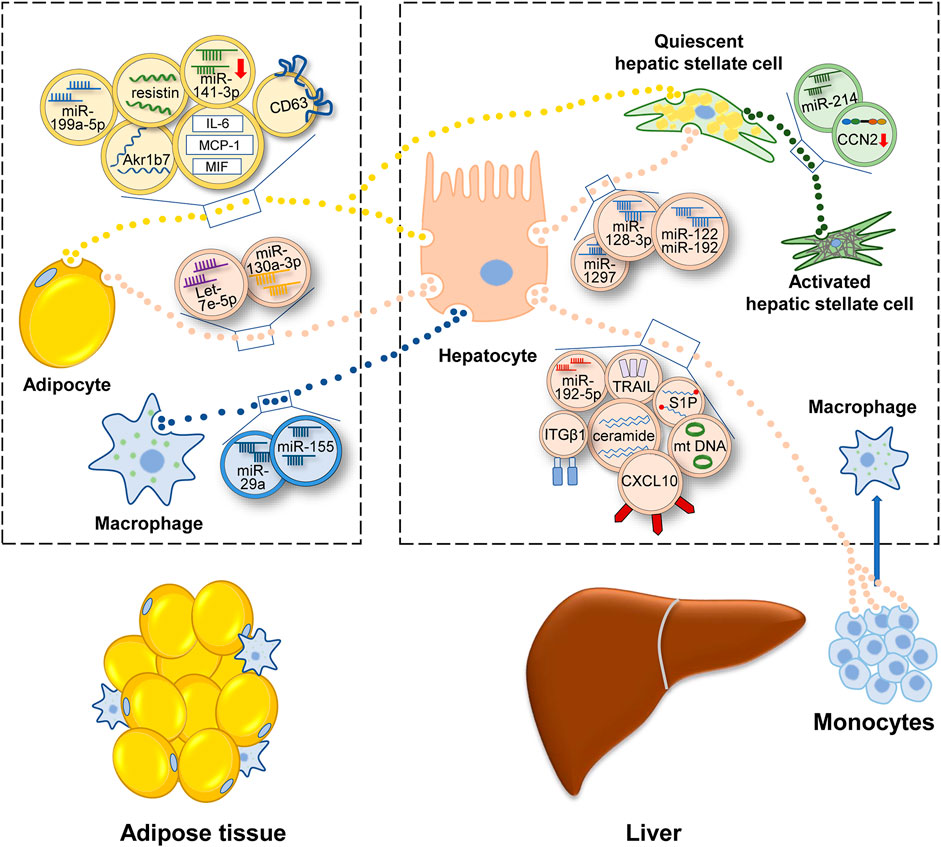
FIGURE 3. Illustration for how EVs participate in the development of MAFLD. Under the condition of metabolic disturbance, cells secrete EVs to transfer information, which contain protein, lipid, miRNA, lncRNA or DNA. Adipocytes and macrophages in adipose tissue transfer EVs to liver and regulate the lipid metabolism and fibrotic phenotype in liver. Inside liver, EVs link up the communication of various hepatic cells and promote MAFLD progression. EVs secreted outside the liver can be consumed by adipocytes and monocytes, then regulating energy metabolism and inflammatory condition. Abbreviation: aldo-keto-reductase 1B7 (Akr1b7), cluster of differentiation 36 (CD36), interleukin- 6 (IL-6), monocyte chemoattractant protein-1 (MCP-1), migration inhibitory factor (MIF), connective tissue growth factor (CCN2), mitochondrial DNA (mtDNA), sphingosine-1-phosphate (S1P), tumor necrosis factor-related apoptosis-inducing ligand (TRAIL), integrin β1(ITGβ1), C-X-C motif ligand 10 (CXCL10).
The lipid dynamics in liver can be charactered by adipose EVs. Meanwhile, hepatocytes also secrete EVs to regulate adipogenesis. In HFD-fed mice, hepatic EVs increased lipid deposition of adipocytes. Detecting the contents of EVs, let-7e-5p had the highest level. It was found that let-7e-5p could target a metabolic gene PPARγ coactivator-1 α (Pgc1α) and accelerate lipogenesis and impede lipid oxidation of adipocytes (Zhao et al., 2020).
As a response to the cellular damage, adipose tissue and liver secrete EVs and interact lipid metabolism. As to the exact role of EVs, it still needs further study. In future researches, we’d better increase the sample volume and incorporate different adipose tissue, such as epididymal, visceral and retroperitoneal adipose tissue into studies. The progressive dysfunction of adipose tissue is the key event of MAFLD. How liver monitor the adipose metabolism by EVs merits more researches.
Extracellular Vesicles: Linkage Between Liver and Inflammatory Cells
The progression of MAFLD is accompanied by the inflammatory response. The hallmarks of activation of the immune system include polarization of Kupffer cells (KCs) and recruitment of bone marrow-derived monocytes. Previous studies revealed that HFD and free fatty acids (FFAs) could activate KCs in vivo and in vitro (Xue et al., 2014; Duarte et al., 2015). Recent studies implied that EVs might be the intermediary agent of inflammatory response (Figure 3). Deng et al. (2009) reinjected EVs of HFD-fed mice into regular chow diet-fed mice. It was found that immature myeloid cells accumulated in liver and the levels of IL-6, aspartate aminotransferase (AST) and alanine aminotransferase (ALT) in serum were elevated (Deng et al., 2009). Whereas, a similar experiment conducted in humans had a contrary result. Circulating EVs isolated from the plasma of normal or obese people intended to impede inflammation in HepG2 cells, evidenced by the declining level of IL-6 and TNF-α (Afrisham et al., 2021). The opposite conclusion still needs further proof because of the small number of study samples. In addition, both two studies did not demonstrate the cell sources of EVs directly. Another study may be more accurate. Garcia-Martinez et al. (2016) isolated EVs from the serum of mice and patients with steatohepatitis and verified that the source of EVs was hepatocyte. These hepatic EVs contained oxidized mitochondrial DNA and recruited inflammatory cells depending on the activation of toll-like receptor (TLR) (Garcia-Martinez et al., 2016).
The accumulation of harmful lipid droplets, such as FFAs, TGs, free cholesterol, lysophosphatidyl choline (LPC), bile acids, and ceramides induces lipotoxicity in liver (Mendez-Sanchez et al., 2018). Many cells in the liver are affected by lipotoxicity, including hepatocytes, stellate cells, macrophages, and bile duct cells (Marra and Svegliati-Baroni, 2018). Palmitate acid (PA), a kind of FFAs, enabled hepatic EVs to recruit bone marrow-derived macrophages (BMDMs). Interestingly, these PA-induced EVs contained a common lipid: ceramide. And IRE1α, which facilitated EVs secretion, was the key director for macrophages accumulation in liver (Kakazu et al., 2016b; Dasgupta et al., 2020). In another study, cutting down the level of S1P in EVs inhibited the chemotaxis of macrophages. Thus, S1P in lipotoxic hepatocytes may also influence macrophages (Liao et al., 2018).
In addition to these bioactive lipid species, EVs also encapsulate a diverse range of cargos, such as signaling proteins, oxidized molecules or miRNAs so as to transmit proinflammatory information (Ipsen and Tveden-Nyborg, 2021). Ibrahim et al. (2016) found that LPC-treated hepatocytes secreted more EVs, which were loaded with CXCL10. Then these EVs activated macrophages by CXCL10-CXCR3 interaction (Ibrahim et al., 2016). Another study elucidated that tumor necrosis factor-related apoptosis-inducing ligand (TRAIL), a membrane protein of lipotoxic EVs, activated macrophage via the interplay with DR5 (Hirsova et al., 2016b). Similarly, LPC activated integrin β1 (ITGβ1) in hepatocytes and cytoplasmic ITGβ1 was shipped into EVs. Then these EVs were released into the circulation and fostered BMDMs adhering to liver sinusoidal endothelial cells (LSECs) by the binding between ITGβ1 and vascular cell adhesion molecule 1 (VCAM-1) (Guo et al., 2019). In another recent study, miR-192-5p in lipotoxic hepatocyte-derived EVs activated macrophages by regulating the Rictor/Akt/FoxO1 signaling cascade (Liu et al., 2020).
Collectively, these evidences suggested the important role of EVs in converting dysfunctional metabolic signal into proinflammatory signal and inducing inflammation signaling cascade. Other immune cells also play a crucial part in MAFLD, such as, neutrophils, T-helper (Th), and cytotoxic CD8+ T cells (Kuchay et al., 2020). There is still a broad space to investigate how EVs regulate immune cells of MAFLD patients.
Extracellular Vesicles Targeting Hepatic Stellate Cell Fuel Fibrosis in Metabolic Associated Fatty Liver Disease
As a major determinant of poor outcome in MAFLD progression, liver fibrosis is characterized by an unbalanced restoration of extracellular matrix (ECM). The key node of hepatic fibrogenesis is the activation of hepatic stellate cells (HSCs). Then HSCs transdifferentiate into myofibroblasts-like cell type (Tsuchida and Friedman, 2017).
Many liver cells can activate HSCs, involving hepatocytes, endothelial cells (Wang et al., 2015), natural killer (NK) cells (Wang et al., 2020) or macrophages. They constitute a cellular network, which is connected by EVs (Figure 3). In this section, we center on those researches whose models are relevant to MAFLD. For example, EVs isolated from lipotoxic hepatocytes leaded to a phenotype switch of HSCs from quiescence to activation. MiR-128-3p was rich in these EVs and targeted PPARγ (Povero et al., 2015). Using a microarray, Lee et al. (2017) found that lipotoxic hepatocytes-derived EVs had a remarkably increased level of miR-122 and miR-192, which were extensively reported in the development of MAFLD (Lee et al., 2017; Khalifa et al., 2020). MiR-192 upregulated the expression of fibrosis markers and activated the profibrogenic function of HSCs. And elevated miR-122 and miR-192 were also observed in patients with advanced-stage MAFLD compared to those with early-stage MAFLD (Lee et al., 2017). Recently, miRNA-1297 in lipotoxic hepatocytes-derived EVs was identified to activate HSCs via PTEN pathway (Luo et al., 2021a). In addition to the treatment with excess fatty acid, obstructive sleep apnea syndrome (OSAS) also contributed to steatohepatitis and fibrosis (Nobili et al., 2014). Hepatocytes under chemic hypoxia were detected to secret EVs, which caused increased expression of profibrotic cytokines in HSCs (Hernández et al., 2020). Besides, HSCs can be awakened by EVs secreted from activated HSCs (Charrier et al., 2014). EVs delivered connective tissue growth factor (CCN2) to other quiescent or activated HSCs and upregulated the expression of fibrotic genes. MiR-214 that regulated CCN2 negatively was also identified in activated HSCs-derived EVs. Correspondingly, the level of miR-214 in EVs was low (Chen et al., 2014). As an endocrine organ, adipose tissue also could trigger fibrosis via delivering EVs into HSCs and modulate transforming growth factor beta (TGF-β) pathway (Koeck et al., 2014).
Extracellular Vesicles: Potential Therapeutical Tools in Metabolic Associated Fatty Liver Disease
The severity of MAFLD depends on the grade of activity and fibrosis progression. A scoring system, NAFLD activity score (NAS), quantifies the activity of MAFLD, including steatosis, inflammation, and hepatocellular ballooning. Recent medical studies confirmed that lifestyle modification could degrade NAS and alleviate the early stage of fibrosis (Romero-Gómez et al., 2017). The underlying mechanism of exercise in systemic improvement is still unclear. Probably, this beneficial effect was modulated by EVs (Safdar et al., 2016). During endurance exercise, the number of EVs was elevated and their cargos, such as proteins and miRNAs were also changed (Estébanez et al., 2021). Then exercise-derived EVs were intended to localize in liver and exchange their compounds (Whitham et al., 2018).
Regardless of the mechanism, it is definite that physical exercise is a primary therapy for MAFLD. But in terms of medical treatment, there has been no breakthrough in drug research. As discussed before, EVs participate in the development of MAFLD. These “harmful” EVs can amplify fat accumulation, promote the polarization and infiltration of immune cells, and activate HSCs. So, it is plausible to attenuate MAFLD by inhibiting the formation, cargo sorting and release of EVs (EL Andaloussi et al., 2013). For example, it turned out that the release of EVs from lipotoxic hepatocytes depended on ROCK1. The injection of ROCK1 inhibitor fasudil decreased the serum EVs of MAFLD mice and ameliorated the severity of MAFLD (Hirsova et al., 2016b). Pharmacological inhibition of MLK3 was found to impede the enrichment of CXCL10 in EVs and alleviate MAFLD progression in mice (Ibrahim et al., 2016; Tomita et al., 2017). Fat, fructose, and cholesterol (FFC) diet-induced steatohepatitis could be attenuated by pharmacologic IRE1α inhibition and genetic IRE1α knockout (Dasgupta et al., 2020). But proteins that modulate the biogenesis of EVs also affect other vital cellular functions, such as endolysosomal trafficking (Hirsova et al., 2016a). So, how to precisely regulate target effects is the important direction of future research.
During the progression of MAFLD, EVs played a “harmful” role in the crosstalk between adipose tissue and liver. However, during experimental stem cell therapy, stem cells-derived EVs may serve as a “salutary” medium to halt the inflammation and fibrosis of MAFLD. For instance, both human adipose tissue-derived mesenchymal stem cells (AD-MSCs) and their EVs reduced the level of ALT and liver fibrosis in MAFLD mice. Meanwhile, it was observed that anti-inflammatory macrophages were increased in liver (Watanabe et al., 2020). Similarly, another study confirmed that AD-MSCs and their EVs attenuated hepatic steatosis through the interaction with macrophages. AD-MSCs-derived EVs delivered signal transducer and activator of transcription3 (STAT3) into macrophages, and shifted them into anti-inflammation M2 phenotype (Zhao et al., 2018).
Besides adipose tissue, mesenchymal stem cells (MSCs) from other sources also released EVs and exerted protective effect in MAFLD. Ohara et al. (2018) injected amnion-derived MSCs (AMSCs)-derived EVs into steatohepatitis mice. Then the inflammation and fibrosis were alleviated perhaps via inactivating Kupffer cells and HSCs (Ohara et al., 2018). Likewise, induced pluripotent stem cells (iPSCs)-derived EVs downregulated the expression of fibrotic genes and inhibited the proliferation and chemotaxis of HSCs. In miRNA profile of iPSC-derived EVs, miR-92a-3p had a high expression level (Povero et al., 2019). A recent study reported that human liver stem cells (HLSCs)-originated EVs improved the inflammation and fibrosis of MAFLD (Bruno et al., 2020a). Notably, after proteomic analysis, several anti-inflammation proteins were enriched in HLSCs-originated EVs. Therefore, it was speculated that these bioactive cargos might serve as the actual mediators.
Compared with conventional drug delivery platforms, EVs have unique superiorities, such as low immunogenicity, less tumorigenicity, biological barriers penetrability, biocompatibility, and less exogenous infection (Bruno et al., 2020b; Hu et al., 2020; Tang et al., 2020). Thus, it is possible to use EVs to transport exogenous molecules. With the structure of hydrophobic lipid membrane and an aqueous core, EVs may address the shortcomings of RNA-based therapies, such as degradation, low uptake, and off-target effect (Wiklander et al., 2019). Qu et al. (2017) transfected pre-miR-181-5p into AD-MSCs and collected EVs with an enrichment of miR-181-5p (Qu et al., 2017). These EVs repressed HSCs activation and induced HSCs autophagy via STAT3/Bcl-2/Beclin1 signaling pathway. Besides, liver fibrosis could be attenuated by EVs in vitro and in vivo. Similarly, AD-MSCs-derived EVs loaded with miR-122 showed therapeutic potential in liver fibrosis (Lou et al., 2017). MiR-130a was decreased in the liver of MAFLD rats and in the islet of non-obese spontaneous type 2 diabetes, indicating that it might be protective for metabolic disorder (Jin et al., 2009; Esguerra et al., 2011; Xiao et al., 2014). Using CRISPR/CAS9 technique and mimic transfection method, the model of miR-130a overexpressed hepatocytes was constructed in vivo and in vitro. EVs released from these hepatocytes contained more miR-130a. It was found that these hepatic EVs suppressed fat synthesis and ameliorated IR of adipocytes. And miR-130a could target PH domain leucine-rich repeat protein phosphatase 2 (PHLPP-2) and AKT-AS160-GLUT4 pathway (Wu et al., 2020b). As we can see, after transfection, parental cells can deliver EVs which contain therapeutic miRNAs. In addition to transfection, Momen-Heravi et al. (2014) provided a novel sight to load EVs with exogenous miRNAs (Momen-Heravi et al., 2014). Using electroporation, EVs were rich in miR-155 mimics and could replenish the hepatic level of miR-155 in miR-155 knockout mice. Additionally, the delivery of miR-155 by EVs was more efficient and less cytotoxic than classical transfection. A recent study even applied gene therapy to EVs delivery (Luo et al., 2021b). After transfection, the clustered regularly interspaced short palindromic repeats (CRISPR)/CRISPR-associated protein 9 (dCas9)-VP64 system could be detected in mice hepatocytes-derived EVs. Then CRISPR/dCas9-VP64 system activated hepatocyte nuclear factor 4a (HNF4α) gene in HSCs and improved fibrosis.
The therapeutic applications of EVs include regulating the formation of EVs and employing EVs as a neo-drug delivery system (Tang et al., 2020). Preclinical studies of MSCs-EVs showed their promising properties in reducing fibrosis and counteracting inflammation. Most studies of MSC-EVs therapy were conducted by direct injection in vivo or co-cultivation in vitro. Future studies can pay more attention to the catalogs and levels of cargos and the mechanisms in modulating target cells. According to the existing reports and www.clinicaltrials.gov, EVs-mediated MAFLD therapy has not yet been tested in clinical study (Massa et al., 2020). Meanwhile a set of challenges need to be tackled in following clinical EVs application, such as the sources, large-scale production, standard of isolated process and purify, half-time degradation, administration route and frequency and dosage (Bruno et al., 2020b; Tang et al., 2020).
Conclusion and Future Prospects
In this review, we highlighted the roles of EVs in cellular and organic communication. Most studies demonstrated that EVs exacerbated MAFLD development. Recently, it was found that in early obesity mice, hepatocytes secreted miR-3075-enriched EVs to improve insulin sensitivity. But in chronic obesity, this compensatory mechanism disappeared. The function of hepatocytes EVs was converted into promoting IR (Ji et al., 2021). This result suggested that EVs had different effects during different stages of MAFLD. It seemed to explain why some EVs showed a protective effect in previous studies. For example, some adipose tissue-derived EVs activated the insulin pathway of hepatocytes; plasma EVs in normal and obese people inhibited inflammation of hepatocytes.
Another research interest in MAFLD is immune response. Immune cells can serve as both donor cells and target cells of EVs. Most studies in EVs are about macrophages or BMDMs. Other immunocytes, such as T cells and neutrophils may also participate in the release and uptake of EVs. Together, they construct an immune network in innate and adaptive immunity. How EVs and their cargos regulate the immune network requires more investigation.
Thus far, MAFLD has no approved drug therapies. Previous researches showed that EVs were associated with the pathogenesis of MAFLD. In this context, impeding harmful functions of EVs may be new targets for clinical intervention. On the other side, there are some protective molecules existing in harmful EVs, which play a compensatory role. Elevating the levels of these molecules exogenously may relieve MAFLD. And for potential clinical use, stem cell-derived EVs may be safer and have fewer side effects. Compared with conventional drug delivery, EVs have superior advantages as secretory nanoparticles. So, manipulating EVs as a neo-drug delivery platform holds great promise for improving MAFLD progression.
Author Contributions
JS wrote the manuscript. YL conceived the idea. DZ provided crucial feedback. All authors contributed to the article and approved the submitted version.
Funding
This work was supported by National Natural Science Foundation of China (Grant Number: 81570519 and 82073419).
Conflict of Interest
The authors declare that the research was conducted in the absence of any commercial or financial relationships that could be construed as a potential conflict of interest.
Publisher’s Note
All claims expressed in this article are solely those of the authors and do not necessarily represent those of their affiliated organizations, or those of the publisher, the editors and the reviewers. Any product that may be evaluated in this article, or claim that may be made by its manufacturer, is not guaranteed or endorsed by the publisher.
References
Afrisham R., Sadegh-Nejadi S., Meshkani R., Emamgholipour S., Bagherieh M., Paknejad M. (2021). Anti-inflammatory Effects of Plasma Circulating Exosomes Obtained from Normal-Weight and Obese Subjects on Hepatocytes. Emiddt 21, 478–484. doi:10.2174/1871530320666200505121426
Andreu Z., Yáñez-Mó M. a. (2014). Tetraspanins in Extracellular Vesicle Formation and Function. Front. Immunol. 5, 442. doi:10.3389/fimmu.2014.00442
Arraud N., Linares R., Tan S., Gounou C., Pasquet J.-M., Mornet S., et al. (2014). Extracellular Vesicles from Blood Plasma: Determination of Their Morphology, Size, Phenotype and Concentration. J. Thromb. Haemost. 12, 614–627. doi:10.1111/jth.12554
Azzu V., Vacca M., Virtue S., Allison M., Vidal-Puig A. (2020). Adipose Tissue-Liver Cross Talk in the Control of Whole-Body Metabolism: Implications in Nonalcoholic Fatty Liver Disease. Gastroenterology 158, 1899–1912. doi:10.1053/j.gastro.2019.12.054
Baietti M. F., Zhang Z., Mortier E., Melchior A., Degeest G., Geeraerts A., et al. (2012). Syndecan-syntenin-ALIX Regulates the Biogenesis of Exosomes. Nat. Cell. Biol. 14, 677–685. doi:10.1038/ncb2502
Balaphas A., Meyer J., Sadoul R., Morel P., Gonelle‐Gispert C., Bühler L. H. (2019). Extracellular Vesicles: Future Diagnostic and Therapeutic Tools for Liver Disease and Regeneration. Liver Int. 39, 1801–1817. doi:10.1111/liv.14189
Ban L., Shackel N., McLennan S. (2016). Extracellular Vesicles: a New Frontier in Biomarker Discovery for Non-alcoholic Fatty Liver Disease. Ijms 17, 376. doi:10.3390/ijms17030376
Bianco F., Perrotta C., Novellino L., Francolini M., Riganti L., Menna E., et al. (2009). Acid Sphingomyelinase Activity Triggers Microparticle Release from Glial Cells. Embo J. 28, 1043–1054. doi:10.1038/emboj.2009.45
Bruno S., Chiabotto G., Camussi G. (2020). Extracellular Vesicles: a Therapeutic Option for Liver Fibrosis. Ijms 21, 4255. doi:10.3390/ijms21124255
Bruno S., Pasquino C., Herrera Sanchez M. B., Tapparo M., Figliolini F., Grange C., et al. (2020). HLSC-derived Extracellular Vesicles Attenuate Liver Fibrosis and Inflammation in a Murine Model of Non-alcoholic Steatohepatitis. Mol. Ther. 28, 479–489. doi:10.1016/j.ymthe.2019.10.016
Cazanave S. C., Mott J. L., Bronk S. F., Werneburg N. W., Fingas C. D., Meng X. W., et al. (2011). Death Receptor 5 Signaling Promotes Hepatocyte Lipoapoptosis. J. Biol. Chem. 286, 39336–39348. doi:10.1074/jbc.M111.280420
Chalasani N., Younossi Z., Lavine J. E., Diehl A. M., Brunt E. M., Cusi K., et al. (2012). The Diagnosis and Management of Non-alcoholic Fatty Liver Disease: Practice Guideline by the American Association for the Study of Liver Diseases, American College of Gastroenterology, and the American Gastroenterological Association. Hepatology 55, 2005–2023. doi:10.1002/hep.25762
Charrier A., Chen R., Chen L., Kemper S., Hattori T., Takigawa M., et al. (2014). Exosomes Mediate Intercellular Transfer of Pro-fibrogenic Connective Tissue Growth Factor (CCN2) between Hepatic Stellate Cells, the Principal Fibrotic Cells in the Liver. Surgery 156, 548–555. doi:10.1016/j.surg.2014.04.014
Chen L., Charrier A., Zhou Y., Chen R., Yu B., Agarwal K., et al. (2014). Epigenetic Regulation of Connective Tissue Growth Factor by MicroRNA-214 Delivery in Exosomes from Mouse or Human Hepatic Stellate Cells. Hepatology 59, 1118–1129. doi:10.1002/hep.26768
Chuo S. T.-Y., Chien J. C.-Y., Lai C. P.-K. (2018). Imaging Extracellular Vesicles: Current and Emerging Methods. J. Biomed. Sci. 25, 91. doi:10.1186/s12929-018-0494-5
Coleman M. L., Sahai E. A., Yeo M., Bosch M., Dewar A., Olson M. F. (2001). Membrane Blebbing during Apoptosis Results from Caspase-Mediated Activation of ROCK I. Nat. Cell. Biol. 3, 339–345. doi:10.1038/35070009
Conde-Vancells J., Rodriguez-Suarez E., Embade N., Gil D., Matthiesen R., Valle M., et al. (2008). Characterization and Comprehensive Proteome Profiling of Exosomes Secreted by Hepatocytes. J. Proteome Res. 7, 5157–5166. doi:10.1021/pr8004887
Crewe C., Scherer P. E. (2021). Intercellular and Interorgan Crosstalk through Adipocyte Extracellular Vesicles. Rev. Endocr. Metab. Disord. 23, 61–69. doi:10.1007/s11154-020-09625-x
Dang S.-Y., Leng Y., Wang Z.-X., Xiao X., Zhang X., Wen T., et al. (2019). Exosomal Transfer of Obesity Adipose Tissue for Decreased miR-141-3p Mediate Insulin Resistance of Hepatocytes. Int. J. Biol. Sci. 15, 351–368. doi:10.7150/ijbs.28522
Dasgupta D., Nakao Y., Mauer A. S., Thompson J. M., Sehrawat T. S., Liao C.-Y., et al. (2020). IRE1A Stimulates Hepatocyte-Derived Extracellular Vesicles that Promote Inflammation in Mice with Steatohepatitis. Gastroenterology 159, 1487–1503. doi:10.1053/j.gastro.2020.06.031
Deng Z.-b., Liu Y., Liu C., Xiang X., Wang J., Cheng Z., et al. (2009). Immature Myeloid Cells Induced by a High-Fat Diet Contribute to Liver Inflammation. Hepatology 50, 1412–1420. doi:10.1002/hep.23148
Devhare P. B., Ray R. B. (2018). Extracellular Vesicles: Novel Mediator for Cell to Cell Communications in Liver Pathogenesis. Mol. Aspects Med. 60, 115–122. doi:10.1016/j.mam.2017.11.001
Duarte N., Coelho I. C., Patarrão R. S., Almeida J. I., Penha-Gonçalves C., Macedo M. P. (2015). How Inflammation Impinges on NAFLD: a Role for Kupffer Cells. BioMed Res. Int. 2015, 1–11. doi:10.1155/2015/984578
El Andaloussi S., Mäger I., Breakefield X. O., Wood M. J. A. (2013). Extracellular Vesicles: Biology and Emerging Therapeutic Opportunities. Nat. Rev. Drug Discov. 12, 347–357. doi:10.1038/nrd3978
Esguerra J. L. S., Bolmeson C., Cilio C. M., Eliasson L. (2011). Differential Glucose-Regulation of microRNAs in Pancreatic Islets of Non-obese Type 2 Diabetes Model Goto-Kakizaki Rat. PLoS One 6, e18613. doi:10.1371/journal.pone.0018613
Eslam M., Newsome P. N., Sarin S. K., Anstee Q. M., Targher G., Romero-Gomez M., et al. (2020). A New Definition for Metabolic Dysfunction-Associated Fatty Liver Disease: An International Expert Consensus Statement. J. Hepatology 73, 202–209. doi:10.1016/j.jhep.2020.03.039
Eslam M., Sanyal A. J., George J., Sanyal A., Neuschwander-Tetri B., Tiribelli C., et al. (2020). MAFLD: a Consensus-Driven Proposed Nomenclature for Metabolic Associated Fatty Liver Disease. Gastroenterology 158, 1999–2014.e1. doi:10.1053/j.gastro.2019.11.312
Estébanez B., Jiménez‐Pavón D., Huang C. J., Cuevas M. J., González‐Gallego J. (2021). Effects of Exercise on Exosome Release and Cargo in In Vivo and Ex Vivo Models: A Systematic Review. J. Cell. Physiol. 236, 3336–3353. doi:10.1002/jcp.30094
Estes C., Anstee Q. M., Arias-Loste M. T., Bantel H., Bellentani S., Caballeria J., et al. (2018). Modeling NAFLD Disease Burden in China, France, Germany, Italy, Japan, Spain, United Kingdom, and United States for the Period 2016-2030. J. Hepatology 69, 896–904. doi:10.1016/j.jhep.2018.05.036
European Association for the Study of the Liver (EASL)European Association for the Study of Diabetes (EASD)European Association for the Study of Obesity (EASO) (2016). EASL-EASD-EASO Clinical Practice Guidelines for the Management of Non-alcoholic Fatty Liver Disease. J. Hepatol. 64, 1388–1402. doi:10.1016/j.jhep.2015.11.004
Garcia-Martinez I., Santoro N., Chen Y., Hoque R., Ouyang X., Caprio S., et al. (2016). Hepatocyte Mitochondrial DNA Drives Nonalcoholic Steatohepatitis by Activation of TLR9. J. Clin. Investig. 126, 859–864. doi:10.1172/jci83885
Gerges S. H., Wahdan S. A., Elsherbiny D. A., El-Demerdash E. (2021). Non-alcoholic Fatty Liver Disease: an Overview of Risk Factors, Pathophysiological Mechanisms, Diagnostic Procedures, and Therapeutic Interventions. Life Sci. 271, 119220. doi:10.1016/j.lfs.2021.119220
Ghossoub R., Lembo F., Rubio A., Gaillard C. B., Bouchet J., Vitale N., et al. (2014). Syntenin-ALIX Exosome Biogenesis and Budding into Multivesicular Bodies Are Controlled by ARF6 and PLD2. Nat. Commun. 5, 3477. doi:10.1038/ncomms4477
Giebel B., Helmbrecht C. (2017). Methods to Analyze EVs. Methods Mol. Biol. 1545, 1–20. doi:10.1007/978-1-4939-6728-5_1
Gu H., Yang K., Shen Z., Jia K., Liu P., Pan M., et al. (2021). ER Stress-Induced Adipocytes Secrete-Aldo-Keto Reductase 1B7-Containing Exosomes that Cause Nonalcoholic Steatohepatitis in Mice. Free Radic. Biol. Med. 163, 220–233. doi:10.1016/j.freeradbiomed.2020.12.011
Guo Q., Furuta K., Lucien F., Gutierrez Sanchez L. H., Hirsova P., Krishnan A., et al. (2019). Integrin β1-enriched Extracellular Vesicles Mediate Monocyte Adhesion and Promote Liver Inflammation in Murine NASH. J. Hepatology 71, 1193–1205. doi:10.1016/j.jhep.2019.07.019
Hernández A., Reyes D., Geng Y., Arab J. P., Cabrera D., Sepulveda R., et al. (2020). Extracellular Vesicles Derived from Fat-Laden Hepatocytes Undergoing Chemical Hypoxia Promote a Pro-fibrotic Phenotype in Hepatic Stellate Cells. Biochimica Biophysica Acta (BBA) - Mol. Basis Dis. 1866, 165857. doi:10.1016/j.bbadis.2020.165857
Hirsova P., Ibrahim S. H., Krishnan A., Verma V. K., Bronk S. F., Werneburg N. W., et al. (2016). Lipid-induced Signaling Causes Release of Inflammatory Extracellular Vesicles from Hepatocytes. Gastroenterology 150, 956–967. doi:10.1053/j.gastro.2015.12.037
Hirsova P., Ibrahim S. H., Verma V. K., Morton L. A., Shah V. H., LaRusso N. F., et al. (2016). Extracellular Vesicles in Liver Pathobiology: Small Particles with Big Impact. Hepatology 64, 2219–2233. doi:10.1002/hep.28814
Hu C., Zhao L., Zhang L., Bao Q., Li L. (2020). Mesenchymal Stem Cell-Based Cell-free Strategies: Safe and Effective Treatments for Liver Injury. Stem Cell. Res. Ther. 11, 377. doi:10.1186/s13287-020-01895-1
Hugel B., Martínez M. C., Kunzelmann C., Freyssinet J.-M. (2005). Membrane Microparticles: Two Sides of the Coin. Physiology 20, 22–27. doi:10.1152/physiol.00029.2004
Ibrahim S. H., Gores G. J., Hirsova P., Kirby M., Miles L., Jaeschke A., et al. (2014). Mixed Lineage Kinase 3 Deficient Mice Are Protected against the High Fat High Carbohydrate Diet-Induced Steatohepatitis. Liver Int. 34, 427–437. doi:10.1111/liv.12353
Ibrahim S. H., Hirsova P., Tomita K., Bronk S. F., Werneburg N. W., Harrison S. A., et al. (2016). Mixed Lineage Kinase 3 Mediates Release of C-X-C Motif Ligand 10-bearing Chemotactic Extracellular Vesicles from Lipotoxic Hepatocytes. Hepatology 63, 731–744. doi:10.1002/hep.28252
Imjeti N. S., Menck K., Egea-Jimenez A. L., Lecointre C., Lembo F., Bouguenina H., et al. (2017). Syntenin Mediates SRC Function in Exosomal Cell-To-Cell Communication. Proc. Natl. Acad. Sci. U.S.A. 114, 12495–12500. doi:10.1073/pnas.1713433114
Ipsen D. H., Tveden-Nyborg P. (2021). Extracellular Vesicles as Drivers of Non-alcoholic Fatty Liver Disease: Small Particles with Big Impact. Biomedicines 9, 93. doi:10.3390/biomedicines9010093
Isokuortti E., Zhou Y., Peltonen M., Bugianesi E., Clement K., Bonnefont-Rousselot D., et al. (2017). Use of HOMA-IR to Diagnose Non-alcoholic Fatty Liver Disease: a Population-Based and Inter-laboratory Study. Diabetologia 60, 1873–1882. doi:10.1007/s00125-017-4340-1
Ji Y., Luo Z., Gao H., Dos Reis F. C. G., Bandyopadhyay G., Jin Z., et al. (2021). Hepatocyte-derived Exosomes from Early Onset Obese Mice Promote Insulin Sensitivity through miR-3075. Nat. Metab. 3, 1163–1174. doi:10.1038/s42255-021-00444-1
Jin X., Ye Y.-F., Chen S.-H., Yu C.-H., Liu J., Li Y.-M. (2009). MicroRNA Expression Pattern in Different Stages of Nonalcoholic Fatty Liver Disease. Dig. Liver Dis. 41, 289–297. doi:10.1016/j.dld.2008.08.008
Kakazu E., Mauer A. S., Yin M., Malhi H. (2016). Hepatocytes Release Ceramide-Enriched Pro-inflammatory Extracellular Vesicles in an IRE1α-dependent Manner. J. Lipid Res. 57, 233–245. doi:10.1194/jlr.M063412
Kakazu E., Mauer A. S., Yin M., Malhi H. (2016). Hepatocytes Release Ceramide-Enriched Pro-inflammatory Extracellular Vesicles in an IRE1α-dependent Manner. J. Lipid Res. 57, 233–245. doi:10.1194/jlr.M063412
Khalifa O., Errafii K., Al-Akl N. S., Arredouani A. (2020). Noncoding RNAs in Nonalcoholic Fatty Liver Disease: Potential Diagnosis and Prognosis Biomarkers. Dis. Markers 2020, 1–16. doi:10.1155/2020/8822859
Khan R. S., Bril F., Cusi K., Newsome P. N. (2019). Modulation of Insulin Resistance in Nonalcoholic Fatty Liver Disease. Hepatology 70, 711–724. doi:10.1002/hep.30429
Koeck E. S., Iordanskaia T., Sevilla S., Ferrante S. C., Hubal M. J., Freishtat R. J., et al. (2014). Adipocyte Exosomes Induce Transforming Growth Factor Beta Pathway Dysregulation in Hepatocytes: a Novel Paradigm for Obesity-Related Liver Disease. J. Surg. Res. 192, 268–275. doi:10.1016/j.jss.2014.06.050
Kornek M., Lynch M., Mehta S. H., Lai M., Exley M., Afdhal N. H., et al. (2012). Circulating Microparticles as Disease-specific Biomarkers of Severity of Inflammation in Patients with Hepatitis C or Nonalcoholic Steatohepatitis. Gastroenterology 143, 448–458. doi:10.1053/j.gastro.2012.04.031
Kostallari E., Hirsova P., Prasnicka A., Verma V. K., Yaqoob U., Wongjarupong N., et al. (2018). Hepatic Stellate Cell-Derived Platelet-Derived Growth Factor Receptor-Alpha-Enriched Extracellular Vesicles Promote Liver Fibrosis in Mice through SHP2. Hepatology 68, 333–348. doi:10.1002/hep.29803
Kranendonk M. E. G., Visseren F. L. J., van Herwaarden J. A., Nolte-'t Hoen E. N. M., de Jager W., Wauben M. H. M., et al. (2014). Effect of Extracellular Vesicles of Human Adipose Tissue on Insulin Signaling in Liver and Muscle Cells. Obesity 22, 2216–2223. doi:10.1002/oby.20847
Kuchay M. S., Choudhary N. S., Mishra S. K. (2020). Pathophysiological Mechanisms Underlying MAFLD. Diabetes & Metabolic Syndrome Clin. Res. Rev. 14, 1875–1887. doi:10.1016/j.dsx.2020.09.026
Lee J., Kim S. R., Lee C., Jun Y. I., Bae S., Yoon Y. J., et al. (2021). Extracellular Vesicles from In Vivo Liver Tissue Accelerate Recovery of Liver Necrosis Induced by Carbon Tetrachloride. J. Extracell. Vesicles 10, e12133. doi:10.1002/jev2.12133
Lee Y.-S., Kim S. Y., Ko E., Lee J.-H., Yi H.-S., Yoo Y. J., et al. (2017). Exosomes Derived from Palmitic Acid-Treated Hepatocytes Induce Fibrotic Activation of Hepatic Stellate Cells. Sci. Rep. 7, 3710. doi:10.1038/s41598-017-03389-2
Li Y., Luan Y., Li J., Song H., Li Y., Qi H., et al. (2020). Exosomal miR-199a-5p Promotes Hepatic Lipid Accumulation by Modulating MST1 Expression and Fatty Acid Metabolism. Hepatol. Int. 14, 1057–1074. doi:10.1007/s12072-020-10096-0
Liao C.-Y., Song M. J., Gao Y., Mauer A. S., Revzin A., Malhi H. (2018). Hepatocyte-derived Lipotoxic Extracellular Vesicle Sphingosine 1-phosphate Induces Macrophage Chemotaxis. Front. Immunol. 9, 2980. doi:10.3389/fimmu.2018.02980
Liu T., Sun Y.-C., Cheng P., Shao H.-G. (2019). Adipose Tissue Macrophage-Derived Exosomal miR-29a Regulates Obesity-Associated Insulin Resistance. Biochem. Biophysical Res. Commun. 515, 352–358. doi:10.1016/j.bbrc.2019.05.113
Liu X. L., Pan Q., Cao H. X., Xin F. Z., Zhao Z. H., Yang R. X., et al. (2020). Lipotoxic Hepatocyte‐Derived Exosomal MicroRNA 192‐5p Activates Macrophages through Rictor/Akt/Forkhead Box Transcription Factor O1 Signaling in Nonalcoholic Fatty Liver Disease. Hepatology 72, 454–469. doi:10.1002/hep.31050
Lomonaco R., Bril F., Portillo-Sanchez P., Ortiz-Lopez C., Orsak B., Biernacki D., et al. (2016). Metabolic Impact of Nonalcoholic Steatohepatitis in Obese Patients with Type 2 Diabetes. Diabetes Care 39, 632–638. doi:10.2337/dc15-1876
Lou G., Yang Y., Liu F., Ye B., Chen Z., Zheng M., et al. (2017). MiR-122 Modification Enhances the Therapeutic Efficacy of Adipose Tissue-Derived Mesenchymal Stem Cells against Liver Fibrosis. J. Cell. Mol. Med. 21, 2963–2973. doi:10.1111/jcmm.13208
Lundström A., Mobarrez F., Rooth E., Thålin C., von Arbin M., Henriksson P., et al. (2020). Prognostic Value of Circulating Microvesicle Subpopulations in Ischemic Stroke and TIA. Transl. Stroke Res. 11, 708–719. doi:10.1007/s12975-019-00777-w
Luo N., Li J., Chen Y., Xu Y., Wei Y., Lu J., et al. (2021). Hepatic Stellate Cell Reprogramming via Exosome-Mediated CRISPR/dCas9-VP64 Delivery. Drug Deliv. 28, 10–18. doi:10.1080/10717544.2020.1850917
Luo X., Luo S.-Z., Xu Z.-X., Zhou C., Li Z.-H., Zhou X.-Y., et al. (2021). Lipotoxic Hepatocyte-Derived Exosomal miR-1297 Promotes Hepatic Stellate Cell Activation through the PTEN Signaling Pathway in Metabolic-Associated Fatty Liver Disease. Wjg 27, 1419–1434. doi:10.3748/wjg.v27.i14.1419
Luukkonen P. K., Zhou Y., Sädevirta S., Leivonen M., Arola J., Orešič M., et al. (2016). Hepatic Ceramides Dissociate Steatosis and Insulin Resistance in Patients with Non-alcoholic Fatty Liver Disease. J. Hepatology 64, 1167–1175. doi:10.1016/j.jhep.2016.01.002
Marchesini G., Brizi M., Bianchi G., Tomassetti S., Bugianesi E., Lenzi M., et al. (2001). Nonalcoholic Fatty Liver Disease. Diabetes 50, 1844–1850. doi:10.2337/diabetes.50.8.1844
Marra F., Svegliati-Baroni G. (2018). Lipotoxicity and the Gut-Liver axis in NASH Pathogenesis. J. Hepatology 68, 280–295. doi:10.1016/j.jhep.2017.11.014
Massa M., Croce S., Campanelli R., Abbà C., Lenta E., Valsecchi C., et al. (2020). Clinical Applications of Mesenchymal Stem/stromal Cell Derived Extracellular Vesicles: Therapeutic Potential of an Acellular Product. Diagnostics 10, 999. doi:10.3390/diagnostics10120999
Matilainen J., Mustonen A.-M., Rilla K., Käkelä R., Sihvo S. P., Nieminen P. (2020). Orotic Acid-Treated Hepatocellular Carcinoma Cells Resist Steatosis by Modification of Fatty Acid Metabolism. Lipids Health Dis. 19, 70. doi:10.1186/s12944-020-01243-5
Mendez-Sanchez N., Cruz-Ramon V., Ramirez-Perez O., Hwang J., Barranco-Fragoso B., Cordova-Gallardo J. (2018). New Aspects of Lipotoxicity in Nonalcoholic Steatohepatitis. Ijms 19, 2034. doi:10.3390/ijms19072034
Momen-Heravi F., Bala S., Bukong T., Szabo G. (2014). Exosome-mediated Delivery of Functionally Active miRNA-155 Inhibitor to Macrophages. Nanomedicine Nanotechnol. Biol. Med. 10, 1517–1527. doi:10.1016/j.nano.2014.03.014
Nobili V., Cutrera R., Liccardo D., Pavone M., Devito R., Giorgio V., et al. (2014). OSAS Affects Liver Histology and Inflammatory Cell Activation in Paediatric NAFLD, Regardless of Obesity/insulin Resistance. Am. J. Respir. Crit. Care Med. 189, 131120192008005–131120192008076. doi:10.1164/rccm.201307-1339OC
Nolan J. P., Duggan E. (2018). Analysis of Individual Extracellular Vesicles by Flow Cytometry. Methods Mol. Biol. 1678, 79–92. doi:10.1007/978-1-4939-7346-0_5
Noureddin M., Sanyal A. J. (2018). Pathogenesis of NASH: the Impact of Multiple Pathways. Curr. Hepatol. Rep. 17, 350–360. doi:10.1007/s11901-018-0425-7
Obata Y., Kita S., Koyama Y., Fukuda S., Takeda H., Takahashi M., et al. (2018). Adiponectin/T-cadherin System Enhances Exosome Biogenesis and Decreases Cellular Ceramides by Exosomal Release. JCI Insight 3. doi:10.1172/jci.insight.99680
Ogese M. O., Jenkins R. E., Adair K., Tailor A., Meng X., Faulkner L., et al. (2019). Exosomal Transport of Hepatocyte‐Derived Drug‐Modified Proteins to the Immune System. Hepatology 70, 1732–1749. doi:10.1002/hep.30701
Ohara M., Ohnishi S., Hosono H., Yamamoto K., Yuyama K., Nakamura H., et al. (2018). Extracellular Vesicles from Amnion-Derived Mesenchymal Stem Cells Ameliorate Hepatic Inflammation and Fibrosis in Rats. Stem Cells Int. 2018, 1–15. doi:10.1155/2018/3212643
Povero D., Eguchi A., Li H., Johnson C. D., Papouchado B. G., Wree A., et al. (2014). Circulating Extracellular Vesicles with Specific Proteome and Liver microRNAs Are Potential Biomarkers for Liver Injury in Experimental Fatty Liver Disease. PLoS One 9, e113651. doi:10.1371/journal.pone.0113651
Povero D., Panera N., Eguchi A., Johnson C. D., Papouchado B. G., de Araujo Horcel L., et al. (2015). Lipid-Induced Hepatocyte-Derived Extracellular Vesicles Regulate Hepatic Stellate Cells via MicroRNA Targeting Peroxisome Proliferator-Activated Receptor-γ. Cell. Mol. Gastroenterology Hepatology 1, 646–663 e4. doi:10.1016/j.jcmgh.2015.07.007
Povero D., Pinatel E. M., Leszczynska A., Goyal N. P., Nishio T., Kim J., et al. (2019). Human Induced Pluripotent Stem Cell-Derived Extracellular Vesicles Reduce Hepatic Stellate Cell Activation and Liver Fibrosis. JCI Insight 4. doi:10.1172/jci.insight.125652
Qu Y., Zhang Q., Cai X., Li F., Ma Z., Xu M., et al. (2017). Exosomes Derived from miR-181-5p-Modified Adipose-Derived Mesenchymal Stem Cells Prevent Liver Fibrosis via Autophagy Activation. J. Cell. Mol. Med. 21, 2491–2502. doi:10.1111/jcmm.13170
Rada P., González-Rodríguez Á., García-Monzón C., Valverde Á. M. (2020). Understanding Lipotoxicity in NAFLD Pathogenesis: Is CD36 a Key Driver? Cell. Death Dis. 11, 802. doi:10.1038/s41419-020-03003-w
Rilla K., Mustonen A.-M., Arasu U. T., Härkönen K., Matilainen J., Nieminen P. (2019). Extracellular Vesicles Are Integral and Functional Components of the Extracellular Matrix. Matrix Biol. 75-76, 201–219. doi:10.1016/j.matbio.2017.10.003
Rinaldi L., Pafundi P. C., Galiero R., Caturano A., Morone M. V., Silvestri C., et al. (2021). Mechanisms of Non-alcoholic Fatty Liver Disease in the Metabolic Syndrome. A Narrative Review. AntioxidantsAntioxidants (Basel) 10, 270. doi:10.3390/antiox10020270
Romero-Gómez M., Zelber-Sagi S., Trenell M. (2017). Treatment of NAFLD with Diet, Physical Activity and Exercise. J. Hepatology 67, 829–846. doi:10.1016/j.jhep.2017.05.016
Rong B., Feng R., Liu C., Wu Q., Sun C. (2019). Reduced Delivery of Epididymal Adipocyte‐derived Exosomal Resistin Is Essential for Melatonin Ameliorating Hepatic Steatosis in Mice. J. Pineal Res. 66, e12561. doi:10.1111/jpi.12561
Roucourt B., Meeussen S., Bao J., Zimmermann P., David G. (2015). Heparanase Activates the Syndecan-Syntenin-ALIX Exosome Pathway. Cell. Res. 25, 412–428. doi:10.1038/cr.2015.29
Safdar A., Saleem A., Tarnopolsky M. A. (2016). The Potential of Endurance Exercise-Derived Exosomes to Treat Metabolic Diseases. Nat. Rev. Endocrinol. 12, 504–517. doi:10.1038/nrendo.2016.76
Smith G. I., Shankaran M., Yoshino M., Schweitzer G. G., Chondronikola M., Beals J. W., et al. (2020). Insulin Resistance Drives Hepatic De Novo Lipogenesis in Nonalcoholic Fatty Liver Disease. J. Clin. Investig. 130, 1453–1460. doi:10.1172/jci134165
Sztolsztener K., Konstantynowicz-Nowicka K., Harasim-Symbor E., Chabowski A. (2021). Time-dependent Changes in Hepatic Sphingolipid Accumulation and PI3K/Akt/mTOR Signaling Pathway in a Rat Model of NAFLD. Ijms 22, 12478. doi:10.3390/ijms222212478
Tang T.-T., Wang B., Lv L.-L., Liu B.-C. (2020). Extracellular Vesicle-Based Nanotherapeutics: Emerging Frontiers in Anti-inflammatory Therapy. Theranostics 10, 8111–8129. doi:10.7150/thno.47865
Tilg H., Adolph T. E., Moschen A. R. (2021). Multiple Parallel Hits Hypothesis in Nonalcoholic Fatty Liver Disease: Revisited after a Decade. Hepatology 73, 833–842. doi:10.1002/hep.31518
Tilg H., Moschen A. R. (2010). Evolution of Inflammation in Nonalcoholic Fatty Liver Disease: the Multiple Parallel Hits Hypothesis. Hepatology 52, 1836–1846. doi:10.1002/hep.24001
Tomita K., Kohli R., MacLaurin B. L., Hirsova P., Guo Q., Sanchez L. H. G., et al. (2017). Mixed-lineage Kinase 3 Pharmacological Inhibition Attenuates Murine Nonalcoholic Steatohepatitis. JCI Insight 2. doi:10.1172/jci.insight.94488
Trajkovic K., Hsu C., Chiantia S., Rajendran L., Wenzel D., Wieland F., et al. (2008). Ceramide Triggers Budding of Exosome Vesicles into Multivesicular Endosomes. Science 319, 1244–1247. doi:10.1126/science.1153124
Tsuchida T., Friedman S. L. (2017). Mechanisms of Hepatic Stellate Cell Activation. Nat. Rev. Gastroenterol. Hepatol. 14, 397–411. doi:10.1038/nrgastro.2017.38
van Niel G., D'Angelo G., Raposo G. (2018). Shedding Light on the Cell Biology of Extracellular Vesicles. Nat. Rev. Mol. Cell. Biol. 19, 213–228. doi:10.1038/nrm.2017.125
Wang L., Wang Y., Quan J. (2020). Exosomes Derived from Natural Killer Cells Inhibit Hepatic Stellate Cell Activation and Liver Fibrosis. Hum. Cell. 33, 582–589. doi:10.1007/s13577-020-00371-5
Wang R., Ding Q., Yaqoob U., de Assuncao T. M., Verma V. K., Hirsova P., et al. (2015). Exosome Adherence and Internalization by Hepatic Stellate Cells Triggers Sphingosine 1-phosphate-dependent Migration. J. Biol. Chem. 290, 30684–30696. doi:10.1074/jbc.M115.671735
Wang Z., Zhao X., Chen S., Wang Y., Cao L., Liao W., et al. (2021). Associations between Nonalcoholic Fatty Liver Disease and Cancers in a Large Cohort in China. Clin. Gastroenterology Hepatology 19, 788–796 e4. doi:10.1016/j.cgh.2020.05.009
Watanabe T., Tsuchiya A., Takeuchi S., Nojiri S., Yoshida T., Ogawa M., et al. (2020). Development of a Non-alcoholic Steatohepatitis Model with Rapid Accumulation of Fibrosis, and its Treatment Using Mesenchymal Stem Cells and Their Small Extracellular Vesicles. Regen. Ther. 14, 252–261. doi:10.1016/j.reth.2020.03.012
Weisberg S. P., McCann D., Desai M., Rosenbaum M., Leibel R. L., Ferrante A. W. (2003). Obesity Is Associated with Macrophage Accumulation in Adipose Tissue. J. Clin. Investig. 112, 1796–1808. doi:10.1172/jci1924610.1172/jci200319246
Whitham M., Parker B. L., Friedrichsen M., Hingst J. R., Hjorth M., Hughes W. E., et al. (2018). Extracellular Vesicles Provide a Means for Tissue Crosstalk during Exercise. Cell. Metab. 27, 237–251 e4. doi:10.1016/j.cmet.2017.12.001
Wiklander O. P. B., Brennan M. Á., Lötvall J., Breakefield X. O., El Andaloussi S. (2019). Advances in Therapeutic Applications of Extracellular Vesicles. Sci. Transl. Med. 11. doi:10.1126/scitranslmed.aav8521
Witwer K. W., Buzás E. I., Bemis L. T., Bora A., Lässer C., Lötvall J., et al. (2013). Standardization of Sample Collection, Isolation and Analysis Methods in Extracellular Vesicle Research. J. Extracell. Vesicles 2, 20360. doi:10.3402/jev.v2i0.20360
Wu J., Dong T., Chen T., Sun J., Luo J., He J., et al. (2020). Hepatic Exosome-Derived miR-130a-3p Attenuates Glucose Intolerance via Suppressing PHLPP2 Gene in Adipocyte. Metabolism 103, 154006. doi:10.1016/j.metabol.2019.154006
Wu Y., Zheng Q., Zou B., Yeo Y. H., Li X., Li J., et al. (2020). The Epidemiology of NAFLD in Mainland China with Analysis by Adjusted Gross Regional Domestic Product: a Meta-Analysis. Hepatol. Int. 14, 259–269. doi:10.1007/s12072-020-10023-3
Xiao F., Yu J., Liu B., Guo Y., Li K., Deng J., et al. (2014). A Novel Function of microRNA 130a-3p in Hepatic Insulin Sensitivity and Liver Steatosis. Diabetes 63, 2631–2642. doi:10.2337/db13-1689
Xu R., Greening D. W., Rai A., Ji H., Simpson R. J. (2015). Highly-purified Exosomes and Shed Microvesicles Isolated from the Human Colon Cancer Cell Line LIM1863 by Sequential Centrifugal Ultrafiltration Are Biochemically and Functionally Distinct. Methods 87, 11–25. doi:10.1016/j.ymeth.2015.04.008
Xu R., Rai A., Chen M., Suwakulsiri W., Greening D. W., Simpson R. J. (2018). Extracellular Vesicles in Cancer - Implications for Future Improvements in Cancer Care. Nat. Rev. Clin. Oncol. 15, 617–638. doi:10.1038/s41571-018-0036-9
Xue J., Schmidt S. V., Sander J., Draffehn A., Krebs W., Quester I., et al. (2014). Transcriptome-based Network Analysis Reveals a Spectrum Model of Human Macrophage Activation. Immunity 40, 274–288. doi:10.1016/j.immuni.2014.01.006
Yan C., Tian X., Li J., Liu D., Ye D., Xie Z., et al. (2021). A High-Fat Diet Attenuates AMPK α1 in Adipocytes to Induce Exosome Shedding and Nonalcoholic Fatty Liver Development In Vivo. Diabetes 70, 577–588. doi:10.2337/db20-0146
Ying W., Riopel M., Bandyopadhyay G., Dong Y., Birmingham A., Seo J. B., et al. (2017). Adipose Tissue Macrophage-Derived Exosomal miRNAs Can Modulate In Vivo and In Vitro Insulin Sensitivity. Cell. 171, 372–384. e12. doi:10.1016/j.cell.2017.08.035
Younossi Z. M., Koenig A. B., Abdelatif D., Fazel Y., Henry L., Wymer M. (2016). Global Epidemiology of Nonalcoholic Fatty Liver Disease-Meta-Analytic Assessment of Prevalence, Incidence, and Outcomes. Hepatology 64, 73–84. doi:10.1002/hep.28431
Younossi Z., Tacke F., Arrese M., Chander Sharma B., Mostafa I., Bugianesi E., et al. (2019). Global Perspectives on Nonalcoholic Fatty Liver Disease and Nonalcoholic Steatohepatitis. Hepatology 69, 2672–2682. doi:10.1002/hep.30251
Zhang J., Tan J., Wang M., Wang Y., Dong M., Ma X., et al. (2021). Lipid-induced DRAM Recruits STOM to Lysosomes and Induces LMP to Promote Exosome Release from Hepatocytes in NAFLD. Sci. Adv. 7, eabh1541. doi:10.1126/sciadv.abh1541
Zhao H., Shang Q., Pan Z., Bai Y., Li Z., Zhang H., et al. (2018). Exosomes from Adipose-Derived Stem Cells Attenuate Adipose Inflammation and Obesity through Polarizing M2 Macrophages and Beiging in White Adipose Tissue. Diabetes 67, 235–247. doi:10.2337/db17-0356
Keywords: extracellular vesicles, lipids, inflammation, pathogenesis, treatment, metabolic associated fatty liver disease
Citation: Sun J, Zhang D and Li Y (2022) Extracellular Vesicles in Pathogenesis and Treatment of Metabolic Associated Fatty Liver Disease. Front. Physiol. 13:909518. doi: 10.3389/fphys.2022.909518
Received: 01 April 2022; Accepted: 23 May 2022;
Published: 13 June 2022.
Edited by:
Henrique Girao, University of Coimbra, PortugalReviewed by:
Ranjitsinh V. Devkar, Maharaja Sayajirao University of Baroda, IndiaBaihai Jiao, University of Connecticut Health Center, United States
Copyright © 2022 Sun, Zhang and Li. This is an open-access article distributed under the terms of the Creative Commons Attribution License (CC BY). The use, distribution or reproduction in other forums is permitted, provided the original author(s) and the copyright owner(s) are credited and that the original publication in this journal is cited, in accordance with accepted academic practice. No use, distribution or reproduction is permitted which does not comply with these terms.
*Correspondence: Yiling Li, bHlsLTcyQDE2My5jb20=; Dianbao Zhang, emhhbmdkaWFuYmFvQGdtYWlsLmNvbQ==