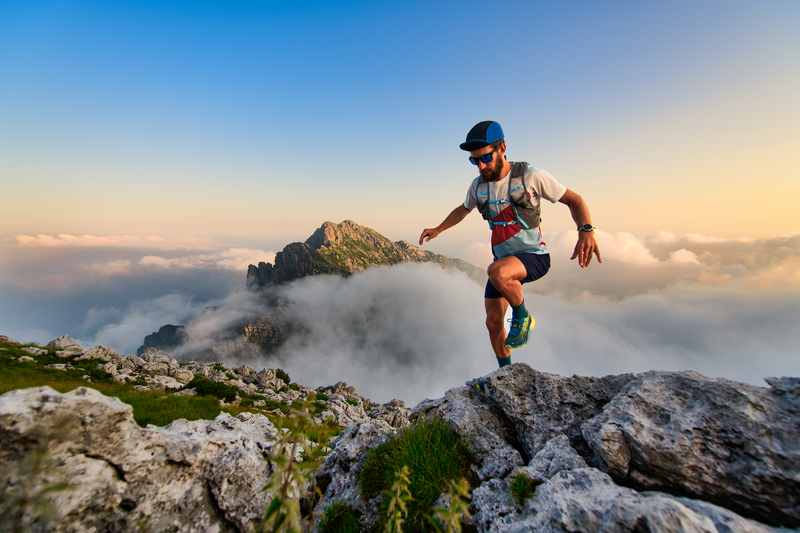
95% of researchers rate our articles as excellent or good
Learn more about the work of our research integrity team to safeguard the quality of each article we publish.
Find out more
REVIEW article
Front. Physiol. , 14 June 2022
Sec. Exercise Physiology
Volume 13 - 2022 | https://doi.org/10.3389/fphys.2022.898395
This article is part of the Research Topic Oxygen Delivery:Demand Matching in Exercising Skeletal Muscle View all 7 articles
Skeletal muscle is one of the most dynamic metabolic organs as evidenced by increases in metabolic rate of >150-fold from rest to maximal contractile activity. Because of limited intracellular stores of ATP, activation of metabolic pathways is required to maintain the necessary rates of ATP re-synthesis during sustained contractions. During the very early phase, phosphocreatine hydrolysis and anaerobic glycolysis prevails but as activity extends beyond ∼1 min, oxidative phosphorylation becomes the major ATP-generating pathway. Oxidative metabolism of macronutrients is highly dependent on the cardiovascular system to deliver O2 to the contracting muscle fibres, which is ensured through a tight coupling between skeletal muscle O2 utilization and O2 delivery. However, to what extent O2 delivery is ideal in terms of enabling optimal metabolic and contractile function is context-dependent and determined by a complex interaction of several regulatory systems. The first part of the review focuses on local and systemic mechanisms involved in the regulation of O2 delivery and how integration of these influences the matching of skeletal muscle O2 demand and O2 delivery. In the second part, alterations in cardiovascular function and structure associated with aging and heart failure, and how these impact metabolic and contractile function, will be addressed. Where applicable, the potential of exercise training to offset/reverse age- and disease-related cardiovascular declines will be highlighted in the context of skeletal muscle metabolic function. The review focuses on human data but also covers animal observations.
Skeletal muscle is one of the most dynamic metabolic organs. Metabolic rate can increase from values at rest of ∼0.02 up to ∼3.7 mmol ATP kg−1 s−1 during maximal intensity exercise (Hargreaves and Spriet, 2020). Given the relatively small intracellular stores of ATP (∼5 mmol kg−1), activation of metabolic pathways is required to maintain the necessary rates of ATP resynthesis during sustained contractile activity. These pathways include phosphocreatine and glycogen breakdown that allows for substrate-level phosphorylation (anaerobic) and oxidative phosphorylation (aerobic). The latter becomes increasingly important as the duration of activity increases and oxidative phosphorylation is already the major ATP-generating pathway when contractile activity extends beyond ∼1 min.
Oxidative metabolism of carbohydrate and fat is highly dependent on the ability of the cardiovascular system to deliver O2 to the contracting muscle fibres. In skeletal muscle, convective O2 delivery, which refers to the active movement of O2 in blood, determines the rates of O2 that enters the muscle. At the microcirculatory level, O2 diffuses across the capillary wall, interstitium and sarcolemma (diffusive O2 transport). Hence, the number (total surface area) and spatial distribution of capillaries within the muscle, rate of red blood cell entry and red cell velocity in each capillary, and O2 saturation are important factors for O2 transfer to, and utilization by, mitochondria (Egginton and Gaffney, 2010; Mendelson et al., 2021).
The increase in blood flow to contracting skeletal muscle is achieved through local vasodilation that is the result of a complex interplay between vasoconstrictor and vasodilator signalling (Joyner and Casey, 2015). Reports on maximal skeletal muscle blood flow during exercise in different species including humans have demonstrated values in the range of 2–4 L kg tissue−1 depending on species, fibre type composition, and training status (Armstrong and Laughlin, 1983; Andersen and Saltin, 1985; Richardson et al., 1995; Mortensen et al., 2008; Joyner and Casey, 2015). As skeletal muscle comprises ∼30%–40% of the total body mass, intense exercise engaging a large proportion of the total muscle mass will pose a threat to arterial blood pressure as maximal cardiac output is insufficient to offset the reduction in peripheral vascular resistance (Calbet et al., 2004; Rowell, 2004). Consequently, a reduction in perfusion and O2 delivery to the contracting muscles obtained through sympathetic constraint of vasodilation is needed to maintain arterial blood pressure and ensure sufficient perfusion of vital organs such as the brain (Rowell, 2004; Joyner and Casey, 2015).
Advancing age is associated with a broad range of alterations in the cardiovascular system such as augmented arterial stiffness that increases afterload on the left ventricle, systolic blood pressure, left ventricular mass, and reduced left ventricular diastolic performance (Lakatta and Levy, 2003b, a; Fleg and Strait, 2012). At the level of skeletal muscle, declines in skeletal muscle mass, endothelial and mitochondrial function, and reduced capillarization and blood flow to exercising muscle are all hallmarks of aging (Nyberg and Hellsten, 2016). These changes in the cardiovascular and skeletal muscle systems are, however, not unique for the aging process, as they are also manifested in a broad range of cardiovascular diseases such as different forms of heart failure. Importantly, many biological alterations that come with advancing age and cardiovascular diseases are the result of a complex interplay between systems that are influenced by genetic and life-style factors. Regarding the latter, one important factor is physical activity due to its profound effects on cardiovascular structure and function, and physical inactivity induces many of the cardiovascular changes associated with aging (Saltin et al., 1968; McGuire et al., 2001a, b).
The present review is composed of two parts. In the first part, local and systemic mechanisms involved in the regulation of O2 delivery and how integration of these influences matching of skeletal muscle O2 demand and O2 delivery will be addressed. Here, the discussions will be centred around exercise engaging a small muscle mass in the form of handgrip and knee-extensor exercise, and those engaging larger proportions of the total mass such as cycling, running, rowing, and cross-country skiing. It should be noted that the physiological responses discussed in this first section represents that of younger (∼18–35 years) subjects in which no significant age-related alterations in cardiovascular function are expected. Furthermore, since most of the studied subjects have been males, one should be cautious when interpreting and generalising the findings. In the second part, the focus will be on how aging and heart failure lead to in part common alterations in cardiovascular structure and function, and how these impact metabolic and contractile function of skeletal muscle. In addition, the potential of exercise training to offset/reverse age- and disease-related cardiovascular declines will be highlighted in the context of skeletal muscle metabolic function.
The matching of skeletal muscle O2 utilization through mitochondrial oxidative phosphorylation and O2 delivery can be assessed through experiments in which O2 delivery is manipulated and can be understood with reference to the “tipping point” hypothesis of Poole et al. (2008) (Figure 1). In such experiments, if an increase or decrease in the rate of O2 delivery does not lead to a change in the rate of oxidative metabolism, this suggests that O2 availability in the control condition is in excess of demand, i.e., that the control condition is situated on the “flat portion” of the relationship between the rate of O2 utilization and the rate of O2 delivery. Conversely, if an increase in the rate of O2 delivery enhances the rate of O2 consumption or speeds O2 uptake kinetics following the onset of exercise, mitochondrial oxidative phosphorylation is likely to be limited by O2 availability. In a setting where a reduction in O2 delivery reduces the rate of O2 utilisation or slows O2 uptake kinetics following the onset of exercise, O2 supply in the control state would not necessarily be inadequate but could be considered to be very closely matched to O2 demand, i.e., to be situated “right on” the tipping point. In addition to the rate of O2 consumption, changes in the rate of substrate-level phosphorylation (evidenced, for example, by changes in muscle phosphocreatine and lactate concentrations), force output, and the ability to perform a given task will also provide essential insight into the extent to which oxidative metabolism is affected by manipulation of O2 delivery.
FIGURE 1. Model demonstrating the effects of altering the rate of O2 delivery on skeletal muscle O2 utilization. If an increase or decrease in the rate of O2 delivery does not lead to a change in the rate of oxidative metabolism, this suggests that O2 availability in the control condition is in excess of demand (flat portion’ of the relationship between the rate of O2 utilization and the rate of O2 delivery). Conversely, if an increase in the rate of O2 delivery enhances the rate of O2 consumption or speeds O2 uptake kinetics following the onset of exercise, mitochondrial oxidative phosphorylation is likely to be limited by O2 availability. In a situation where a reduction in O2 delivery reduces the rate of O2 utilisation or slows O2 uptake kinetics following the onset of exercise, O2 supply in the control state would not necessarily be inadequate but could be very closely matched to O2 demand (right on the tipping point). Note that aging and HF shifts the operating domain to the left on the continuum.
There are several ways of experimentally increasing perfusion of skeletal muscle. By arterial infusion of a vasodilator substance and by increasing perfusion pressure through changes in hydrostatic pressure (e.g., arm positioned below vs. above the level of the heart), blood flow and O2 delivery to the contracting muscle can be enhanced. Notably, although breathing hyperoxic air increases blood O2 content, this does typically not increase the rate of O2 delivery due to reduction in blood flow to maintain the rate of O2 delivery (Gonzalez-Alonso et al., 2002). Reductions in skeletal muscle O2 delivery can be obtained by arterial infusion of a vasoconstrictor substance and by increasing sympathetic nervous activity and constraint of vasodilation. By introducing hypoxia, large reductions in arterial O2 content can be achieved. As also discussed later, a compensatory increase in blood flow that allows O2 delivery to be maintained is observed in some circumstances. In the following sections, various models in which the O2 delivery has been experimentally altered will be used to provide insight into how skeletal muscle O2 demand and delivery are matched.
The initial phase of exercise is characterized by a rapid increase in O2 delivery to meet the higher metabolic demand of the contracting muscle. During moderate-intensity knee-extensor exercise, which engages 2–3 kg of muscle mass, the increase in O2 delivery in the initial phase appears to supersede oxidative metabolism. This may in part relate to mechanical factors, as deformation obtained via high extravascular pressure (Kirby et al., 2007) and passive limb movement (Mortensen et al., 2012a) have been shown to elicit rapid vasodilation that decreases over time. This mechanically induced vasodilation could serve as a feed-forward mechanism during the very early phase of exercise where metabolic vasodilation is less prominent. Such a mechanism is in line with the observation that the difference between skeletal muscle O2 delivery and O2 uptake is greatest in the initial phase of exercise (Nyberg et al., 2010), although it is important to underscore that this relationship may also be reflective of a potential hyperperfusion in areas of the muscle that are inactive as well as in non-recruited muscles. Nevertheless, a perfusion limitation appears to be unlikely as a reduction in exercise-induced leg blood flow and O2 delivery by ∼25%–45%, obtained through pharmacological inhibition of nitric oxide synthase (NOS) and cyclooxygenase (COX), does not alter skeletal muscle O2 uptake due to compensatory increase in O2 extraction (Nyberg et al., 2010). It should be noted that the contraction-induced change in blood flow in this study did not appear to be affected by the pharmacological blockade (as the difference was present during passive movement of the leg) and future studies should address how significant alterations in blood flow kinetics may affect the rate of O2 consumption.
As opposed to moderate-intensity exercise where predominantly slow-twitch (ST) fibres are engaged, both ST and fast-twitch (FT) fibres are recruited during more intense exercise (Krustrup et al., 2004). In humans, FT fibres have a lower oxidative capacity than ST fibres (Essen-Gustavsson and Henriksson, 1984) and the rise in skeletal muscle O2 uptake is also slowed in the transition from moderate to intense knee-extensor exercise (Nyberg et al., 2021). Additionally, O2 delivery relative to leg O2 utilization appears to be reduced in an intensity-dependent manner (Nyberg et al., 2010; Christensen et al., 2013; Nyberg et al., 2014). Hence, a “tipping point” regarding O2 delivery beyond which O2 uptake kinetics become progressively slowed with further reductions in O2 delivery may exist during intense exercise (Poole et al., 2008). Similar to findings during moderate-intensity knee-extensor exercise (Nyberg et al., 2010), pharmacological inhibition of NOS and COX reduced exercise-induced skeletal muscle O2 delivery by ∼25%–50% in the initial phase of intense knee-extensor exercise (∼86% of incremental test peak power) (Christensen et al., 2013). In this setting, however, the rise in skeletal muscle O2 uptake was slowed despite a higher O2 extraction. This finding suggests that FT fibres are more sensitive to a reduction in O2 delivery than ST fibres but also emphasizes that a marked reduction in O2 availability cannot be tolerated at higher intensities. To gain more clarity on how close to the tipping point the muscle was operating, experiments in which O2 delivery are reduced to a lesser extent are warranted. Notably, increasing blood flow and O2 delivery ∼2-fold by arterial ATP infusion in a similar bout of intense knee-extensor exercise does not increase skeletal muscle O2 uptake in the initial phase of exercise (Nyberg et al., 2014), suggesting that O2 availability still matches or exceeds mitochondrial capacity for oxidative phosphorylation in the control situation.
During continuous knee-extensor exercise, skeletal muscle blood flow and O2 delivery is closely matched to the rate of O2 consumption across the full intensity spectrum (Andersen and Saltin, 1985; Mortensen et al., 2008). This matching, which is the result of a complex interplay between the sympathetic nervous system and local vasoactive systems (Hellsten and Nyberg, 2015; Joyner and Casey, 2015), is important to secure adequate O2 availability in the contracting fibres. One example of this is in hypoxic conditions where reductions in arterial O2 content by up to ∼25% is compensated by an increase in blood flow that allows O2 delivery and utilization to be maintained at moderate intensities (Koskolou et al., 1997; Gonzalez-Alonso et al., 2002; Mortensen et al., 2011). The importance of this matching between O2 supply and utilization is further underscored by the great degree of redundancy that exists between vasodilator systems regulating skeletal muscle blood flow that allows for preservation of blood flow in conditions where one or more vasoactive systems may be compromised (Hellsten and Nyberg, 2015; Joyner and Casey, 2015). At moderate intensity, there even appears to be hyperperfusion of the exercising knee-extensors as a pharmacologically induced reduction in leg blood flow and O2 delivery of ∼10%–25% is compensated by increased O2 extraction so that skeletal muscle O2 utilization is maintained (Nyberg et al., 2010; Mortensen et al., 2012b; Nyberg et al., 2015a).
During sustained intense knee-extensor exercise, skeletal muscle perfusion appears to more closely match O2 demand. Combined inhibition of NOS and COX, which reduces exercise-induced blood flow and O2 delivery by ∼20% during moderate-intensity exercise (Nyberg et al., 2010), did not reduce O2 delivery and utilization despite pronounced effects on these variables in the initial phase of exercise (Christensen et al., 2013). The mechanism underlying the preservation of blood flow is likely to involve compensatory contribution from redundant vasodilator systems that were activated, which may have been a direct effect of insufficient skeletal muscle O2 availability. Interestingly, when blood flow was increased through arterial ATP infusion during intense exercise, O2 uptake was found to be reduced after 30 s and until predetermined exercise termination at 4 min (Nyberg et al., 2014). This observation may relate to the capacity of intravascular ATP to override sympathetic vasoconstrictor activity (Rosenmeier et al., 2004; Mortensen et al., 2012b). During exercise, sympathetic activity reduces perfusion of inactive muscles whereas this effect is blunted in contracting muscle (termed functional sympatholysis), thus directing blood flow away from areas of lower metabolic activity and toward areas of higher metabolic demand (Remensnyder et al., 1962; Saltin and Mortensen, 2012). Importantly, the vasoconstrictor effects of muscle sympathetic nervous activity are not abolished (Remensnyder et al., 1962; Buckwalter et al., 2001), and sympathetic restraint of blood flow remains even in highly active skeletal muscle (Joyner et al., 1992; Buckwalter and Clifford, 2001). In the setting of ATP-induced vasodilation in regions under sympathetic vasoconstrictor control, the precise matching of O2 delivery and demand at the microvascular level may be disturbed as selective and controlled perfusion of vessels supplying regions in need of O2 is required for optimal tissue oxygenation (Sprague et al., 2010; Ellsworth et al., 2016). Given the very high blood flow rates (∼50% above control), mean transit time in capillaries perfusing highly active fibres may also have reached levels that do not allow for sufficient O2 off-loading from haemoglobin.
Taken collectively, the above findings highlight the close matching that exist between skeletal muscle O2 delivery and oxidative metabolism and indicate that the knee-extensor muscles may be operating closer to a tipping point with regards to O2 delivery with increasing exercise intensity; however, the regulation of O2 consumption still resides at the level of the mitochondria.
Hand gripping is evidently a small muscle mass exercise as <1 kg of muscle is being activated. However, as compared to the locomotor muscles of the lower extremities, the muscles in the forearm serve different functions such as ensuring very precise and coordinated movements. Furthermore, forearm muscles are subjected to lower hydrostatic pressures relative to the legs and they also display lower arterial wall thickness (Astrand et al., 2003), reduced adrenergic responsiveness (Pawelczyk and Levine, 2002; Nyberg et al., 2018), and enhanced response to vasodilator substances (Proctor and Newcomer, 2006). These structural and functional differences could entail differences in the matching of skeletal muscle O2 delivery and O2 demand. In accordance with this proposition, exercising with the arm below compared to above the level of the heart to augment perfusion pressure, is associated with increased blood flow and skeletal muscle O2 uptake in the initial phase of moderate-intensity exercise (Hughson et al., 1996). In a more recent study using a combination of diffuse correlation spectroscopy and near-infrared spectroscopy (NIRS), exercising with the arm above the heart was associated with slower blood flow kinetics, however, the observed ∼25% slower O2 uptake kinetics did not reach statistical significance (Tucker et al., 2019). By using a different experimental setup, skeletal muscle blood flow and O2 uptake was found to rise more rapidly when mean arterial blood pressure (MAP) was increased through stimulation of chemoreflexes in the calf muscles (Perrey et al., 2001). Furthermore, in a series of experiments in which prior exercise and/or ischemia were applied prior to intense exercise, a strong correlation between the rates of increase in blood flow and oxidative metabolism were observed (Faisal et al., 2010), suggesting that the acceleration of the skeletal muscle O2 uptake at the onset of intense forearm exercise is linked to O2 delivery.
In contrast to the apparent perfusion limitation across the intensity continuum at the onset of forearm exercise, some degree of surplus in O2 availability may exist during steady state conditions performed at moderate intensity. Evidence from this comes from steady state exercise, where a combination of hypoxia and pharmacological inhibition of NOS and COX, that led to a ∼10% reduction in O2 delivery, was associated with increased O2 extraction and preserved O2 uptake (Crecelius et al., 2011). Somewhat in line with this observation, a ∼30% increase in convective O2 delivery obtained through increased perfusion pressure was not associated with enhanced skeletal muscle O2 uptake at steady state, although a trend toward a higher value was detected (Tucker et al., 2019). It should also be noted that intra-arterial infusion of ATP has been shown to have an additive effect on exercise hyperaemia during moderate intensity exercise (Shepherd et al., 2016) and infusion of ATP at a rate sufficient to double resting blood flow, has no effect on the amount and rate of blood-debt repayment detected after exercise (Patterson and Shepherd, 1954). During more intense exercise, blood flow and O2 uptake appears to reach a plateau at ∼80% of maximal workload (Nyberg et al., 2017). This finding may indicate that O2 delivery is limiting oxidative metabolism during intense forearm exercise, but more evidence is needed to support this.
Skeletal muscle has substantial vasodilator capacity as evidenced by reports of maximal blood flow values of 3–4 L kg−1 min−1 in humans during isolated muscle contractions (Andersen and Saltin, 1985; Richardson et al., 1995). During exercise that engages a large muscle mass, this ability of skeletal muscle to vasodilate can potentially outstrip cardiac output, which will then pose a threat to mean arterial pressure that needs to be maintained at ∼100 mmHg to secure perfusion of vital organs such as the brain (Calbet et al., 2004). Based on this great vasodilator capacity, skeletal muscle has also been described as the “sleeping giant” (Rowell, 2004). In addition to increases in cardiac output, enhanced sympathetic activation elicits vasoconstriction in less active tissues and constraint of vasodilation in more active tissues to maintain total peripheral resistance and blood pressure (Buckwalter and Clifford, 2001; Hansen et al., 2020) and redirect blood flow from less metabolically active tissues to the exercising muscles (Perko et al., 1998). This was elegantly demonstrated in a study of cross-country skiers in which values for maximal vasodilation (vascular conductance) of legs and arms were obtained. In these subjects who are characterised by highly trained upper and lower extremities, maximal vasodilation of both arms and legs would lead to a drop in mean arterial pressure to ∼75 mmHg; however, pressure remained at ∼95 mmHg during maximal exercise due to peripheral constraint of vasodilation that reduced the need for cardiac output by ∼15% (Calbet et al., 2004).
In the kidney and liver, sympathetic vasoconstriction can reduce perfusion by ∼75% during intense exercise in humans, thus allowing for ∼10% of maximal cardiac output (∼2 L min−1) to be redistributed (Joyner and Casey, 2015). In active skeletal muscle, the magnitude of decrease in vascular conductance and blood flow is dependent on muscle sympathetic nervous activity, metabolic activity, and the ability of the muscle for functional sympatholysis with respiratory muscles being less affected than limb skeletal muscles (Remensnyder et al., 1962; Saltin, 2007; Saltin and Mortensen, 2012). Depending on the extent of vascular restraint, matching of skeletal muscle O2 delivery and demand may be altered to an extent that will affect metabolic performance as discussed in the following sections.
Cycling and running can be defined as large muscle mass exercise as these types of activity recruits ∼50% of the total mass. During cycling performed at submaximal intensities, evidence supports that the limitation to skeletal muscle oxidative metabolism resides at the level of the mitochondria both at onset of exercise as well as during steady state conditions. For instance, application of lower body negative pressure, which is known to lead to a significant reduction in leg blood flow during exercise, does not change pulmonary oxygen kinetics during moderate and heavy intensity cycling (Williamson et al., 1996). During steady state conditions, decreasing cardiac output and leg blood flow by use of β-adrenergic blockade lead to a compensatory increase in O2 extraction to maintain leg O2 uptake during cycling performed at ∼40%–84% of maximal O2 uptake (Pawelczyk et al., 1992).
During very intense maximal cycling exercise eliciting maximal O2 uptake, systemic and leg O2 delivery and utilization fail to sufficiently rise to meet the increase in metabolic demand, contributing to task failure (Gonzalez-Alonso and Calbet, 2003; Mortensen et al., 2005; Mortensen et al., 2008; Trinity et al., 2012). The blunting of cardiac output is associated with the attainment of maximal heart rate and a plateau or even a decrease in stroke volume (Gonzalez-Alonso and Calbet, 2003; Mortensen et al., 2005; Mortensen et al., 2008; Trinity et al., 2012) that is likely to reflect restrictions in ventricular filling (Munch et al., 2014). This central limitation changes the site of regulation of skeletal muscle O2 consumption from mitochondrial respiration to convective O2 delivery (Levine, 2008; Skattebo et al., 2020). This shift is supported by very low femoral venous O2 saturation levels that range from ∼15% in habitually active (Mortensen et al., 2005; Mortensen et al., 2008) and down to 8% in well-trained individuals (Gonzalez-Alonso and Calbet, 2003; Munch et al., 2014) during maximal intensity cycling. Of note, the remaining O2 in the venous drainage is likely to reflect perfusion of less active tissues such as the skin and bone as well as a diffusion limitation across the capillary wall, interstitium, and sarcolemma given the expected low capillary PO2 in regions of very high metabolic activity (Skattebo et al., 2020). In line with this central limitation, ex vivo measurements have demonstrated a 2-fold higher mitochondrial capacity relative to maximal in vivo O2 uptake (Boushel and Saltin, 2013). Moreover, 3–8 weeks of endurance training leads to improvements in maximal O2 uptake that are driven primarily by increases in systemic O2 delivery (Montero et al., 2015). However, it should be emphasized that peripheral adaptations are also evident and these are needed as a response to the training-induced increases in cardiac output and skeletal muscle blood flow (Hellsten and Nyberg, 2015).
In summary, a tipping point for O2 delivery is apparent during very intense and maximal aerobic cycling with critical power/speed typically being at around 80%–90% of VO2max. Perfusion during lower intensities is of a magnitude that allows for compensatory O2 extraction in situations where blood flow is compromised.
A commonly used approach to study the effects of exercising with a large muscle mass on systemic and local hemodynamics is the combined arm and leg model. In a meta-analysis, blood flow to the leg was found to decline ∼10% when higher intensities of arm exercise was superimposed on leg exercise (Secher and Volianitis, 2006). In this setting of reduced O2 delivery, a compensatory increase in O2 extraction to maintain O2 uptake is a general finding (Savard et al., 1989; Richter et al., 1992; Richardson et al., 1995) although one finding of reduced O2 uptake has been reported (Secher et al., 1977). A decline in skeletal muscle perfusion is also apparent in the arms when a large muscle mass is recruited, as evidenced by reductions in blood flow to the arms during combined arm and leg exercise. However, in contrast to the legs, the increase in O2 extraction is insufficient to maintain oxidative metabolism in arm muscles (Volianitis and Secher, 2002; Volianitis et al., 2003; Volianitis et al., 2004).
The reduction in O2 utilization in the face of a decline in O2 delivery in the arms during combined exercise agrees with the previously discussed observations from studies utilizing handgrip exercise in which O2 supply appears to be limiting oxidative metabolism both at the onset of exercise as well as during intense sustained contractions. Furthermore, a training-induced increase in peak arm muscle O2 uptake during arm-cranking was also demonstrated to be an effect of increases in convective and diffuse O2 transport (Boushel et al., 2014). It should be noted that during maximal exercise in untrained individuals, O2 saturation in the venous drainage of the legs (femoral vein) reaches levels of ∼15% (Mortensen et al., 2005; Mortensen et al., 2008), whereas venous blood returning from the arms (subclavian vein) remains 40% saturated (Volianitis et al., 2004). In well-trained cross-country skiers performing maximal diagonal skiing involving both arms and legs, venous O2 saturation in the subclavian vein has been reported to be ∼14%, however, femoral venous O2 saturation levels were still significantly lower at ∼7% (Calbet et al., 2005). This lower capacity for O2 extraction in the arms is likely to reflect higher heterogeneity in blood flow distribution, shorter mean transit time, smaller diffusing area, and larger diffusing distance (Calbet et al., 2005).
Taken collectively, the findings from the various types of exercise suggest that leg skeletal muscle hemodynamics and vascular network architecture allows for greater increases in O2 extraction without compromising skeletal muscle O2 consumption across the entire intensity and recruited muscle mass continuum (Figure 2). Given the locomotor function of the legs, it is not surprising that evolution would favour such a physiological system in which multiple redundant systems secure sufficient O2 delivery to muscles involved in movement.
FIGURE 2. Regulation of skeletal muscle O2 delivery to match oxidative metabolism and maintain arterial blood pressure. During exercise engaging a small muscle mass such as handgrip and knee-extensor exercise, skeletal muscle blood flow and O2 delivery is closely matched to the metabolic demands of contraction. Here, the magnitude of perfusion allows for an increased O2 extraction so that skeletal muscle O2 utilization is maintained even in the setting of reduced blood flow, thus placing the limitation for oxidative metabolism at the level of the mitochondria. This hyperperfusion is most prominent in the lower extremities. During intense exercise engaging a large proportion of the total muscle mass, arterial pressure may be compromised as maximal cardiac output is insufficient to offset the reduction in peripheral vascular resistance. Consequently, vasoconstriction is needed in the active muscles to maintain arterial blood pressure to ensure sufficient perfusion of vital organs such as the brain, thus reducing perfusion and O2 delivery to the contracting muscles. In this setting, leg skeletal muscle hemodynamics and vascular network architecture allows for greater increases in O2 extraction compared to arm muscles. In both upper and lower extremities, the limitation to skeletal muscle oxidative metabolism during very intense/maximal exercise resides at the level of systemic and local convective O2 transport.
Aging poses the largest risk factor for cardiovascular disease (North and Sinclair, 2012). Pathological alterations such as cardiac hypertrophy, altered left ventricular (LV) diastolic function, diminished LV systolic reverse capacity, increased arterial stiffness, and impaired endothelial function are all associated with the aging process (Lakatta and Levy, 2003b, a). In skeletal muscle, declines in muscle mass, force generation, endothelial and mitochondrial function, and capillarization are all hallmarks of aging and these alterations could potentially underlie the lower exercise-induced blood flow that has been reported in some studies (Kirby et al., 2009; Nyberg and Hellsten, 2016). Hence, both systemic and peripheral limitations may affect skeletal muscle metabolic performance in older individuals.
In older individuals, contraction-induced rapid-onset vasodilation as well as blood flow and vasodilator kinetics are slowed in forearm muscles (Casey and Joyner, 2012; Casey et al., 2015; Hughes et al., 2018). This blunted hemodynamic response has been proposed to be caused by blunted NO signaling (Casey et al., 2015). During sustained contractions, forearm vascular conductance and blood flow have similarly been described to be lower in aged individuals with more pronounced reductions at higher intensities (Jasperse et al., 1994; Kirby et al., 2012; Richards et al., 2014; Casey et al., 2015). This reduction in exercise hyperaemia have been reported to be related to less exercise-induced NO- and prostanoid-mediated vasodilatation, increased sympathetic outflow, and impaired functional sympatholysis (Taylor et al., 1992; Dinenno et al., 2005; Schrage et al., 2007). Despite the apparent alterations in skeletal muscle O2 delivery it remains unclear to what extent the rate of O2 consumption is affected as most studies have focused on regulation of vascular tone and blood flow. In one study, blood flow was found to be reduced with no compensatory increase in O2 extraction. This led to a lowering of skeletal muscle O2 utilisation by ∼7%–17% (at 5%–25% MVC), however, this did not reach statistical significance (Kirby et al., 2012). A potential effect on O2 uptake is supported by observations made with acute ascorbic acid supplementation that increased skeletal muscle blood flow and O2 uptake in older individuals (Richards et al., 2015). As previously discussed, forearm muscles operate close to the tipping point in terms of O2 delivery in healthy individuals. Hence, it is very plausible that age-related impairments in convective O2 transport could be limiting oxidative metabolism; however, more evidence is needed to support this.
In the setting of knee-extensor exercise, older individuals display a slower increase in skeletal muscle vascular conductance, blood flow, and O2 delivery in the transition from rest to steady-state exercise at low- and moderate-intensity (Piil et al., 2018). Notably, the rate of increase in O2 uptake was similar in the group of young and older individuals because of higher a-vO2 difference in the older group. In the same group of older individuals, exercise training augmented the increase in vascular conductance and blood flow during the onset of moderate-intensity exercise without altering the rate O2 uptake. These initial observations suggest that skeletal muscle O2 delivery is not limiting for O2 utilisation in the initial phase of low- and moderate-intensity exercise engaging only the knee-extensors.
Skeletal muscle vascular conductance, blood flow, and O2 delivery have been reported to be lower in older individuals during steady state knee-extensor exercise, which may be a consequence of impaired endothelial function, functional sympatholysis, cGMP signalling and/or endothelin A mediated vasoconstriction (Donato et al., 2006; Mortensen et al., 2012b; Nyberg et al., 2012; Barrett-O'Keefe et al., 2015; Nyberg et al., 2015b). To what extent the attenuation in O2 delivery has consequences for the rate of O2 utilisation is currently unclear. In one group of older life-long sedentary individuals, skeletal muscle O2 delivery and O2 uptake were reported to be lower with no compensatory increase in a-vO2 difference (Mortensen et al., 2012b; Nyberg et al., 2012), thus indicating impairments in convective and potentially diffusive O2 transport. In line with this proposition, acute potentiation of cGMP signalling with phosphodiesterase 5 inhibition increases O2 delivery and utilisation during low-to moderate-intensity exercise in older but not young subjects (Nyberg et al., 2015b; Piil et al., 2018).
Exercise training leads to marked cardiovascular and skeletal muscle adaptations to structure and function, and physical inactivity also shares many similarities with aging when it comes to deterioration in these systems (Saltin et al., 1968; McGuire et al., 2001a, b; Hellsten and Nyberg, 2015). Hence, one obvious question is to what extent impairments in these systems are a consequence of aging, inactivity, or a combination of the two. One piece to this puzzle comes from the observation that training-induced potentiation of cGMP signaling leads to improvements in skeletal muscle blood flow and O2 delivery in older subjects (Piil et al., 2018). Furthermore, lifelong physical activity is associated with enhanced leg endothelial function, purinergic signalling, and ability for functional sympatholysis as well as preserved skeletal muscle oxidative metabolism during knee-extensor exercise (Mortensen et al., 2012b; Nyberg et al., 2012).
The first report of an association between aging and reduced skeletal muscle blood flow was published in 1974 and was based on observations made during cycling (Wahren et al., 1974). This association has since been confirmed and in part attributed to inefficient sympatholysis (Proctor et al., 1998; Koch et al., 2003; Poole et al., 2003). During submaximal exercise, increased O2 extraction compensates for the lower O2 delivery so that leg skeletal muscle O2 uptake is maintained for a given absolute work rate (Proctor et al., 1998; Poole et al., 2003). Importantly, there are also reports of unaltered leg blood flow, a-vO2 difference, and oxidative metabolism during similar absolute submaximal work rates (Beere et al., 1999; Proctor et al., 2003), indicating that leg hemodynamics and metabolism during cycling may not solely be reflective of age per se.
It is well established that maximal systemic O2 uptake and power output during cycling declines with advancing age. The mechanisms underlying this reduction has been suggested to be lower stroke volume, heart rate, cardiac output, systemic a-vO2 difference, leg blood flow, and leg O2 delivery and utilisation, however, there is inconsistency between studies in terms of the contribution from each specific mechanism that, at least in part, may reflect differences in gender and physical activity level (Beere et al., 1999; McGuire et al., 2001a; Poole et al., 2003; Proctor et al., 2003; Proctor et al., 2004; Carrick-Ranson et al., 2020; Pandey et al., 2020).
Findings from training studies have provided some support for a more prominent role of a peripheral limitation. In one study of older men, maximal systemic O2 uptake was reported to increase as a result of enhanced leg blood flow and O2 uptake in the absence of altered central hemodynamics, highlighting an improved distribution of cardiac output to the exercising muscles in the lower extremities (Beere et al., 1999). An increase in maximal O2 uptake in the absence of increased cardiac output has also been reported in another study where a training-induced increase in stroke volume was counterbalanced by a decline in maximal heart rate in older men (McGuire et al., 2001b). However, lifelong endurance exercise in women was found to be associated with increased maximal systemic O2 uptake that was driven by enhanced stroke volume and cardiac output as a-vO2 difference was unaltered (Carrick-Ranson et al., 2020).
Based on the findings during these various types of exercise engaging the upper and lower extremities, most evidence suggest that age-related processes lead to declines in both central and peripheral components of the O2 transport cascade although convective and potentially diffusive O2 transport in skeletal muscle appear to play more prominent roles in limiting oxidative metabolism and muscle performance (Figure 3). Future studies should aim at disentangling the effects of physical inactivity and aging to further deepen our understanding of age-related declines in cardiometabolic function. Furthermore, given the underrepresentation of female subjects in studies and the previously described differences in cardiovascular adaptations to exercise training in males and females, future studies should also aim at improving our understanding of gender differences in age- and inactivity-related declines in cardiovascular structure and function.
FIGURE 3. Cardiovascular limitations to skeletal muscle oxidative metabolism. Overview of limitations in convective (central and peripheral) and diffusive O2 transport to skeletal muscle O2 utilization in different populations. α: Intense or maximal exercise engaging a small muscle mass/submaximal exercise engaging a larger muscle mass. β: Intense/maximal exercise engaging a large muscle mass. *Diffusive O2 transport is closely linked to convective O2 transport. Hence, given the reductions in bulk blood flow, inherent heterogeneity of microvascular blood flow and imperfect matching between O2 delivery and metabolic demand at the level of the myocyte, the extent to which diffusive O2 transport is a limiting factor in aging and HF may be overestimated.
Heart failure (HF) is defined as a clinical syndrome with symptoms and/or signs caused by a structural and/or functional cardiac abnormality that is corroborated by elevated natriuretic peptide levels and/or objective evidence of pulmonary or systemic congestion (Bozkurt et al., 2021). Common for these patients is a reduction in maximal cardiac output as well as structural and functional alterations in skeletal muscle, which at the more progressed stages of the disease has severe implications for the ability to perform even simple daily activities.
Historically, patients have been characterised and segmented by their left ventricular (LV) ejection fraction (EF) so that patients with values ≤40% were classified as HF with reduced EF (HFrEF) and patients displaying LV values ≥50% as HF with preserved EF (HFpEF). Importantly, the mechanisms underlying the cardiac insult and disease progression are distinct. In HFrEF, LV (systolic) dysfunction is driven by progressive loss of cardiomyocytes due to ischemia, infection, or toxicity whereas myocardial remodelling and LV (diastolic) dysfunction in HFpEF results from a systemic proinflammatory state induced by comorbidities such as overweight/obesity, diabetes mellitus, chronic kidney disease, and chronic obstructive pulmonary disease (Paulus and Tschope, 2013; Pandey et al., 2021). In relation to these differences in pathophysiology, there is compelling evidence that skeletal muscle, in combination with cardiac dysfunction, plays a role in the impairment of O2 delivery and utilisation in HFrEF (Poole et al., 2012; Hirai et al., 2015) with the peripheral component potentially being more pronounced in HFpEF (Haykowsky et al., 2015; Sarma and Levine, 2015).
HF is largely a disease of the elderly with 50% of all HF diagnoses and 90% of all HF deaths occurring in patients over the age of 70 and HFpEF has also recently been described as a geriatric syndrome (Strait and Lakatta, 2012; Pandey et al., 2021). Although aging does not cause HF, the structural and functional changes associated with aging does lower the threshold for manifestations of the disease as many of these are integral components of HF pathology. However, to what extent HF-related impairments in O2 transport are a direct effect of age remain to be established. The following sections are meant to highlight the current understanding of mechanisms operating in HF.
In addition to the apparent central limitation in HFrEF, peripheral alterations may compromise the matching of O2 delivery and demand. These include, albeit that a great degree of heterogeneity exists in the patient population, a shift in fibre type distribution from slow-twitch oxidative to fast-twitch glycolytic fibres, fibre atrophy, reduced mitochondrial volume density, loss of capillaries supporting red blood cell flux, and impaired vascular function (Drexler et al., 1992; Poole et al., 2012; Poole et al., 2021). These structural and functional alterations can all have a substantial impact on skeletal muscle convective and diffusive O2 delivery capacity (Poole et al., 2012).
If both the capacity to increase convective and diffusive O2 transport are attenuated in response to physical activity, skeletal muscle O2 utilisation could potentially be impaired even during small muscle mass exercise. During sustained handgrip exercise, blood flow (Wiener et al., 1986; Massie et al., 1987; Arnold et al., 1990; Shoemaker et al., 1999) and O2 uptake (Arnold et al., 1990; Shoemaker et al., 1999) have been reported to be similar between HFrEF patients and controls at submaximal work rates. However, a steeper slope of the Pi/PCr-to-power output relationship, lower muscle and venous pH, and higher venous H+ were detected in patients (Wiener et al., 1986; Massie et al., 1987; Shoemaker et al., 1999), suggesting an altered skeletal muscle metabolism that is in part driven by augmented glycolytic flux. These observations also indicate that skeletal muscle hemodynamics are not altered when exercise is confined to forearm muscles but does not exclude the possibility that O2 delivery to muscles in the upper extremities may be compromised during conditions where a substantial demand for cardiac output and increase in sympathetic drive are prominent.
When knee-extensor exercise was performed with one leg, skeletal muscle blood flow and O2 uptake were found to be similar between HFrEF patients and controls at any given submaximal absolute work rate (Magnusson et al., 1997; Munch et al., 2018). When performing knee-extensor exercise with both legs, lower cardiac output and skeletal muscle perfusion were observed in patients, while control subjects maintained perfusion of the exercising muscles that was enabled via higher cardiac output. At peak intensity, blood flow and O2 uptake were similar in both legs of the controls while these variables were reduced in patients. Despite apparent impairments in O2 delivery in HFrEF, the a-vO2 difference was not different between the two groups at peak exercise with one and two legs (Magnusson et al., 1997). Similar findings were made in two separate studies in which skeletal muscle O2 delivery, a-vO2 difference, and O2 uptake were found to be comparable in HFrEF patients and controls during submaximal knee-extensor exercise performed at similar absolute work rates (Esposito et al., 2015), whereas O2 supply and utilisation, but not a-vO2 difference, were lower at peak exercise in HFrEF patients (Esposito et al., 2011). Moreover, muscle O2 diffusive capacity was lower in patients and in that same study the increase in maximal knee-extensor O2 uptake was also shown to be the result of a training-induced increase in both convective and diffusive O2 transport capacity (Esposito et al., 2011). Regarding skeletal muscle diffusive O2 capacity, it should be mentioned that this variable is portrayed as the ratio of peak skeletal muscle O2 consumption and O2 pressure gradient between microvessels and mitochondria. However, in practice it is calculated as the ratio of blood flow x a-vO2 difference and a-vO2 pressure gradient across the entire muscle/limb. Hence, reports of reduced skeletal muscle diffusive O2 capacity may to a large extent reflect impairments in convective O2 transport and blood flow distribution as these variables are severely affected in HFrEF.
Reduced blood flow during single-leg submaximal knee-extensor exercise has also been reported in HFrEF patients but is unclear how this affected oxidative metabolism as sampling of arterial and venous blood was not performed (Barrett-O'Keefe et al., 2014). Taken collectively, these findings provide evidence that limitations in both skeletal muscle convective and potentially diffusive O2 transport contribute to reduced peak O2 uptake in HFrEF patients during knee-extensor exercise, which contrasts with healthy individuals where the site of limitation resides at the level of the mitochondria.
Central hemodynamics, leg blood flow, and metabolic responses to upright cycle exercise have also been assessed in HFrEF patients. At similar absolute work rates, cardiac output, leg blood flow, and leg vascular conductance were found to be lower at all submaximal and maximal intensities in HFrEF patients compared to controls (Sullivan et al., 1989). This impairment in skeletal muscle perfusion was associated with an increased a-vO2 difference, however, O2 uptake was only preserved at the lowest intensity with O2 utilisation falling when the work rate was increased. The higher vascular resistance in these patients was an important contributor to systemic vascular resistance that allowed preservation of mean arterial pressure and the maintenance of sufficient perfusion pressure across vital organs at all work rates. In line with this observation, peak systemic and leg O2 delivery, a-vO2 difference, muscle O2 diffusional conductance, and O2 uptake was reported to be lower in HFrEF patients (Esposito et al., 2011; Dhakal et al., 2015).
To disclose potential peripheral limitations to O2 uptake in HFrEF, insights from studies using training with a small muscle group can be informative as such protocols limits cardiac adaptations. By using this approach, increases in capillary-to-fibre ratio, mitochondrial volume density, oxidative enzyme activity, type 1 fibre fraction, and functional sympatholysis have been reported alongside higher levels of peak O2 delivery, a-vO2 difference, muscle O2 diffusional conductance, and O2 uptake during knee-extensor exercise (Magnusson et al., 1996; Tyni-Lenne et al., 1999; Esposito et al., 2011; Munch et al., 2018). Importantly, these peripheral adaptations were also associated with increases in peak systemic O2 uptake during cycling exercise (Tyni-Lenne et al., 2001; Esposito et al., 2011). Furthermore, cycle exercise training increased peak leg blood flow, O2 delivery, a-vO2 difference, and O2 uptake whereas the increase in cardiac output did not reach statistical significance (Sullivan et al., 1988). Hence, reductions in both cardiac output and skeletal muscle convective and diffusive O2 transport capacity in skeletal muscle appear to contribute to impaired peripheral oxidative capacity and exercise intolerance in HFrEF patients. This peripheral limitation during exercise engaging both legs is in line with that observed during knee-extensor exercise and should be viewed in the context of augmented peripheral vascular resistance and insufficient muscle perfusion needed to uphold mean arterial pressure.
HFpEF, which is characterized by abnormal relaxation of the LV and decreased LV compliance, is a multiorgan geriatric syndrome driven by mechanisms related to multimorbidity, systemic inflammation, obesity, aging, and physical inactivity (Paulus and Tschope, 2013; Pandey et al., 2021). Hence, HFpEF is distinct from HFrEF in many regards as also evidenced by findings from clinical trials in which neurohumoral blockade improves mortality and HF hospitalisations in HFrEF but not HFpEF. Recently, the angiotensin receptor-neprilysin inhibitor sacubitril-valsartan and the sodium–glucose cotransporter 2 inhibitor empagliflozin were shown to also have clinical benefits in HFpEF; however, an attenuation of benefits in patients with higher EF was evident in both trials (Solomon et al., 2019; Anker et al., 2021).
Following the onset of exercise, HFpEF patients display greater increases in pulmonary arterial pressure, pulmonary capillary wedge pressure, LV end diastolic pressure, and late systolic load that in conjunction with a lower increase in heart rate results in reduced cardiac output (Borlaug et al., 2010; Pandey et al., 2021). Although exercise-induced pulmonary oedema is a primary cause of exertional intolerance in HFpEF, abnormalities in skeletal muscle O2 uptake are apparent even during submaximal exercise (Sarma and Levine, 2015). Contributors to this peripheral maladaptation to acute exercise in HFpEF include reduced vascular function, capillary-to-fibre ratio, mitochondrial content and oxidative capacity, loss of type 1 fibres, and muscle fat infiltration that all may affect skeletal muscle convective and diffusive O2 transport and utilisation (Kitzman et al., 2014; Sarma and Levine, 2015; Marechaux et al., 2016; Molina et al., 2016; Pandey et al., 2021).
Only very few studies have investigated hemodynamic and metabolic variables in HFpEF patients during isolated forearm and knee-extensor exercise. In one study, skeletal muscle vascular conductance and blood flow were found to be reduced at higher submaximal intensities in patients in the absence of macrovascular dysfunction and dysregulated central hemodynamics (Ratchford et al., 2020). In another study, a-vO2 difference was reduced across all brachial blood flows in patients, however, peak O2 uptake was preserved in HFpEF through a statistically non-significant enhancement (∼14%) of blood flow (Zamani et al., 2020). Notably, forearm diffusional O2 conductance was not different among HFpEF patients and healthy control subjects. During knee-extensor exercise, HFpEF patients have been shown to exhibit marked exercise intolerance compared to controls, with almost 25% of patients unable to continue beyond unloaded ergometer exercise (Lee et al., 2016). These patients also displayed lower skeletal muscle vascular conductance and blood flow during exercise that was not related to dysregulated central hemodynamics. These initial observations during small muscle mass exercise are indicative of abnormalities in skeletal muscle convective O2 transport but additional studies are warranted to confirm this.
By using a modified Astrand–Saltin incremental treadmill protocol, it was demonstrated that HFpEF patients were able to increase cardiac output and other indices of cardiac reserve to a similar extent as that of controls during submaximal and maximal running (Bhella et al., 2011). This preservation of central hemodynamics was, however, associated with lower systemic a-vO2 difference and O2 uptake, which was likely to be caused by a lower capacity for skeletal muscle oxidative metabolism as measured by magnetic resonance spectroscopy. Interestingly, a similar hyperdynamic response (∆cardiac output/∆O2 uptake slope) is observed in patients with mitochondrial myopathies (Taivassalo et al., 2003). In line with this peripheral limitation, impaired skeletal muscle O2 extraction attributable to impaired diffusive O2 transport and utilization has also been shown in HFpEF patients during upright maximal intensity cycling (Dhakal et al., 2015; Houstis et al., 2018). These studies also demonstrated lower cardiac output and systemic O2 uptake and it is important to note that the vast majority (97%) of patients with HFpEF harboured defects at multiple steps of the O2 pathway with a high degree of heterogeneity in terms of identity and magnitude (Houstis et al., 2018). This heterogeneity resonates well with a multiorgan syndrome driven by a broad range of pathophysiological mechanisms and underscores that the extent to which a disease-induced alteration in convective and diffusive O2 transport and/or utilization may contribute to impaired skeletal muscle metabolic function will be patient and context-dependent.
Several clinical trials have evaluated the efficacy of exercise training in improving systemic O2 uptake and cardiac function and found that peak O2 uptake increases without significant changes in LV systolic or diastolic function measured at rest (Pandey et al., 2015). Evidently, more training studies are needed to assess whether central hemodynamics are altered during exercise and to what extent skeletal muscle convective and diffusive O2 transport and utilization are altered with training to shed more light on central vs. peripheral limitations in HFpEF.
AJ and MN drafted and approved the final version of the manuscript.
Author MN was employed by the company Novo Nordisk.
The remaining author declares that the research was conducted in the absence of any commercial or financial relationships that could be construed as a potential conflict of interest.
All claims expressed in this article are solely those of the authors and do not necessarily represent those of their affiliated organizations, or those of the publisher, the editors and the reviewers. Any product that may be evaluated in this article, or claim that may be made by its manufacturer, is not guaranteed or endorsed by the publisher.
Andersen P., Saltin B. (1985). Maximal Perfusion of Skeletal Muscle in Man. J. Physiol. 366, 233–249. doi:10.1113/jphysiol.1985.sp015794
Anker S. D., Butler J., Filippatos G., Ferreira J. P., Bocchi E., Böhm M., et al. (2021). Empagliflozin in Heart Failure with a Preserved Ejection Fraction. N. Engl. J. Med. 385, 1451–1461. doi:10.1056/nejmoa2107038
Armstrong R. B., Laughlin M. H. (1983). Blood Flows within and Among Rat Muscles as a Function of Time during High Speed Treadmill Exercise. J. Physiol. 344, 189–208. doi:10.1113/jphysiol.1983.sp014933
Arnold J. M., Ribeiro J. P., Colucci W. S. (1990). Muscle Blood Flow during Forearm Exercise in Patients with Severe Heart Failure. Circulation 82, 465–472. doi:10.1161/01.cir.82.2.465
Åstrand H., Sandgren T., Ahlgren Å. R., Länne T. (2003). Noninvasive Ultrasound Measurements of Aortic Intima-Media Thickness: Implications for In Vivo Study of Aortic Wall Stress1 1Competition of Interest: None. J. Vasc. Surg. 37, 1270–1276. doi:10.1016/s0741-5214(02)75344-5
Barrett-O'Keefe Z., Ives S. J., Trinity J. D., Morgan G., Rossman M. J., Donato A. J., et al. (2015). Endothelin-A-mediated Vasoconstriction during Exercise with Advancing Age. J. Gerontol. A Biol. Sci. Med. Sci. 70, 554–565. doi:10.1093/gerona/glu065
Barrett-O'Keefe Z., Lee J. F., Berbert A., Witman M. A. H., Nativi-Nicolau J., Stehlik J., et al. (2014). Hemodynamic Responses to Small Muscle Mass Exercise in Heart Failure Patients with Reduced Ejection Fraction. Am. J. Physiology-Heart Circulatory Physiology 307, H1512–H1520. doi:10.1152/ajpheart.00527.2014
Beere P. A., Russell S. D., Morey M. C., Kitzman D. W., Higginbotham M. B. (1999). Aerobic Exercise Training Can Reverse Age-Related Peripheral Circulatory Changes in Healthy Older Men. Circulation 100, 1085–1094. doi:10.1161/01.cir.100.10.1085
Bhella P. S., Prasad A., Heinicke K., Hastings J. L., Arbab-Zadeh A., Adams-Huet B., et al. (2011). Abnormal Haemodynamic Response to Exercise in Heart Failure with Preserved Ejection Fraction. Eur. J. Heart Fail. 13, 1296–1304. doi:10.1093/eurjhf/hfr133
Borlaug B. A., Nishimura R. A., Sorajja P., Lam C. S. P., Redfield M. M. (2010). Exercise Hemodynamics Enhance Diagnosis of Early Heart Failure with Preserved Ejection Fraction. Circ. Heart Fail. 3, 588–595. doi:10.1161/circheartfailure.109.930701
Boushel R., Ara I., Gnaiger E., Helge J. W., González-Alonso J., Munck-Andersen T., et al. (2014). Low-intensity Training Increases Peak Arm VO2by Enhancing Both Convective and Diffusive O2delivery. Acta Physiol. 211, 122–134. doi:10.1111/apha.12258
Boushel R., Saltin B. (2013). Ex Vivo measures of Muscle Mitochondrial Capacity Reveal Quantitative Limits of Oxygen Delivery by the Circulation during Exercise. Int. J. Biochem. Cell Biol. 45, 68–75. doi:10.1016/j.biocel.2012.09.024
Bozkurt B., Coats A. J. S., Tsutsui H., Abdelhamid C. M., Adamopoulos S., Albert N., et al. (2021). Universal Definition and Classification of Heart Failure: a Report of the Heart Failure Society of America, Heart Failure Association of the European Society of Cardiology, Japanese Heart Failure Society and Writing Committee of the Universal Definition of Heart Failure. Eur. J. Heart Fail 23, 352–380. doi:10.1002/ejhf.2115
Buckwalter J. B., Clifford P. S. (2001). The Paradox of Sympathetic Vasoconstriction in Exercising Skeletal Muscle. Exerc. Sport Sci. Rev. 29, 159–163. doi:10.1097/00003677-200110000-00005
Buckwalter J. B., Naik J. S., Valic Z., Clifford P. S. (2001). Exercise Attenuates α-adrenergic-receptor Responsiveness in Skeletal Muscle Vasculature. J. Appl. Physiology 90, 172–178. doi:10.1152/jappl.2001.90.1.172
Calbet J. A. L., Holmberg H.-C., Rosdahl H., van Hall G., Jensen-Urstad M., Saltin B. (2005). Why Do Arms Extract Less Oxygen Than Legs during Exercise? Am. J. Physiology-Regulatory, Integr. Comp. Physiology 289, R1448–R1458. doi:10.1152/ajpregu.00824.2004
Calbet J. A. L., Jensen-Urstad M., van Hall G., Holmberg H.-C., Rosdahl H., Saltin B. (2004). Maximal Muscular Vascular Conductances during Whole Body Upright Exercise in Humans. J. Physiol. 558, 319–331. doi:10.1113/jphysiol.2003.059287
Carrick-Ranson G., Sloane N. M., Howden E. J., Bhella P. S., Sarma S., Shibata S., et al. (2020). The Effect of Lifelong Endurance Exercise on Cardiovascular Structure and Exercise Function in Women. J. Physiol. 598, 2589–2605. doi:10.1113/JP278503
Casey D. P., Joyner M. J. (2012). Influence of α-adrenergic Vasoconstriction on the Blunted Skeletal Muscle Contraction-Induced Rapid Vasodilation with Aging. J. Appl. Physiology 113, 1201–1212. doi:10.1152/japplphysiol.00734.2012
Casey D. P., Ranadive S. M., Joyner M. J. (2015). Aging Is Associated with Altered Vasodilator Kinetics in Dynamically Contracting Muscle: Role of Nitric Oxide. J. Appl. Physiology 119, 232–241. doi:10.1152/japplphysiol.00787.2014
Christensen P. M., Nyberg M., Mortensen S. P., Nielsen J. J., Secher N. H., Damsgaard R., et al. (2013). Leg Oxygen Uptake in the Initial Phase of Intense Exercise Is Slowed by a Marked Reduction in Oxygen Delivery. Am. J. Physiology-Regulatory, Integr. Comp. Physiology 305, R313–R321. doi:10.1152/ajpregu.00048.2013
Crecelius A. R., Kirby B. S., Voyles W. F., Dinenno F. A. (2011). Augmented Skeletal Muscle Hyperaemia during Hypoxic Exercise in Humans Is Blunted by Combined Inhibition of Nitric Oxide and Vasodilating Prostaglandins. J. Physiol. 589, 3671–3683. doi:10.1113/jphysiol.2011.209486
Dhakal B. P., Malhotra R., Murphy R. M., Pappagianopoulos P. P., Baggish A. L., Weiner R. B., et al. (2015). Mechanisms of Exercise Intolerance in Heart Failure with Preserved Ejection Fraction. Circ. Heart Fail. 8, 286–294. doi:10.1161/circheartfailure.114.001825
Dinenno F. A., Masuki S., Joyner M. J. (2005). Impaired Modulation of Sympathetic α-adrenergic Vasoconstriction in Contracting Forearm Muscle of Ageing Men. J. Physiol. 567, 311–321. doi:10.1113/jphysiol.2005.087668
Donato A. J., Uberoi A., Wray D. W., Nishiyama S., Lawrenson L., Richardson R. S. (2006). Differential Effects of Aging on Limb Blood Flow in Humans. Am. J. Physiology-Heart Circulatory Physiology 290, H272–H278. doi:10.1152/ajpheart.00405.2005
Drexler H., Riede U., Münzel T., König H., Funke E., Just H. (1992). Alterations of Skeletal Muscle in Chronic Heart Failure. Circulation 85, 1751–1759. doi:10.1161/01.cir.85.5.1751
Egginton S., Gaffney E. (2010). Experimental Physiology -Review Article: Tissue Capillary Supply - It's Quality Not Quantity that Counts!. Exp. Physiol. 95, 971–979. doi:10.1113/expphysiol.2010.053421
Ellsworth M. L., Ellis C. G., Sprague R. S. (2016). Role of Erythrocyte-Released ATP in the Regulation of Microvascular Oxygen Supply in Skeletal Muscle. Acta Physiol. 216, 265–276. doi:10.1111/apha.12596
Esposito F., Reese V., Shabetai R., Wagner P. D., Richardson R. S. (2011). Isolated Quadriceps Training Increases Maximal Exercise Capacity in Chronic Heart Failure. J. Am. Coll. Cardiol. 58, 1353–1362. doi:10.1016/j.jacc.2011.06.025
Esposito F., Wagner P. D., Richardson R. S. (2015). Incremental Large and Small Muscle Mass Exercise in Patients with Heart Failure: Evidence of Preserved Peripheral Haemodynamics and Metabolism. Acta Physiol. 213, 688–699. doi:10.1111/apha.12423
Essén-gustavsson B., Henriksson J. (1984). Enzyme Levels in Pools of Microdissected Human Muscle Fibres of Identified Type: Adaptive Response to Exercise. Acta Physiol. Scand. 120, 505–515. doi:10.1111/j.1748-1716.1984.tb07414.x
Faisal A., Dyson K. S., Hughson R. L. (2010). Prolonged Ischaemia Impairs Muscle Blood Flow and Oxygen Uptake Dynamics during Subsequent Heavy Exercise. J. Physiol. 588, 3785–3797. doi:10.1113/jphysiol.2010.188698
Fleg J. L., Strait J. (2012). Age-associated Changes in Cardiovascular Structure and Function: a Fertile Milieu for Future Disease. Heart Fail Rev. 17, 545–554. doi:10.1007/s10741-011-9270-2
González-Alonso J., Calbet J. A. (2003). Reductions in Systemic and Skeletal Muscle Blood Flow and Oxygen Delivery Limit Maximal Aerobic Capacity in Humans. Circulation 107, 824–830. doi:10.1161/01.cir.0000049746.29175.3f
González-Alonso J., Olsen D. B., Saltin B. (2002). Erythrocyte and the Regulation of Human Skeletal Muscle Blood Flow and Oxygen Delivery: Role of Circulating ATP. Circ. Res. 91, 1046–1055. doi:10.1161/01.res.0000044939.73286.e2
Hansen A. B., Moralez G., Romero S. A., Gasho C., Tymko M. M., Ainslie P. N., et al. (2020). Mechanisms of Sympathetic Restraint in Human Skeletal Muscle during Exercise: Role of α-adrenergic and Nonadrenergic Mechanisms. Am. J. Physiology-Heart Circulatory Physiology 319, H192–H202. doi:10.1152/ajpheart.00208.2020
Hargreaves M., Spriet L. L. (2020). Skeletal Muscle Energy Metabolism during Exercise. Nat. Metab. 2, 817–828. doi:10.1038/s42255-020-0251-4
Haykowsky M. J., Tomczak C. R., Scott J. M., Paterson D. I., Kitzman D. W. (2015). Determinants of Exercise Intolerance in Patients with Heart Failure and Reduced or Preserved Ejection Fraction. J. Appl. Physiology 119, 739–744. doi:10.1152/japplphysiol.00049.2015
Hellsten Y., Nyberg M. (2015). Cardiovascular Adaptations to Exercise Training. Compr. Physiol. 6, 1–32. doi:10.1002/cphy.c140080
Hirai D. M., Musch T. I., Poole D. C. (2015). Exercise Training in Chronic Heart Failure: Improving Skeletal Muscle O2transport and Utilization. Am. J. Physiology-Heart Circulatory Physiology 309, H1419–H1439. doi:10.1152/ajpheart.00469.2015
Houstis N. E., Eisman A. S., Pappagianopoulos P. P., Wooster L., Bailey C. S., Wagner P. D., et al. (2018). Exercise Intolerance in Heart Failure with Preserved Ejection Fraction. Circulation 137, 148–161. doi:10.1161/circulationaha.117.029058
Hughes W. E., Kruse N. T., Casey D. P. (2018). Age-associated Impairments in Contraction-Induced Rapid-Onset Vasodilatation within the Forearm Are Independent of Mechanical Factors. Exp. Physiol. 103, 728–737. doi:10.1113/ep086908
Hughson R. L., Shoemaker J. K., Tschakovsky M. E., Kowalchuk J. M. (1996). Dependence of muscleV˙o 2on Blood Flow Dynamics at Onset of Forearm Exercise. J. Appl. Physiology 81, 1619–1626. doi:10.1152/jappl.1996.81.4.1619
Jasperse J. L., Seals D. R., Callister R. (1994). Active Forearm Blood Flow Adjustments to Handgrip Exercise in Young and Older Healthy Men. J. Physiol. 474, 353–360. doi:10.1113/jphysiol.1994.sp020027
Joyner M. J., Casey D. P. (2015). Regulation of Increased Blood Flow (Hyperemia) to Muscles during Exercise: a Hierarchy of Competing Physiological Needs. Physiol. Rev. 95, 549–601. doi:10.1152/physrev.00035.2013
Joyner M. J., Nauss L. A., Warner M. A., Warner D. O. (1992). Sympathetic Modulation of Blood Flow and O2 Uptake in Rhythmically Contracting Human Forearm Muscles. Am. J. Physiology-Heart Circulatory Physiology 263, H1078–H1083. doi:10.1152/ajpheart.1992.263.4.h1078
Kirby B. S., Carlson R. E., Markwald R. R., Voyles W. F., Dinenno F. A. (2007). Mechanical Influences on Skeletal Muscle Vascular Tone in Humans: Insight into Contraction-Induced Rapid Vasodilatation. J. Physiol. 583, 861–874. doi:10.1113/jphysiol.2007.131250
Kirby B. S., Crecelius A. R., Voyles W. F., Dinenno F. A. (2012). Impaired Skeletal Muscle Blood Flow Control with Advancing Age in Humans. Circ. Res. 111, 220–230. doi:10.1161/circresaha.112.269571
Kirby B. S., Voyles W. F., Simpson C. B., Carlson R. E., Schrage W. G., Dinenno F. A. (2009). Endothelium-dependent Vasodilatation and Exercise Hyperaemia in Ageing Humans: Impact of Acute Ascorbic Acid Administration. J. Physiol. 587, 1989–2003. doi:10.1113/jphysiol.2008.167320
Kitzman D. W., Nicklas B., Kraus W. E., Lyles M. F., Eggebeen J., Morgan T. M., et al. (2014). Skeletal Muscle Abnormalities and Exercise Intolerance in Older Patients with Heart Failure and Preserved Ejection Fraction. Am. J. Physiology-Heart Circulatory Physiology 306, H1364–H1370. doi:10.1152/ajpheart.00004.2014
Koch D. W., Leuenberger U. A., Proctor D. N. (2003). Augmented Leg Vasoconstriction in Dynamically Exercising Older Men during Acute Sympathetic Stimulation. J. Physiology 551, 337–344. doi:10.1113/jphysiol.2003.042747
Koskolou M. D., Calbet J. A., Radegran G., Roach R. C. (1997). Hypoxia and the Cardiovascular Response to Dynamic Knee-Extensor Exercise. Am. J. Physiology-Heart Circulatory Physiology 272, H2655–H2663. doi:10.1152/ajpheart.1997.272.6.h2655
Krustrup P., Soderlund K., Mohr M., Gonzalez-Alonso J., Bangsbo J. (2004). Recruitment of Fibre Types and Quadriceps Muscle Portions during Repeated, Intense Knee-Extensor Exercise in Humans. Pflugers Arch. - Eur. J. Physiol. 449, 56–65. doi:10.1007/s00424-004-1304-3
Lakatta E. G., Levy D. (2003a). Arterial and Cardiac Aging: Major Shareholders in Cardiovascular Disease Enterprises. Circulation 107, 139–146. doi:10.1161/01.cir.0000048892.83521.58
Lakatta E. G., Levy D. (2003b). Arterial and Cardiac Aging: Major Shareholders in Cardiovascular Disease Enterprises. Circulation 107, 346–354. doi:10.1161/01.cir.0000048893.62841.f7
Lee J. F., Barrett-O'Keefe Z., Nelson A. D., Garten R. S., Ryan J. J., Nativi-Nicolau J. N., et al. (2016). Impaired Skeletal Muscle Vasodilation during Exercise in Heart Failure with Preserved Ejection Fraction. Int. J. Cardiol. 211, 14–21. doi:10.1016/j.ijcard.2016.02.139
Levine B. D. (2008). : what Do We Know, and what Do We Still Need to Know? J. Physiol. 586, 25–34. doi:10.1113/jphysiol.2007.147629
Magnusson G., Gordon A., Kaijser L., Sylven C., Isberg B., Karpakka J., et al. (1996). High Intensity Knee Extensor Training, in Patients with Chronic Heart Failure: Major Skeletal Muscle Improvement. Eur. Heart J. 17, 1048–1055. doi:10.1093/oxfordjournals.eurheartj.a015001
Magnusson G., Kaijser L., Sylvén C., Karlberg K.-E., Isberg B., Saltin B. (1997). Peak Skeletal Muscle Perfusion Is Maintained in Patients with Chronic Heart Failure when Only a Small Muscle Mass Is Exercised. Cardiovasc Res. 33, 297–306. doi:10.1016/s0008-6363(96)00249-0
Maréchaux S., Samson R., van Belle E., Breyne J., de Monte J., Dédrie C., et al. (2016). Vascular and Microvascular Endothelial Function in Heart Failure with Preserved Ejection Fraction. J. Cardiac Fail. 22, 3–11. doi:10.1016/j.cardfail.2015.09.003
Massie B., Conway M., Yonge R., Frostick S., Ledingham J., Sleight P., et al. (1987). Skeletal Muscle Metabolism in Patients with Congestive Heart Failure: Relation to Clinical Severity and Blood Flow. Circulation 76, 1009–1019. doi:10.1161/01.cir.76.5.1009
McGuire D. K., Levine B. D., Williamson J. W., Snell P. G., Blomqvist C. G., Saltin B., et al. (2001a). A 30-Year Follow-Up of the Dallas Bed Rest and Training Study. Circulation 104, 1350–1357. doi:10.1161/circ.104.12.1350
McGuire D. K., Levine B. D., Williamson J. W., Snell P. G., Blomqvist C. G., Saltin B., et al. (2001b). A 30-Year Follow-Up of the Dallas Bed Rest and Training Study. Circulation 104, 1358–1366. doi:10.1161/hc3701.096099
Mendelson A. A., Milkovich S., Hunter T., Vijay R., Choi Y. H., Milkovich S., et al. (2021). The Capillary Fascicle in Skeletal Muscle: Structural and Functional Physiology of RBC Distribution in Capillary Networks. J. Physiol. 599, 2149–2168. doi:10.1113/jp281172
Molina A. J. A., Bharadwaj M. S., Van Horn C., Nicklas B. J., Lyles M. F., Eggebeen J., et al. (2016). Skeletal Muscle Mitochondrial Content, Oxidative Capacity, and Mfn2 Expression Are Reduced in Older Patients with Heart Failure and Preserved Ejection Fraction and Are Related to Exercise Intolerance. JACC Heart Fail. 4, 636–645. doi:10.1016/j.jchf.2016.03.011
Montero D., Diaz-cañestro C., Lundby C. (2015). Endurance Training and V˙O2max. Med. Sci. Sports Exerc 47, 2024–2033. doi:10.1249/mss.0000000000000640
Mortensen S. P., Askew C. D., Walker M., Nyberg M., Hellsten Y. (2012a). The Hyperaemic Response to Passive Leg Movement Is Dependent on Nitric Oxide: a New Tool to Evaluate Endothelial Nitric Oxide Function. J. Physiol. 590, 4391–4400. doi:10.1113/jphysiol.2012.235952
Mortensen S. P., Damsgaard R., Dawson E. A., Secher N. H., González-Alonso J. (2008). Restrictions in Systemic and Locomotor Skeletal Muscle Perfusion, Oxygen Supply andVO2during High-Intensity Whole-Body Exercise in Humans. J. Physiol. 586, 2621–2635. doi:10.1113/jphysiol.2007.149401
Mortensen S. P., Dawson E. A., Yoshiga C. C., Dalsgaard M. K., Damsgaard R., Secher N. H., et al. (2005). Limitations to Systemic and Locomotor Limb Muscle Oxygen Delivery and Uptake during Maximal Exercise in Humans. J. Physiol. 566, 273–285. doi:10.1113/jphysiol.2005.086025
Mortensen S. P., Nyberg M., Winding K., Saltin B. (2012b). Lifelong Physical Activity Preserves Functional Sympatholysis and Purinergic Signalling in the Ageing Human Leg. J. Physiol. 590, 6227–6236. doi:10.1113/jphysiol.2012.240093
Mortensen S. P., Thaning P., Nyberg M., Saltin B., Hellsten Y. (2011). Local Release of ATP into the Arterial Inflow and Venous Drainage of Human Skeletal Muscle: Insight from ATP Determination with the Intravascular Microdialysis Technique. J. Physiol. 589, 1847–1857. doi:10.1113/jphysiol.2010.203034
Munch G. D. W., Svendsen J. H., Damsgaard R., Secher N. H., González-Alonso J., Mortensen S. P. (2014). Maximal Heart Rate Does Not Limit Cardiovascular Capacity in Healthy Humans: Insight from Right Atrial Pacing during Maximal Exercise. J. Physiology 592, 377–390. doi:10.1113/jphysiol.2013.262246
Munch G. W., Iepsen U. W., Ryrsø C. K., Rosenmeier J. B., Pedersen B. K., Mortensen S. P. (2018). Effect of 6 Wk of High-Intensity One-Legged Cycling on Functional Sympatholysis and ATP Signaling in Patients with Heart Failure. Am. J. Physiol. Heart Circ. Physiol. 314, H616–H626. doi:10.1152/ajpheart.00379.2017
North B. J., Sinclair D. A. (2012). The Intersection between Aging and Cardiovascular Disease. Circ. Res. 110, 1097–1108. doi:10.1161/circresaha.111.246876
Nyberg M., Piil P., Egelund J., Sprague R. S., Mortensen S. P., Hellsten Y. (2015b). Potentiation of cGMP Signaling Increases Oxygen Delivery and Oxidative Metabolism in Contracting Skeletal Muscle of Older but Not Young Humans. Physiol. Rep. 3, 1. doi:10.14814/phy2.12508
Nyberg M., Blackwell J. R., Damsgaard R., Jones A. M., Hellsten Y., Mortensen S. P. (2012). Lifelong Physical Activity Prevents an Age-Related Reduction in Arterial and Skeletal Muscle Nitric Oxide Bioavailability in Humans. J. Physiol. 590, 5361–5370. doi:10.1113/jphysiol.2012.239053
Nyberg M., Christensen P. M., Blackwell J. R., Hostrup M., Jones A. M., Bangsbo J. (2021). Nitrate‐rich Beetroot Juice Ingestion Reduces Skeletal Muscle O 2 Uptake and Blood Flow during Exercise in Sedentary Men. J. Physiol. 599, 5203–5214. doi:10.1113/jp281995
Nyberg M., Christensen P. M., Mortensen S. P., Hellsten Y., Bangsbo J. (2014). Infusion of ATP Increases Leg Oxygen Delivery but Not Oxygen Uptake in the Initial Phase of Intense Knee-Extensor Exercise in Humans. Exp. Physiol. 99, 1399–1408. doi:10.1113/expphysiol.2014.081141
Nyberg M., Hellsten Y. (2016). Reduced Blood Flow to Contracting Skeletal Muscle in Ageing Humans: Is it All an Effect of Sand through the Hourglass? J. Physiol. 594, 2297–2305. doi:10.1113/jp270594
Nyberg M., Mortensen S. P., Saltin B., Hellsten Y., Bangsbo J. (2010). Low Blood Flow at Onset of Moderate-Intensity Exercise Does Not Limit Muscle Oxygen Uptake. Am. J. Physiology-Regulatory, Integr. Comp. Physiology 298, R843–R848. doi:10.1152/ajpregu.00730.2009
Nyberg M., Piil P., Egelund J., Sprague R. S., Mortensen S. P., Hellsten Y. (2015a). Effect of PDE5 Inhibition on the Modulation of Sympathetic α-adrenergic Vasoconstriction in Contracting Skeletal Muscle of Young and Older Recreationally Active Humans. Am. J. Physiology-Heart Circulatory Physiology 309, H1867–H1875. doi:10.1152/ajpheart.00653.2015
Nyberg M., Piil P., Kiehn O. T., Maagaard C., Jørgensen T. S., Egelund J., et al. (2018). Probenecid Inhibits α-Adrenergic Receptor-Mediated Vasoconstriction in the Human Leg Vasculature. Hypertension 71, 151–159. doi:10.1161/hypertensionaha.117.10251
Nyberg S. K., Berg O. K., Helgerud J., Wang E. (2017). Blood Flow Regulation and Oxygen Uptake during High-Intensity Forearm Exercise. J. Appl. Physiology 122, 907–917. doi:10.1152/japplphysiol.00983.2016
Pandey A., Kraus W. E., Brubaker P. H., Kitzman D. W. (2020). Healthy Aging and Cardiovascular Function. JACC Heart Fail. 8, 111–121. doi:10.1016/j.jchf.2019.08.020
Pandey A., Parashar A., Kumbhani D. J., Agarwal S., Garg J., Kitzman D., et al. (2015). Exercise Training in Patients with Heart Failure and Preserved Ejection Fraction. Circ. Heart Fail. 8, 33–40. doi:10.1161/circheartfailure.114.001615
Pandey A., Shah S. J., Butler J., Kellogg D. L., Lewis G. D., Forman D. E., et al. (2021). Exercise Intolerance in Older Adults with Heart Failure with Preserved Ejection Fraction. J. Am. Coll. Cardiol. 78, 1166–1187. doi:10.1016/j.jacc.2021.07.014
Patterson G. C., Shepherd J. T. (1954). The Effects of Continuous Infusions into the Brachial Artery of Adenosine Triphosphate, Histamine and Acetylcholine on the Amount and Rate of Blood Debt Repayment Following Rhythmic Exercise of the Forearm Muscles. Clin. Sci. 13, 85–91.
Paulus W. J., Tschöpe C. (2013). A Novel Paradigm for Heart Failure with Preserved Ejection Fraction. J. Am. Coll. Cardiol. 62, 263–271. doi:10.1016/j.jacc.2013.02.092
Pawelczyk J. A., Hanel B., Pawelczyk R. A., Warberg J., Secher N. H. (1992). Leg Vasoconstriction during Dynamic Exercise with Reduced Cardiac Output. J. Appl. Physiology 73, 1838–1846. doi:10.1152/jappl.1992.73.5.1838
Pawelczyk J. A., Levine B. D. (2002). Heterogeneous Responses of Human Limbs to Infused Adrenergic Agonists: a Gravitational Effect? J. Appl. Physiology 92, 2105–2113. doi:10.1152/japplphysiol.00979.2001
Perko M. J., Nielsen H. B., Skak C., Clemmesen J. O., Schroeder T. V., Secher N. H. (1998). Mesenteric, Coeliac and Splanchnic Blood Flow in Humans during Exercise. J. Physiol. 513 (Pt 3), 907–913. doi:10.1111/j.1469-7793.1998.907ba.x
Perrey S., Tschakovsky M. E., Hughson R. L. (2001). Muscle Chemoreflex Elevates Muscle Blood Flow and O2 Uptake at Exercise Onset in Nonischemic Human Forearm. J. Appl. Physiology 91, 2010–2016. doi:10.1152/jappl.2001.91.5.2010
Piil P., Jørgensen T. S., Egelund J., Rytter N., Gliemann L., Bangsbo J., et al. (2018). Effects of Aging and Exercise Training on Leg Hemodynamics and Oxidative Metabolism in the Transition from Rest to Steady-State Exercise: Role of cGMP Signaling. Am. J. Physiology-Regulatory, Integr. Comp. Physiology 315, R274–R283. doi:10.1152/ajpregu.00446.2017
Poole D. C., Barstow T. J., McDonough P., Jones A. M. (2008). Control of Oxygen Uptake during Exercise. Med. Sci. Sports Exerc 40, 462–474. doi:10.1249/mss.0b013e31815ef29b
Poole D. C., Behnke B. J., Musch T. I. (2021). The Role of Vascular Function on Exercise Capacity in Health and Disease. J. Physiol. 599, 889–910. doi:10.1113/jp278931
Poole D. C., Hirai D. M., Copp S. W., Musch T. I. (2012). Muscle Oxygen Transport and Utilization in Heart Failure: Implications for Exercise (In)tolerance. Am. J. Physiology-Heart Circulatory Physiology 302, H1050–H1063. doi:10.1152/ajpheart.00943.2011
Poole J. G., Lawrenson L., Kim J., Brown C., Richardson R. S. (2003). Vascular and Metabolic Response to Cycle Exercise in Sedentary Humans: Effect of Age. Am. J. Physiology-Heart Circulatory Physiology 284, H1251–H1259. doi:10.1152/ajpheart.00790.2002
Proctor D. N., Koch D. W., Newcomer S. C., Le K. U., Smithmyer S. L., Leuenberger U. A. (2004). Leg Blood Flow and &OV0312;O2 during Peak Cycle Exercise in Younger and Older Women. Med. Sci. Sports Exerc. 36, 623–631. doi:10.1249/01.mss.0000121951.10417.b5
Proctor D. N., Newcomer S. C. (2006). Is There a Difference in Vascular Reactivity of the Arms and Legs? Med. Sci. Sports Exerc 38, 1819–1828. doi:10.1249/01.mss.0000230340.79247.52
Proctor D. N., Newcomer S. C., Koch D. W., Le K. U., MacLean D. A., Leuenberger U. A. (2003). Leg Blood Flow during Submaximal Cycle Ergometry Is Not Reduced in Healthy Older Normally Active Men. J. Appl. Physiology 94, 1859–1869. doi:10.1152/japplphysiol.00898.2002
Proctor D. N., Shen P. H., Dietz N. M., Eickhoff T. J., Lawler L. A., Ebersold E. J., et al. (1998). Reduced Leg Blood Flow during Dynamic Exercise in Older Endurance-Trained Men. J. Appl. Physiology 85, 68–75. doi:10.1152/jappl.1998.85.1.68
Ratchford S. M., Clifton H. L., La Salle D. T., Broxterman R. M., Lee J. F., Ryan J. J., et al. (2020). Cardiovascular Responses to Rhythmic Handgrip Exercise in Heart Failure with Preserved Ejection Fraction. J. Appl. Physiology 129, 1267–1276. doi:10.1152/japplphysiol.00468.2020
Remensnyder J. P., Mitchell J. H., Sarnoff S. J. (1962). Functional Sympatholysis during Muscular Activity. Circulation Res. 11, 370–380. doi:10.1161/01.res.11.3.370
Richards J. C., Crecelius A. R., Larson D. G., Dinenno F. A. (2015). Acute Ascorbic Acid Ingestion Increases Skeletal Muscle Blood Flow and Oxygen Consumption via Local Vasodilation during Graded Handgrip Exercise in Older Adults. Am. J. Physiology-Heart Circulatory Physiology 309, H360–H368. doi:10.1152/ajpheart.00209.2015
Richards J. C., Luckasen G. J., Larson D. G., Dinenno F. A. (2014). Role of α-adrenergic Vasoconstriction in Regulating Skeletal Muscle Blood Flow and Vascular Conductance during Forearm Exercise in Ageing Humans. J. Physiol. 592, 4775–4788. doi:10.1113/jphysiol.2014.278358
Richardson R. S., Kennedy B., Knight D. R., Wagner P. D. (1995). High Muscle Blood Flows Are Not Attenuated by Recruitment of Additional Muscle Mass. Am. J. Physiology-Heart Circulatory Physiology 269, H1545–H1552. doi:10.1152/ajpheart.1995.269.5.h1545
Richter E. A., Kiens B., Hargreaves M., Kjaer M. (1992). Effect of Arm-Cranking on Leg Blood Flow and Noradrenaline Spillover during Leg Exercise in Man. Acta Physiol. Scand. 144, 9–14. doi:10.1111/j.1748-1716.1992.tb09261.x
Rosenmeier J. B., Hansen J., González-Alonso J. (2004). Circulating ATP-Induced Vasodilatation Overrides Sympathetic Vasoconstrictor Activity in Human Skeletal Muscle. J. Physiol. 558, 351–365. doi:10.1113/jphysiol.2004.063107
Rowell L. B. (2004). Ideas about Control of Skeletal and Cardiac Muscle Blood Flow (1876-2003): Cycles of Revision and New Vision. J. Appl. Physiology 97, 384–392. doi:10.1152/japplphysiol.01220.2003
Saltin B., Blomqvist G., Mitchell J. H., Johnson R. L., Wildenthal K., Chapman C. B. (1968). Response to Exercise after Bed Rest and after Training. Circulation 38, VII1–78. doi:10.1161/01.cir.38.5s7.vii-1
Saltin B. (2007). Exercise Hyperaemia: Magnitude and Aspects on Regulation in Humans. J. Physiol. 583, 819–823. doi:10.1113/jphysiol.2007.136309
Saltin B., Mortensen S. P. (2012). Inefficient Functional Sympatholysis Is an Overlooked Cause of Malperfusion in Contracting Skeletal Muscle. J. Physiol. 590, 6269–6275. doi:10.1113/jphysiol.2012.241026
Sarma S., Levine B. D. (2015). Soothing the Sleeping Giant: Improving Skeletal Muscle Oxygen Kinetics and Exercise Intolerance in HFpEF. J. Appl. Physiology 119, 734–738. doi:10.1152/japplphysiol.01127.2014
Savard G. K., Richter E. A., Strange S., Kiens B., Christensen N. J., Saltin B. (1989). Norepinephrine Spillover from Skeletal Muscle during Exercise in Humans: Role of Muscle Mass. Am. J. Physiology-Heart Circulatory Physiology 257, H1812–H1818. doi:10.1152/ajpheart.1989.257.6.h1812
Schrage W. G., Eisenach J. H., Joyner M. J. (2007). Ageing Reduces Nitric-Oxide- and Prostaglandin-Mediated Vasodilatation in Exercising Humans. J. Physiol. 579, 227–236. doi:10.1113/jphysiol.2006.124313
Secher N. H., Clausen J. P., Klausen K., Noer I., Trap-Jensen J. (1977). Central and Regional Circulatory Effects of Adding Arm Exercise to Leg Exercise. Acta Physiol. Scand. 100, 288–297. doi:10.1111/j.1748-1716.1977.tb05952.x
Secher N. H., Volianitis S. (2006). Are the Arms and Legs in Competition for Cardiac Output? Med. Sci. Sports Exerc 38, 1797–1803. doi:10.1249/01.mss.0000230343.64000.ac
Shepherd J. R. A., Joyner M. J., Dinenno F. A., Curry T. B., Ranadive S. M. (2016). Prolonged Adenosine Triphosphate Infusion and Exercise Hyperemia in Humans. J. Appl. Physiology 121, 629–635. doi:10.1152/japplphysiol.01034.2015
Shoemaker J. K., Naylor H. L., Hogeman C. S., Sinoway L. I. (1999). Blood Flow Dynamics in Heart Failure. Circulation 99, 3002–3008. doi:10.1161/01.cir.99.23.3002
Skattebo Ø., Calbet J. A. L., Rud B., Capelli C., Hallén J. (2020). Contribution of Oxygen Extraction Fraction to Maximal Oxygen Uptake in Healthy Young Men. Acta Physiol. (Oxf) 230, e13486. doi:10.1111/apha.13486
Solomon S. D., McMurray J. J. V., Anand I. S., Ge J., Lam C. S. P., Maggioni A. P., et al. (2019). Angiotensin-Neprilysin Inhibition in Heart Failure with Preserved Ejection Fraction. N. Engl. J. Med. 381, 1609–1620. doi:10.1056/nejmoa1908655
Sprague R. S., Goldman D., Bowles E. A., Achilleus D., Stephenson A. H., Ellis C. G., et al. (2010). Divergent Effects of Low-O2 Tension and Iloprost on ATP Release from Erythrocytes of Humans with Type 2 Diabetes: Implications for O2 Supply to Skeletal Muscle. Am. J. Physiology-Heart Circulatory Physiology 299, H566–H573. doi:10.1152/ajpheart.00430.2010
Strait J. B., Lakatta E. G. (2012). Aging-associated Cardiovascular Changes and Their Relationship to Heart Failure. Heart Fail. Clin. 8, 143–164. doi:10.1016/j.hfc.2011.08.011
Sullivan M. J., Higginbotham M. B., Cobb F. R. (1988). Exercise Training in Patients with Severe Left Ventricular Dysfunction. Hemodynamic and Metabolic Effects. Circulation 78, 506–515. doi:10.1161/01.cir.78.3.506
Sullivan M. J., Knight J. D., Higginbotham M. B., Cobb F. R. (1989). Relation between Central and Peripheral Hemodynamics during Exercise in Patients with Chronic Heart Failure. Muscle Blood Flow Is Reduced with Maintenance of Arterial Perfusion Pressure. Circulation 80, 769–781. doi:10.1161/01.cir.80.4.769
Taivassalo T., Jensen T. D., Kennaway N., DiMauro S., Vissing J., Haller R. G. (2003). The Spectrum of Exercise Tolerance in Mitochondrial Myopathies: a Study of 40 Patients. Brain 126, 413–423. doi:10.1093/brain/awg028
Taylor J. A., Hand G. A., Johnson D. G., Seals D. R. (1992). Augmented Forearm Vasoconstriction during Dynamic Exercise in Healthy Older Men. Circulation 86, 1789–1799. doi:10.1161/01.cir.86.6.1789
Trinity J. D., Lee J. F., Pahnke M. D., Beck K. C., Coyle E. F. (2012). Attenuated Relationship between Cardiac Output and Oxygen Uptake during High-Intensity Exercise. Acta Physiol. 204, 362–370. doi:10.1111/j.1748-1716.2011.02341.x
Tucker W. J., Rosenberry R., Trojacek D., Chamseddine H. H., Arena‐Marshall C. A., Zhu Y., et al. (2019). Studies into the Determinants of Skeletal Muscle Oxygen Consumption: Novel Insight from Near‐infrared Diffuse Correlation Spectroscopy. J. Physiol. 597, 2887–2901. doi:10.1113/jp277580
Tyni-Lenné R., Dencker K., Gordon A., Jansson E., Sylvén C. (2001). Comprehensive Local Muscle Training Increases Aerobic Working Capacity and Quality of Life and Decreases Neurohormonal Activation in Patients with Chronic Heart Failure. Eur. J. Heart Fail. 3, 47–52. doi:10.1016/s1388-9842(00)00087-8
Tyni-Lenné R., Gordon A., Jensen-Urstad M., Dencker K., Jansson E., Sylvén C. (1999). Aerobic Training Involving a Minor Muscle Mass Shows Greater Efficiency Than Training Involving a Major Muscle Mass in Chronic Heart Failure Patients. J. Cardiac Fail. 5, 300–307. doi:10.1016/s1071-9164(99)91334-9
Volianitis S., Krustrup P., Dawson E., Secher N. H. (2003). Arm Blood Flow and Oxygenation on the Transition from Arm to Combined Arm and Leg Exercise in Humans. J. Physiology 547, 641–648. doi:10.1113/jphysiol.2002.034496
Volianitis S., Secher N. H. (2002). Arm Blood Flow and Metabolism during Arm and Combined Arm and Leg Exercise in Humans. J. Physiology 544, 977–984. doi:10.1113/jphysiol.2002.023556
Volianitis S., Yoshiga C. C., Nissen P., Secher N. H. (2004). Effect of Fitness on Arm Vascular and Metabolic Responses to Upper Body Exercise. Am. J. Physiology-Heart Circulatory Physiology 286, H1736–H1741. doi:10.1152/ajpheart.01001.2003
Wahren J., Saltin B., Jorfeldt L., Pernow B. (1974). Influence of Age on the Local Circulatory Adaptation to Leg Exercise. Scand. J. Clin. Laboratory Investigation 33, 79–86. doi:10.3109/00365517409114201
Wiener D. H., Fink L. I., Maris J., Jones R. A., Chance B., Wilson J. R. (1986). Abnormal Skeletal Muscle Bioenergetics during Exercise in Patients with Heart Failure: Role of Reduced Muscle Blood Flow. Circulation 73, 1127–1136. doi:10.1161/01.cir.73.6.1127
Williamson J., Raven P., Whipp B. (1996). Unaltered Oxygen Uptake Kinetics at Exercise Onset with Lower-Body Positive Pressure in Humans. Exp. Physiol. 81, 695–705. doi:10.1113/expphysiol.1996.sp003970
Keywords: O2 uptake kinetics, handgrip exercise, knee-extensor exercise, cycling, blood flow
Citation: Nyberg M and Jones AM (2022) Matching of O2 Utilization and O2 Delivery in Contracting Skeletal Muscle in Health, Aging, and Heart Failure. Front. Physiol. 13:898395. doi: 10.3389/fphys.2022.898395
Received: 17 March 2022; Accepted: 05 May 2022;
Published: 14 June 2022.
Edited by:
Michael E. Tschakovsky, Queen’s University, CanadaReviewed by:
Simon Green, Western Sydney University, AustraliaCopyright © 2022 Nyberg and Jones. This is an open-access article distributed under the terms of the Creative Commons Attribution License (CC BY). The use, distribution or reproduction in other forums is permitted, provided the original author(s) and the copyright owner(s) are credited and that the original publication in this journal is cited, in accordance with accepted academic practice. No use, distribution or reproduction is permitted which does not comply with these terms.
*Correspondence: Michael Nyberg, em55YkBub3Zvbm9yZGlzay5jb20=
Disclaimer: All claims expressed in this article are solely those of the authors and do not necessarily represent those of their affiliated organizations, or those of the publisher, the editors and the reviewers. Any product that may be evaluated in this article or claim that may be made by its manufacturer is not guaranteed or endorsed by the publisher.
Research integrity at Frontiers
Learn more about the work of our research integrity team to safeguard the quality of each article we publish.