- 1Department of Integrative Neurophysiology, Graduate School of Medical Sciences, Kanazawa University, Kanazawa, Japan
- 2Laboratory of Molecular Neuroscience, Medical Research Institute, Tokyo Medical and Dental University (TMDU), Tokyo, Japan
- 3Division of Integrated Omics Research, Research Center for Experimental Modeling of Human Disease, Kanazawa University, Kanazawa, Japan
- 4Division of Animal Disease Model, Research Center for Experimental Modeling of Human Disease, Kanazawa University, Kanazawa, Japan
The suprachiasmatic nucleus (SCN), the central circadian clock in mammals, is a neural network consisting of various types of GABAergic neurons, which can be differentiated by the co-expression of specific peptides such as vasoactive intestinal peptide (VIP) and arginine vasopressin (AVP). VIP has been considered as a critical factor for the circadian rhythmicity and synchronization of individual SCN neurons. However, the precise mechanisms of how VIP neurons regulate SCN circuits remain incompletely understood. Here, we generated ViptTA knock-in mice that express tetracycline transactivator (tTA) specifically in VIP neurons by inserting tTA sequence at the start codon of Vip gene. The specific and efficient expression of tTA in VIP neurons was verified using EGFP reporter mice. In addition, combined with Avp-Cre mice, ViptTA mice enabled us to simultaneously apply different genetic manipulations to VIP and AVP neurons in the SCN. Immunostaining showed that VIP is expressed at a slightly reduced level in heterozygous ViptTA mice but is completely absent in homozygous mice. Consistently, homozygous ViptTA mice showed impaired circadian behavioral rhythms similar to those of Vip knockout mice, such as attenuated rhythmicity and shortened circadian period. In contrast, heterozygous mice demonstrated normal circadian behavioral rhythms comparable to wild-type mice. These data suggest that ViptTA mice are a valuable genetic tool to express exogenous genes specifically in VIP neurons in both normal and VIP-deficient mice, facilitating the study of VIP neuronal roles in the SCN neural network.
Introduction
The SCN is a heterogeneous structure made up of various types of neurons (Antle and Silver, 2005). Almost all SCN neurons contain γ-aminobutyric acid (GABA) as a neurotransmitter. These neurons can be differentiated by the co-expression of specific peptides (Welsh et al., 2010). These include AVP-producing neurons located predominantly in the dorsomedial part or the shell of the SCN, as well as vasoactive intestinal peptide (VIP)-producing neurons and gastrin-releasing peptide (GRP)-producing neurons in the ventrolateral part or the core of the SCN. VIP is expressed in approximately 10% of SCN neurons (Abrahamson and Moore, 2001; Herzog et al., 2017). It is the most important contributor to the synchronization among SCN neurons (Harmar et al., 2002; Colwell et al., 2003; Aton et al., 2005; Maywood et al., 2006). VIP neurons receive direct projections from the retina, and VIP has been implicated in the photoentrainment of the central circadian clock of the SCN (Abrahamson and Moore, 2001; Colwell et al., 2003; Jones et al., 2015, 2018; Vosko et al., 2015). Therefore, mice deficient in VIP (Vip−/−) or its receptor (Vipr2−/−) demonstrate a variety of abnormalities in the circadian rhythmicity and synchrony, including arrhythmicity, multiple circadian periods, shortened free-running period, and reduced responsiveness to the light (Harmar et al., 2002; Colwell et al., 2003; Aton et al., 2005). However, the precise mechanisms of how VIP neurons regulate SCN circuits remain incompletely understood. Also, other neuropeptides, such as AVP and GRP, may play some roles in the intercellular communication of SCN neurons (Li et al., 2009; Maywood et al., 2011; Ono et al., 2016).
Genetic manipulation specific to VIP neurons is a powerful approach to studying these neurons’ functions. So far, genetically modified mice utilizing the Cre/loxP system and FLP/FRT system have been generated, namely, Vip-ires-Cre and Vip-ires-Flp mice, respectively (Taniguchi et al., 2011; He et al., 2016). The Tet system is another genetic engineering system in which tetracycline transactivator (tTA) binds tetracycline-responsive element (TRE) and activates the gene downstream of TRE (Gossen and Bujard, 1992; Aiba and Nakao, 2007). Moreover, the tTA activity can be turned off (Tet-off system) or turned on (reverse tTA, Tet-on system) in the presence of tetracycline or its analogue doxycycline. Here, we established a ViptTA knock-in mouse line in which tTA2 (Urlinger et al., 2000) is expressed specifically in VIP neurons. In combination with a series of genetically modified mice and viral vectors equipped with TRE-driven gene expression, ViptTA mice can express various exogenous genes specifically in VIP neurons. Furthermore, because the transcription of the endogenous Vip coding sequence was blocked in the ViptTA allele, homozygous mice of the line behaved as Vip-deficient mice. Thus, this new mouse line provides a valuable tool for the functional study of VIP neurons.
Materials and Methods
Animals
To generate ViptTA mice, we inserted a tTA2-polyA cassette at the start codon of Vip gene in its second exon by the CRISPR/Cas9-mediated targeting strategy (Figure 1A). The donor DNA was synthesized, containing tTA2 cDNA (Urlinger et al., 2000), SV40 polyA signal, and 300 bp sequences of the mouse Vip gene (NCBI Gene: 22353) 5′ and 3′ to the start codon. C57BL/6J mice were purchased from Japan SLC, Inc. (Shizuoka, Japan). One crRNA targeting exon 2 (5′- TCTTTTCAGAGGCACCGAGA -3′) of the mouse Vip gene was designed using the online sgRNA design tool available at https://crispr.mit.edu/ and purchased from Integrated DNA Technologies (Coralville, IA). The fertilized pronuclear-stage embryos were prepared by in vivo fertilization in human tubal fluid medium (ARK Resource; Kumamoto, Japan) with sperms from two C57BL/6J males and oocytes from five superovulated females injected with anti-inhibin serum and human chorionic gonadotropin. Next, the ssODN (40 ng/μl) and the complex of crRNAs (0.61 μM), tracrRNA (0.61 μM), and Cas9 protein (30 ng/μl) (Integrated DNA Technologies) in TE were injected into the nucleus of approximately two hundred pronuclear-stage embryos by microinjection. Embryos were then washed and cultured in potassium simplex optimization medium (ARK Resource) overnight. The obtained ninety-three 2-cell embryos were transferred to recipient mice. Fourteen mice were born and tested for the correct targeting of the tTA2-polyA cassette by genomic PCR and sequencing. Six out of 14 mice (F0) had a correct insertion of the cassette, and two F0 mice were bred with C57BL/6J mice to obtain F1 generation. These two lines backcrossed to C57BL/6J mice at least twice were used for further analyses. We did not discriminate between these two lines in this manuscript because they were indistinctive regarding the tTA2 expression and circadian behavior. To evaluate the specific expression of tTA2, ViptTA mice were crossed to Actb-tetO-EGFP reporter mice. Actb-tetO-EGFP reporter mice were generated from Actb-tetO-FLEX-EGFP mice (Aida et al., 2015) by crossing with germ cell-specific Prdm1-Cre mice (JAX 008827) to eliminate the Cre-dependency of EGFP expression.
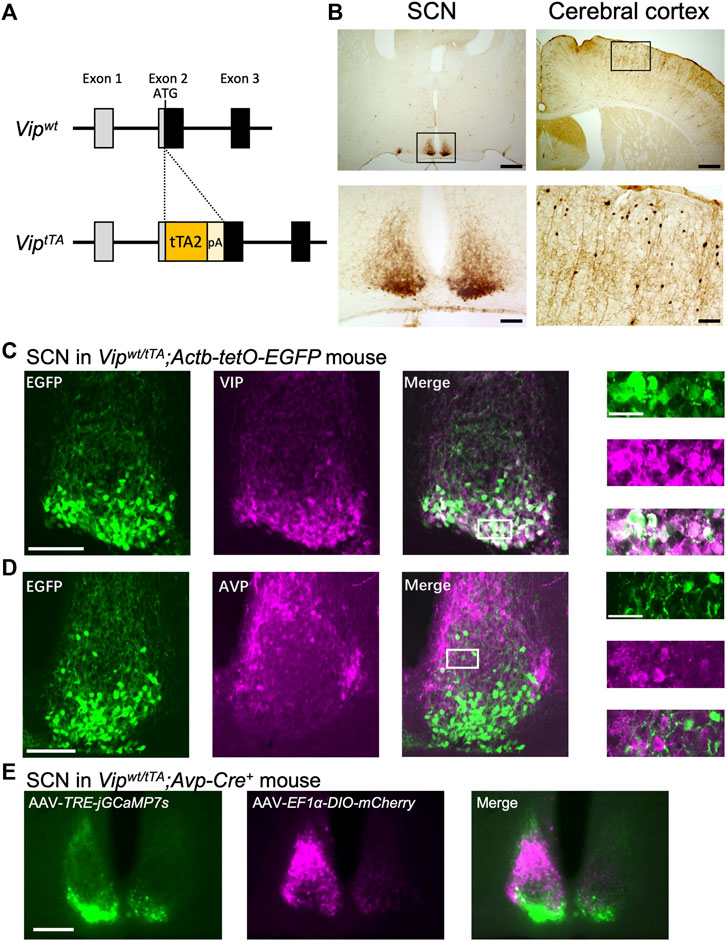
FIGURE 1. Generation and validation of ViptTA knock-in mice. (A) Targeting strategy for generating ViptTA mice. A tTA2-polyA cassette was inserted at the start codon of Vip gene in exon 2. (B) tTA-mediated EGFP expression was mostly restricted in the SCN and cerebral cortex in Vipwt/tTA mice crossed with Actb-tetO-EGFP reporter mice. Coronal brain sections were immunostained for GFP in brown. Lower panels (scale bar, 100 μm) are magnified images of regions indicated by rectangles in upper panels (scale bar, 500 μm). (C–D) Coronal brain sections prepared from Vipwt/tTA;Actb-tetO-EGFP mice were immunostained for VIP (C) or AVP (D) in magenta (middle). Native EGFP fluorescent images are shown on left. The white rectangles in the merged images (scale bar, 100 μm) indicate the regions of the enlarged images (far right; scale bar, 30 μm). For fluorescent immunostaining, mice were pretreated with intracerebroventricular injections of colchicine (40 μg in 1 μl saline) for 48 h before transcardial perfusion of 4% paraformaldehyde fixative. (E) Differential labeling of VIP and AVP neurons in the SCN of Vipwt/tTA;Avp-Cre+ mice. AAV-EF1α-DIO-mCherry and AAV-TRE-jGCaMP7s were injected unilaterally into the SCN in Vipwt/tTA;Avp-Cre+ mice. A representative coronal section of the SCN is shown (scale bar, 200 μm) (n = 3). Note that jGCaMP7s-labeled neurons (left, green) are in the SCN core, while mCherry-labeled neurons (middle, magenta) are in the SCN shell. These two populations of neurons rarely overlap (right).
Avp-Cre mice were reported previously (Mieda et al., 2015) and used in hemizygous condition. This line is a transgenic mouse harboring a modified BAC transgene, which has an insertion of codon-improved Cre recombinase gene immediately 5′ to the translation initiation codon of exogenous Avp gene in the BAC, but without manipulation of the endogenous Avp loci in the mouse.
All mice were maintained under a strict 12 h light/12 h dark (LD) cycle in a temperature- and humidity-controlled room and fed ad libitum. All experimental procedures involving animals were approved by the appropriate institutional animal care and use committees of Kanazawa University and Tokyo Medical and Dental University.
Immunohistochemistry
Immunostaining was performed as described previously (Mieda et al., 2015). Mice were sacrificed at approximately ZT5 by transcardial perfusion of PBS followed by 4% paraformaldehyde fixative. To examine the specificity of tTA2 expression by fluorescent immunostaining (in Figures 1C,D), Vipwt/tTA;Actb-tetO-EGFP mice were pretreated with intracerebroventricular colchicine injections (40 µg in 1 µl saline) for 48 h before perfusion fixation. Serial coronal brain sections (30 µm thick) were prepared with a cryostat (CM 1860, Leica) and collected in 4 series—one of which was further immunostained. The antibodies used were: rabbit anti-GFP (Thermo Fisher Scientific, 1:1,000), rabbit anti-AVP (Millipore, 1:4,000); rabbit anti-VIP (Immunostar, 1:1,000); biotinylated goat anti-rabbit IgG antibody (Vector Lab, 1:1,000), and Alexa 488-conjugated goat anti-rabbit IgG (Molecular Probes, 1:1,000). The expression of EGFP was detected by its native fluorescence for fluorescent immunostaining (Figures 1C,D). The VIP expression levels were quantified by Photoshop (Adobe) as follows (Figure 2B). First, the images were transformed to grayscale. Then, the mean intensities of pixels within the SCN were calculated. Finally, the values of the region lateral to the SCN were regarded as background and were subtracted from those of the SCN. For immunohistochemistry using diaminobenzidine (DAB) reactions (Figure 1B), color development was performed using the VECTASTAIN Elite ABC-HRP Kit (PK6100, Vector Lab) and DAB Substrate Kit (SK4100, Vector Lab). For Figures 1A,C,D representative optical section was imaged from each stained section by laser-confocal microscopy (Olympus, FluoView FV10i), then fluorescent cells in the images were counted. For Figures 1B, 2A, immunostaining of EGFP or VIP in sections was observed by epifluorescence or bright-field microscopy (KEYENCE, BZ-9000E).
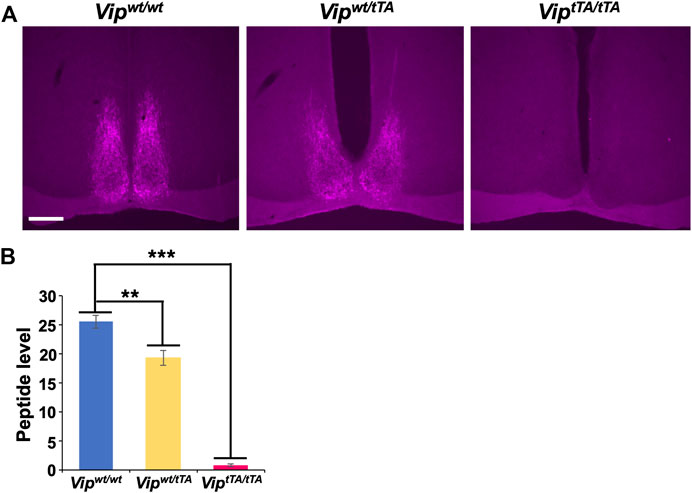
FIGURE 2. VIP expression is absent in ViptTA/ tTA mice. (A) Coronal brain sections containing the SCN prepared from Vipwt/wt, Vipwt/tTA, and ViptTA/tTA mice were immunostained for VIP (magenta; scale bar, 200 μm). Representative images are shown. (B) VIP immunostaining was slightly reduced in Vipwt/tTA (n = 4) compared to Vipwt/wt (n = 3) and almost absent in ViptTA/tTA (n = 5) mice. Error bars indicate SEM. **, p < 0.005; ***, p < 0.001 by one-way ANOVA followed by post-hoc Tukey tests.
Generation and Focal Injection of Recombinant AAV Vectors
The AAV-2 ITR-containing plasmid pAAV-TRE-ChR2-EYFP (Addgene #110339, a gift from Dr. Hyungbae Kwon) was modified to construct pAAV-TRE-jGCaMP7s by replacing a BamHI-HindIII fragment containing hChR2-EYFP cDNA with a BamHI-HindIII fragment containing jGCaMP7s cDNA amplified by PCR from the plasmid pGP-AAV-CAG-FLEX-jGCaMP7s-WPRE (Addgene #104495, a gift from Dr. Douglas Kim & GENIE Project), using the following primers: 5′- ataggatccgccaccATGggttctcatca -3′ and 5′- gcgaagctTCActtcgctgtcatcatttg -3′. pAAV-EF1a-DIO-mCherry was a gift from Dr. Bryan Roth (Addgene #50462).
Recombinant AAV vectors (AAV2-rh10), AAV-TRE-jGCaMP7s and AAV-EF1α-DIO-mCherry, were produced using a triple-transfection, helper-free method and purified as described previously (Mieda et al., 2015). The titers of recombinant AAV vectors were determined by quantitative PCR: AAV-TRE-jGCaMP7s, 6.3 × 1011; and AAV-EF1α-DIO-mCherry, 5.2 × 1012 genome copies/ml. Stereotaxic injection of AAV vectors into the SCN of Vipwt/tTA;Avp-Cre+ mice was performed as described previously (Maejima et al., 2021). Two weeks after surgery, coronal brain slices (100 µm thick) were prepared, and fluorescence of jGCaMP7s and mCherry was observed as described above.
Behavioral Analyses
Male and female, Vipwt/wt, Vipwt/tTA, and ViptTA/tTA littermates, aged 8–17 weeks, were individually housed in a polycarbonate cage placed in a light-tight box. Spontaneous movements in the homecage were monitored by infrared sensors (O’hara) in 1-min bins as described previously (Mieda et al., 2015). Actogram, activity profile, and χ2 periodogram analyses were performed via ClockLab (Actimetrics). The free-running period and amplitude (Qp values) were calculated for the last 10 days in constant darkness (DD) by periodogram. The activity onset was calculated from the daily activity profile (average pattern of activity) of the last 7 days in LD using the mean activity level as a threshold.
Statistics
All results are expressed as mean ± SEM. For comparison of three or four groups in Figures 2, 4, one-way ANOVA followed by Tukey post hoc tests were performed. Probability (p) values less than 0.05 were considered to be statistically significant. Only relevant information from the statistical analysis was indicated in the text and figures.
Results
Generation of ViptTA Knock-In Mice
We generated knock-in mice that express tTA2 (Urlinger et al., 2000) specifically in VIP neurons. To do this, we employed the CRISPR/Cas9-mediated homologous recombination to target the Vip gene of the mouse genome to insert a tTA2-polyA cassette at the start codon of Vip gene in its exon 2 (ViptTA) (Figure 1A). To localize tTA2 activity, we crossed Vipwt/tTA mice to Actb-tetO-EGFP reporter mice, which express EGFP in the presence of tTA. EGFP expression was mostly restricted in the SCN and the cerebral cortex in these mice (Figure 1B). The cerebral cortex contains a population of GABAergic interneurons that expresses VIP (Taniguchi et al., 2011). In contrast, there were few EGFP + cells in other regions where some neurons express VIP, such as the hippocampus and amygdala. In the SCN, EGFP expression was almost completely colocalized with VIP immunoreactivity (83.76 ± 0.91% of VIP + cells were also EGFP+, 87.11 ± 1.47% of EGFP + cells were also VIP+; counts of three SCN slices each from three mice) (Figure 1C). In contrast, there was almost no overlap with AVP immunoreactivity (2.45 ± 0.59% of AVP + cells were also EGFP+, 5.21 ± 1.16% of EGFP + cells were also AVP+) (Figure 1D). Thus, the expression of tTA2 occurred specifically and efficiently in VIP neurons within the SCN, confirming that ViptTA mice are a useful tool for VIP-neuron-specific genetic manipulations.
Differential Labeling of Vasoactive Intestinal Peptide and Arginine Vasopressin Neurons in the Suprachiasmatic Nucleus of Vipwt/tTA;Avp-Cre+ Mice
Next, we examined whether ViptTA mice are useful to simultaneously apply different genetic manipulations to VIP and AVP neurons nearby within the SCN. To do so, we crossed Vipwt/tTA mice to hemizygous Avp-Cre mice (Avp-Cre+) that express improved Cre recombinase specifically in AVP neurons (Mieda et al., 2015). Then, we focally injected two AAV vectors in the SCN of Vipwt/tTA;Avp-Cre+ mice: AAV-TRE-jGCaMP7s and AAV-EF1α-DIO-mCherry that express a green fluorescent Ca2+ indicator protein jGCaMP7s (Dana et al., 2019) and a red fluorescent protein mCherry in a tTA- and Cre-dependent manner, respectively. As expected, jGCaMP7s was expressed specifically in the SCN shell, where AVP neurons locate, whereas mCherry expression was restricted in the SCN core, where VIP neurons locate (Figure 1E). Furthermore, there was almost no overlap in the expression of these two proteins. Thus, we successfully labeled VIP and AVP neurons differentially within the SCN local circuit.
Homozygous ViptTA Mice Lack Vasoactive Intestinal Peptide Expression
The Vip coding sequence was interrupted by a tTA2-polyA sequence in the ViptTA allele, resulting in the expression of tTA2 under the Vip promoter. In other words, the ViptTA allele should act equivalently to a Vip knockout allele. Therefore, we next examined the VIP expression in heterozygous Vipwt/tTA and homozygous ViptTA/tTA mice by immunostaining. We found that VIP-immunoreactivity in Vipwt/tTA mice was slightly reduced (∼20%) compared to wild-type mice (Vipwt/wt) but was completely absent in ViptTA/tTA (Figure 2). These results suggested that the insertion of a tTA2-polyA sequence at the translation initiation site completely blocks the expression of VIP peptide.
Homozygous ViptTA Mice Show Impaired Circadian Rhythms
Previous studies of locomotor activity rhythm in Vip−/− mice have shown significantly attenuated circadian rhythmicity in constant darkness (DD), either with a shortened free-running period or multiple circadian periods (Colwell et al., 2003; Aton et al., 2005). A small number of Vip−/− mice even demonstrate arrhythmicity. Therefore, we next recorded the daily rhythm of spontaneous locomotor activity of Vipwt/wt, Vipwt/tTA, and ViptTA/tTA mice. In these experiments, mice were individually housed in cages, first in a 12 h/12 h light/dark (LD) cycle for 10–15 days. Under this condition, all groups were entrained to the LD cycle and exhibited nocturnal rhythms in their activity (Figure 3).
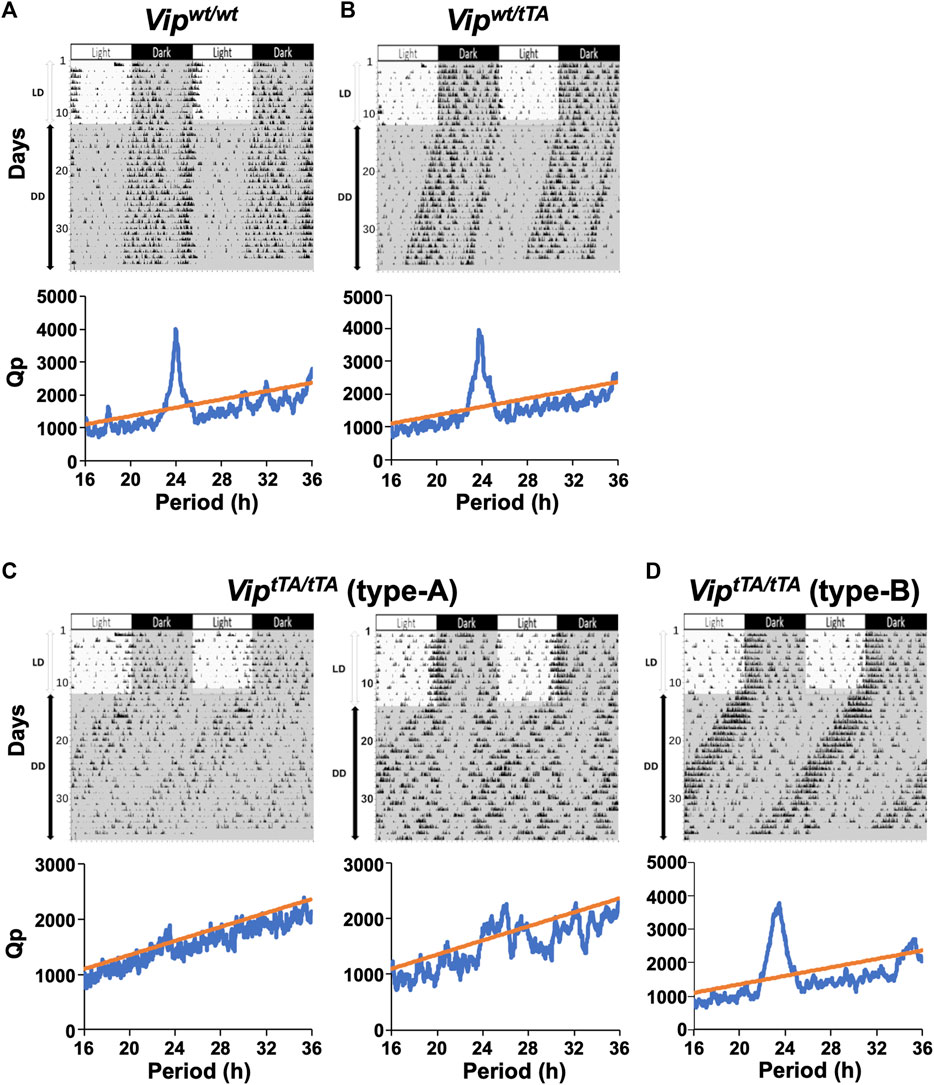
FIGURE 3. ViptTA/tTA mice demonstrate a variety of abnormalities in circadian rhythmicity and synchrony. (A–D) Representative actograms and periodograms of the locomotor activity rhythm of one Vipwt/wt (A), one Vipwt/tTA (B), two type-A ViptTA/tTA (C), and one type-B ViptTA/tTA mice. Animals were initially housed in 12:12 h light/dark (LD) conditions and then transferred to constant darkness (DD). Gray shadings in actograms indicate the time when lights were off. Periodograms are for the last 10 days in DD. Peaks above the diagonal line (indicating the 99.9% confidence level) between 16 and 36 h were considered significant circadian periods. Vipwt/wt and Vipwt/tTA mice show a clear dominant peak around 24 h (A,B). Note that type-A ViptTA/tTA mice expressed no clear period (i.e., arrhythmic; C left) or multiple periods (C right), whereas those of type-B showed more coherent circadian behavior, with a single, free-running period, although shortened (D).
Mice were then placed into DD, and their free-running rhythms were recorded. Under these conditions, several striking differences emerged in ViptTA/tTA mice compared to Vipwt/wt and Vipwt/tTA mice. All Vipwt/wt and Vipwt/tTA mice free-ran with a single, stable circadian period (Figures 3A,B). The free-running periods were comparable between Vipwt/wt (23.89 ± 0.03 h) and Vipwt/tTA mice (23.82 ± 0.02 h) (Figure 4B). In contrast, among ViptTA/tTA mice, one-half (5 of 12) exhibited multiple circadian periods or no statistically significant circadian period (type-A) (Figure 3C). The remaining half (7 of 12) free-ran with a single circadian period (type-B) (Figure 3D). However, the period of type-B ViptTA/tTA mice (23.30 ± 0.16 h) was significantly shorter than that of Vipwt/wt mice (Figure 4B).
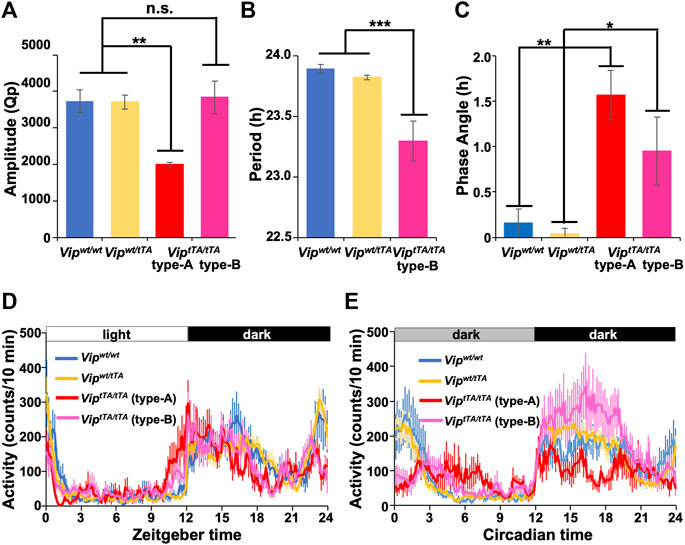
FIGURE 4. ViptTA/tTA mice show impaired circadian rhythms. (A) The circadian amplitude of rhythms was significantly reduced in type-A ViptTA/tTA mice (n = 5) compared to Vipwt/wt (n = 7), Vipwt/tTA (n = 12), and type-B ViptTA/tTA (n = 7) mice. Bars show circadian amplitudes at the dominant period as determined by χ2 periodogram analysis (Qp values). (B) Free-running period was shortened in type-B ViptTA/tTA mice. (C) Phase angle of entrainment under LD. (D–E) Daily profiles of circadian behavioral rhythms under LD (D) or DD (E). Error bars indicate SEM. *, p < 0.05; **, p < 0.005; ***, p < 0.001 by one-way ANOVA followed by post-hoc Tukey tests.
Type-A ViptTA/tTA mice (Qp 1997.28 ± 61.92) also showed circadian amplitude significantly lower than that of Vipwt/wt mice (Qp 3,733.60 ± 306.41) in DD (Figure 4A). In contrast, there was no significant difference between type-B ViptTA/tTA (Qp 3,840.01 ± 443.14) and Vipwt/wt mice. In addition, the circadian amplitude of Vipwt/tTA (Qp 3,706.82 ± 193.27) mice was not significantly different from Vipwt/wt mice (Figure 4A). The mean daily profiles of locomotor activity rhythm also demonstrated the attenuation of circadian rhythm of type-A ViptTA/tTA mice both in LD and DD (Figures 4C,D). In contrast, type-B ViptTA/tTA mice increased their locomotor activity during CT12∼18 (evening component) while they reduced that during CT21∼3 (morning component) in DD (Figure 4D). In LD, the activity onset was ∼1.5 h earlier in type-A and ∼0.8 h in type-B ViptTA/tTA mice compared to other groups (Figures 3C,D and Figures 4C,D).
Based on the above data, we concluded that the circadian behavior rhythm of Vipwt/tTA mice was not significantly different compared to Vipwt/wt mice. However, ViptTA/tTA mice were deficient in VIP expression and exhibited a variety of impairments in their circadian behavioral rhythms. The circadian phenotypes of ViptTA/tTA mice were all consistent with the previous reports concerning Vip−/− mice (Colwell et al., 2003; Aton et al., 2005).
Discussion
In this study, we generated ViptTA knock-in mice in which tTA2-coding sequence was introduced into the endogenous Vip locus. As expected, the expression of tTA in Vipwt/tTA mice was highly specific to VIP neurons in the SCN. Therefore, in combination with transgenic mice or viral vectors with TRE-mediated transgene expression, ViptTA mice can be used to express any protein specifically in VIP neurons. Furthermore, the double transgenic mice containing ViptTA and Avp-Cre enabled us to simultaneously apply different genetic manipulations to VIP and AVP neurons in the SCN. Such a dual-targeting strategy would be potent for the study of SCN, a small but complicated neuronal network consisting of multiple types of neurons, including VIP neurons. A series of Cre driver mice specific to a particular type of SCN neurons are currently available, such as Avp-Cre, Nms-Cre, Grp-Cre, Drd1a-Cre, and Vipr2-Cre (Taniguchi et al., 2011; Lee et al., 2015; Mieda et al., 2015; Smyllie et al., 2016; Inoue et al., 2018; Cheng et al., 2019). Therefore, by crossing with one of them, ViptTA mice would provide opportunities to directly examine the interactions between VIP neurons and another type of SCN neurons.
Homozygous ViptTA/tTA mice were VIP-deficient and behaved similarly to VIP−/- mice (Colwell et al., 2003; Aton et al., 2005). The neuropeptide VIP, which is produced by a part of retinorecipient neurons of the SCN, has been demonstrated to be especially important for the maintenance and synchronization of cellular clocks in individual SCN neurons (Aton et al., 2005; Maywood et al., 2006). Therefore, attenuated oscillation and synchronization of SCN neurons may account for the arrhythmicity and multiple circadian periods observed in the half of ViptTA/tTA mice (type-A). The short-period rhythmicity observed in type B ViptTA/tTA mice is also a feature common to one-third of VIP−/- mice. However, the cause of their shortened period remains unclear. The advanced activity onset of ViptTA/tTA mice in LD conditions may be due to their shortened circadian period and the reduction in photoentrainment, in which VIP signaling has been implicated (Colwell et al., 2003; Jones et al., 2015, 2018; Vosko et al., 2015). Thus, ViptTA/tTA mice provide a novel model of VIP-deficiency in which we can target genetic manipulations to the putative VIP neurons. In contrast, heterozygous Vipwt/tTA mice were comparable to wild-type mice in the VIP expression and circadian behavior. Therefore, Vipwt/tTA mice enable us to target genetic manipulations to VIP neurons without influencing circadian behavior.
In summary, we generated a novel mouse line ViptTA. By using it, we can target exogenous gene expression to VIP neurons in both normal and VIP-deficient mice. In addition to the previously developed Vipires-Cre and Vipires-Flp mice (Taniguchi et al., 2011; He et al., 2016), ViptTA mice are the third genetic tool based on the Tet system for manipulating VIP neurons. It will expand the opportunity for the genetic dissection of complex neuronal networks, such as the SCN, in combination with other genetic tools utilizing the Cre/loxP or FLP/FRT system.
Data Availability Statement
The original contributions presented in the study are included in the article, further inquiries can be directed to the corresponding author.
Ethics Statement
The animal study was reviewed and approved by Animal care and use committees of Kanazawa University and Tokyo Medical and Dental University.
Author Contributions
YP and MM designed research; YP, YT, AM, SH, TD, and MM performed research; YP and MM analyzed data; YH and KT provided an essential resource; and YP, SH, TD, and MM wrote the paper.
Funding
This study was supported in part by MEXT/JSPS KAKENHI Grant Numbers 18K19421, 19H03399, 20K21498; by the Takeda Science Foundation; by the Daiichi Sankyo Foundation of Life Science; and by Kanazawa University CHOZEN project (MM); by Nanken-Kyoten, TMDU, Grant Numbers 2020-13, 2021-09 (MM, KT); by KAKENHI 20K07259 (YT).
Conflict of Interest
The authors declare that the research was conducted in the absence of any commercial or financial relationships that could be construed as a potential conflict of interest.
Publisher’s Note
All claims expressed in this article are solely those of the authors and do not necessarily represent those of their affiliated organizations, or those of the publisher, the editors and the reviewers. Any product that may be evaluated in this article, or claim that may be made by its manufacturer, is not guaranteed or endorsed by the publisher.
Acknowledgments
We thank K. Hyungbae for pAAV-TRE-ChR2-EYFP; B. Roth for pAAV-DIO-mCherry; D. Kim and GENIE Project for pGP-AAV-CAG-FLEX-jGCaMP7s-WPRE; Penn Vector Core for pAAV2-rh10; and H. Okamoto for technical support to generate Avp-Cre mouse. We thank T. Maejima for discussion; M. Fukushi, M. Kawabata, J. Terakawa, and Y. Inoue for technical assistance.
References
Abrahamson E. E., Moore R. Y. (2001). Suprachiasmatic Nucleus in the Mouse: Retinal Innervation, Intrinsic Organization and Efferent Projections. Brain Res. 916, 172–191. doi:10.1016/s0006-8993(01)02890-6
Aiba A., Nakao H. (2007). Conditional Mutant Mice Using Tetracycline-Controlled Gene Expression System in the Brain. Neurosci. Res. 58, 113–117. doi:10.1016/J.NEURES.2007.01.009
Aida T., Chiyo K., Usami T., Ishikubo H., Imahashi R., Wada Y., et al. (2015). Cloning-free CRISPR/Cas System Facilitates Functional Cassette Knock-In in Mice. Genome Biol. 16, 87. doi:10.1186/S13059-015-0653-X
Antle M. C., Silver R. (2005). Orchestrating Time: Arrangements of the Brain Circadian Clock. Trends Neurosciences 28, 145–151. doi:10.1016/j.tins.2005.01.003
Aton S. J., Colwell C. S., Harmar A. J., Waschek J., Herzog E. D. (2005). Vasoactive Intestinal Polypeptide Mediates Circadian Rhythmicity and Synchrony in Mammalian Clock Neurons. Nat. Neurosci. 8, 476–483. doi:10.1038/nn1419
Balsalobre A., Damiola F., Schibler U. (1998). A Serum Shock Induces Circadian Gene Expression in Mammalian Tissue Culture Cells. Cell 93, 929–937. doi:10.1016/S0092-8674(00)81199-X
Cheng A. H., Fung S. W., Cheng H.-Y. M. (2019). Limitations of the Avp-IRES2-Cre (JAX #023530) and Vip-IRES-Cre (JAX #010908) Models for Chronobiological Investigations. J. Biol. Rhythms 34, 634–644. doi:10.1177/0748730419871184
Colwell C. S., Michel S., Itri J., Rodriguez W., Tam J., Lelievre V., et al. (2003). Disrupted Circadian Rhythms in VIP- and PHI-Deficient Mice. Am. J. Physiology-Regulatory, Integr. Comp. Physiol. 285, R939–R949. doi:10.1152/ajpregu.00200.2003
Dana H., Sun Y., Mohar B., Hulse B. K., Kerlin A. M., Hasseman J. P., et al. (2019). High-performance Calcium Sensors for Imaging Activity in Neuronal Populations and Microcompartments. Nat. Methods 16, 649–657. doi:10.1038/s41592-019-0435-6
Gossen M., Bujard H. (1992). Tight Control of Gene Expression in Mammalian Cells by Tetracycline-Responsive Promoters. Proc. Natl. Acad. Sci. U.S.A. 89, 5547–5551. doi:10.1073/PNAS.89.12.5547
Harmar A. J., Marston H. M., Shen S., Spratt C., West K. M., Sheward W. J., et al. (2002). The VPAC2 Receptor Is Essential for Circadian Function in the Mouse Suprachiasmatic Nuclei. Cell 109, 497–508. doi:10.1016/s0092-8674(02)00736-5
He M., Tucciarone J., Lee S., Nigro M. J., Kim Y., Levine J. M., et al. (2016). Strategies and Tools for Combinatorial Targeting of GABAergic Neurons in Mouse Cerebral Cortex. Neuron 92, 555. doi:10.1016/J.NEURON.2016.10.009
Herzog E. D., Hermanstyne T., Smyllie N. J., Hastings M. H. (2017). Regulating the Suprachiasmatic Nucleus (SCN) Circadian Clockwork: Interplay between Cell-Autonomous and Circuit-Level Mechanisms. Cold Spring Harb. Perspect. Biol. 9, a027706. doi:10.1101/cshperspect.a027706
Inoue R., Abdou K., Hayashi-Tanaka A., Muramatsu S.-i., Mino K., Inokuchi K., et al. (2018). Glucocorticoid Receptor-Mediated Amygdalar Metaplasticity Underlies Adaptive Modulation of Fear Memory by Stress. Elife 7, e34135. doi:10.7554/ELIFE.34135
Jones J. R., Simon T., Lones L., Herzog E. D. (2018). SCN VIP Neurons Are Essential for normal Light-Mediated Resetting of the Circadian System. J. Neurosci. 38, 7986–7995. doi:10.1523/JNEUROSCI.1322-18.2018
Jones J. R., Tackenberg M. C., Mcmahon D. G. (2015). Manipulating Circadian Clock Neuron Firing Rate Resets Molecular Circadian Rhythms and Behavior. Nat. Neurosci. 18, 373–375. doi:10.1038/nn.3937
Lee I. T., Chang A. S., Manandhar M., Shan Y., Fan J., Izumo M., et al. (2015). Neuromedin S-Producing Neurons Act as Essential Pacemakers in the Suprachiasmatic Nucleus to Couple Clock Neurons and Dictate Circadian Rhythms. Neuron 85, 1086–1102. doi:10.1016/j.neuron.2015.02.006
Li J. D., Burton K. J., Zhang C., Hu S. B., Zhou Q. Y. (2009). Vasopressin Receptor V1a Regulates Circadian Rhythms of Locomotor Activity and Expression of Clock-Controlled Genes in the Suprachiasmatic Nuclei. Am. J. Physiology-Regulatory, Integr. Comp. Physiol. 296, R824–R830. doi:10.1152/ajpregu.90463.2008
Maejima T., Tsuno Y., Miyazaki S., Tsuneoka Y., Hasegawa E., Islam M. T., et al. (2021). GABA from Vasopressin Neurons Regulates the Time at Which Suprachiasmatic Nucleus Molecular Clocks Enable Circadian Behavior. Proc. Natl. Acad. Sci. U.S.A. 118, e2010168118. doi:10.1073/pnas.2010168118
Maywood E. S., Chesham J. E., O'Brien J. A., Hastings M. H. (2011). A Diversity of Paracrine Signals Sustains Molecular Circadian Cycling in Suprachiasmatic Nucleus Circuits. Proc. Natl. Acad. Sci. U.S.A. 108, 14306–14311. doi:10.1073/pnas.1101767108
Maywood E. S., Reddy A. B., Wong G. K. Y., O'Neill J. S., O'Brien J. A., McMahon D. G., et al. (2006). Synchronization and Maintenance of Timekeeping in Suprachiasmatic Circadian Clock Cells by Neuropeptidergic Signaling. Curr. Biol. 16, 599–605. doi:10.1016/j.cub.2006.02.023
Mieda M., Ono D., Hasegawa E., Okamoto H., Honma K.-i., Honma S., et al. (2015). Cellular Clocks in AVP Neurons of the SCN Are Critical for Interneuronal Coupling Regulating Circadian Behavior Rhythm. Neuron 85, 1103–1116. doi:10.1016/j.neuron.2015.02.005
Ono D., Honma S., Honma K. I. (2016). Differential Roles of AVP and VIP Signaling in the Postnatal Changes of Neural Networks for Coherent Circadian Rhythms in the SCN. Sci. Adv. 2, 1–13. doi:10.1126/sciadv.1600960
Reppert S. M., Weaver D. R. (2002). Coordination of Circadian Timing in Mammals. Nature 418, 935–941. doi:10.1038/nature00965
Smyllie N. J., Chesham J. E., Hamnett R., Maywood E. S., Hastings M. H. (2016). Temporally Chimeric Mice Reveal Flexibility of Circadian Period-Setting in the Suprachiasmatic Nucleus. Proc. Natl. Acad. Sci. U.S.A. 113, 3657–3662. doi:10.1073/pnas.1511351113
Taniguchi H., He M., Wu P., Kim S., Paik R., Sugino K., et al. (2011). A Resource of Cre Driver Lines for Genetic Targeting of GABAergic Neurons in Cerebral Cortex. Neuron 71, 995–1013. doi:10.1016/j.neuron.2011.07.026
Urlinger S., Baron U., Thellmann M., Hasan M. T., Bujard H., Hillen W. (2000). Exploring the Sequence Space for Tetracycline-dependent Transcriptional Activators: Novel Mutations Yield Expanded Range and Sensitivity. Proc. Natl. Acad. Sci. U.S.A. 97, 7963–7968. doi:10.1073/PNAS.130192197
Vosko A., van Diepen H. C., Kuljis D., Chiu A. M., Heyer D., Terra H., et al. (2015). Role of Vasoactive Intestinal Peptide in the Light Input to the Circadian System. Eur. J. Neurosci. 42, 1839–1848. doi:10.1111/EJN.12919
Keywords: circadian rhythm, biological clock, vasoactive intestinal peptide, suprachiasmatic nucleus, Tet system, genetically engineered mice, neural circuit, cerebral cortex
Citation: Peng Y, Tsuno Y, Matsui A, Hiraoka Y, Tanaka K, Horike S-i, Daikoku T and Mieda M (2022) Cell Type-Specific Genetic Manipulation and Impaired Circadian Rhythms in ViptTA Knock-In Mice. Front. Physiol. 13:895633. doi: 10.3389/fphys.2022.895633
Received: 14 March 2022; Accepted: 18 April 2022;
Published: 03 May 2022.
Edited by:
Wataru Nakamura, Nagasaki University, JapanReviewed by:
Jorge Mendoza, Université de Strasbourg, FranceRyosuke Enoki, National Institutes of Natural Sciences, Japan
Copyright © 2022 Peng, Tsuno, Matsui, Hiraoka, Tanaka, Horike, Daikoku and Mieda. This is an open-access article distributed under the terms of the Creative Commons Attribution License (CC BY). The use, distribution or reproduction in other forums is permitted, provided the original author(s) and the copyright owner(s) are credited and that the original publication in this journal is cited, in accordance with accepted academic practice. No use, distribution or reproduction is permitted which does not comply with these terms.
*Correspondence: Michihiro Mieda, bWllZGFAbWVkLmthbmF6YXdhLXUuYWMuanA=