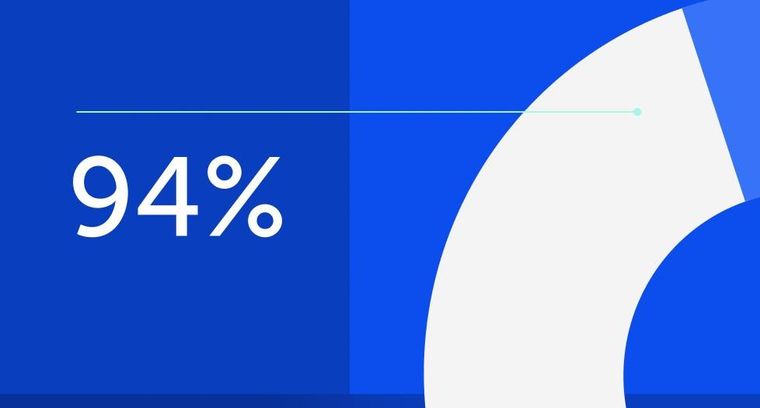
94% of researchers rate our articles as excellent or good
Learn more about the work of our research integrity team to safeguard the quality of each article we publish.
Find out more
MINI REVIEW article
Front. Physiol., 06 June 2022
Sec. Exercise Physiology
Volume 13 - 2022 | https://doi.org/10.3389/fphys.2022.894921
This article is part of the Research TopicRising Stars in Exercise PhysiologyView all 9 articles
Immersion water sports involve long-term apneas; therefore, athletes must physiologically adapt to maintain muscle oxygenation, despite not performing pulmonary ventilation. Breath-holding (i.e., apnea) is common in water sports, and it involves a decrease and increases PaO2 and PaCO2, respectively, as the primary signals that trigger the end of apnea. The principal physiological O2 sensors are the carotid bodies, which are able to detect arterial gases and metabolic alterations before reaching the brain, which aids in adjusting the cardiorespiratory system. Moreover, the principal H+/CO2 sensor is the retrotrapezoid nucleus, which is located at the brainstem level; this mechanism contributes to detecting respiratory and metabolic acidosis. Although these sensors have been characterized in pathophysiological states, current evidence shows a possible role for these mechanisms as physiological sensors during voluntary apnea. Divers and swimmer athletes have been found to displayed longer apnea times than land sports athletes, as well as decreased peripheral O2 and central CO2 chemoreflex control. However, although chemosensitivity at rest could be decreased, we recently found marked sympathoexcitation during maximum voluntary apnea in young swimmers, which could activate the spleen (which is a reservoir organ for oxygenated blood). Therefore, it is possible that the chemoreflex, autonomic function, and storage/delivery oxygen organ(s) are linked to apnea in immersion water sports. In this review, we summarized the available evidence related to chemoreflex control in immersion water sports. Subsequently, we propose a possible physiological mechanistic model that could contribute to providing new avenues for understanding the respiratory physiology of water sports.
Immersion sports, such as apnea diving, artistic swimming, classical swimming, or long-term swimming, involve acute and chronic cardiorespiratory and muscular adjustments (Viana et al., 2019; Wasfy et al., 2019; Elia et al., 2021). All of these sports activities involve continuous and/or intermittent long-term apneas that are concomitant with stern exercise efforts during several training sessions and competitions (Guimard et al., 2014). Of note, and in contrast to land sports, swimmer athletes are able to maintain O2 supplies to active tissues during exercise, although they do not perform pulmonary ventilation for several seconds or minutes (approximately 4 min) (Heusser et al., 2009), which could confer robust storage/delivery oxygen to active muscles (Schagatay et al., 2000; Andersson et al., 2002; Engan et al., 2013; Konstantinidou and Chairopoulou, 2017). Similarly, it has been demonstrated that during a maximum breath-hold, alveolar O2 ventilation can decrease to 30 mmHg, and oxygen saturation can also decrease to 50% (Ferretti et al., 1991). However, despite the abrupt decrease in alveolar ventilation and arterial desaturation, skeletal muscle functionality is preserved (Ferretti, 2001; Kjeld et al., 2018). In addition, arterial CO2 accumulation and the decrease in O2 during a breath hold can stimulate central and peripheral chemoreceptors, respectively, thus triggering autonomic and cardiovascular adjustments, which may correspondingly uncouple the end of apnea (Dempsey et al., 2012; Kumar and Prabhakar, 2012).
The principal peripheral chemoreceptors are the carotid bodies (CB), which are bilaterally located on the common carotid artery bifurcation (Iturriaga et al., 2021). This organ is composed of chemoreceptor type I cells and glial cells (type II cells) (Iturriaga and Alcayaga, 2004; Iturriaga et al., 2021). Carotid body type I cells are considered to be polymodal receptors and can respond to several stimuli, due to the fact that it is the main component of the homeostatic acute oxygen-sensing system required to trigger cardiorespiratory and ventilatory adjustments during hypoxemia (López-Barneo et al., 2008; Iturriaga et al., 2016; López-Barneo et al., 2016). Furthermore, central chemoreceptors are found in different areas of the brainstem; nevertheless, it has been proposed that the retrotrapezoid nucleus (RTN) is the more important site for regulating central chemosensitivity to CO2/H+ (Guyenet, 2012). Central respiratory chemosensitivity refers to the homeostatic reflex by which brainstem circuits regulate breathing in response to changes in CO2 or its proxy H+ (Kumar et al., 2015). Although peripheral/central chemoreceptors could be determinants of the maximum apnea duration, there is no conclusive evidence showing their role in apnea duration in water sports. Nevertheless, we recently showed that, in accordance with a longer apnea duration, the chemoreflex is reduced in swimmers’ athletes compared to a control condition (Arce-Álvarez et al., 2021). Of note, this adaptative process is not only observed in mammals, if not also in amphibian and reptile species (Santin, 2017), showing neuroplasticity impacting CO2/O2 chemoreflex. Indeed, it has been speculated to increase breath-hold duration to lengthen dive time adaptively for these animals. Therefore, and considering this parallelism between mammals and amphibians, it is possible to propose that peripheral/central chemoreflex function partially governs the apnea duration in swimmer and/or diver athletes. Therefore, and considering this parallelism between mammals and amphibians, it is possible to propose that peripheral/central chemoreflex function partially governs the apnea duration in swimmer and/or diver athletes. Nevertheless, although several mechanisms have been proposed to explain apnea and its breakpoint, this mini-review focuses on the responses and possible adaptations of the central and peripheral chemoreflex and their possible role to activate the spleen in maintaining respiration; additionally, we will explore and discuss potential mechanisms to explain a breath-hold in divers and swimmers’ athletes.
The carotid body is a bilateral sensory organ, with an estimated average volume of approximately 20 mm3, and it is located in the common carotid artery bifurcation and innervated by the carotid sinus nerve (Iturriaga et al., 2021; Forbes and Menezes, 2021; Joyner et al., 2018; Ortega-Sáenz and López-Barneo, 2020). These organs are the main oxygen sensors and mostly respond to hypoxemia, thus promoting hyperventilation and sympathoexcitation, which contribute to restoring arterial blood gas homeostasis (Iturriaga et al., 2016; Iturriaga et al., 2021; Ortega-Sáenz and López-Barneo, 2020). CB chemoreceptors are composed of chemoreceptor type I cells and glial cells (type II cells) (Iturriaga and Alcayaga, 2004; Iturriaga and Alcayaga, 2021; Ortega-Sáenz and López-Barneo, 2020). CB type I cells are considered to be polymodal receptors that respond to hypoxemia, hypercapnia, acidosis, blood flow, temperature, leptin and insulin concentrations, osmolality, and lactate; additionally, they are the main component of the homeostatic acute oxygen-sensing system required to produce cardiorespiratory and ventilatory adjustments during several stimuli (López-Barneo et al., 2008; Iturriaga et al., 2016; Iturriaga et al., 2021; Ortega-Sáenz and López-Barneo, 2020; Torres-Torrelo et al., 2021). Furthermore, type II cells or glia-like stem cells mainly have a functional role, and although their function is not completely known, they have been associated with the adaptive growth processes of CB in prolonged hypoxic stimuli (Xu et al., 2003; Pardal et al., 2007). Several hypotheses have been proposed to explain the mechanism related to the excitation of CB type I cells during hypoxia (Rakoczy and Wyatt, 2018). Chang et al. (2015) proposed that hypoxia inhibits electron transport of the mitochondria, which favors the formation of lactate, which activates the Olfr78 receptor, producing the inhibition of K+ channels, depolarizing the cell, and releasing neurotransmitters in CB type 1 cells (Chang et al., 2015). In addition, Wyatt and Buckler (2004), propose that hypoxia-induced inhibition of electron transport reduces ATP production and closes weakly rectifying K+ channels (TWIK)-related acid-sensing K+ (TASK), promoting depolarization of the cell and the release of neurotransmitters (Wyatt and Buckler, 2004). Interestingly, it has been proposed that the inhibition of mitochondrial electron transport and the consequent fall in ATP activates AMPK, which phosphorylates membrane ion channels causing depolarization and release of neurotransmitters (Evans et al., 2016; Wyatt et al., 2007). On the other hand, it has been proposed that hypoxia is able to inhibit mitochondrial electron transport, which increases reactive oxygen species, reducing the nucleotides of mitochondrial complex 1, modifying the redox state of the membrane channels, exciting the cell (Fernandez-Aguera et al., 2015). However, it is important to mention that independent of all these possible mechanisms, The most accepted mechanism related to glomus cell depolarization during hypoxia (PaO2 below 60 Torr or pH below 7.20) is related to decreased K+ permeability and increases permeability and Ca2+ influx. Carotid sinus afferent fibers, which project to nucleus of the solitary tract (NTS), trigger the excitation of NTS neurons and finally hyperventilation (Figure 1) (Gourine and Funk, 2017; Zera et al., 2019). The NTS is an integrating center that receives information sensed by the CB and stimulates other respiratory centers to trigger the hypoxic ventilatory response (HVR). Alternatively, Torres-Torrelo et al. (2021) proposed a novel CB activation model, which theorizes the existence of a metabolic pathway that is mediated through lactate signaling. Indeed, the authors proposed that lactate is transported into the cells by monocarboxylate transporters 2 and 4, which are rapidly converted to pyruvate with the production of NADH, which correspondingly activates membrane cation channels to produce cell depolarization (Torres-Torrelo et al., 2021). Notably, the increase in NADH production may be facilitated by intracellular acidification and promoted by hypoxemia (Torres-Torrelo et al., 2021). In addition, pyruvate can also increase the production of reactive oxygen species in the mitochondria, which could also contribute to the activation of CB glomus cells (Iturriaga et al., 2021; Torres-Torrelo et al., 2021). Although the CB activation mechanism could be different, the NTS, through glutamatergic synapses toward the RVLM, increases sympathetic discharge to the heart and blood vessels, which is concomitant with an increase in the respiratory drive (Figure 1). Therefore, the CB-mediated control of ventilation and sympathoexcitation is finely coordinated between the peripheral sensors and neuronal nuclei at the brainstem.
FIGURE 1. Mechanism of signal transduction and cell excitability of the peripheral chemoreceptor cells. Peripheral chemoreflex signal transduction mechanism in which type 1 glomus cells are activated by hypoxia (i.e., decrease O2 bioavailability) and metabolic stress (i.e., lactate) (left panel). Of note, hypoxia inhibits the mitochondrial electron transport, decreasing ATP production, which promotes lactate production and activation of adenosine monophosphate kinase (AMPK). Metabolic shift-dependent lactate production and accumulation which is transported through monocarboxylate cotransporter type 1 (MCT1) increase NADH production, concomitant to AMPK, are the molecular entities responsible for closing K+ channels, activating voltage-dependent calcium channel (Ca+2 influx to the cell). In addition, type II cells express Pannexin 1 (Panx-1), by which ATP is released, affecting ATP-dependent K+ channels. All this mechanism contributed to induces the release of neurotransmitters such as acetylcholine, dopamine, and adenosine by exocytosis to the carotid sinus nerve. Mechanism of signal transduction and cell excitability of the Central chemoreceptor cells (right panel). Neurons from the retrotrapezoid nucleus (RTN) are activated by CO2 and its proxy H+ in the cerebrospinal fluid (pH-sensitive). Acidosis activates the related G protein-coupled receptors 4 (GRP4), which produces closure of tandem pore domain in weakly rectifying K+ channels (TWIK), consequently depolarizing the membrane and opening of Ca+2 channels, inducing the release of neurotransmitters by exocytosis, promoting hyperventilation and sympathoexcitation. In addition, RTN neurons possibly also respond to changes in extracellular HCO3− concentration by a K+-independent mechanism. Of note, central chemotransduction, algo could be dependent on the astrocytes. The increase of PaCO2, promotes an increase of H+ production activating the Na+/Ca2+ exchanger (NCX), producing the influx of Ca2+, which allows the release of ATP by exocytosis from the astrocyte towards extracellular space. The ATP released by the astrocytes activates purinergic receptors of the RTN chemoreceptor neurons, triggering depolarization of these neurons and stimulating the central pattern generator (CPG).
In addition, it has been shown that the sensitivity of the central chemoreceptor to hypercapnia is also dependent on CB afferent activity to control ventilation (Paula-Ribeiro and Rocha, 2016). The interaction between both chemoreflex mechanisms is still controversial. However, the activation of peripheral and/or central chemoreceptors is apparently dependent on each other. In support of this notion, Smith et al. (2015) studied this interaction in non-anesthetized dogs by stimulating central chemoreceptors with hypercapnia, through a vascularly isolated and extracorporeally-perfused CBs preparation. They concluded that the relationship between central and peripheral chemoreflex is hyperaddictive or synergistic where stimulation of one chemoreflex increases the response of the other (Smith et al., 2015). However, evidence is limited regarding the adaptations of these mechanisms associated with breath-hold at rest and during exercise. Of note, our group recently showed that swimmer athletes displayed a decrease in CB-mediated respiratory responses to hypoxia compared to a control condition (Arce-Alvarez et al., 2021). Therefore, when considering the evidence, it is possible to hypothesize that these athletes displayed both peripheral (CB sensor) and/or central alterations, which could confer greater resistance to the early termination of maximum voluntary apnea.
Central respiratory chemosensitivity refers to a homeostatic reflex by which brainstem circuits regulate breathing in response to changes in CO2, HCO3- or pH of cerebrospinal fluid (Kumar et al., 2015; Goncalves and Mulkey, 2018). Central chemoreceptors are located in different brain areas including the brainstem, ventral medulla, cerebellum, hypothalamus, and midbrain (Nattie and Li, 2012; Gourine and Dale, 2022). Neuronal and glial groups of the retrotrapezoid nucleus (RTN), solitary tract nucleus (NTS), locus coeruleus, fastigial nucleus, or pre-Bötzinger are cell groups that generate the ventilatory reflex adjustments under different insults. Despite the numerous nuclei with chemosensory cells, the RTN is considered a critical nucleus in central chemoreception (Guyenet, 2012) due to this area accounts for ∼90% of the total central chemoreflex drive during exposure to hypercapnia (Kumar et al., 2015). Neurons in this nucleus detect brain extracellular fluid acidification by increasing PaCO2, thus promoting ventilatory adjustments to regulate CO2 excretion (Kumar et al., 2015). Mechanistically, RTN neuron chemosensitivity depends on the expression of proton-sensing G-protein coupled receptor type 4, which is activated by an increase of [H+] in the cerebrospinal fluid; this effect correspondingly produces a closed tandem pore domain in weakly rectifying K+ channels (TWIK), increasing the membrane potential and producing neuron depolarization and finally hyperventilation (Kumar et al., 2015) (Figure 1). Interestingly, Goncalves and Mulkey (2018) showed that RTN neurons possibly also respond to changes in extracellular HCO3- by mechanisms independent of K+ sensing. Particularly, they showed that changes in HCO3-, above or below physiological levels, modify the activity of the chemosensitive neurons of the RTN; however, the mechanistic evidence that this signaling pathway is still limited (Goncalves and Mulkey, 2018). In addition, RTN neurons can control breathing patterns through a glutamatergic projection to the pontomedullary regions (Guyenet, 2012). Indeed, RTN depolarization of glutamatergic neurons produces an activation of the respiratory pattern generator and sympathoexcitatory vasomotor neurons from the rostral ventrolateral medulla, thus generating sympathoexcitation toward tissues and causing a positive chronotropic and inotropic effect of the heart, which increases the tone of blood vessels in accordance with hyperventilation (Moreira et al., 2006) (Figure 1). In addition, glial cells from RTN have also been shown to play an important role in central chemoreception signaling. Current evidence shows that RTN astrocytes cells also have chemosensory functions and respond to changes in CO2 and H+ (Gourine and Dale, 2022). Nevertheless, the mechanism by which astrocytes participate in the respiratory drive induced by hypercapnia is not well described. However, it has been proposed that CO2-induced intracellular acidification of astrocyte cells stimulates Na+ influx mediated by the opening of the Na+/HCO3- NBC cotransporter (Gourine and Dale, 2022). The increase in voltage produces activation of the Na+/Ca2+ exchanger (NCX), producing the influx of Ca2+, which allows the release of ATP by exocytosis from the astrocyte towards extracellular space (Mulkey and Wenker, 2011; Gourine and Dale, 2022). The ATP released by the astrocytes activates purinergic receptors of the RTN chemoreceptor neurons, triggering depolarization of these neurons and stimulating the central pattern generator (CPG) cells through excitatory glutamatergic synapsis producing the ventilatory drive. Therefore, when considering that peripheral and central chemoreflex activation is apparently dependent on each other (Paula-Ribeiro and Rocha, 2016) and swimmer athletes display a reduction in HVR (Arce-Alvarez et al., 2021), it is plausible to hypothesize that water sports athletes exhibit peripheral and/or central chemoreceptor alteration mechanisms, which are able to increase the apnea time by retarding or decreasing the ventilatory drive stimulated by CO2/H+ accumulation (hypercapnia) and/or arterial O2 reduction (hypoxia) induced by a breath hold. Although the evidence studying the ventilatory response to hypercapnia (HCVR) is limited, studies have shown that in elite breath-hold divers the HCVR is blunted with respect to control subjects, which could be an adaptive response to their training regimen and would allow them to maintain prolonged times of sub-aquatic apnea (Grassi et al., 1994).
Apnea involves voluntary (i.e., in aquatic immersion sports) or involuntary (i.e., central and/or obstructive sleep apnea) cessation of ventilation, which decreases PaO2 (hypoxemia) and increases PaCO2 (hypercapnia) (Sasse et al., 1996). The cessation of ventilation promotes several physiological protection mechanisms against O2 deprivation, which involves a decrease in metabolic demands and the redistribution of blood flow to vital organs, such as the brain and heart (Bouten et al., 2020). These autonomic responses are mediated by signaling circuits that are dependent on the central and peripheral chemoreceptors. Thus, peripheral and central chemoreflexes, despite depending synchronously on each other and working under independent mechanisms (St Croix et al., 1996; Barash et al., 2009; Paula-Ribeiro and Rocha, 2016), can both be activated during a maximum breath-hold. Notably, several studies have shown that chemoreflex stimulation promotes an increase in sympathetic activity, thus generating a positive chronotropic response and vasoconstriction to ensure cerebral blood flow (Kara et al., 2003; Keir et al., 2019; Stuckless et al., 2020). However, the possible hypothetical role of chemoreflex control during a voluntary breath hold at rest and during exercise (dynamic apnea) has only been determined under controlled conditions and not in a natural environment for swimmer athletes (Arce-Alvarez et al., 2021; Bruce et al., 2021; Holmström et al., 2021). Interestingly, although the chronotropic response following central and/or peripheral chemoreflex stimulation in aquatic immersion sports is well documented, the results have been controversial. In fact, Trembach and Zabolotskikh (2017) showed that transient CO2 inhalation was associated with maximum voluntary apnea in healthy individuals; however, Bain et al. (2017) showed that the central respiratory chemoreflex, through the mechanism of stimulation by hyperoxic rebreathing, was not related to maximum breath-hold duration. Of note, while Trembach and Zabolotskikh (2017) recruited healthy participants, Bain et al. (2017) recruited apnea divers. Therefore, the controversial results could be related to the study population and possibly explained by the heterogeneity with which the chemoreflex was determined. Additionally, other limitations that can explain the controversial results could be related to pharmacological approaches. Indeed, no studies have focused on chemoreflex activity via the use of pharmacological strategies to reduce or increase chemoreflex control in swimmer athletes. Additionally, the aforementioned studies have mostly focused on autonomic and cardiovascular responses, thus excluding other organs that could contribute to the maintenance of oxygen saturation despite not experiencing ventilation. One of these organs could be the spleen, which has been shown to play an important role in the responses and adaptations to hypoxic training; however, studies of this organ in voluntary apnea conditions are extremely limited (Engan et al., 2013; Pernett et al., 2021).
The spleen is an intraperitoneal organ and is a fundamental part of the reticuloendothelial system, with key implications for the immune response (Baas et al., 1994:; Kapila et al., 2021). In addition to the production and differentiation of immune cells, the spleen is considered a reservoir organ for oxygenated blood, with this organ able to store approximately 215 ml of blood in the normal population and approximately 336 ml of blood in elite divers (Prassopoulos et al., 1997). This reservoir of blood, which is delivered into the systemic circulation, is capable of contributing to maintaining oxygen saturation for a long time period, despite not experiencing pulmonary ventilation (Bakovic et al., 2003; Richardson et al., 2008). Additionally, it can maintain hemoglobin levels during prolonged voluntary apnea or short-term exposure to eupneic normobaric hypoxia (Bakovic et al., 2003; Richardson et al., 2008; Lodin-Sundström and Schagatay, 2010; Pernett et al., 2021). The blood contained in the spleen is released into the systemic circulation via splenic contraction, which is dependent on sympathetic activity (Bakovic et al., 2013). As has been previously observed, decreases in arterial O2 and/or increases in CO2 pressure produce an increase in sympathetic activity that is mediated by peripheral and central chemoreflexes, respectively, which correspondingly produce spleen contraction. Sympathetic-mediated spleen contraction occurs because the spleen is almost completely innervated by sympathetic nerve fibers, which occupy approximately 98% of the total splenic nerve (Williams et al., 1981). An increase in sympathetic activity, as well as an increase in the release of catecholamines, have been shown to decrease the spleen volume and increase the hematocrit; nevertheless, hypoxia, exercise, and any type of stress can produce contraction of the spleen (Stewart and McKenzie, 2002) (Figure 3). Similarly, Ilardo et al. (2018) showed that aborigines of the Bajau tribe, which is a tribe of extreme immersion hunters, displayed significant adaptation at the spleen level that allows them to maintain long-term immersion times. Indeed, aborigines of the Bajau tribe exhibited an increase in the size of the spleen, which would allow them to release significant amounts of oxygenated blood into the bloodstream, thus likely contributing to severe apnea times (Ilardo et al., 2018). Accordingly, it has been shown that the most successful swimmers (with a greater emptying of the spleen) exhibit a time difference of 15 s of apnea vs the least successful swimmers (Schagatay et al., 2012) (Figure 3). Therefore, the chemoreceptor-sympathetic drive-spleen axis could be a determinant for maintaining oxygen supply to active muscles during swimming exercises; however, the mechanism is not completely clear.
One of the more important limitations regarding determining the role of chemoreflexes on breath-hold in immersion sports athletes is related to the experimental setting. In fact, there are few studies that have been conducted in aquatic environments, which limits the conclusions. Conversely, most studies have been conducted in controlled laboratory environments under simulated conditions (Arce-Alvarez et al., 2021). As a consequence of the abovementioned conditions, the results related to ventilatory responses and adaptations to hypoxia and hypercapnia have been controversial. Song et al. (1963) showed that the hypercapnic and hypoxic ventilatory responses were not different between divers and control subjects (Song et al., 1963). Conversely, 18 years later, Masuda et al. (1981) conducted a study in the same subjects who participated in the study of Song et al. (1963) and assessed the ventilatory response to hypercapnia and hypoxia. They concluded that these divers (due to adaptive phenomena derived from their activity) demonstrated a blunted response to hypoxia with a normal response to hypercapnia, compared to the control group (Masuda et al., 1981). Interestingly, 1 year later, Masuda et al. (1981) demonstrated opposing results in a different group of divers. Thus, it is possible to theorize that the results could be participant-dependent and not necessarily due to a special characteristic of divers; nevertheless, this phenomenon is still under discussion.
When regarding sports water activities, the results are also controversial. Indeed, Davis et al. (1987) demonstrated a blunted ventilatory response to hypercapnia in underwater hockey players compared to land athletes. Nevertheless, Bjurstrom and Schoene (1987) showed that the hypoxic ventilatory response was blunted, without changes in hypercapnic ventilatory drive in the national synchronized swim team, compared to a control group. Similarly, breath-hold elite divers displayed no significant differences in autonomic, ventilatory, and cardiovascular responses to hypoxia (Breskovic et al., 2010a) and to hypercapnia (Dujic et al., 2008), compared to a control condition. Additionally, after 1 month of endurance intensity training, apnea divers exhibited normal peripheral chemoreflex regulation, compared to an untrained control group (Breskovic et al., 2010b). Accordingly, it is possible to propose that exercise training may not impact the breath-hold and/or chemoreflex response; however, the effect of apnea training on chemoreflex control has not been extensively studied. Therefore, further research needs to investigate this important issue.
The role of chemoreflex control in breath holding is limited and controversial. Additionally, most evidence is associated with long-term apnea sports (breath-hold divers); nevertheless, the evidence regarding intermittent apnea sports is even more limited (Ohkuwa et al., 1980; Arce-Alvarez et al., 2021). Indeed, Ohkuwa et al. (1980) showed that the ventilatory response to hypercapnia was markedly reduced in swimmers, compared to an untrained group (Ohkuwa et al., 1980). Additionally, we found similar results, which demonstrated that young swimmer athletes displayed a robust reduction in the hypoxic chemoreflex response, compared to a control group (Arce-Alvarez et al., 2021).
Although there is robust evidence depicting ventilatory chemoreflex and breath-hold duration in water sports, mostly these manuscripts recreate aquatic conditions in an environmental laboratory, which could explain, in part, the controversial findings. In addition, most manuscripts fail to determine whether chemoreflex contributed to apnea duration or if apnea training-dependent chemoreflex control. Of note, performance studies have shown that apnea training could positively impact the sports performance of swimmer athletes. Along with this, sub-aquatic apnea training has been shown to be an effective strategy for improving swimming technique at both maximal and sub-maximal intensity (Lemaître et al., 2009). Indeed, two weeks of dynamic apneic training enhanced apnea-induced diving bradycardia increasing the number of heart rate reserve beats (Mulder et al., 2021). Nevertheless, we showed that during a maximum static apnea effort, under laboratory conditions, the HR decreased less compared to a control condition, which apparently was a sympathetic-mediated effect (Arce-Alvarez et al., 2021). In addition, the combination of pre-competitive warm-up plus apnea exercise was shown to improve 400-m performance during a swimming race (Robertson et al., 2020). Therefore, apparently, apnea training could be a feasible maneuver that is able to promote an improvement of competitive performance. However, until now there is no evidence showing both, the real role of chemoreflex control on apnea duration and their possible effects on sports performance. In fact, it is not possible so far to rule out whether the chemoreflex is just a consequence of freediving training, with no role in performance. Therefore, further manuscripts should address this important question.
Immersion water sports athletes are characterized by long-term breath holding; however, the possible role of peripheral O2 and/or central CO2 chemoreflex control is not completely clear. In fact, although chemosensitivity (peripheral and/or central) at rest could be reduced, marked sympathoexcitation during a maximum voluntary breath-hold in young swimmers has been observed. Importantly, the sympathetic drive could activate the spleen, which is a reservoir organ for oxygenated blood, thus contributing to the maintenance of apnea (Figure 2). However, although the chemoreflex could apparently be related to breath holding in swimmer and diver athletes, the evidence is limited. In fact, the mechanism explaining long-term apnea is currently unknown. Therefore, future studies should elucidate whether the explanation to breath hold is related to the sensor (peripheral and/or central chemoreceptors), central command (respiratory and autonomic nuclei at brainstem level), or effectors through the respiratory drive (i.e., respiratory muscles).
FIGURE 2. Hypothetical schematic representation model related to the influence of the central and peripheral chemoreflex on a breath hold. Peripheral and central chemoreflex responses of land sports athletes and immersion water sports athletes (left and right, respectively). Carotid body peripheral chemoreceptors send afferences to the nucleus of tractus solitary (NTS) in response to decreases of arterial O2 (hypoxia). Accordingly, the NTS project to the central pattern generator (CPG), which is able to produce hyperventilation. In addition, activation of peripheral and central chemoreceptors increases sympathetic activity, producing contraction of the spleen which releases oxygenated blood into the bloodstream. Note that adaptations in immersion athletes involve desensitization of peripheral chemoreceptors which possibly decreases input to NTS and thus to CPG, as well as an increase in size and storage of oxygenated blood in the spleen which allows greater release of blood into the systemic circulation increasing the time of apnea. Nevertheless, apparently central chemoreceptors do not have greater relevance in the breath-hold.
AA-A and CS-A contributed to the draft and preparation of the manuscript. CS-A, CC, VP, MV-M, CJ, RR-C and MI contributed to the preparation of the manuscript. DCA contributed to the concept of the project. DCA contributed to the preparation of the manuscript. All authors approved the final version of the manuscript.
This study was supported by Minera Escondida Ltda. MEL2203; the “Agencia Nacional de Investigación y Desarrollo (ANID)”, through Fondecyt de Iniciación #11220870 and Anillo ACT210083.
The authors declare that the research was conducted in the absence of any commercial or financial relationships that could be construed as a potential conflict of interest.
All claims expressed in this article are solely those of the authors and do not necessarily represent those of their affiliated organizations, or those of the publisher, the editors and the reviewers. Any product that may be evaluated in this article, or claim that may be made by its manufacturer, is not guaranteed or endorsed by the publisher.
Authors thanks to the Minera Escondida Ltda. MI was funded in part by grant from the Spanish Ministry of Economy, “Ministerio de Ciencia e Innovación” (PID 2020-113098RB-I00).
Andersson J. P. A., Linér M. H., Rünow E., Schagatay E. K. A. (2002). Diving Response and Arterial Oxygen Saturation during Apnea and Exercise in Breath-Hold Divers. J. Appl. Physiology 93, 882–886. doi:10.1152/japplphysiol.00863.2001
Arce-Álvarez A., Veliz C., Vazquez-Muñoz M., von Igel M., Alvares C., Ramirez-Campillo R., et al. (2021). Hypoxic Respiratory Chemoreflex Control in Young Trained Swimmers. Front. Physiol. 12, 632603. doi:10.3389/fphys.2021.632603
Baas J., Senninger N., Elser H. (1994). Das Retikuloendotheliale System. The Reticuloendothelial System. An Overview of Function, Pathology and Recent Methods of Measurement. Z. Gastroenterol. 32, 117–123.
Bain A. R., Barak O. F., Hoiland R. L., Drvis I., Bailey D. M., Dujic Z., et al. (2017). Forced Vital Capacity and Not Central Chemoreflex Predicts Maximal Hyperoxic Breath-Hold Duration in Elite Apneists. Respir. Physiology Neurobiol. 242, 8–11. doi:10.1016/j.resp.2017.02.015
Bakovic D., Pivac N., Zubin Maslov P., Breskovic T., Damonja G., Dujic Z. (2013). Spleen Volume Changes during Adrenergic Stimulation with Low Doses of Epinephrine. J. Physiol. Pharmacol. 64, 649–655.
Baković D., Valic Z., Eterović D., Vukovic I., Obad A., Marinović-Terzić I., et al. (2003). Spleen Volume and Blood Flow Response to Repeated Breath-Hold Apneas. J. Appl. Physiol. (1985) 95, 1460–1466. doi:10.1152/japplphysiol.00221.2003
Barash P. G., Cullen B. F., Stoelting R. K., Cahalan M., Stock M. C. (2009). Clinical Anesthesia. 6th ed. Philadelphia, PA: Lippincott, Williams and Wilkins, 240.
Bjurström R. L., Schoene R. B. (1987). Control of Ventilation in Elite Synchronized Swimmers. J. Appl. Physiol. 63, 1019–1024.
Bouten J., Bourgois J. G., Boone J. (2020). Hold Your Breath: Peripheral and Cerebral Oxygenation during Dry Static Apnea. Eur. J. Appl. Physiol. 120, 2213–2222. doi:10.1007/s00421-020-04445-y
Breskovic T., Ivancev V., Banic I., Jordan J., Dujic Z. (2010a). Peripheral Chemoreflex Sensitivity and Sympathetic Nerve Activity Are Normal in Apnea Divers during Training Season. Aut. Neurosci. 154, 42–47. doi:10.1016/j.autneu.2009.11.001
Breskovic T., Valic Z., Lipp A., Heusser K., Ivancev V., Tank J., et al. (2010b). Peripheral Chemoreflex Regulation of Sympathetic Vasomotor Tone in Apnea Divers. Clin. Auton. Res. 20, 57–63. doi:10.1007/s10286-009-0034-1
Bruce C. D., Vanden Berg E. R., Pfoh J. R., Steinback C. D., Day T. A. (2021). Prior Oxygenation, but Not Chemoreflex Responsiveness, Determines Breath-Hold Duration during Voluntary Apnea. Physiol. Rep. 9, e14664. doi:10.14814/phy2.14664
Chang A. J., Ortega F. E., Riegler J., Madison D. V., Krasnow M. A. (2015). Oxygen Regulation of Breathing through an Olfactory Receptor Activated by Lactate. Nature 527, 240–244. doi:10.1038/nature15721
Davis F. M., Graves M. P., Guy H. J., Prisk G. K., Tanner T. E. (1987). Carbon Dioxide Response and Breath-Hold Times in Underwater Hockey Players. Undersea Biomed. Res. 14, 527–534.
Dempsey J. A., Smith C. A., Blain G. M., Xie A., Gong Y., Teodorescu M. (2012). Role of Central/peripheral Chemoreceptors and Their Interdependence in the Pathophysiology of Sleep Apnea. Adv. Exp. Med. Biol. 758, 343–349. doi:10.1007/978-94-007-4584-1_46
Dujic Z., Ivancev V., Heusser K., Dzamonja G., Palada I., Valic Z., et al. (2008). Central Chemoreflex Sensitivity and Sympathetic Neural Outflow in Elite Breath-Hold Divers. J. Appl. Physiol. 104, 205–211. doi:10.1152/japplphysiol.00844.2007
Elia A., Gennser M., Harlow P. S., Lees M. J. (2021). Physiology, Pathophysiology and (Mal)adaptations to Chronic Apnoeic Training: A State-Of-The-Art Review. Eur. J. Appl. Physiol. 121, 1543–1566. doi:10.1007/s00421-021-04664-x
Engan H., Richardson M. X., Lodin-Sundström A., van Beekvelt M., Schagatay E. (2013). Effects of Two Weeks of Daily Apnea Training on Diving Response, Spleen Contraction, and Erythropoiesis in Novel Subjects. Scand. J. Med. Sci. Sports. 23, 340–348. doi:10.1111/j.1600-0838.2011.01391.x
Evans A. M., Mahmoud A. D., Moral-Sanz J., Hartmann S. (2016). The Emerging Role of AMPK in the Regulation of Breathing and Oxygen Supply. Biochem. J. 473, 2561–2572. doi:10.1042/bcj20160002
Fernández-Agüera M. C., Gao L., González-Rodríguez P., Pintado C. O., Arias-Mayenco I., García-Flores P., et al. (2015). Oxygen Sensing by Arterial Chemoreceptors Depends on Mitochondrial Complex I Signaling. Cell Metab. 22, 825–837. doi:10.1016/j.cmet.2015.09.004
Ferretti G. (2001). Extreme Human Breath-Hold Diving. Eur. J. Appl. Physiol. 84, 254–271. doi:10.1007/s004210000377
Ferretti G., Costa M., Ferrigno M., Grassi B., Marconi C., Lundgren C. E., et al. (1991). Alveolar Gas Composition and Exchange during Deep Breath-Hold Diving and Dry Breath Holds in Elite Divers. J. Appl. Physiology 70, 794–802. doi:10.1152/jappl.1991.70.2.794
Forbes J., Menezes R. G. (2021). “Anatomy, Head and Neck, Carotid Bodies,” in StatPearls (Treasure Island (FL): StatPearls Publishing). 2021 Jan-. Available from: https://www.ncbi.nlm.nih.gov/books/NBK562237/.
Gonçalves C. M., Mulkey D. K. (2018). Bicarbonate Directly Modulates Activity of Chemosensitive Neurons in the Retrotrapezoid Nucleus. J. Physiol. 596, 4033–4042. doi:10.1113/JP276104
Gourine A. V., Dale N. (2022). Brain H +/CO 2 Sensing and Control by Glial Cells. Glia. doi:10.1002/glia.24152
Gourine A. V., Funk G. D. (2017). On the Existence of a Central Respiratory Oxygen Sensor. J. Appl. Physiology 123, 1344–1349. doi:10.1152/japplphysiol.00194.2017
Grassi B., Ferretti G., Costa M., Ferrigno M., Panzacchi A., Lundgren C. E. G., et al. (1994). Ventilatory Responses to Hypercapnia and Hypoxia in Elite Breath-Hold Divers. Respir. Physiol. 97, 323–332. doi:10.1016/0034-5687(94)90068-x
Guimard A., Prieur F., Zorgati H., Morin D., Lasne F., Collomp K. (2014). Acute Apnea Swimming: Metabolic Responses and Performance. J. Strength Cond. Res. 28, 958–963. doi:10.1519/jsc.0000000000000254
Guyenet P. (2012). How Does CO2activate the Neurons of the Retrotrapezoid Nucleus? J. Physiol. 590, 2183–2184. doi:10.1113/jphysiol.2012.230466
Heusser K., Dzamonja G., Tank J., Palada I., Valic Z., Bakovic D., et al. (2009). Cardiovascular Regulation during Apnea in Elite Divers. Hypertension 53, 719–724. doi:10.1161/hypertensionaha.108.127530
Holmström P. K., Bird J. D., Thrall S. F., Kalker A., Herrington B. A., Soriano J. E., et al. (2021). The Effects of High Altitude Ascent on Splenic Contraction and the Diving Response during Voluntary Apnoea. Exp. Physiol. 106, 160–174. doi:10.1113/EP088571
Ilardo M. A., Moltke I., Korneliussen T. S., Cheng J., Stern A. J., Racimo F., et al. (2018). Physiological and Genetic Adaptations to Diving in Sea Nomads. Cell 173, 569–580. doi:10.1016/j.cell.2018.03.054
Iturriaga R., Alcayaga J. (2004). Neurotransmission in the Carotid Body: Transmitters and Modulators Between Glomus Cells and Petrosal Ganglion Nerve Terminals. Brain Res. Rev. 47, 46–53. doi:10.1016/j.brainresrev.2004.05.007
Iturriaga R., Alcayaga J., Chapleau M. W., Somers V. K. (2021). Carotid Body Chemoreceptors: Physiology, Pathology, and Implications for Health and Disease. Physiol. Rev. 101, 1177–1235. doi:10.1152/physrev.00039.2019
Iturriaga R., Del Rio R., Idiaquez J., Somers V. K. (2016). Carotid Body Chemoreceptors, Sympathetic Neural Activation, and Cardiometabolic Disease. Biol. Res. 49, 13. doi:10.1186/s40659-016-0073-8
Joyner M. J., Limberg J. K., Wehrwein E. A., Johnson B. D. (2018). Role of the Carotid Body Chemoreceptors in Glucose Homeostasis and Thermoregulation in Humans. J. Physiol. 596, 3079–3085. doi:10.1113/jp274354
Kapila V., Wehrle C. J., Tuma F. (2021). “Physiology, Spleen,” in StatPearls (Treasure Island (FL): StatPearls Publishing). Available from: https://www.ncbi.nlm.nih.gov/books/NBK537307/.
Kara T., Narkiewicz K., Somers V. K. (2003). Chemoreflexes - Physiology and Clinical Implications. Acta Physiol. Scand. 177, 377–384. doi:10.1046/j.1365-201x.2003.01083.x
Keir D. A., Duffin J., Millar P. J., Floras J. S. (2019). Simultaneous Assessment of Central and Peripheral Chemoreflex Regulation of Muscle Sympathetic Nerve Activity and Ventilation in Healthy Young Men. J. Physiol. 597, 3281–3296. doi:10.1113/jp277691
Kjeld T., Stride N., Gudiksen A., Hansen E. G., Arendrup H. C., Horstmann P. F., et al. (2018). Oxygen Conserving Mitochondrial Adaptations in the Skeletal Muscles of Breath Hold Divers. PLoS One 13, e0201401. doi:10.1371/journal.pone.0201401
Konstantinidou S., Chairopoulou C. (2017). Apnea Training Specificity and its Implications for Performance in Aquatic Sports: Case Study Reports. Int. J. Sports Exerc. Med. 3, 073. doi:10.23937/2469-5718/1510073
Kumar N. N., Velic A., Soliz J., Shi Y., Li K., Wang S., et al. (2015). Regulation of Breathing by CO 2 Requires the Proton-Activated Receptor GPR4 in Retrotrapezoid Nucleus Neurons. Science 348, 1255–1260. doi:10.1126/science.aaa0922
Kumar P., Prabhakar N. R. (2012). Peripheral Chemoreceptors: Function and Plasticity of the Carotid Body. Compr. Physiol. 2, 141–219. doi:10.1002/cphy.c100069
Lemaître F., Seifert L., Polin D., Juge J., Tourny-Chollet C., Chollet D. (2009). Apnea Training Effects on Swimming Coordination. J. Strength Cond. Res. 23, 1909–1914. doi:10.1519/JSC.0b013e3181b073a8
Lodin-Sundström A., Schagatay E. (2010). Spleen Contraction during 20 Min Normobaric Hypoxia and 2 Min Apnea in Humans. Aviat. Space Environ. Med. 81, 545–549. doi:10.3357/asem.2682.2010
López-Barneo J., González-Rodríguez P., Gao L., Fernández-Agüera M. C., Pardal R., Ortega-Sáenz P. (2016). Oxygen Sensing by the Carotid Body: Mechanisms and Role in Adaptation to Hypoxia. Am. J. Physiol. Cell Physiol. 310, C629–C642. doi:10.1152/ajpcell.00265.2015
López-Barneo J., Ortega-Sáenz P., Pardal R., Pascual A., Piruat J. I. (2008). Carotid Body Oxygen Sensing. Eur. Respir. J. 32, 1386–1398. doi:10.1183/09031936.00056408
Masuda Y., Yoshida A., Hayashi F., Sasaki K., Honda Y. (1981). The Ventilatory Responses to Hypoxia and Hypercapnia in the Ama. Jpn. J. Physiol. 31, 187–197. doi:10.2170/jjphysiol.31.187
Moreira T. S., Takakura A. C., Colombari E., Guyenet P. G. (2006). Central Chemoreceptors and Sympathetic Vasomotor Outflow. J. Physiol. 577, 369–386. doi:10.1113/jphysiol.2006.115600
Mulder E. R., Holmström P. K., Schagatay E. K. (2021). Effects of Dynamic Apnea Training on Diving Bradycardia and Short Distance Swimming Performance. J. Sports Med. Phys. Fit. 21. doi:10.23736/S0022-4707.21.12549-6
Mulkey D. K., Wenker I. C. (2011). Astrocyte Chemoreceptors: Mechanisms of H+sensing by Astrocytes in the Retrotrapezoid Nucleus and Their Possible Contribution to Respiratory Drive. Exp. Physiol. 96, 400–406. doi:10.1113/expphysiol.2010.053140
Nattie E., Li A. (2012). Central Chemoreceptors: Locations and Functions. Compr. Physiol. 2, 221–254. doi:10.1002/cphy.c100083
Ohkuwa T., Fujitsuka N., Utsuno T., Miyamura M. (1980). Ventilatory Response to Hypercapnia in Sprint and Long-Distance Swimmers. Eur. J. Appl. Physiol. 43, 235–241. doi:10.1007/bf00421837
Ortega-Sáenz P., López-Barneo J. (2020). Physiology of the Carotid Body: From Molecules to Disease. Annu. Rev. Physiol. 82, 127–149. doi:10.1146/annurev-physiol-020518-114427
Pardal R., Ortega-Sáenz P., Durán R., López-Barneo J. (2007). Glia-like Stem Cells Sustain Physiologic Neurogenesis in the Adult Mammalian Carotid Body. Cell 131, 364–377. doi:10.1016/j.cell.2007.07.043
Paula-Ribeiro M., Rocha A. (2016). The Peripheral-Central Chemoreflex Interaction: Where Do We Stand and what Is the Next Step? J. Physiol. 594, 1527–1528. doi:10.1113/jp271901
Pernett F., Schagatay F., Vildevi C., Schagatay E. (2021). Spleen Contraction during Sudden Eupneic Hypoxia Elevates Hemoglobin Concentration. Front. Physiol. 12, 729123. doi:10.3389/fphys.2021.729123
Prassopoulos P., Daskalogiannaki M., Raissaki M., Hatjidakis A., Gourtsoyiannis N. (1997). Determination of Normal Splenic Volume on Computed Tomography in Relation to Age, Gender and Body Habitus. Eur. Radiol. 7, 246–248. doi:10.1007/s003300050145
Rakoczy R. J., Wyatt C. N. (2018). Acute Oxygen Sensing by the Carotid Body: A Rattlebag of Molecular Mechanisms. J. Physiol. 596, 2969–2976. doi:10.1113/jp274351
Richardson M. X., Lodin A., Reimers J., Schagatay E. (2008). Short-term Effects of Normobaric Hypoxia on the Human Spleen. Eur. J. Appl. Physiol. 104, 395–399. doi:10.1007/s00421-007-0623-4
Robertson C., Lodin-Sundström A., O'Hara J., King R., Wainwright B., Barlow M. (2020). Effects of Pre-race Apneas on 400-m Freestyle Swimming Performance. J. Strength. Cond. Res. 34, 828–837. doi:10.1519/jsc.0000000000002711
Santin J. M. (2018). How Important Is the CO2 Chemoreflex for the Control of Breathing? Environmental and Evolutionary Considerations. Comp. Biochem. Physiology Part A Mol. Integr. Physiology 215, 6–19. doi:10.1016/j.cbpa.2017.09.015
Sasse S. A., Berry R. B., Nguyen T. K., Light R. W., Kees Mahutte C. (1996). Arterial Blood Gas Changes during Breath-Holding from Functional Residual Capacity. Chest 110, 958–964. doi:10.1378/chest.110.4.958
Schagatay E., Richardson M. X., Lodin-Sundström A. (2012). Size Matters: Spleen and Lung Volumes Predict Performance in Human Apneic Divers. Front. Physio. 3, 173. doi:10.3389/fphys.2012.00173
Schagatay E., van Kampen M., Emanuelsson S., Holm B. (2000). Effects of Physical and Apnea Training on Apneic Time and the Diving Response in Humans. Eur. J. Appl. Physiology 82, 161–169. doi:10.1007/s004210050668
Smith C. A., Blain G. M., Henderson K. S., Dempsey J. A. (2015). Peripheral Chemoreceptors Determine the Respiratory Sensitivity of Central Chemoreceptors to CO2: Role of Carotid Body CO2. J. Physiol. 593, 4225–4243. doi:10.1113/jp270114
Song S. H., Kang D. H., Kang B. S., Hong S. K. (1963). Lung Volumes and Ventilatory Responses to High CO2 and Low O2 in the Ama. J. Appl. Physiology 18, 466–470. doi:10.1152/jappl.1963.18.3.466
St Croix C. M., Cunningham D. A., Paterson D. H. (1996). Nature of the Interaction between Central and Peripheral Chemoreceptor Drives in Human Subjects. Can. J. Physiol. Pharmacol. 74, 640–646. doi:10.1139/y96-049
Stewart I. B., McKenzie D. C. (2002). The Human Spleen during Physiological Stress. Sports Med. 32, 361–369. doi:10.2165/00007256-200232060-00002
Stuckless T. J. R., Vermeulen T. D., Brown C. V., Boulet L. M., Shafer B. M., Wakeham D. J., et al. (2020). Acute Intermittent Hypercapnic Hypoxia and Sympathetic Neurovascular Transduction in Men. J. Physiol. 598, 473–487. doi:10.1113/jp278941
Torres-Torrelo H., Ortega-Sáenz P., Gao L., López-Barneo J. (2021). Lactate Sensing Mechanisms in Arterial Chemoreceptor Cells. Nat. Commun. 12, 4166. doi:10.1038/s41467-021-24444-7
Trembach N., Zabolotskikh I. (2017). Breath-holding Test in Evaluation of Peripheral Chemoreflex Sensitivity in Healthy Subjects. Respir. Physiology Neurobiol. 235, 79–82. doi:10.1016/j.resp.2016.10.005
Viana E., Bentley D. J., Logan-Sprenger H. M. (2019). A Physiological Overview of the Demands, Characteristics, and Adaptations of Highly Trained Artistic Swimmers: A Literature Review. Sports Med. - Open 5, 16. doi:10.1186/s40798-019-0190-3
Wasfy M. M., Weiner R. B., Wang F., Berkstresser B., Deluca J., Hutter A. M., et al. (2019). Myocardial Adaptations to Competitive Swim Training. Med. Sci. Sports Exerc. 51, 1987–1994. doi:10.1249/mss.0000000000002022
Williams J. M., Peterson R. G., Shea P. A., Schmedtje J. F., Bauer D. C., Felten D. L. (1981). Sympathetic Innervation of Murine Thymus and Spleen: Evidence for a Functional Link between the Nervous and Immune Systems. Brain Res. Bull. 6, 83–94. doi:10.1016/s0361-9230(81)80072-x
Wyatt C. N., Buckler K. J. (2004). The Effect of Mitochondrial Inhibitors on Membrane Currents in Isolated Neonatal Rat Carotid Body Type I Cells. J. Physiol. 556, 175–191. doi:10.1113/jphysiol.2003.058131
Wyatt C. N., Mustard K. J., Pearson S. A., Dallas M. L., Atkinson L., Kumar P., et al. (2007). AMP-activated Protein Kinase Mediates Carotid Body Excitation by Hypoxia. J. Biol. Chem. 282, 8092–8098. doi:10.1074/jbc.m608742200
Xu J., Tse F. W., Tse A. (2003). ATP Triggers Intracellular Ca2+Release in Type II Cells of the Rat Carotid Body. J. Physiology 549, 739–747. doi:10.1113/jphysiol.2003.039735
Keywords: water sports, peripheral chemoreflex, central chemoreflex, autonomic nervous system, apnea
Citation: Arce-Álvarez A, Salazar-Ardiles C, Cornejo C, Paez V, Vásquez-Muñoz M, Stillner-Vilches K, Jara CR, Ramirez-Campillo R, Izquierdo M and Andrade DC (2022) Chemoreflex Control as the Cornerstone in Immersion Water Sports: Possible Role on Breath-Hold. Front. Physiol. 13:894921. doi: 10.3389/fphys.2022.894921
Received: 12 March 2022; Accepted: 10 10 May 20222022;
Published: 06 June 2022.
Edited by:
Monoem Haddad, Qatar University, QatarReviewed by:
Zied Abbes, Qatar University, QatarCopyright © 2022 Arce-Álvarez, Salazar-Ardiles, Cornejo, Paez, Vásquez-Muñoz, Stillner-Vilches, Jara, Ramirez-Campillo, Izquierdo and Andrade. This is an open-access article distributed under the terms of the Creative Commons Attribution License (CC BY). The use, distribution or reproduction in other forums is permitted, provided the original author(s) and the copyright owner(s) are credited and that the original publication in this journal is cited, in accordance with accepted academic practice. No use, distribution or reproduction is permitted which does not comply with these terms.
*Correspondence: David C. Andrade, ZGF2aWQuYW5kcmFkZUB1YW50b2YuY2w=, ZGNhbmRyYWRlQHVjLmNs
†These authors contributed equally to this work
Disclaimer: All claims expressed in this article are solely those of the authors and do not necessarily represent those of their affiliated organizations, or those of the publisher, the editors and the reviewers. Any product that may be evaluated in this article or claim that may be made by its manufacturer is not guaranteed or endorsed by the publisher.
Research integrity at Frontiers
Learn more about the work of our research integrity team to safeguard the quality of each article we publish.