- 1Division of Pulmonary and Critical Care Medicine, Department of Medicine, Johns Hopkins University School of Medicine, Baltimore, MD, United States
- 2Department of Environmental Health and Engineering, Johns Hopkins Bloomberg School of Public Health, Baltimore, MD, United States
- 3Section of Pulmonary, Critical Care, and Sleep Medicine, Department of Medicine, Baylor College of Medicine and the Center for Innovations in Quality, Effectiveness, and Safety, Michael E. DeBakey VA Medical Center, Houston, TX, United States
Obese asthma is a unique phenotype of asthma characterized by non-allergic airway hyperresponsiveness (AHR) and inflammation which responds poorly to standard asthma therapy. Metformin is an oral hypoglycemic drug with insulin-sensitizing and anti-inflammatory properties. The objective of the current study was to test the effect of metformin on AHR in a mouse model of diet-induced obesity (DIO). We fed 12-week-old C57BL/6J DIO mice with a high fat diet for 8 weeks and treated them with either placebo (control, n = 10) or metformin (n = 10) added in drinking water (300 mg/kg/day) during the last 2 weeks of the experiment. We assessed AHR, metabolic profiles, and inflammatory markers after treatments. Metformin did not affect body weight or fasting blood glucose, but significantly reduced serum insulin (p = 0.0117). Metformin reduced AHR at 30 mg/ml of methacholine challenge (p = 0.0052) without affecting baseline airway resistance. Metformin did not affect circulating white blood cell counts or lung cytokine mRNA expression, but modestly decreased circulating platelet count. We conclude that metformin alleviated AHR in DIO mice. This finding suggests metformin has the potential to become an adjuvant pharmacological therapy in obese asthma.
Introduction
Obesity affects over 650 million adults across the world (World Health Organization, 2021) and is a major risk factor for developing comorbidities such as asthma. Beyond increasing the risk of developing asthma, obesity is associated with more frequent and severe exacerbations as well as resistance to standard asthma therapy (Peters et al., 2018). The pathogenesis of obese asthma is poorly understood, and effective treatment is lacking (Peters et al., 2018). Several physiological and immunological mechanisms have been suggested, including obesity-related mechanical effects on airway, lung, and chest wall (Mead et al., 1970; Kapsali et al., 2000; Jones and Nzekwu, 2006; Raviv et al., 2011; Bates and Dixon, 2015), non-allergic airway hyperresponsiveness (AHR) and inflammation (Kim H. Y et al., 2014; Fricke et al., 2018; Younas et al., 2019), hyperleptinemia, hyperglycemia, and insulin resistance (McKeever et al., 2005; Shore et al., 2005; Wu et al., 2019a). Each of these factors may contribute to the development or worsening of asthma in obese individuals as well as their blunted response to usual therapy.
Our group previously showed that, in mice, diet-induced obesity (DIO) caused airway inflammation with elevation of interleukin (IL)-1β levels and AHR, which resembled non-eosinophilic asthma in humans (Younas et al., 2019; Fricke et al., 2018). Compared to lean mice, DIO animals had markedly increased total resistance of the respiratory system after methacholine challenge, but not at baseline (Younas et al., 2019; Fricke et al., 2018). This form of obesity-induced AHR was abolished by caloric restriction or by daily injections of the IL-1β receptor blocker anakinra (Younas et al., 2019). Interestingly, anakinra also improved insulin resistance, evidenced by the correction of hyperinsulinemia without a significant change in glucose (Younas et al., 2019). These findings suggest a potential role of insulin resistance in obesity-related AHR.
Metformin is a first-line drug widely prescribed for the management of type 2 diabetes. Metformin suppresses hepatic glucose output and increases insulin sensitivity leading to reductions in circulating glucose (An and He, 2016; Rena et al., 2017). Beyond its glucose-lowering effect, metformin has shown anti-inflammatory properties in different disease models (Saisho, 2015; Vaez et al., 2016; Hyun et al., 2013). Several retrospective cohort studies in patients with diabetes revealed a lower risk of incident asthma and better asthma-related outcomes in metformin users than non-users (Li et al., 2016; Chen et al., 2017; Wu et al., 2019b), suggesting a potential therapeutic role of metformin in asthma. However, evidence of clinical benefit is limited by the lack of randomized controlled trials. Metformin was shown to reduce airway inflammation and remodeling in allergic murine asthma models (Park et al., 2012; Calixto et al., 2013; Guo et al., 2021). By contrast, metformin did not affect AHR in studies of db/db mice or obese Swiss mice induced by overfeeding (Shore et al., 2008; Dias et al., 2018). Effects of metformin on airway hyperresponsiveness or inflammation in DIO mice in the absence of allergic sensitization have not been examined. We hypothesized that metformin treatment can alleviate non-allergic AHR in diet-induced obesity. In the current study, we tested our hypothesis in C57BL/6J mice on a high-fat diet.
Materials and Methods
Animals and Study Design
The study was approved by the Institutional Animal Care and Use Committee of the Johns Hopkins University and complied with the American Physiological Society Guidelines for Animal Studies. In total, 20 C57BL/6J male DIO mice (Jackson Labs Bar Harbor, MA, 12 weeks old) were used in the study. Mice were housed in 4 or 5 per cage, in a temperature and humidity-controlled room with a 12/12 light/dark cycle (9 am–9 pm lights on/9 pm–9 am lights off) with free access to water. The experiment consisted of 2 groups, metformin group (n = 10) and control group (n = 10). Both groups were fed with high fat diet (TD 03584, Teklad WI, 5.4 kcal/g, 35.2% fat, and 58.4% kcal from fat) ad libitum for 8 weeks. During the last 14 days of the experiment, metformin group was treated with metformin added in drinking water with a target dose of 300 mg/kg/day. The dose of metformin and the time course were chosen based on the previous studies (Shore et al., 2008; Dias et al., 2018). Metformin hydrochloride (PeproTech, NJ) was dissolved 3.375 g/L in drinking water, calculated based on the average body weight of mice and an estimated water consumption of 4 ml/mouse/day as Dias et al. (Dias et al., 2018). Control group was drinking water without metformin. The two groups were weight-matched before the start of metformin or placebo treatment. The body weights, food and water intake of the animals were monitored weekly. Daily food consumption was calculated by weighing food and measuring water twice a week in each cage and then divided by the number of animals and days. The food was measured both in the feeder and in the cage (given the difference in color and consistency of high fat food with bedding and feces, this was easily achieved). Cages were checked frequently, water bottles changed weekly, and no metformin-water spillage occurred during the experiment. Blood glucose levels were measured at 3:30 p.m. after a 6-h fasting (9:30 a.m.—3:30 p.m.) by tail-snip technique using a hand-held glucometer (FreeStyle Freedom Lite, Abbott, CA) once every 2 weeks.
Physiological Measurements and Harvest
After 8 weeks’ experiment, mice were anesthetized with ketamine/xylazine i. p. and depth of anesthesia was confirmed by negative toe-pinch response before tracheostomy with an 18G stub cannula. Total resistance of the respiratory system (Rrs) was measured by forced oscillation technique (Flexivent, SCIREQ Québec, Canada) at baseline and after methacholine aerosol challenge at 3 and 30 mg/ml as described (Bishai and Mitzner, 2008; Fricke et al., 2018). Briefly, each animal was ventilated (Flexivent; Scireq, Montreal, PQ, Canada) in the supine position after injection with 5 mg/kg succinylcholine I.M. with a tidal volume of 0.25 ml of 100% oxygen at a rate of 150 breaths/min, with a positive end-expiratory pressure of 3 cmH2O. A deep inspiration (to 27 cmH2O for 10 s) was given, and then the animal was returned to normal ventilation. One minute later, a sinusoidal oscillation at 2.5 Hz was applied to determine baseline dynamic resistance (Rrs) and elastance (Ers). Following the perturbation, the mouse was returned to normal ventilation for 1 min. This cycle was repeated after every methacholine dose, and average values for each dose were reported. Consistent nebulization was achieved using a 50% duty cycle for 10 seconds under normal ventilation parameters (Ultrafine Aeroneb; Aerogen, Galway, Ireland). The nebulizer was flushed between each mouse with 30 ml of water and dried to clear any residual methacholine. Blood was collected from the aorta. The thorax was opened, and the right lung was tied off, dissected free and immediately frozen in liquid nitrogen and stored at −80°C. The remaining left lung was inflated with formalin at 26 cm H2O pressure for 10 min, tied off and placed inflated in formalin for 2 days. Left lung volumes were measured by fluid displacement method (AU—Limjunyawong et al., 2015).
Blood and Lung Tissue Analysis
Complete blood count (CBC) was measured by the ProCyte Dx Hematology Analyzer (IDEXX Laboratories, ME). Serum insulin, leptin and adiponectin were measured with enzyme-linked immunosorbent assay (ELISA) kits from Alpco Diagnostics (Salem, NH), Abcam (Cambridge, MA) and Millipore (Billerica, MA), respectively. Homeostasis model assessment-insulin resistance (HOMA-IR) index was calculated as fasting insulin (μU/mL) × fasting glucose (mmol/L)/22.5 for the assessment of insulin sensitivity (Matthews et al., 1985). Total RNA was extracted from lung tissue with a Trizol reagent (Life Technologies, Rockville, MD). cDNA was produced from total RNA using Advantage RT for Polymerase chain reaction (PCR) kit from Clontech (Palo Alto, CA). Real time PCR was performed for the cytokine panel, including interleukins (IL) 1β, 5, 6, 10, 13, 17, 18, and TNF-α with premade primers from Invitrogen (Carlsbad, CA) and Taqman probes from Applied Biosystems (Foster City, CA) using 18 S as a housekeeping gene. Custom made 18 S primers were forward 5′-CTCTTTCGAGGCCCTGTAATTGT-3′, reverse, 5′-AACTGCAGCAACTTTAATATACGCTATT-3′ and the probe 6FAM-AGTCCACTTTAAATCCTT. Target mRNA level was normalized to 18 s rRNA, using 2−ΔΔCt relative quantitative method. The results were expressed as relative fold changes to controls.
Statistical Analyses
Statistical analyses were performed using STATA version 15.1 (StataCorp LLC, United States). All values were reported as means ± standard error of the mean (SEM). Data was checked for normality with Shapiro-Wilk W test. Normally distributed variables were compared between two groups by two-sided unpaired Student’s t-test. Non-normally distributed values were analyzed between two groups by Mann-Whitney U test. p values <0.05 were considered statistically significant.
Results
Basic Characteristics of the Animals
Mice in metformin and control groups gained similar amounts of weight over the 8-weeks study period. Body weights were identical in the two groups before and after treatments (Figure 1). Food and water intake were also similar between the two groups. The actual metformin intake was 247.8 mg/kg/mouse/day in metformin group (Table 1).
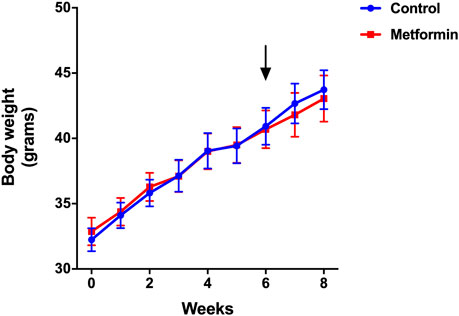
FIGURE 1. Weight trajectory of metformin and control groups over the period of 8 weeks. Data was presented as mean ± standard error of the mean. The arrow denotes the start of metformin or placebo treatment.
Metabolic Profiles
Metformin did not affect fasting blood glucose levels, which were similar between the two groups before and after treatments (Figure 2). Serum insulin and insulin resistance measured by the HOMA-IR index were significantly lower in metformin group compared to control group (Figures 3A,B; Supplementary Table S1). There was no significant difference in adiponectin and leptin levels between groups (Figures 3C,D).
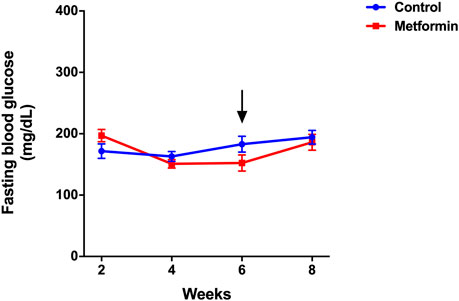
FIGURE 2. Fasting blood glucose of metformin and control groups over the period of 8 weeks. Data was presented as mean ± standard error of the mean. The arrow denotes the start of metformin or placebo treatment.
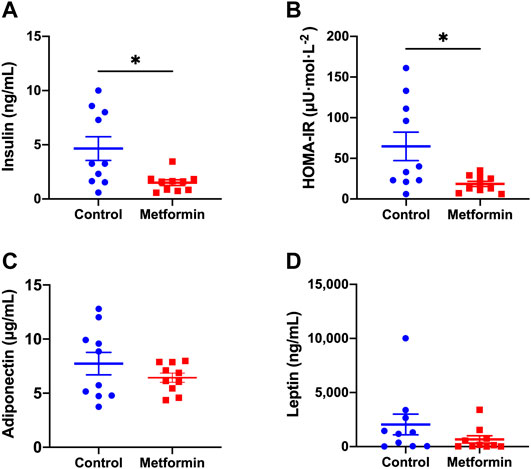
FIGURE 3. Metabolic profiles of metformin and control groups after treatments. Data was presented as mean ± standard error of the mean. * denote that these values were significantly different between two groups; Figure 3A: p = 0.0117; Figure 3B: p = 0.0179. HOMA-IR: Homeostatic Model Assessment for Insulin Resistance.
Methacholine Challenge Test
At baseline, metformin had no effect on Rrs, which was 0.54 ± 0.01 cm H2O.s/ml in the metformin group vs. 0.56 ± 0.02 cm H2O.s/ml in the control group. After a 3 mg/ml methacholine challenge, Rrs increased to a similar extent in both groups. However, after a high dose (30 mg/ml) methacholine challenge, the metformin-treated mice exhibited lower AHR than placebo-treated mice. At this dose of methacholine, metformin-treated mice had Rrs of 5.02 ± 0.76 cm H2O.s/ml showing a 9.2 ± 1.3-fold increase from baseline, whereas placebo-treated mice had Rrs of 8.13 ± 0.97 cm H2O.s/mL showing a 14.4 ± 1.6-fold increase from baseline; p = 0.0052 for the effect of metformin (Figure 4). Effect of metformin on lung elastance mirrored changes in Rrs (Supplementary Table S2).
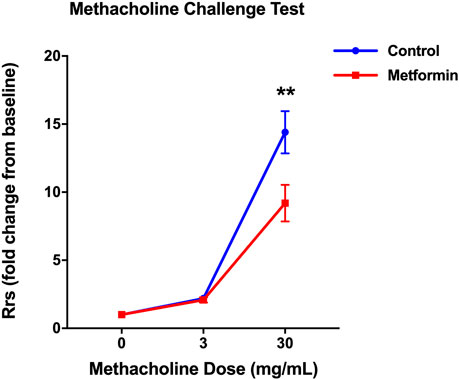
FIGURE 4. Metformin treatment decreased total resistance of the respiratory system (Rrs) in response to methacholine in diet-induced obese mice. The Rrs values were normalized to baseline (no significant difference between groups at baseline). Data was presented as mean ± standard error of the mean. ** denotes p < 0.01 between two groups.
Peripheral Blood Cells and Lung Volume Analysis
Metformin did not affect circulating white blood cell counts, but modestly decreased the platelet count compared to the control group (Table 2). Lung volumes were similar between the two groups, 0.17 ± 0.01 ml in metformin and 0.15 ± 0.01 ml in control group (p > 0.05).
Lung Cytokines
The transcription of interleukin (IL)-1β, IL-6, tumor necrosis factor alpha (TNF-α), and IL-18 in lung tissue were not significantly different between the two groups (Figure 5). Messenger RNAs of cytokines IL-5, IL-10, IL-13, and IL-17 were not detected in lung tissues of either mouse group.
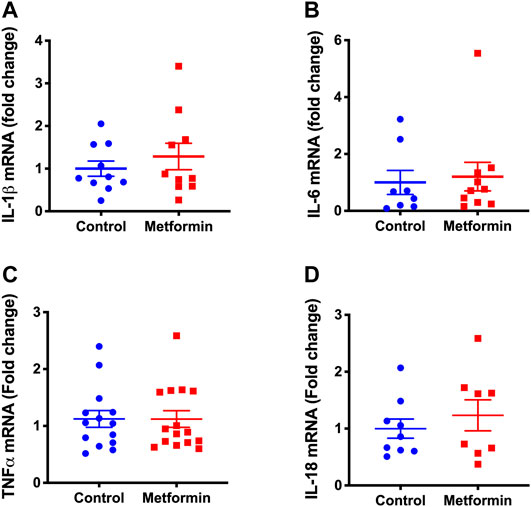
FIGURE 5. Transcription of lung cytokines in metformin and control groups after treatments. (A) Interleukin-1 beta (IL-1β), (B) Interleukin-6 (IL-6), (C) Tumor necrosis factor alpha (TNF-α), (D) Interleukin-18 (IL-18) mRNA levels in lung tissue of metformin and control groups after treatments. The results were expressed as relative fold changes to controls. Not detectable: IL-10, IL-17, IL-5, and IL-13 mRNA.
Discussion
The main finding of this study was that metformin treatment for 2 weeks alleviated AHR in a diet-induced obese asthma model. Reduction in AHR was associated with decreases in insulin resistance and the blood platelet count. In the following discussion, we address the potential mechanisms and translational implications of our findings.
AHR is a cardinal feature of asthma. The degree of AHR correlates with asthma severity, exacerbation, lung function decline, and mortality (Cockcroft et al., 1977; Brutsche et al., 2006; Leuppi et al., 2001; Becker et al., 2013). While several breakthroughs have been achieved in the treatment of eosinophilic asthma in recent years (Farne et al., 2017; Guntern and Eggel, 2020), therapy of obese non-eosinophilic asthma has not been effective. Recent human data suggest a link between obese asthma and insulin resistance (Cardet et al., 2016; Kim K.-M et al., 2014). Foer et al. provide further evidence that insulin resistance may cause or at least predispose to asthma as adult patients with asthma prescribed glucagon-like peptide-1 receptor (GLP-1R) agonists for type 2 diabetes had lower counts of asthma exacerbations (Foer et al., 2021). In diabetic cohorts, metformin users had lower risk of developing asthma, asthma exacerbations and asthma-related hospitalization than non-users (Li et al., 2016; Wu et al., 2019b; Chen et al., 2017). However, the effect of metformin on asthma has not been assessed in randomized controlled trials and mechanistic insights are lacking. A recent study showed that metformin decreases airway hyperreactivity in obese rats (Calco et al., 2021). Our experimental findings provide further evidence that metformin may be an effective treatment of obese asthma.
Studies linking metabolic syndrome to the risk of asthma and AHR in obesity have identified hyperinsulinemia as a culprit (Singh et al., 2013). In a Taiwanese diabetic cohort, insulin use increased the risk of developing asthma (Chen et al., 2017). Hyperinsulinemia in a high fat diet-induced obese rat model was associated with increased AHR to vagus nerve stimulation, which was prevented by reducing serum insulin with the pancreatic β-cell toxic agent, streptozotocin (Nie et al., 2014). Additionally, in vitro insulin treatment induced hypercontractility of airway smooth muscle (Nie et al., 2014; Dekkers et al., 2009), a phenotype described in asthmatic airways (Ma et al., 2002).
Our data showed that metformin corrected obesity-induced insulin resistance (↓HOMA-IR) and hyperinsulinemia independently of weight change and was associated with alleviation of AHR, supporting a role of insulin resistance in bronchial reactivity. This data is corroborated by findings by Calco et al which showed that male DIO rats treated with metformin had decreased bronchoconstriction induced by vagus nerve stimulation while groups treated solely with diet reversal did not experience the same reduction (Calco et al., 2021). On the other hand, our findings differ from prior studies in db/db mice (Shore et al., 2008) and obese Swiss mice (Dias et al., 2018) which show no effect of metformin on methacholine-induced AHR. These discrepancies could be attributed not only to strain differences, but also to the differences in metabolic profiles and methodologies for assessing AHR. First, db/db mice in Shore et al‘s study (Shore et al., 2008) were much more obese than the DIO mice in our study (body weight ∼60 vs. ∼ 43 g). Extreme obesity results in significant reductions in lung volumes and chest wall compliance due to mechanical effects of adiposity (Peters and Dixon, 2018). These mechanical factors augment AHR (Peters and Dixon, 2018), which is unlikely to be reduced by metformin. Second, db/db mice were severely diabetic, with ∼79 mg/dl higher fasting glucose in the control group (Shore et al., 2008) than the control mice in our study. Although metformin reduced fasting glucose by approximately 50% in db/db mice (Shore et al., 2008), residual hyperglycemia may also contribute to high post-treatment AHR. It is important to note that the fasting period for glucose measurement was much shorter in our study (6 h) than in Shore’s study (overnight) (Shore et al., 2008). The difference in fasting glucose between Shore’s db/db mice and our DIO mice could be even greater if the same period of fasting was administered. In fact, the lack of effect of metformin on fasting blood glucose in our study may represent the “floor effect”, whereas the correction of hyperinsulinemia without change in glucose suggested an improvement in insulin sensitivity. Similarly to our study, Dias et al reported that metformin decreased fasting insulin in obese Swiss mice without altering glucose (Dias et al., 2018). However, the relatively modest improvement in hyperinsulinemia and HOMA-IR in Dias et al. study may explain the unchanged AHR after metformin treatment. Overall, our data suggest that insulin resistance may be directly implicated in the pathogenesis of obese asthma, but molecular mechanisms remain uncertain.
Non-allergic airway inflammation is another feature of obese asthma (Kim H. Y et al., 2014; Younas et al., 2019; Fricke et al., 2018). We previously showed that diet-induced obesity in mice increased AHR in association with increased IL-1β gene expression in the lung (Younas et al., 2019) (Fricke et al., 2018) and IL-1β receptor blockade by anakinra prevented obesity-induced AHR (Younas et al., 2019), suggesting a mechanistic role of IL-1β in obese asthma. In the current study, metformin did not affect the expression of IL-1β or other pro-inflammatory cytokines in the lung. Metformin has been shown to inactivate toll-like receptor 4 and nuclear factor κB, interrupting the priming signal for the alveolar macrophage inflammasome NLRP3, an important mediator of obese asthma development in humans and mice (Kim H. Y et al., 2014). This mechanism is sufficient to suppress inflammasome-induced IL-1β activation in humans, but not in mice (Swanson et al., 2019). Metformin did not affect circulating white blood cell count and its components, but decreased peripheral platelet count in our model, suggesting a modest effect on systemic inflammation. Alternatively, metformin may affect platelets directly. In fact, metformin was shown to inhibit platelet activation and aggregation in in vitro and in vivo studies (Xin et al., 2016; Gin et al., 1989) as well as mtDNA release from platelets, an important upstream event in NLRP3 activation (Swanson et al., 2019). Platelets have been shown to actively contribute to certain features of asthma including AHR, allergic airway inflammation and airway remodeling (Idzko et al., 2015; (Takeda et al., 2018). Therefore, it is possible that metformin alleviated obesity-induced AHR through modifying the effect on platelets. Adipokine dysregulation has been shown to relate to insulin resistance (Rabe et al., 2008) and AHR (Shore et al., 2005; Coffey et al., 2015; Nigro et al., 2016). As metformin treatment did not significantly affect leptin or adiponectin levels in our model, it is unlikely that metformin improved AHR through modifying the effect of these adipokines.
Our study had several limitations. First, we did not measure metformin plasma levels (Chaudhari et al., 2020) and did not directly address mechanisms by which metformin improved AHR in our obese mouse model. Second, we did not measure pulmonary recruitment of platelets, which may play a role in obesity-induced AHR. Finally, we did not investigate sex differences by including female mice.
Conclusion
Metformin reduced airway hyperresponsiveness in diet-induced obese mice. This finding suggests that metformin can be considered for adjuvant pharmacological therapy in obese asthma. Human studies are warranted to examine the translational significance of these findings.
Data Availability Statement
The original contributions presented in the study are included in the article/Supplementary Material, further inquiries can be directed to the corresponding author.
Ethics Statement
The animal study was reviewed and approved by Institutional Animal Care and Use Committee of the Johns Hopkins University.
Author Contributions
CG, TW, JJ, MM, NH, WM, and VP conceived and carried out experiments. CG, JL, RL, and SB-F. carried out experiments. CG performed statistical analysis. All authors were involved in writing the paper and had final approval of the submitted and published versions.
Funding
NH, MM, and VP are supported by NIEHS grant P50 ES018176 and EPA Agreements 83615201 and 83451001. VP is also supported by NHLBI grants R01 HL128970, HL133100, and HL138932.
Conflict of Interest
The authors declare that the research was conducted in the absence of any commercial or financial relationships that could be construed as a potential conflict of interest.
Publisher’s Note
All claims expressed in this article are solely those of the authors and do not necessarily represent those of their affiliated organizations, or those of the publisher, the editors and the reviewers. Any product that may be evaluated in this article, or claim that may be made by its manufacturer, is not guaranteed or endorsed by the publisher.
Supplementary Material
The Supplementary Material for this article can be found online at: https://www.frontiersin.org/articles/10.3389/fphys.2022.883275/full#supplementary-material
Supplementary Table S1 | Raw data of insulin ELISA.
Supplementary Table S2 | Raw data of Methacholine Challenge Test.
References
An H., He L. (2016). Current Understanding of Metformin Effect on the Control of Hyperglycemia in Diabetes. J. Endocrinol. 228, R97–R106. doi:10.1530/JOE-15-0447
Bates J. H. T., Dixon A. E. (20151985). Potential Role of the Airway wall in the Asthma of Obesity. J. Appl. Physiol. 118, 36–41. doi:10.1152/japplphysiol.00684.2014
Becker E.-C., Wölke G., Heinrich J. (2013). Bronchial Responsiveness, Spirometry and Mortality in a Cohort of Adults. J. Asthma 50, 427–432. doi:10.3109/02770903.2013.769265
Bishai J. M., Mitzner W. (2008). Effect of Severe Calorie Restriction on the Lung in Two Strains of Mice. Am. J. Physiology-Lung Cell Mol. Physiol. 295, L356–L362. doi:10.1152/ajplung.00514.2007
Brutsche M. H., Downs S. H., Schindler C., Gerbase M. W., Schwartz J., Frey M., et al. (2006). Bronchial Hyperresponsiveness and the Development of Asthma and COPD in Asymptomatic Individuals: SAPALDIA Cohort Study. Thorax 61, 671–677. doi:10.1136/thx.2005.052241
Calco G. N., Proskocil B. J., Jacoby D. B., Fryer A. D., Nie Z. (2021). Metformin Prevents Airway Hyperreactivity in Rats with Dietary Obesity. Am. J. Physiology-Lung Cell Mol. Physiol. 321, L1105–L1118. doi:10.1152/ajplung.00202.2021
Calixto M. C., Lintomen L., André D. M., Leiria L. O., Ferreira D., Lellis-Santos C., et al. (2013). Metformin Attenuates the Exacerbation of the Allergic Eosinophilic Inflammation in High Fat-Diet-Induced Obesity in Mice. PLoS One 8, e76786. doi:10.1371/journal.pone.0076786
Cardet J. C., Ash S., Kusa T., Camargo C. A., Israel E. (2016). Insulin Resistance Modifies the Association between Obesity and Current Asthma in Adults. Eur. Respir. J. 48, 403–410. doi:10.1183/13993003.00246-2016
Chaudhari K., Wang J., Xu Y., Winters A., Wang L., Dong X., et al. (2020). Determination of Metformin Bio-Distribution by LC-MS/MS in Mice Treated with a Clinically Relevant Paradigm. PLOS ONE 15, e0234571. doi:10.1371/journal.pone.0234571
Chen C.-Z., Hsu C.-H., Li C.-Y., Hsiue T.-R. (2017). Insulin Use Increases Risk of Asthma but Metformin Use Reduces the Risk in Patients with Diabetes in a Taiwanese Population Cohort. J. Asthma 54, 1019–1025. doi:10.1080/02770903.2017.1283698
Cockcroft D. W., Killian D. N., Mellon J. J. A., Hargreave F. E. (1977). Bronchial Reactivity to Inhaled Histamine: a Method and Clinical Survey. Clin. Exp. Allergy 7, 235–243. doi:10.1111/j.1365-2222.1977.tb01448.x
Coffey M. J., Torretti B., Mancuso P. (20152015). Adipokines and Cysteinyl Leukotrienes in the Pathogenesis of Asthma. J. Allergy 2015, 1–9. doi:10.1155/2015/157919
Dekkers B. G. J., Schaafsma D., Tran T., Zaagsma J., Meurs H. (2009). Insulin-induced Laminin Expression Promotes a Hypercontractile Airway Smooth Muscle Phenotype. Am. J. Respir. Cel Mol Biol 41, 494–504. doi:10.1165/rcmb.2008-0251OC
Dias M. D., Goulart M., Dalécio C., Enes-Marques S., Salles É. d. S. L., Venâncio M., et al. (2018). Metformin Influences on Respiratory System in Obese Mice Induced by Postnatal Overnutrition. Respir. Physiol. Neurobiol. 247, 96–102. doi:10.1016/j.resp.2017.09.010
Dixon A. E., Peters U. (2018). The Effect of Obesity on Lung Function. Expert Rev. Respir. Med. 12, 755–767. doi:10.1080/17476348.2018.1506331
Farne H. A., Wilson A., Powell C., Bax L., Milan S. J. (2017). Anti-IL5 Therapies for Asthma. Cochrane Database Syst. Rev. 9, CD010834. doi:10.1002/14651858.CD010834.pub3
Foer D., Beeler P. E., Cui J., Karlson E. W., Bates D. W., Cahill K. N. (2021). Asthma Exacerbations in Patients with Type 2 Diabetes and Asthma on Glucagon-like Peptide-1 Receptor Agonists. Am. J. Respir. Crit. Care Med. 203, 831–840. doi:10.1164/rccm.202004-0993OC
Fricke K., Vieira M., Younas H., Shin M.-K., Bevans-Fonti S., Berger S., et al. (2018). High Fat Diet Induces Airway Hyperresponsiveness in Mice. Sci. Rep. 8, 6404. doi:10.1038/s41598-018-24759-4
Gin H., Freyburger G., Boisseau M., Aubertin J. (1989). Study of the Effect of Metformin on Platelet Aggregation in Insulin-dependent Diabetics. Diabetes Res. Clin. Pract. 6, 61–67. doi:10.1016/0168-8227(89)90058-2
Guntern P., Eggel A. (2020). Past, Present, and Future of anti‐IgE Biologics. Allergy 75, 2491–2502. doi:10.1111/all.14308
Guo Y., Shi J., Wang Q., Hong L., Chen M., Liu S., et al. (2021). Metformin Alleviates Allergic Airway Inflammation and Increases Treg Cells in Obese Asthma. J. Cel Mol Med 25, 2279–2284. doi:10.1111/jcmm.16269
Hyun B., Shin S., Lee A., Lee S., Song Y., Ha N.-J., et al. (2013). Metformin Down-Regulates TNF-α Secretion via Suppression of Scavenger Receptors in Macrophages. Immune Netw. 13, 123–132. doi:10.4110/in.2013.13.4.123
Idzko M., Pitchford S., Page C. (2015). Role of Platelets in Allergic Airway Inflammation. J. Allergy Clin. Immunol. 135, 1416–1423. doi:10.1016/j.jaci.2015.04.028
Jones R. L., Nzekwu M.-M. U. (2006). The Effects of Body Mass index on Lung Volumes. Chest 130, 827–833. doi:10.1378/chest.130.3.827
Kapsali T., Permutt S., Laube B., Scichilone N., Togias A. (20001985). Potent Bronchoprotective Effect of Deep Inspiration and its Absence in Asthma. J. Appl. Physiol. 89, 711–720. doi:10.1152/jappl.2000.89.2.711
Kim H. Y., Lee H. J., Chang Y.-J., Pichavant M., Shore S. A., Fitzgerald K. A., et al. (2014). Interleukin-17-producing Innate Lymphoid Cells and the NLRP3 Inflammasome Facilitate Obesity-Associated Airway Hyperreactivity. Nat. Med. 20, 54–61. doi:10.1038/nm.3423
Kim K.-M., Kim S.-S., Lee S.-H., Song W.-J., Chang Y.-S., Min K.-U., et al. (2014). Association of Insulin Resistance with Bronchial Hyperreactivity. Asia Pac. Allergy 4, 99–105. doi:10.5415/apallergy.2014.4.2.99
Leuppi J. D., Salome C. M., Jenkins C. R., Anderson S. D., Xuan W., Marks G. B., et al. (2001). Predictive Markers of Asthma Exacerbation During Stepwise Dose Reduction of Inhaled Corticosteroids. Am. J. Respir. Crit. Care Med. 163 (2), 406–12.
Li C.-Y., Erickson S. R., Wu C.-H. (2016). Metformin Use and Asthma Outcomes Among Patients with Concurrent Asthma and Diabetes. Respirology 21, 1210–1218. doi:10.1111/resp.12818
Limjunyawong N., Mock J., Mitzner W. (2015). Instillation and Fixation Methods Useful in Mouse Lung Cancer Research. J. Vis. Exp. (102), e52964. doi:10.3791/52964
Ma X., Cheng Z., Kong H., Wang Y., Unruh H., Stephens N. L., et al. (2002). Changes in Biophysical and Biochemical Properties of Single Bronchial Smooth Muscle Cells from Asthmatic Subjects. Am. J. Physiology-Lung Cell Mol. Physiol. 283, L1181–L1189. doi:10.1152/ajplung.00389.2001
Matthews D. R., Hosker J. P., Rudenski A. S., Naylor B. A., Treacher D. F., Turner R. C. (1985). Homeostasis Model Assessment: Insulin Resistance and ?-cell Function from Fasting Plasma Glucose and Insulin Concentrations in Man. Diabetologia 28, 412–419. doi:10.1007/bf00280883
McKeever T. M., Weston P. J., Hubbard R., Fogarty A. (2005). Lung Function and Glucose Metabolism: an Analysis of Data from the Third National Health and Nutrition Examination Survey. Am. J. Epidemiol. 161, 546–556. doi:10.1093/aje/kwi076
Mead J., Takishima T., Leith D. (1970). Stress Distribution in Lungs: a Model of Pulmonary Elasticity. J. Appl. Physiol. 28, 596–608. doi:10.1152/jappl.1970.28.5.596
Nie Z., Jacoby D. B., Fryer A. D. (2014). Hyperinsulinemia Potentiates Airway Responsiveness to Parasympathetic Nerve Stimulation in Obese Rats. Am. J. Respir. Cel Mol Biol 51, 140307075149003–140307075149261. doi:10.1165/rcmb.2013-0452OC
Nigro E., Matteis M., Roviezzo F., Mattera Iacono V., Scudiero O., Spaziano G., et al. (2016). Role of Adiponectin in Sphingosine-1-Phosphate Induced Airway Hyperresponsiveness and Inflammation. Pharmacol. Res. 103, 114–122. doi:10.1016/j.phrs.2015.10.004
Park C. S., Bang B.-R., Kwon H.-S., Moon K.-A., Kim T.-B., Lee K.-Y., et al. (2012). Metformin Reduces Airway Inflammation and Remodeling via Activation of AMP-Activated Protein Kinase. Biochem. Pharmacol. 84, 1660–1670. doi:10.1016/j.bcp.2012.09.025
Peters U., Dixon A. E., Forno E. (2018). Obesity and Asthma. J. Allergy Clin. Immunol. 141, 1169–1179. doi:10.1016/j.jaci.2018.02.004
Rabe K., Lehrke M., Parhofer K. G., Broedl U. C. (2008). Adipokines and Insulin Resistance. Mol. Med. 14, 741–751. doi:10.2119/2008-00058
Raviv S., Dixon A. E., Kalhan R., Shade D., Smith L. J. (2011). Effect of Obesity on Asthma Phenotype Is Dependent upon Asthma Severity. J. Asthma 48, 98–104. doi:10.3109/02770903.2010.534220
Rena G., Hardie D. G., Pearson E. R. (2017). The Mechanisms of Action of Metformin. Diabetologia 60, 1577–1585. doi:10.1007/s00125-017-4342-z
Saisho Y. (2015). Metformin and Inflammation: Its Potential Beyond Glucose-Lowering Effect. Endocr. Metab. Immune. Disord. Drug Targets 15 (3), 196–205.
Shore S. A., Schwartzman I. N., Mellema M. S., Flynt L., Imrich A., Johnston R. A. (2005). Effect of Leptin on Allergic Airway Responses in Mice. J. Allergy Clin. Immunol. 115, 103–109. doi:10.1016/j.jaci.2004.10.007
Shore S. A., Williams E. S., Zhu M. (20081985). No Effect of Metformin on the Innate Airway Hyperresponsiveness and Increased Responses to Ozone Observed in Obese Mice. J. Appl. Physiol. 105, 1127–1133. doi:10.1152/japplphysiol.00117.2008
Singh S., Prakash Y. S., Linneberg A., Agrawal A. (20132013). Insulin and the Lung: Connecting Asthma and Metabolic Syndrome. J. Allergy 2013, 1–8. doi:10.1155/2013/627384
Swanson K. V., Deng M., Ting J. P.-Y. (2019). The NLRP3 Inflammasome: Molecular Activation and Regulation to Therapeutics. Nat. Rev. Immunol. 19, 477–489. doi:10.1038/s41577-019-0165-0
Takeda T., Morita H., Saito H., Matsumoto K., Matsuda A. (2018). Recent Advances in Understanding the Roles of Blood Platelets in the Pathogenesis of Allergic Inflammation and Bronchial Asthma. Allergol. Int. 67, 326–333. doi:10.1016/j.alit.2017.11.008
Vaez H., Najafi M., Toutounchi N. S., Barar J., Barzegari A., Garjani A. (2016). Metformin Alleviates Lipopolysaccharide-Induced Acute Lung Injury through Suppressing Toll-like Receptor 4 Signaling. Iran J. Allergy Asthma Immunol. 15, 498–507.
World Health Organization (2021). Obesity and Overweight. Available at: https://www.who.int/news-room/fact-sheets/detail/obesity-and-overweight (Accessed February 22, 2022).
Wu T. D., Brigham E. P., Keet C. A., Brown T. T., Hansel N. N., McCormack M. C. (2019a). Association between Prediabetes/Diabetes and Asthma Exacerbations in a Claims-Based Obese Asthma Cohort. J. Allergy Clin. Immunol. Pract. 7, 1868–1873. doi:10.1016/j.jaip.2019.02.029
Wu T. D., Keet C. A., Fawzy A., Segal J. B., Brigham E. P., McCormack M. C. (2019b). Association of Metformin Initiation and Risk of Asthma Exacerbation. A Claims-Based Cohort Study. Ann. ATS 16, 1527–1533. doi:10.1513/AnnalsATS.201812-897OC
Xin G., Wei Z., Ji C., Zheng H., Gu J., Ma L., et al. (2016). Metformin Uniquely Prevents Thrombosis by Inhibiting Platelet Activation and mtDNA Release. Sci. Rep. 6, 36222. doi:10.1038/srep36222
Keywords: obesity, asthma, insulin resistance, metformin, mouse model
Citation: Gu C, Loube J, Lee R, Bevans-Fonti S, Wu TD, Barmine JH, Jun JC, McCormack MC, Hansel NN, Mitzner W and Polotsky VY (2022) Metformin Alleviates Airway Hyperresponsiveness in a Mouse Model of Diet-Induced Obesity. Front. Physiol. 13:883275. doi: 10.3389/fphys.2022.883275
Received: 24 February 2022; Accepted: 14 March 2022;
Published: 29 April 2022.
Edited by:
Yu Ru Kou, Hualien Tzu Chi Hospital, TaiwanReviewed by:
Katarzyna Kaczynska, Polish Academy of Sciences, PolandMatthew Poynter, University of Vermont, United States
Dale Bergren, Creighton University, United States
Copyright © 2022 Gu, Loube, Lee, Bevans-Fonti, Wu, Barmine, Jun, McCormack, Hansel, Mitzner and Polotsky. This is an open-access article distributed under the terms of the Creative Commons Attribution License (CC BY). The use, distribution or reproduction in other forums is permitted, provided the original author(s) and the copyright owner(s) are credited and that the original publication in this journal is cited, in accordance with accepted academic practice. No use, distribution or reproduction is permitted which does not comply with these terms.
*Correspondence: Vsevolod Y. Polotsky, dnBvbG90czFAamhtaS5lZHU=