- 1Department of Cardiology, Nanjing First Hospital, Nanjing Medical University, Nanjing, China
- 2Department of Intensive Medicine, The Affiliated Jiangning Hospital of Nanjing Medical University, Nanjing, China
Heart failure (HF), the terminal state of different heart diseases, imposed a significant health care burden worldwide. It is the last battlefield in dealing with cardiovascular diseases. HF with preserved ejection fraction (HFpEF) is a type of HF in which the symptoms and signs of HF are mainly ascribed to diastolic dysfunction of left ventricle, whereas systolic function is normal or near-normal. Compared to HF with reduced ejection fraction (HFrEF), the diagnosis and treatment of HFpEF have made limited progress, partly due to the lack of suitable animal models for translational studies in the past. Given metabolic disturbance and inflammatory burden contribute to HFpEF pathogenesis, recent years have witnessed emerging studies focusing on construction of animal models with HFpEF phenotype by mimicking metabolic disorders. These models prefer to recapitulate the metabolic disorders and endothelial dysfunction, leading to the more detailed understanding of the entity. In this review, we summarize the currently available animal models of HFpEF with metabolic disorders, as well as their advantages and disadvantages as tools for translational studies.
Introduction
Heart failure (HF) refers to a complex clinical syndrome when cardiac output failed to meet the body’s need. The typical symptoms and signs include dyspnea, fatigue, lower limb edema and so on (Witte and Clark, 2007). At age of 40 years, the lifetime risk for HF was nearly 20% for both men and women (Lloyd-Jones et al., 2002). Despite considerable advances in clinical management and treatment, morbidity and mortality of HF remain high after initial diagnosis (Metra and Teerlink, 2017).
The understandings of HF are dynamic and new challenges continue to emerge. Considering any cardiac structural and/or functional dysfunctions might contribute to HF, updated classification of HF is proposed according to left ventricular ejection fraction (LVEF) (Writing Committee et al., 2021). The four classes of HF are HF with reduced ejection fraction (HFrEF), where LVEF ≤40%; HF with median range ejection fraction (HFmrEF), where LVEF ranged from 41% to 49%; HF with preserved ejection fraction (HFpEF), where LVEF ≥50%; and HF with improved ejection fraction (HFimpEF), where LVEF ≤40% at baseline, but increase ≥10% from baseline and LVEF >40% at the second measurement.
Notably, HFpEF and HFrEF are the two most common types of HF. HFrEF has an overt decreasing of myocardial systolic capacity, that is often caused by myocardial infarction, hypertension, heart valvular diseases and dilated cardiomyopathy. Due to the relatively clear etiology of HFrEF, many animal models have been established for HFrEF researches (Noll et al., 2020).
The surgically induced HFrEF models are most widely used in mechanistic studies. For example, the hallmark murine model with thoracic aorta constriction (TAC) reported by Rockman (Rockman et al., 1991; Rockman et al., 1994), which became the standard surgery to construct stress-load-induced HFrEF. Post-myocardial infarction model created by the left anterior descending artery (LAD) ligation is usually adopted for exploring the mechanisms and treatment alternatives for ischemic HF (Sawall et al., 2017). Moreover, the pulmonary artery constriction-induced right ventricular hypertrophy and right HF model was successfully established to evaluate pathophysiological mechanisms of right ventricular dysfunction (Rockman et al., 1994; Wang et al., 2019). Furthermore, uninephrectomy, combined with angiotensin II and salt loading were applied in mice to recreate HF model associated with hypertensive heart disease (Tsukamoto et al., 2013).
Besides that, there are HFrEF models established by single medicine induction, including isoproterenol (Chang et al., 2018), angiotensin II (Pan G et al., 2018), alcohol (Guo and Ren, 2010) and doxorubin (Zeiss et al., 2019). These models have been adopted in study of specific intrinsic or extrinsic stimulus-induced HF. Furthermore, gene editing models were used in HFrEF model construction, given that genetic factors play an important role in development of dilated cardiomyopathy. For example, RNA-binding motif protein-20 (RBM20) gene-edited pigs (Schneider et al., 2020) and myosin binding protein C knockout mouse model (MYBPC3−/−) (Harris et al., 2002) showed HFrEF phenotypes. These models have facilitated the development of molecular diagnosis and targeted therapeutic approaches for HFrEF.
Metabolic Syndrome and HFpEF
HFpEF accounts for about 50% of all cases with HF diagnosis. However, there is a scarcity of definite etiologies and unanimous clinical diagnostic criteria for HFpEF. Furthermore, effective therapeutic alternatives, such as inhibitors of the renin-angiotensin system, beta blockers, aldosterone inhibitors and neprylisin inhibitors (Ferrari et al., 2020) for HFrEF, have failed to demonstrate improvements in mortality for patients with HFpEF.
An impediment for developing effective therapies comes from the diversity of clinical comorbidities and heterogeneities of HFpEF patients. However, many comorbidities of HFpEF are metabolic disorders. Metabolic syndrome is the aggregate of cardiovascular risk factors comprising central and abdominal obesity, systemic hypertension, insulin resistance (or type 2 diabetes mellitus), and atherogenic dyslipidemia. These conditions are interrelated and share underlying mediators (Eckel et al., 2005). Metabolic disorders are associated with altered substrate utilization and energy transduction in the myocardium and skeletal muscles. Energy molecule adenosine triphosphate (ATP) production is reduced due to mitochondrial free radical damage in HFpEF (Bates et al., 2014). It is suggested that increased glycolysis is the earliest energy metabolic change during HFpEF development. However, uncoupling of glycolysis and glucose oxidation may occur in HFpEF (Fillmore et al., 2018). In a metabolic risk-related rat model of HFpEF, the free mitochondrial calcium concentration is higher in HFpEF owing to alterations in mitochondrial and cytosolic Ca2+ handling. it might trigger mitochondrial permeability transition pore opening and reduced ATP production (Miranda-Silva et al., 2020).In addition, Ca2+ reuptake by sarcoplasmic reticulum in cardiomyocytes is primarily dependent on Ca2+ -ATPase (SERCA2a). Increased levels of reactive oxygen species (ROS) in cardiomyocytes impaired the phosphorylation of SERCA2a, and reduced the ability of the sarcoplasmic reuptake of Ca2+(Gómez-Viquez et al., 2021; McCandless et al., 2022), leading to intracellular Ca2+ overload and diastolic dysfunction (Runte et al., 2017; Silverman et al., 2020).
Insulin resistance also induces endothelial dysfunction. In obese rats with HFpEF, arginine-metabolizing enzymes were noticed to be increased in blood and heart, and nitric oxide (NO) turnover was reduced (Büttner et al., 2021). PROMIS-HFpEF study demonstrated a high prevalence of coronary microvascular dysfunction in HFpEF without unrevascularized macrovascular stenosis (Shah et al., 2018).
Chronic low-level metabolic inflammation (Withaar et al., 2021a; Withaar et al., 2021b; Mishra and Kass, 2021) is predisposed to the development of HFpEF as well. The mouse model of HFpEF, increased assembly of NLPR3 inflammasome on hyperacetylated mitochondria contributes to overproduction of interleukin-1β/IL-18 and tissue fibrosis (Deng et al., 2021). Plasma fibroblast growth factor 23 (FGF23) is higher in HFpEF patients and is strongly associated with exercise incapacity and prognosis (Kanagala et al., 2020). Plasma IL-6 level is associated with an increased risk of developing HFpEF in community-dwelling individuals, independent of potential confounders. (Chia et al., 2021) (Figure 1).
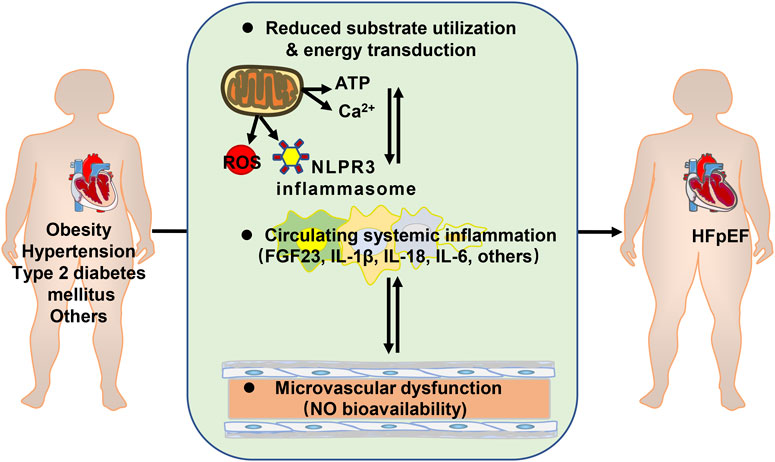
FIGURE 1. Metabolic disorders contributing to heart failure with preserved ejection fraction (HFpEF). ATP: adenosine triphosphate; ROS: reactive oxygen species; FGF23: fibroblast growth factor 23; IL: interleukin; NO: nitric oxide.
Machine learning and cluster analysis were applied to identify similar patients based on their combined features. In a previous study, female patients were divided into three groups. The first group had a low proportion of vascular risk factors. The second group had a higher proportion of hypertension and diabetes, but lower proportion of kidney disease and diastolic dysfunction. The third group had higher rates of atrial fibrillation (AF) and chronic kidney disease and worst long-term outcomes. Similarly, three groups of male patients were identified by machine learning. The first group had a high proportion of coronary artery disease (CAD), dyslipidemia, higher serum creatinine, and diastolic dysfunction. The second group had highest BMI, and high proportion of CAD, obstructive sleep apnea, and poorly controlled diabetes. The third group had high rates of AF, elevated brain natriuretic peptide (BNP) and biventricular remodeling with a higher cardiovascular mortality (Nouraei and Rabkin, 2021). The high prevalence of metabolic disorders suggests metabolic diseases should be taken into consideration during constructing appropriate animal models for clinical translation. Herein, we reviewed animal models for HFpEF with metabolic disorders (Table 1).
Small Animal Models of HFpEF
Mice are the most commonly used animal models in HF studies because they share most of their genes with humans, and approximately 85% of the protein-coding regions of the human genome can be found in the mouse genome. Mice also have the advantages of short reproductive cycle, low feeding cost, and being ready to gene editing. At present, some rodent HFpEF models have been constructed through chemical agents, diet, surgical intervention, genome editing and so on.
How to Evaluate Cardiac Function of Small Animals
To build the animal models of HFpEF, tools to assess the atrial, ventricular or overall cardiac function of small animals should be established first. However, imaging or functional modalities are limited in small animals for estimating cardiac function. Up to date, aggressive cardiac pressure-volume loop analysis has been deemed as the “gold standard” in assessment of load-dependent and load-independent cardiac function. After the preparation of anesthesia and mechanical ventilation, conductance catheter had to be inserted through rodents’ apical stab or right carotid artery, which generally end up at the cost of termination of modeling. It was suggested that transient aortic occlusion can be used to alter afterload, whereas transient inferior Vena Cava occlusion can be used to alter preload. Hemodynamic parameters such as LV end-systolic pressure, LV end-diastolic pressure (LVEDP), peak positive LV dp/dt, peak negative LV dp/dt, time constant relaxation (tau), stroke volume, and cardiac output could be analyzed by pressure-volume conductance catheter technique (Warren, 1974; Pacher et al., 2008; Abraham and Mao, 2015).
Transthoracic echocardiography (TTE) specific to small animals (Fayssoil and Tournoux, 2013; Schnelle et al., 2018; Wang et al., 2018) were performed to evaluate ventricular function of small animals, with the advantage of non-aggressive modality. It is pivotal for evaluating the preservation of ventricular function and stability of HFpEF phenotype in time course studies. Myocardial systolic function in small animals assessed by echocardiography were ventricular EF, fraction shortening (FS) and +dp/dtmax. Impaired diastolic function in echocardiographic transmitral flow profile showed a “restrictive” filling pattern with increased early (E-wave) and decreased late (A-wave) diastolic transmitral filling velocities. Augmented Doppler E/E’ and left atrial diameter, rapid deceleration of the early filling wave, isovolumic relaxation time (IVRT) as well as diastolic reverse peak longitudinal strain rate (RPLSR) were important to estimate diastolic dysfunction.
In addition to hemodynamic and imaging measurements, exercise stress and energy metabolism can be used to evaluate cardiovascular function when taking mouse physiology into account. Therefore, the mouse-graded maximal exercise testing (GXTm) was designed by staged increases in inclination as speed progress until exercise exhaustion. During exercise test, metabolic chamber encloses the treadmill that mice run on. Run time, maximum run speed, oxygen consumption (VO2), carbon dioxide expiration (VCO2), and VCO2/VO2 could be generated from the gas exchange occurring inside the metabolic chamber. Therefore, GXTm could be used as a functional assessment to determine the cardio-metabolic phenotype of various small animal models (Petrosino et al., 2016).
Pharmacological Agents or Diet-Induced HFpEF Models
Hypertension, the chronic pathological stress resulting in cardiac hypertrophy and fibrosis, as well as renal dysfunction, is an important metabolic risk factor for HFpEF incidence. Angiotensin II (Ang II), a vasoactive peptide, is known to regulate physiological blood pressure and is one of main players in hypertension development (Forrester et al., 2018). Ang II is applied for studying hypertension-related heart diseases (Peng et al., 2011; Valero-Munoz et al., 2017; Brenes-Castro et al., 2018). Kamiya et al. (2021) found that C57BL/6J mice treated with Ang II showed a significant increase in LVEDP and end-diastolic pressure-volume at the 4th week. Significant fibrosis and cardiac hypertrophy were also observed in the Ang II group, with no significant difference in LVEF and E/A. They also found that Ang II-mediated cardiac fibrosis was dependent on a cardiac cytokine, transforming growth factor (TGF)-β1. However, ANG II has also been used to establish animal models for HFrEF (Pan G et al., 2018). Therefore, whether ANG II a pharmacological stimulus for HFpEF modeling or eventually develops into a HFrEF model largely depends on modeling time.
Obesity induces metabolic and inflammatory disturbances and is linked to incidence of HFpEF (Tabucanon et al., 2020; Mishra and Kass, 2021). After 18 weeks of high-fat diet (HFD) on 45% fat only, C57/B6 mice showed impaired diastolic function and increased ratio of wet lung weight/dry lung weight, suggesting pulmonary congestion. And high-fat-fed mice showed hyperglycaemia (Liu et al., 2018). Echocardiography implied that there was no difference in systolic function between normal diet group (ND) and HFD group, and values of LVEF in both groups were normal. LVEDP and Tau index were increased in HFD group, whereas -dp/dt was decreased. Mechanistically, HFD induced myocardial insults such as oxidative stress and apoptosis. Melatonin (N-acetyl-5-methoxytryptamine), the neurohormone that maintains circadian rhythms, is involved in the regulation of blood pressure (Stauch et al., 2019). It was found that melatonin protected the damaged myocardium and improved HFpEF phenotype by promoting fat-derived CTRP3 secretion. This model sheds insight on obesity-related HFpEF and indicates the potential role of melatonin in improving cardiac diastolic dysfunction in HFpEF.
Two-Hit or Multi-Hit HFpEF Models
HFpEF is a multi-etiological and complex clinical syndrome in clinical reality. Many prediction models for HFpEF incidence and prognosis were proposed recently, with a spectrum of clinical predictors such as obesity, old age, anemia, elevated blood pressure, renal dysfunction, atrial fibrillation and pulmonary hypertension (Reddy et al., 2018; Zhang J. X et al., 2019; Liang et al., 2021). As to an individual patient, the interaction of different pathological processes might contribute to HFpEF development. Therefore, two-hit or multi-hit models using the combined risks of metabolic syndrome and aging were introduced to establish small animal models of HFpEF. HFD with 60% fat and Nω-Nitro-l-arginine methyl ester hydrochloride (l-NAME) increased blood pressure and reduced glucose tolerance in C57BL/6N mice, leading to HFpEF phenotype such as poor left ventricular overall longitudinal strain % (GLS%), pulmonary edema, myocardial hypertrophy, cardiac fibrosis, increased aortic stiffness, decreased exercise endurance and the mitral flow deceleration time (Schiattarella et al., 2019). Notably, murine LVEF retention was observed in this model during the prolonged time course at the 5th, 15th, 24th, 50th week. Thus, considering a relatively short model-establishing period and prolonged EF preservation, this model is relatively ideal for studying pathophysiological mechanisms underlying HFpEF.
Given that aging is a pathological factor in the development of HFpEF, female, aged (18–22 months) C57BL/6J mice were administered with 60% HFD for 12 weeks and Ang II for the last 4 weeks (Withaar et al., 2020). Metabolic disorder related HFpEF phenotypes such as obesity, impaired glucose tolerance, and inflammation were observed in aging mice by HFD + Ang II intervention. HFD + Ang II mice demonstrated cardiac concentric hypertrophy assessed by echocardiography, with significantly decreased GLS% and diastolic RPLSR, suggesting that the diastolic ability of this group of mice was impaired. RNA-sequencing showed that in HFD + ANG II group, the expression of TGF families was upregulated. Intriguingly, the researchers found that male mice treated with HFD + Ang II showed HFrEF phenotype whereas female mice tended to be HFpEF phenotype. The gender difference is consistent with the clinical feature that women are more likely to develop HFpEF.
In addition to Ang II and l-NAME, deoxycorticosterone acetate (DOCA) and desoxycorticosterone pivalate (DOCP) were used as agent for increasing blood pressure and constructing two-hit or multi-hit model of hypertension. An obese, senile, and hypertensive murine model induced by HFD for 13 months combined DOCP in the last month successfully produced HFpEF phenotypes (Deng et al., 2021). Mice in HFD + DOCP group showed impaired glucose tolerance, myocardial hypertrophy, exercise intolerance, cardiac fibrosis, pulmonary edema, decreased -/dt, and significantly decreased end-diastolic volume. The HFpEF mice exhibited overproduction of IL-1β/IL-18. Mechanistically, the interaction between mitochondrial hyperacetylation and inflammation was a key driver in the pathogenesis of obesity and hypertension-related HFpEF, which can be salvaged by elevating the abundance of β-OHB in vivo and in vitro. These findings provide clues to targeting mitochondria-inflammation circuit to mitigate HFpEF.
Surgery-Induced HFpEF Models
Patients with chronic kidney diseases (CKD) and renal dysfunction represent a subpopulation of HFpEF with worse outcome (Kirkman et al., 2021). To study CKD-related HFpEF, uninephrectomy of mice is gradually adopted as a surgery to induce HFpEF phenotype (Valero-Munoz et al., 2016; Yang et al., 2020). 4 weeks after uninephrectomy, aldosterone or DOCA were administered, C57BL/6J mice manifested with pulmonary edema, increased mitral valve velocity E and A, and significantly reduced IVRT and deceleration time (DT). Mice that underwent uninephrectomy and received a continuous infusion of d-aldosterone (0.15 mg/h) for 4 weeks displayed HFpEF phenotype assessed by ultrasound and Millar catheter transducer (Zhang et al., 2021). HFpEF mice showed markedly upregulated levels of plasma IL-1β, IL-6, and tumor necrosis factor (TNF)-α. The advantage of surgical intervention is the shortening of modeling period. However, the surgical procedure is a challenge to the operators. Other limitations are bias between groups and poor reproducibility of different research groups.
Gene Editing Induced HFpEF Models
Some gene-edited mice were predisposed to develop HFpEF due to altered genotypes. Leptin resistant db/db mice constructed under the C57BL/6J background spontaneously developed severe obesity and diabetes (Chen et al., 1996; Chen et al., 2012; Alex et al., 2018; Monma et al., 2020). Alex et al. (2018) found that at 6 months of age, both male and female db/db mice developed HFpEF, showed as cardiac hypertrophy, cardiac fibrosis, and significantly higher E/E’ than WT group. In addition, the influence of sex difference on diastolic function in db/db mice was also studied. LVEDP of female db/db mice was significantly higher than that of female WT group. Shimokawa and his colleagues reported that cardiac diastolic function of db/db mice were impaired at 20 weeks, assessed with transthoracic echocardiography and invasive haemodynamic analysis. Interestingly, low-intensity pulsed ultrasound (LIPUS) therapy ameliorates cardiac diastolic dysfunction through improvement of eNOS-NO-cGMP-PKG pathway and cardiomyocyte Ca2+-handling system. They indicated that the LIPUS therapy significantly upregulated the expression of SERCA2a and phosphorylated eNOS (Monma et al., 2021). Some studies have reported significant reductions in LVEF in db/db mice compared with control (Barouch et al., 2006; Shen et al., 2009; Plante et al., 2015). Others have not shown any functional or structural differences between db/db mice and age-matched control (Ko et al., 2017). Due to these conflicting conclusions, further research is needed to determine whether db/db mice are really suitable for HFpEF study.
Other gene-edited mice with phenotypes of HFpEF have been reported. Sirtuin 3 plays a critical role in the regulation of endothelial glycolysis and angiogenesis. Research from Chen’s group showed loss of Sirtuin 3 in endothelial cells impaired myocardial microcirculation and induced diastolic dysfunction (He et al., 2017). Col4a3−/− mice was used in a model of human Alport syndrome with hypertension and kidney failure phenotype. Col4a3−/− mice manifested diastolic dysfunction, cardiac hypertrophy, fibrosis and pulmonary edema via upregulation of osteopontin (OPN). 2-Oxo-Glutarate Dehydrogenase-like (OGDHL), a mitochondrial protein involved in metabolic substrate fluxes and signaling, mediates OPN-induced mitochondrial dysfunction in promoting a HFpEF-like cardiac phenotype in this model (Yousefi et al., 2019). Aldehyde dehydrogenase 2 (ALDH2) is a mitochondrial aldehyde detoxifying enzyme in the metabolism of acetaldehyde, especially lipid peroxidation-derived reactive aldehyde under oxidative stress. ALDH2 rs671 polymorphism caused reduced activities of ALDH2. We found patients with ALDH2 rs671 polymorphism were associated with higher risk of HFpEF (Zhang N et al., 2019; Xia et al., 2020). HFD-fed type 2 diabetic ALDH2*2 mutant mice (Pan X et al., 2018) or ALDH2 knockout mice with diabetes induced by streptozotocin (Wang et al., 2016) exhibited HFpEF phenotypes when measured by cardiac echo stress test. Altogether, the limitation of knockout mouse models were the long reproductive cycle and high feeding cost.
Strain Induced HFpEF Models
The mouse strain had an important influence on the construction of HFpEF model. Meng et al. (2017) reported that AKR/J mice fed a 60% fat energy diet for 20 weeks showed ventricular hypertrophy, pulmonary artery remodeling, glucose intolerance, and increased LVEDP. And HFD-treated AKR/J mice had higher body weights and glycated hemoglobin levels. This model may be useful in study of pulmonary arterial hypertension secondary to HFpEF. Gevaert et al. found that another AKR/J background accelerated aging mice (SAM) showed HFpEF phenotype after HFD. The cardiac alteration of SAM + HFD at 24 weeks of age was diastolic dysfunction, left ventricular hypertrophy, left atrial dilatation, and interstitial fibrosis. Exercise capacity was reduced and lung weight increased in SAM + HFD mice (Gevaert et al., 2017). This model is useful for studying age-related HFpEF. However, compared with the physiologically senile mice with C57BL/6 background (Roh et al., 2020), the accelerated aging state of SAM-HFD model may affect the pathophysiological studies of HFpEF.
Rat Models of HFpEF
Obese diabetic Zucker fatty/spontaneously hypertensive HF F1 hybrid (ZSF1-OB) rats exhibit spontaneous type 2 diabetes, diabetic kidney disease, hypertension, hyperlipidemia, obesity and metabolic syndrome features (Hohendanner et al., 2018; Davila et al., 2019; Leite et al., 2019; Park et al., 2020; Schauer et al., 2020). A number of studies demonstrated that ZSF1-OB had cardiac manifestations such as normal LVEF, increased E/A, left atrial enlargement, elevated LVEDP, upward EDPVR curve, and reduced activity tolerance (Leite et al., 2019; Satoh et al., 2021; Schauer et al., 2021). In addition, compared with lean ZSF1(ZSF1-LN) in the control group, ZSF1-OB displayed significant aortic remodeling and arterial collagen deposition, central vascular remodeling, which aggravated the hypertensive phenotype and promoted HFpEF. ZSF1-OB is a commonly used rat model of HFpEF. However, because ZSF1 rats were constructed by crossing leptin non-hypertensive Zucker diabetic obese female rats (ZDF, +/FA) and leptin spontaneous hypertensive male rats with HF (SHHF/MCC, +/FACP), the ZSF1 rats had no ability to reproduce, which limited the generalization of this model. Bing et al. found that salt-sensitive rats (DSS) on a high-salt diet (HS) for 7 weeks showed increased left ventricular diastolic stiffness and decreased left ventricular relaxation (Bing et al., 2020; Yin et al., 2020). Zhang et al. revealed that compared with the low-salt diet group (DSS + LS), the endocardial and epicardial radial strain peaks of DSS + HS rats were significantly reduced (Zhang et al., 2020), accompanied by increased lung and kidney mass and impaired diastolic function. Additionally, glucose transporter 1 expression and cardiac glycolysis were increased. And a marked increase in proton production was observed. It should be noted that this model only represents part of HFpEF secondary to hypertension and is suitable for the study of the mechanisms of HFpEF induced by hypertension. A transgenic rat model that overexpresses human β3 -adrenoceptor (hβ3 -AR) in the endothelium was reported to assume increasing diastolic dysfunction with age (Dhot et al., 2020). Mesquita found reduced E/A, increased E/E’, increased lung weight, cardiac hypertrophy, atrial enlargement, and decreased motor ability in female Fischer F344 rats aged 21–24 months (Mesquita et al., 2020). In addition to diastolic dysfunction, this rat model also has a propensity for atrial fibrillation, which is helpful in exploring the causal relationship between atrial fibrillation and HFpEF. However, ion channel expression in the model was not measured, and AF in this rodent model is self-terminating, unlike human disease.
Large Animal Models of HFpEF
Although rodents are widely used as models to study the mechanisms of human heart diseases, they do not fully meet the needs of heart disease models due to limited information obtained by measurements of heart anatomic structures and function. The construction of large animal models using sheep, pigs or dogs provides approaches to solve this problem. But ethical concerns, cost, study duration and difficulties in gene editing limit large animal models of HFpEF.
How to Evaluate Cardiac Function of Large Animals
Similar to the evaluation of cardiac function of humans, clinical modalities, such as ECG-gated multidetector computed tomography (MDCT), cardiovascular magnetic resonance (CMR), echocardiography and cardiac catheter were used to assess functional, metabolic, and structural changes of myocardium in large animal models (LeBlanc et al., 2017). Myocardial perfusion and myocardial work could be calculated by positron emission tomography (PET), in combination with [(15)O] water and oxygen consumption using [(11)C] acetate (Tarkia et al., 2015). CMR at rest, as well as during dobutamine stress, were applied in estimate left ventricular/atrial function and myocardial mass in large animals. Additionally, strains and torsion were evaluated from tagged cine imaging. By virtue of 4D phase-contrast measurements, blood flow and peak velocities, including transmitral early-diastolic (E) and myocardial tissue (E’) velocities, as well as coronary sinus blood flow were assessed in large animals, making it possible to estimate myocardial perfusion reserve from stress-to-rest time-averaged coronary sinus flow (Reiter et al., 2016). Although these non-invasive methods provide a variety of choices to assess cardiac pathophysiological alterations, measurement of hemodynamics by cardiac catheter is still the golden standard to assess LV compliance and relaxation in HFpEF.
Surgery-Induced HFpEF Models
Hypertension-induced LV hypertrophy (OHT) was built by bilateral kidney ligation on elderly dogs that developed hypertension 8 weeks later. Compared with the control group, the OHT group showed HFpEF phenotype as increased left ventricular mass, myocardial hypertrophy, abnormal Tau index and BNP level. Mechanistically, functional impairment of sarcomeres may be an early event of HFpEF as this model suggested, mainly manifesting hypophosphorylation of myofilament proteins and increased Ca2+ sensitivity (Hamdani et al., 2013). However, the requirement for old animals (8–13 years old) makes this model relatively impractical and expensive.
Unilateral renal artery stenosis with renovascular hypertension (RVHT) was constructed by placing an irritant coil in the main renal artery under fluoroscopy, which leads to a gradual narrowing of the renal artery. In combination of HFD, this model displayed increased LV mass and diastolic dysfunction. In the HFD + RVHT pig model, the elevation in LV filling pressures with volume loading was mitigated by percutaneous approach using pericardial resection (Borlaug et al., 2017). Myocardial expression of TNF-α and IL-6 was upregulated in RVHT pigs. Furthermore, in pigs with RVHT, percutaneous transluminal renal angioplasty, along with intra-renal. Bendavia, a mitochondrial targeted peptide, can improve cardiac function in RVHT pigs. Mesenchymal stem cells delivery restored renal and cardiac function (Eirin et al., 2014; Eirin et al., 2015). This RVHT model hints the possibility of therapeutic options for patients with this specific subtype of HFpEF.
Multi-Hit HFpEF Models
Thirty-nine-week-old female landrace pigs that were delivered with Ang II and DOCA, and fed with HFD exhibited a significant increase in the LV thickness compared with the Normal group. Total cholesterol (TC), triglyceride (TG) and low-density lipoprotein (LDL) were markedly increased in HFpEF group. As expected, the levels of IL-6 and TNF-α were elevated in the aortas of HFpEF pigs. And the plasma BNP, plasma epinephrine, norepinephrine and Ang II concentrations in HFpEF pigs were strongly increased when compared with the normal group. Invasive right cardiac catheterization was performed to show that PASP and PCWP were significantly higher in HFpEF pigs compared with the normal group, especially at the 18th week (Zhang J. X et al., 2019).
HFpEF was induced in pigs by subcutaneous implantation of DOCA pellets along with a high-salt, high-sugar, high-potassium diet over 3 months. When compared with weight-matched normal pigs, cardiomyocyte changes were similar in subepicardial, midmyocardial and subendocardial regions but DOCA-induced changes in the interstitium appeared to be more pronounced in the subendocardial ventricular wall layers (Muhlfeld et al., 2020). The results of this study suggest a pivotal role of the subendocardial interstitium in the pathogenesis of HFpEF. Three comorbidities, including DM (induced by streptozotocin), hypercholesterolemia (produced by HFD), and hypertension (resulted from renal artery embolization), were established in female swine. The combination treatment leads to systemic inflammation, myocardial oxidative stress, and coronary microvascular dysfunction, which associate with myocardial stiffening and LV diastolic dysfunction with preserved EF (Sorop et al., 2018).
Summary and Perspectives
In this review, we summarized the published animal models of HFpEF and metabolic disorders, as well as the imaging and functional modalities for animal cardiac assessment (Figure 2). First and foremost, the reliability and stability of the existing HFpEF animal model should be considered, as well as the optimized evaluation approaches of HFpEF. Next, diagnostic criteria of HFpEF patients is used as a reference to evaluate success of animal phenotype. Therefore, researchers should pay attention to the physiological difference of the animals and human beings. Lastly, targeting cardiac metabolism has great potentials to ameliorate HFpEF prognosis. EMPEROR-Preserved study released that sodium-glucose cotransporter 2 inhibitors empagliflozin reduced the combined risk of cardiovascular death or hospitalization for patients with HFpEF. It is noteworthy that some HFmrEF patients have been clinically diagnosed with HFpEF and the pathogenesis. Nonetheless, therapeutic effects of HFmrEF are similar to HFrEF indeed. 33% of the patients in EMPEROR-Preserved study had a baseline EF of 41–50% and were actually HFmrEF patients (Anker et al., 2020; Anker et al., 2021). Whether empagliflozin treatment benefits HFpEF patients with LVEF ≥50% is unknown. New trials enrolled patients with LVEF ≥50% are crucial to ascertain the efficacy of novel HFpEF therapies. Potential metabolic targets to attenuate animal myocardial fibrosis and improve cardiac function are needed for clinical translation in the future.
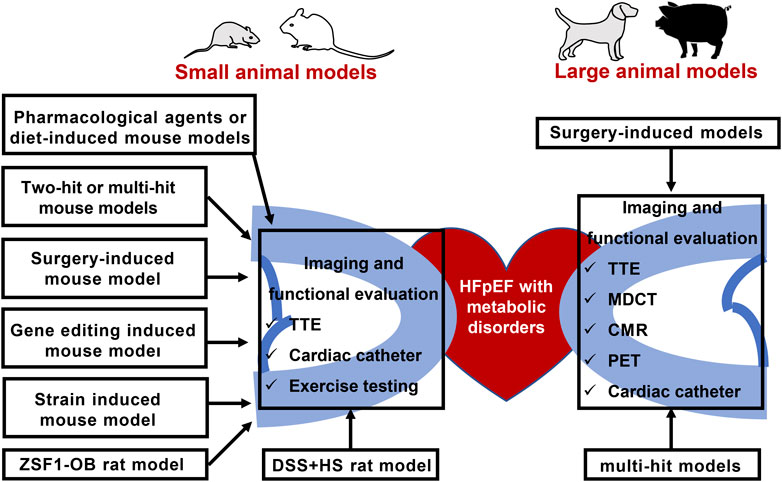
FIGURE 2. Animal models of HFpEF and metabolic disorders, as well as the imaging and functional modalities for animal cardiac assessment. TTE: Transthoracic echocardiography; HFpEF: heart failure with preserved ejection fraction; ZSF1-OB: Obese diabetic Zucker fatty/spontaneously hypertensive heart failure F1 hybrid rats; DSS + HS: salt-sensitive rats on a high-salt diet; PET: positron emission tomography; MDCT: multidetector computed tomography; CMR: cardiovascular magnetic resonance.
Author Contributions
HL, Y-YX, and C-LX drafted the manuscript. ZL prepared the table. YS prepared figures. J-XZ and X-BL revised it critically for important intellectual content and approved the final version to be submitted. All authors were involved in manuscript editing and approved the version submitted for publication.
Funding
This study is supported by the National Natural Science Foundation of China (NSFC) Grant No. 81970309 and No. 81700398.
Conflict of Interest
The authors declare that the research was conducted in the absence of any commercial or financial relationships that could be construed as a potential conflict of interest.
Publisher’s Note
All claims expressed in this article are solely those of the authors and do not necessarily represent those of their affiliated organizations, or those of the publisher, the editors and the reviewers. Any product that may be evaluated in this article, or claim that may be made by its manufacturer, is not guaranteed or endorsed by the publisher.
References
Abraham D., Mao L. (2015). Cardiac Pressure-Volume Loop Analysis Using Conductance Catheters in Mice. JoVE 103, 52942. doi:10.3791/52942
Alex L., Russo I., Holoborodko V., Frangogiannis N. G. (2018). Characterization of a Mouse Model of Obesity-Related Fibrotic Cardiomyopathy that Recapitulates Features of Human Heart Failure with Preserved Ejection Fraction. Am. J. Physiology-Heart Circulatory Physiol. 315 (4), H934–H949. doi:10.1152/ajpheart.00238.2018
Anker S. D., Butler J., Filippatos G., Ferreira J. P., Bocchi E., Böhm M., et al. (2021). Empagliflozin in Heart Failure with a Preserved Ejection Fraction. N. Engl. J. Med. 385 (16), 1451–1461. doi:10.1056/NEJMoa2107038
Anker S. D., Butler J., Filippatos G., Shahzeb Khan M., Ferreira J. P., Bocchi E., et al. (2020). Baseline Characteristics of Patients with Heart Failure with Preserved Ejection Fraction in the EMPEROR‐Preserved Trial. Eur. J. Heart Fail. 22 (12), 2383–2392. doi:10.1002/ejhf.2064
Barouch L. A., Gao D., Chen L., Miller K. L., Xu W., Phan A. C., et al. (2006). Cardiac Myocyte Apoptosis Is Associated with Increased DNA Damage and Decreased Survival in Murine Models of Obesity. Circ. Res. 98 (1), 119–124. doi:10.1161/01.RES.0000199348.10580.1d
Bates A., Shen Q., Hiebert J. B., Thimmesch A., Pierce J. D. (2014). Myocardial Energetics and Ubiquinol in Diastolic Heart Failure. Nurs. Health Sci. 16 (4), 428–433. doi:10.1111/nhs.12168
Bing F., Wang X., Shen W., Li L., Niu P., Chen Y., et al. (2020). Inhalation of Ultrafine Zinc Particles Impaired Cardiovascular Functions in Hypertension-Induced Heart Failure Rats with Preserved Ejection Fraction. Front. Bioeng. Biotechnol. 8, 13. doi:10.3389/fbioe.2020.00013
Borlaug B. A., Carter R. E., Melenovsky V., DeSimone C. V., Gaba P., Killu A., et al. (2017). Percutaneous Pericardial Resection. Circ. Heart Fail. 10 (4), e003612. doi:10.1161/CIRCHEARTFAILURE.116.003612
Bozkurt B., Coats A. J. S., Tsutsui H., Abdelhamid C. M., Adamopoulos S., Albert N., et al. (2021). Universal Definition and Classification of Heart Failure: a Report of the Heart Failure Society of America, Heart Failure Association of the European Society of Cardiology, Japanese Heart Failure Society and Writing Committee of the Universal Definition of Heart Failure. Eur. J. Heart Fail. 23, 352–380. doi:10.1002/ejhf.2115
Brenes-Castro D., Castillo E. C., Vázquez-Garza E., Torre-Amione G., García-Rivas G. (2018). Temporal Frame of Immune Cell Infiltration during Heart Failure Establishment: Lessons from Animal Models. Ijms 19 (12), 3719. doi:10.3390/ijms19123719
Büttner P., Werner S., Baskal S., Tsikas D., Adams V., Lurz P., et al. (2021). Arginine Metabolism and Nitric Oxide Turnover in the ZSF1 Animal Model for Heart Failure with Preserved Ejection Fraction. Sci. Rep. 11 (1), 20684. doi:10.1038/s41598-021-00216-7
Chang S. C., Ren S., Rau C. D., Wang J. J. (2018). Isoproterenol-Induced Heart Failure Mouse Model Using Osmotic Pump Implantation. Methods Mol. Biol. 1816, 207–220. doi:10.1007/978-1-4939-8597-5_16
Chen H., Charlat O., Tartaglia L. A., Woolf E. A., Weng X., Ellis S. J., et al. (1996). Evidence that the Diabetes Gene Encodes the Leptin Receptor: Identification of a Mutation in the Leptin Receptor Gene in Db/db Mice. Cell 84 (3), 491–495. doi:10.1016/s0092-8674(00)81294-5
Chen J.-X., Zeng H., Reese J., Aschner J. L., Meyrick B. (2012). Overexpression of Angiopoietin-2 Impairs Myocardial Angiogenesis and Exacerbates Cardiac Fibrosis in the Diabetic Db/db Mouse Model. Am. J. Physiology-Heart Circulatory Physiol. 302 (4), H1003–H1012. doi:10.1152/ajpheart.00866.2011
Chia Y. C., Kieneker L. M., van Hassel G., Binnenmars S. H., Nolte I. M., van Zanden J. J., et al. (2021). Interleukin 6 and Development of Heart Failure with Preserved Ejection Fraction in the General Population. Jaha 10 (11), e018549. doi:10.1161/jaha.120.018549
Davila A., Tian Y., Czikora I., Li J., Su H., Huo Y., et al. (2019). Adenosine Kinase Inhibition Augments Conducted Vasodilation and Prevents Left Ventricle Diastolic Dysfunction in Heart Failure with Preserved Ejection Fraction. Circ. Heart Fail. 12 (8), e005762. doi:10.1161/CIRCHEARTFAILURE.118.005762
Deng Y., Xie M., Li Q., Xu X., Ou W., Zhang Y., et al. (2021). Targeting Mitochondria-Inflammation Circuit by β-Hydroxybutyrate Mitigates HFpEF. Circ. Res. 128 (2), 232–245. doi:10.1161/CIRCRESAHA.120.317933
Dhot J., Ferron M., Prat V., Persello A., Roul D., Stévant D., et al. (2020). Overexpression of Endothelial β 3 ‐adrenergic Receptor Induces Diastolic Dysfunction in Rats. ESC Heart Fail. 7, 4159–4171. doi:10.1002/ehf2.13040
Eckel R. H., Grundy S. M., Zimmet P. Z. (2005). The Metabolic Syndrome. The Lancet 365 (9468), 1415–1428. doi:10.1016/S0140-6736(05)66378-7
Eirin A., Williams B. J., Ebrahimi B., Zhang X., Crane J. A., Lerman A., et al. (2014). Mitochondrial Targeted Peptides Attenuate Residual Myocardial Damage after Reversal of Experimental Renovascular Hypertension. J. Hypertens. 32 (1), 154–165. doi:10.1097/HJH.0b013e3283658a53
Eirin A., Zhu X.-Y., Ferguson C. M., Riester S. M., van Wijnen A. J., Lerman A., et al. (2015). Intra-renal Delivery of Mesenchymal Stem Cells Attenuates Myocardial Injury after Reversal of Hypertension in Porcine Renovascular Disease. Stem Cell Res Ther 6, 7. doi:10.1186/scrt541
Fayssoil A., Tournoux F. (2013). Analyzing Left Ventricular Function in Mice with Doppler Echocardiography. Heart Fail. Rev. 18 (4), 511–516. doi:10.1007/s10741-012-9345-8
Ferrari R., Cardoso J., Cardoso J., Fonseca M. C., Aguiar C., Moreira J. I., et al. (2020). ARNIs: Balancing "the Good and the Bad" of Neuroendocrine Response to HF. Clin. Res. Cardiol. 109 (5), 599–610. doi:10.1007/s00392-019-01547-2
Fillmore N., Levasseur J. L., Fukushima A., Wagg C. S., Wang W., Dyck J. R. B., et al. (2018). Uncoupling of Glycolysis from Glucose Oxidation Accompanies the Development of Heart Failure with Preserved Ejection Fraction. Mol. Med. 24 (1), 3. doi:10.1186/s10020-018-0005-x
Forrester S. J., Booz G. W., Sigmund C. D., Coffman T. M., Kawai T., Rizzo V., et al. (2018). Angiotensin II Signal Transduction: An Update on Mechanisms of Physiology and Pathophysiology. Physiol. Rev. 98 (3), 1627–1738. doi:10.1152/physrev.00038.2017
Gevaert A. B., Shakeri H., Leloup A. J., Van Hove C. E., De Meyer G. R. Y., Vrints C. J., et al. (2017). Endothelial Senescence Contributes to Heart Failure with Preserved Ejection Fraction in an Aging Mouse Model. Circ. Heart Fail. 10 (6). doi:10.1161/CIRCHEARTFAILURE.116.003806
Gómez-Viquez N. L., Balderas-Villalobos J., Bello-Sánchez M. D., Mayorga-Luna M., Mailloux-Salinas P., García-Castañeda M., et al. (2021). Oxidative Stress in Early Metabolic Syndrome Impairs Cardiac RyR2 and SERCA2a Activity and Modifies the Interplay of These Proteins during Ca2+ Waves. Arch. Physiol. Biochem. 1, 1–13. doi:10.1080/13813455.2021.1895224
Guo R., Ren J. (2010). Alcohol Dehydrogenase Accentuates Ethanol-Induced Myocardial Dysfunction and Mitochondrial Damage in Mice: Role of Mitochondrial Death Pathway. PLoS One 5 (1), e8757. doi:10.1371/journal.pone.0008757
Hamdani N., Bishu K. G., von Frieling-Salewsky M., Redfield M. M., Linke W. A. (2013). Deranged Myofilament Phosphorylation and Function in Experimental Heart Failure with Preserved Ejection Fraction. Cardiovasc. Res. 97 (3), 464–471. doi:10.1093/cvr/cvs353
Harris S. P., Bartley C. R., Hacker T. A., McDonald K. S., Douglas P. S., Greaser M. L., et al. (2002). Hypertrophic Cardiomyopathy in Cardiac Myosin Binding Protein-C Knockout Mice. Circ. Res. 90 (5), 594–601. doi:10.1161/01.res.0000012222.70819.64
He X., Zeng H., Chen S. T., Roman R. J., Aschner J. L., Didion S., et al. (2017). Endothelial Specific SIRT3 Deletion Impairs Glycolysis and Angiogenesis and Causes Diastolic Dysfunction. J. Mol. Cell Cardiol. 112, 104–113. doi:10.1016/j.yjmcc.2017.09.007
Hohendanner F., Bode D., Primessnig U., Guthof T., Doerr R., Jeuthe S., et al. (2018). Cellular Mechanisms of Metabolic Syndrome-Related Atrial Decompensation in a Rat Model of HFpEF. J. Mol. Cell Cardiol. 115, 10–19. doi:10.1016/j.yjmcc.2017.12.012
Kamiya M., Asai K., Maejima Y., Shirakabe A., Murai K., Noma S., et al. (2021). β3-Adrenergic Receptor Agonist Prevents Diastolic Dysfunction in an Angiotensin II-Induced Cardiomyopathy Mouse Model. J. Pharmacol. Exp. Ther. 376 (3), 473–481. doi:10.1124/jpet.120.000140
Kanagala P., Arnold J. R., Khan J. N., Singh A., Gulsin G. S., Eltayeb M., et al. (2020). Fibroblast‐growth‐factor‐23 in Heart Failure with Preserved Ejection Fraction: Relation to Exercise Capacity and Outcomes. ESC Heart Fail. 7 (6), 4089–4099. doi:10.1002/ehf2.13020
Kirkman D. L., Carbone S., Canada J. M., Trankle C., Kadariya D., Buckley L., et al. (2021). The Chronic Kidney Disease Phenotype of HFpEF: Unique Cardiac Characteristics. Am. J. Cardiol. 142, 143–145. doi:10.1016/j.amjcard.2020.12.012
Ko K.-Y., Wu Y.-W., Liu C.-W., Cheng M.-F., Yen R.-F., Yang W.-S. (2017). Longitudinal Evaluation of Myocardial Glucose Metabolism and Contractile Function in Obese Type 2 Diabetic Db/db Mice Using Small-Animal Dynamic 18F-FDG PET and Echocardiography. Oncotarget 8 (50), 87795–87808. doi:10.18632/oncotarget.21202
LeBlanc N. L., Scollan K. F., Stieger-Vanegas S. M. (2017). Cardiac Output Measured by Use of Electrocardiogram-Gated 64-slice Multidector Computed Tomography, Echocardiography, and Thermodilution in Healthy Dogs. Am. J. Vet. Res. 78 (7), 818–827. doi:10.2460/ajvr.78.7.818
Leite S., Cerqueira R. J., Ibarrola J., Fontoura D., Fernández-Celis A., Zannad F., et al. (2019). Arterial Remodeling and Dysfunction in the ZSF1 Rat Model of Heart Failure with Preserved Ejection Fraction. Circ. Heart Fail. 12 (7), e005596. doi:10.1161/CIRCHEARTFAILURE.118.005596
Liang W., Wu Y., Xue R., Wu Z., Wu D., He J., et al. (2021). C2HEST Score Predicts Clinical Outcomes in Heart Failure with Preserved Ejection Fraction: a Secondary Analysis of the TOPCAT Trial. BMC Med. 19 (1), 44. doi:10.1186/s12916-021-01921-w
Liu Y., Li L.-N., Guo S., Zhao X.-Y., Liu Y.-Z., Liang C., et al. (2018). Melatonin Improves Cardiac Function in a Mouse Model of Heart Failure with Preserved Ejection Fraction. Redox Biol. 18, 211–221. doi:10.1016/j.redox.2018.07.007
Lloyd-Jones D. M., Larson M. G., Leip E. P., Beiser A., D’Agostino R. B., Kannel W. B., et al. (2002). Lifetime Risk for Developing Congestive Heart Failure. Circulation 106 (24), 3068–3072. doi:10.1161/01.cir.0000039105.49749.6f
McCandless M. G., Altara R., Booz G. W., Kurdi M. (2022). What Role Do Mitochondria Have in Diastolic Dysfunction? Implications for Diabetic Cardiomyopathy and Heart Failure with Preserved Ejection Function (HFpEF). J. Cardiovasc. Pharmacol. 2022. Publish Ahead of Print. doi:10.1097/fjc.0000000000001228
Meng Q., Lai Y.-C., Kelly N. J., Bueno M., Baust J. J., Bachman T. N., et al. (2017). Development of a Mouse Model of Metabolic Syndrome, Pulmonary Hypertension, and Heart Failure with Preserved Ejection Fraction. Am. J. Respir. Cell Mol Biol 56 (4), 497–505. doi:10.1165/rcmb.2016-0177OC
Mesquita T. R. R., Zhang R., de Couto G., Valle J., Sanchez L., Rogers R. G., et al. (2020). Mechanisms of Atrial Fibrillation in Aged Rats with Heart Failure with Preserved Ejection Fraction. Heart Rhythm 17 (6), 1025–1033. doi:10.1016/j.hrthm.2020.02.007
Metra M., Teerlink J. R. (2017). Heart Failure. The Lancet 390 (10106), 1981–1995. doi:10.1016/S0140-6736(17)31071-1
Miranda‐Silva D., Wüst R. C. I., Conceição G., Gonçalves‐Rodrigues P., Gonçalves N., Gonçalves A., et al. (2020). Disturbed Cardiac Mitochondrial and Cytosolic Calcium Handling in a Metabolic Risk‐related Rat Model of Heart Failure with Preserved Ejection Fraction. Acta Physiol. 228 (3), e13378. doi:10.1111/apha.13378
Mishra S., Kass D. A. (2021). Cellular and Molecular Pathobiology of Heart Failure with Preserved Ejection Fraction. Nat. Rev. Cardiol. 18 (6), 400–423. doi:10.1038/s41569-020-00480-6
Monma Y., Shindo T., Eguchi K., Kurosawa R., Kagaya Y., Ikumi Y., et al. (2020). Low-intensity Pulsed Ultrasound Ameliorates Cardiac Diastolic Dysfunction in Mice: a Possible Novel Therapy for Heart Failure with Preserved Left Ventricular Ejection Fraction. Cardiovasc. Res. 117, 1325–1338. doi:10.1093/cvr/cvaa221
Monma Y., Shindo T., Eguchi K., Kurosawa R., Kagaya Y., Ikumi Y., et al. (2021). Low-intensity Pulsed Ultrasound Ameliorates Cardiac Diastolic Dysfunction in Mice: a Possible Novel Therapy for Heart Failure with Preserved Left Ventricular Ejection Fraction. Cardiovasc. Res. 117 (5), 1325–1338. doi:10.1093/cvr/cvaa221
Mühlfeld C., Rajces A., Manninger M., Alogna A., Wierich M. C., Scherr D., et al. (2020). A Transmural Gradient of Myocardial Remodeling in Early‐stage Heart Failure with Preserved Ejection Fraction in the Pig. J. Anat. 236 (3), 531–539. doi:10.1111/joa.13117
Noll N. A., Lal H., Merryman W. D. (2020). Mouse Models of Heart Failure with Preserved or Reduced Ejection Fraction. Am. J. Pathol. 190 (8), 1596–1608. doi:10.1016/j.ajpath.2020.04.006
Nouraei H., Rabkin S. W. (2021). A New Approach to the Clinical Subclassification of Heart Failure with Preserved Ejection Fraction. Int. J. Cardiol. 331, 138–143. doi:10.1016/j.ijcard.2021.01.052
Pacher P., Nagayama T., Mukhopadhyay P., Bátkai S., Kass D. A. (2008). Measurement of Cardiac Function Using Pressure-Volume Conductance Catheter Technique in Mice and Rats. Nat. Protoc. 3 (9), 1422–1434. doi:10.1038/nprot.2008.138
Pan G., Munukutla S., Kar A., Gardinier J., Thandavarayan R. A., Palaniyandi S. S. (2018). Type-2 Diabetic Aldehyde Dehydrogenase 2 Mutant Mice (ALDH 2*2) Exhibiting Heart Failure with Preserved Ejection Fraction Phenotype Can Be Determined by Exercise Stress Echocardiography. PLoS One 13 (4), e0195796. doi:10.1371/journal.pone.0195796
Pan X., Shao Y., Wu F., Wang Y., Xiong R., Zheng J., et al. (2018). FGF21 Prevents Angiotensin II-Induced Hypertension and Vascular Dysfunction by Activation of ACE2/Angiotensin-(1-7) Axis in Mice. Cell Metab. 27 (6), 1323–1337. e1325. doi:10.1016/j.cmet.2018.04.002
Park S.-H., Farooq M. A., Gaertner S., Bruckert C., Qureshi A. W., Lee H.-H., et al. (2020). Empagliflozin Improved Systolic Blood Pressure, Endothelial Dysfunction and Heart Remodeling in the Metabolic Syndrome ZSF1 Rat. Cardiovasc. Diabetol. 19 (1), 19. doi:10.1186/s12933-020-00997-7
Peng H., Yang X.-P., Carretero O. A., Nakagawa P., D’Ambrosio M., Leung P., et al. (2011). Angiotensin II-Induced Dilated Cardiomyopathy in Balb/c but Not C57BL/6J Mice. Exp. Physiol. 96 (8), 756–764. doi:10.1113/expphysiol.2011.057612
Petrosino J. M., Heiss V. J., Maurya S. K., Kalyanasundaram A., Periasamy M., LaFountain R. A., et al. (2016). Graded Maximal Exercise Testing to Assess Mouse Cardio-Metabolic Phenotypes. PLoS One 11 (2), e0148010. doi:10.1371/journal.pone.0148010
Plante E., Menaouar A., Danalache B. A., Yip D., Broderick T. L., Chiasson J.-L., et al. (2015). Oxytocin Treatment Prevents the Cardiomyopathy Observed in Obese Diabetic Male Db/db Mice. Endocrinology 156 (4), 1416–1428. doi:10.1210/en.2014-1718
Reddy Y. N. V., Carter R. E., Obokata M., Redfield M. M., Borlaug B. A. (2018). A Simple, Evidence-Based Approach to Help Guide Diagnosis of Heart Failure with Preserved Ejection Fraction. Circulation 138 (9), 861–870. doi:10.1161/CIRCULATIONAHA.118.034646
Reiter U., Reiter G., Manninger M., Adelsmayr G., Schipke J., Alogna A., et al. (2016). Early-stage Heart Failure with Preserved Ejection Fraction in the Pig: a Cardiovascular Magnetic Resonance Study. J. Cardiovasc. Magn. Reson. 18 (1), 63. doi:10.1186/s12968-016-0283-9
Rockman H. A., Ono S., Ross R. S., Jones L. R., Karimi M., Bhargava V., et al. (1994). Molecular and Physiological Alterations in Murine Ventricular Dysfunction. Proc. Natl. Acad. Sci. U.S.A. 91 (7), 2694–2698. doi:10.1073/pnas.91.7.2694
Rockman H. A., Ross R. S., Harris A. N., Knowlton K. U., Steinhelper M. E., Field L. J., et al. (1991). Segregation of Atrial-specific and Inducible Expression of an Atrial Natriuretic Factor Transgene in an In Vivo Murine Model of Cardiac Hypertrophy. Proc. Natl. Acad. Sci. U.S.A. 88 (18), 8277–8281. doi:10.1073/pnas.88.18.8277
Roh J. D., Houstis N., Yu A., Chang B., Yeri A., Li H., et al. (2020). Exercise Training Reverses Cardiac Aging Phenotypes Associated with Heart Failure with Preserved Ejection Fraction in Male Mice. Aging Cell 19 (6), e13159. doi:10.1111/acel.13159
Runte K. E., Bell S. P., Selby D. E., Häußler T. N., Ashikaga T., LeWinter M. M., et al. (2017). Relaxation and the Role of Calcium in Isolated Contracting Myocardium from Patients with Hypertensive Heart Disease and Heart Failure with Preserved Ejection Fraction. Circ. Heart Fail. 10 (8). doi:10.1161/circheartfailure.117.004311
Satoh T., Wang L., Espinosa-Diez C., Wang B., Hahn S. A., Noda K., et al. (2021). Metabolic Syndrome Mediates ROS-miR-193b-NFYA-dependent Downregulation of Soluble Guanylate Cyclase and Contributes to Exercise-Induced Pulmonary Hypertension in Heart Failure with Preserved Ejection Fraction. Circulation 144, 615–637. doi:10.1161/CIRCULATIONAHA.121.053889
Sawall S., Franke D., Kirchherr A., Beckendorf J., Kuntz J., Maier J., et al. (2017). In Vivo Quantification of Myocardial Infarction in Mice Using Micro-CT and a Novel Blood Pool Agent. Contrast Media Mol. Imaging 2017, 1–7. doi:10.1155/2017/2617047
Schauer A., Adams V., Augstein A., Jannasch A., Draskowski R., Kirchhoff V., et al. (2021). Sacubitril/Valsartan Improves Diastolic Function but Not Skeletal Muscle Function in a Rat Model of HFpEF. Ijms 22 (7), 3570. doi:10.3390/ijms22073570
Schauer A., Draskowski R., Jannasch A., Kirchhoff V., Goto K., Männel A., et al. (2020). ZSF1 Rat as Animal Model for HFpEF: Development of Reduced Diastolic Function and Skeletal Muscle Dysfunction. ESC Heart Fail. 7 (5), 2123–2134. doi:10.1002/ehf2.12915
Schiattarella G. G., Altamirano F., Tong D., French K. M., Villalobos E., Kim S. Y., et al. (2019). Nitrosative Stress Drives Heart Failure with Preserved Ejection Fraction. Nature 568 (7752), 351–356. doi:10.1038/s41586-019-1100-z
Schneider J. W., Oommen S., Qureshi M. Y., Goetsch S. C., Pease D. R., Sundsbak R. S., et al. (2020). Dysregulated Ribonucleoprotein Granules Promote Cardiomyopathy in RBM20 Gene-Edited Pigs. Nat. Med. 26 (11), 1788–1800. doi:10.1038/s41591-020-1087-x
Schnelle M., Catibog N., Zhang M., Nabeebaccus A. A., Anderson G., Richards D. A., et al. (2018). Echocardiographic Evaluation of Diastolic Function in Mouse Models of Heart Disease. J. Mol. Cell Cardiol. 114, 20–28. doi:10.1016/j.yjmcc.2017.10.006
Shah S. J., Lam C. S. P., Svedlund S., Saraste A., Hage C., Tan R.-S., et al. (2018). Prevalence and Correlates of Coronary Microvascular Dysfunction in Heart Failure with Preserved Ejection Fraction: PROMIS-HFpEF. Eur. Heart J. 39 (37), 3439–3450. doi:10.1093/eurheartj/ehy531
Shen E., Li Y., Li Y., Shan L., Zhu H., Feng Q., et al. (2009). Rac1 Is Required for Cardiomyocyte Apoptosis during Hyperglycemia. Diabetes 58 (10), 2386–2395. doi:10.2337/db08-0617
Silverman D. N., Rambod M., Lustgarten D. L., Lobel R., LeWinter M. M., Meyer M. (2020). Heart Rate-Induced Myocardial Ca 2+ Retention and Left Ventricular Volume Loss in Patients with Heart Failure with Preserved Ejection Fraction. Jaha 9 (17), e017215. doi:10.1161/jaha.120.017215
Sorop O., Heinonen I., van Kranenburg M., van de Wouw J., de Beer V. J., Nguyen I. T. N., et al. (2018). Multiple Common Comorbidities Produce Left Ventricular Diastolic Dysfunction Associated with Coronary Microvascular Dysfunction, Oxidative Stress, and Myocardial Stiffening. Cardiovasc. Res. 114 (7), 954–964. doi:10.1093/cvr/cvy038
Stauch B., Johansson L. C., McCorvy J. D., Patel N., Han G. W., Huang X.-P., et al. (2019). Structural Basis of Ligand Recognition at the Human MT1 Melatonin Receptor. Nature 569 (7755), 284–288. doi:10.1038/s41586-019-1141-3
Tabucanon T., Wilcox J., Tang W. H. W. (2020). Does Weight Loss Improve Clinical Outcomes in Overweight and Obese Patients with Heart Failure? Curr. Diab Rep. 20 (12), 75. doi:10.1007/s11892-020-01367-z
Tarkia M., Stark C., Haavisto M., Kentala R., Vähäsilta T., Savunen T., et al. (2015). Cardiac Remodeling in a New Pig Model of Chronic Heart Failure: Assessment of Left Ventricular Functional, Metabolic, and Structural Changes Using PET, CT, and Echocardiography. J. Nucl. Cardiol. 22 (4), 655–665. doi:10.1007/s12350-015-0068-9
Tsukamoto Y., Mano T., Sakata Y., Ohtani T., Takeda Y., Tamaki S., et al. (2013). A Novel Heart Failure Mice Model of Hypertensive Heart Disease by Angiotensin II Infusion, Nephrectomy, and Salt Loading. Am. J. Physiology-Heart Circulatory Physiol. 305 (11), H1658–H1667. doi:10.1152/ajpheart.00349.2013
Valero-Muñoz M., Backman W., Sam F. (2017). Murine Models of Heart Failure with Preserved Ejection Fraction. JACC: Basic Translational Sci. 2 (6), 770–789. doi:10.1016/j.jacbts.2017.07.013
Valero-Muñoz M., Li S., Wilson R. M., Hulsmans M., Aprahamian T., Fuster J. J., et al. (2016). Heart Failure with Preserved Ejection Fraction Induces Beiging in Adipose Tissue. Circ. Heart Fail. 9 (1), e002724. doi:10.1161/CIRCHEARTFAILURE.115.002724
Wang C., Fan F., Cao Q., Shen C., Zhu H., Wang P., et al. (2016). Mitochondrial Aldehyde Dehydrogenase 2 Deficiency Aggravates Energy Metabolism Disturbance and Diastolic Dysfunction in Diabetic Mice. J. Mol. Med. 94 (11), 1229–1240. doi:10.1007/s00109-016-1449-5
Wang L. W., Kesteven S. H., Huttner I. G., Feneley M. P., Fatkin D. (2018). High-Frequency Echocardiography ― Transformative Clinical and Research Applications in Humans, Mice, and Zebrafish ―. Circ. J. 82 (3), 620–628. doi:10.1253/circj.CJ-18-0027
Wang Q., Chen K., Lin H., He M., Huang X., Zhu H., et al. (2019). Induction of Right Ventricular Failure by Pulmonary Artery Constriction and Evaluation of Right Ventricular Function in Mice. JoVE 147. doi:10.3791/59431
Warren D. J. (1974). A Thermistor Probe for Measurement of Blood Temperature and Cardiac Output in Small Animals. Cardiovasc. Res. 8 (4), 566–569. doi:10.1093/cvr/8.4.566
Withaar C., Lam C. S. P., Schiattarella G. G., de Boer R. A., Meems L. M. G. (2021b). Heart Failure with Preserved Ejection Fraction in Humans and Mice: Embracing Clinical Complexity in Mouse Models. Eur. Heart J. 42 (43), 4420–4430. doi:10.1093/eurheartj/ehab389
Withaar C., Meems L. M. G., de Boer R. A. (2021a). Fighting HFpEF in Women: Taking Aim at Belly Fat. Eur. Heart J. 42 (16), 1606–1608. doi:10.1093/eurheartj/ehaa952
Withaar C., Meems L. M. G., Markousis-Mavrogenis G., Boogerd C. J., Silljé H. H. W., Schouten E. M., et al. (2020). The Effects of Liraglutide and Dapagliflozin on Cardiac Function and Structure in a Multi-Hit Mouse Model of Heart Failure with Preserved Ejection Fraction. Cardiovasc. Res. 117, 2108–2124. doi:10.1093/cvr/cvaa256
Witte K. K., Clark A. L. (2007). Why Does Chronic Heart Failure Cause Breathlessness and Fatigue? Prog. Cardiovasc. Dis. 49 (5), 366–384. doi:10.1016/j.pcad.2006.10.003
Xia C.-L., Chu P., Liu Y.-X., Qu X.-L., Gao X.-F., Wang Z.-M., et al. (2020). ALDH2 Rs671 Polymorphism and the Risk of Heart Failure with Preserved Ejection Fraction (HFpEF) in Patients with Cardiovascular Diseases. J. Hum. Hypertens. 34 (1), 16–23. doi:10.1038/s41371-019-0182-2
Yang H. J., Kong B., Shuai W., Zhang J. j., Huang H. (2020). MD1 Deletion Exaggerates Cardiomyocyte Autophagy Induced by Heart Failure with Preserved Ejection Fraction through ROS/MAPK Signalling Pathway. J. Cell Mol Med 24 (16), 9300–9312. doi:10.1111/jcmm.15579
Yin Z., Zhang W., Zhao D., Sulejmani F., Feng Y., Huo Y., et al. (2020). Cardiac wall Mechanics Analysis in Hypertension-Induced Heart Failure Rats with Preserved Ejection Fraction. J. Biomech. 98, 109428. doi:10.1016/j.jbiomech.2019.109428
Yousefi K., Irion C. I., Takeuchi L. M., Ding W., Lambert G., Eisenberg T., et al. (2019). Osteopontin Promotes Left Ventricular Diastolic Dysfunction through a Mitochondrial Pathway. J. Am. Coll. Cardiol. 73 (21), 2705–2718. doi:10.1016/j.jacc.2019.02.074
Zeiss C. J., Gatti D. M., Toro-Salazar O., Davis C., Lutz C. M., Spinale F., Stearns T., Furtado M. B., Churchill G. A. (2019). Doxorubicin-Induced Cardiotoxicity in Collaborative Cross (CC) Mice Recapitulates Individual Cardiotoxicity in Humans. G3 (Bethesda) 9 (8), 2637–2646. doi:10.1534/g3.119.400232
Zhang J. X., Liu Y. X., Xia C. L., Chu P., Qu X. L., Zhu L. L., et al. (2019). Risks of Incident Heart Failure with Preserved Ejection Fraction in Chinese Patients Hospitalized for Cardiovascular Diseases. J. Geriatr. Cardiol. 16 (12), 885–893. doi:10.11909/j.issn.1671-5411.2019.12.005
Zhang L., Chen J., Yan L., He Q., Xie H., Chen M. (2021). Resveratrol Ameliorates Cardiac Remodeling in a Murine Model of Heart Failure with Preserved Ejection Fraction. Front. Pharmacol. 12, 646240. doi:10.3389/fphar.2021.646240
Zhang N., Feng B., Ma X., Sun K., Xu G., Zhou Y. (2019). Dapagliflozin Improves Left Ventricular Remodeling and Aorta Sympathetic Tone in a Pig Model of Heart Failure with Preserved Ejection Fraction. Cardiovasc. Diabetol. 18 (1), 107. doi:10.1186/s12933-019-0914-1
Keywords: heart failure with preserved ejection fraction, metabolic disturbance, animal models, ventricular diastolic dysfunction, clinical translation
Citation: Li H, Xia Y-Y, Xia C-L, Li Z, Shi Y, Li X-B and Zhang J-X (2022) Mimicking Metabolic Disturbance in Establishing Animal Models of Heart Failure With Preserved Ejection Fraction. Front. Physiol. 13:879214. doi: 10.3389/fphys.2022.879214
Received: 19 February 2022; Accepted: 30 March 2022;
Published: 03 May 2022.
Edited by:
Arpana Vibhuti, SRM University (Delhi-NCR), IndiaReviewed by:
Min Zhang, King’s College London, United KingdomSandeep Kumar Shukla, Institute of Nuclear Medicine & Allied Sciences (DRDO), India
Copyright © 2022 Li, Xia, Xia, Li, Shi, Li and Zhang. This is an open-access article distributed under the terms of the Creative Commons Attribution License (CC BY). The use, distribution or reproduction in other forums is permitted, provided the original author(s) and the copyright owner(s) are credited and that the original publication in this journal is cited, in accordance with accepted academic practice. No use, distribution or reproduction is permitted which does not comply with these terms.
*Correspondence: Xiao-Bo Li, bGliZXIwOTA4QDE2My5jb20=; Jun-Xia Zhang, emhhbmdqdW5zaGlhQG5qbXUuZWR1LmNu
†These authors have contributed equally to this work and share first authorship