- 1Department of Gastroenterology, Digestive Disease Hospital, Affiliated Hospital of Zunyi Medical University, Zunyi, China
- 2Collaborative Innovation Center of Tissue Damage Repair and Regeneration Medicine, Zunyi, China
Hypoxia refers to a state of oxygen limitation, which mainly mediates pathological processes in the human body and participates in the regulation of normal physiological processes. In the hypoxic environment, the main regulator of human body homeostasis is the hypoxia-inducible factor family (HIF). HIF can regulate the expression of many hypoxia-induced genes and then participate in various physiological and pathological processes of the human body. Ion-transporting proteins are extremely important types of proteins. Ion-transporting proteins are distributed on cell membranes or organelles and strictly control the inflow or outflow of ions in cells or organelles. Changes in ions in cells are often closely related to extensive physiological and pathological processes in the human body. Numerous studies have confirmed that hypoxia and its regulatory factors can regulate the transcription and expression of ion-transporting protein-related genes. Under hypoxic stress, the regulation and interaction of ion-transporting proteins by hypoxia often leads to diseases of various human systems and even tumors. Using ion-transporting proteins and hypoxia as targets to explore the mechanism of digestive system diseases and targeted therapy is expected to become a new breakthrough point.
1 Introduction
Oxygen is vital to the human body. The human body allows oxygen to enter the body through lung ventilation, and the oxygen is exchanged in the alveoli and delivered to the capillaries through the alveolar-capillary exchange system. In capillaries, oxygen combines with hemoglobin to enter cells of various tissues, and finally participates in energy production in mitochondria in cells to meet the metabolic needs of cells (Ortiz-Prado et al., 2019). However, each organ in the human body has different metabolic levels and functional states, so under physiological conditions, most organs are in their unique physiological normoxic state (Nakayama and Kataoka, 2019). For example, the partial pressure of oxygen (PO2) in the brain is 30–48 mmHg, and the PO2 in the superficial epidermis of the kidney is 72 ± 20 mmHg. The PO2 in the liver is 55.5 ± 21.3 mmHg, the PO2 around the portal vein is about 60–65 mmHg, while in the perivenous area it drops to about 30–35 mmHg (Kietzmann, 2019). There is a unique oxygen gradient in the human gut. The partial pressure of oxygen in the colon wall is 42–71 mmHg, the PO2 near the recess-cavity interface is 5–10 mmHg, and the PO2 in the ascending colon cavity and sigmoid colon cavity are 11 and 3 mmHg, respectively. The PO2 of the small intestinal wall is 59 mmHg, the tip of the villi is about 22 mmHg, and the PO2 of the small intestinal lumen is < 10 mmHg (Singhal and Shah, 2020). The PO2 of the pancreas is about 40 mmHg (Lo et al., 2013; Ortiz-Prado et al., 2019). However, in the event of conditions including respiratory failure, insufficient blood flow to end organs, dysfunctional or low levels of hemoglobin, or chemically induced hypoxia, the partial pressure of oxygen will fall below physiological normoxic levels. The oxygen will be limited, and the cells will be in a hypoxic environment. A hypoxic microenvironment can trigger changes in cellular metabolism and trigger different molecular responses. The hypoxia response can be part of the normal physiological activities of the human body, but it mainly mediates the pathological process and promotes the progression of the disease.
1.1 Hypoxia and its Regulating Factors
The main regulator of human tissue homeostasis under hypoxia is the hypoxia-inducible factor (HIF) family. Hypoxia-inducible factor was originally discovered as an enhancer of the erythropoietin (EPO) gene in the process of identifying hypoxia response elements (HREs) (Ke and Costa, 2006). The HIF family has three main members, namely, HIF-1, HIF-2, and HIF-3. The functional subunit forms of these three members are HIF-1α, HIF-2α, and HIF-3α. Currently, the effect of HIF-3α is unclear. Studies have only found that HIF-3α can inhibit the transcription of HIF-1/2α and act as a dominant negative regulator of HIF-1/2α activity. HIF-2α has a similar structure to HIF-1α, but its expression pattern is different because in contrast to widespread HIF-1α, HIF-2α is expressed only in certain tissues. Therefore, HIF-1α is most widely known (Loboda et al., 2010; Duan, 2016). The stability and activity of HIF-1α are regulated by posttranslational modification, hydroxylation, acetylation, and phosphorylation. Hypoxia-inducible factor-1α can interact with various protein factors, including PHD, pVHL, ARD-1, p300/CBP, RBX1, Elongin B, and Elongin C. Under normoxic conditions, the hypoxia-inducible factor-1α subunit can be triggered by the hydroxylation of proline and the acetylation of lysine in a polypeptide segment called the oxygen-dependent degradation (ODD) domain, and combined with von Hippel-Lindau tumor suppressor gene product (pVHL), pVHL mediates the ubiquitin–proteasome composed of RBX1, Elongin B, Elongin C, and VHL and rapidly degrades HIF through the ubiquitin–proteasome pathway. In contrast, under hypoxic conditions, the hypoxia-inducible factor-1α subunit becomes stable and interacts with coactivators, such as p300/CBP. Thus, the transcriptional activity of hypoxia-inducible factor-1α is enhanced, and under hypoxic conditions, it becomes the main regulator of many hypoxia-inducible genes (Lee et al., 2004). These genes are involved in cell survival, proliferation, movement, metabolism, pH regulation, extracellular matrix function, inflammatory cell recruitment, and angiogenesis (Joseph et al., 2018). HIF-1 regulates these genes and leads to various pathological processes (Figure 1). In conclusion, HIF is an important factor regulating the oxygen balance in the human body and an important target of hypoxia-mediated activities (Li et al., 2020).
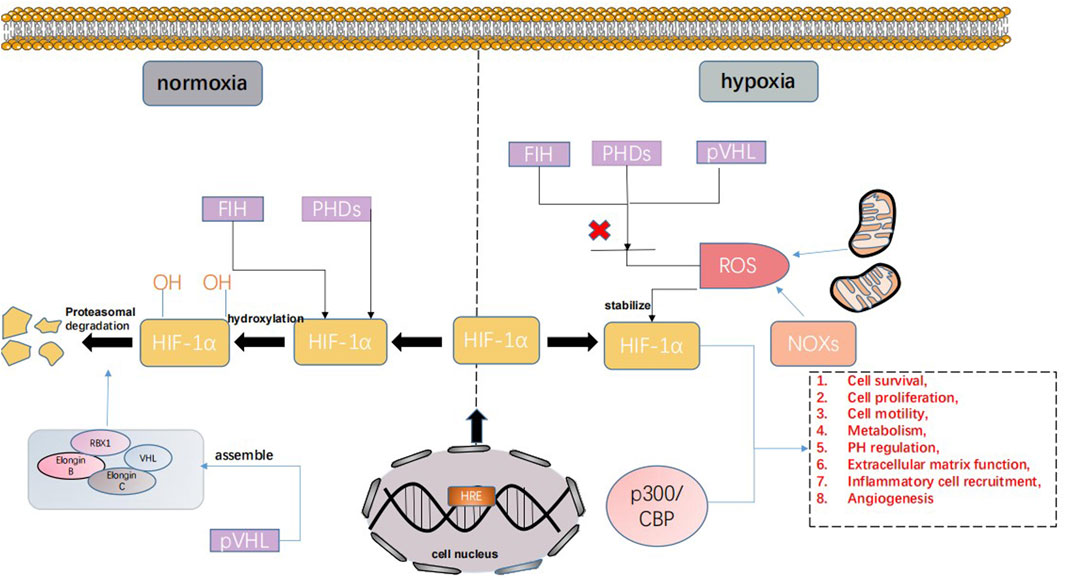
FIGURE 1. HIF can be expressed in different ways under normoxia and hypoxia. Under normoxic conditions, the hypoxia-inducible factor-1α subunit can bind the von Hippel-Lindau tumor suppressor gene product (PVHL). pVHL mediates the ubiquitin–proteasome, which is composed of RBX1, Elongin B, Elongin C, and VHL. The ubiquitin–proteasome pathway rapidly degrades HIF. In contrast, under hypoxic conditions, the hypoxia-inducible factor-1α subunit becomes stable and interacts with coactivators, such as p300/CBP, and the transcriptional activity of hypoxia-inducible factor-1α is enhanced. Under hypoxic conditions, it has become the main regulator of many hypoxia-induced genes involved in cell survival, proliferation, exercise, metabolism, pH regulation, extracellular matrix function, inflammatory cell recruitment, angiogenesis, etc.
1.2 The Role of Hypoxia and its Regulating Factors in the Physiological and Pathological Processes of the Digestive System
The impact of hypoxia and its regulatory factors on the human body has always been a hot topic and studies have increasingly shown that hypoxia can play an important role in the human body (Fuentes and Christianson, 2016). In the past, a large number of studies on the effects of hypoxia on the human body tended to focus on the cardiovascular, respiratory, urinary, and nervous systems, such as myocardial apoptosis and hypertrophy (Eigel et al., 2004; Chu et al., 2012), pulmonary artery contraction and remodeling (Dong et al., 2012; Simonneau et al., 2014; Parpaite et al., 2016), nerve cell apoptosis (Aarts et al., 2003), and renal ischemia reperfusion (Kita et al., 2007; Liu et al., 2018). However, the effects of hypoxia on the human body are not limited to the cardiovascular, respiratory, nervous, urinary, and other systems. In recent years, the effects of hypoxia on the digestive system have also received increasing attention. Increasing evidence shows that hypoxia and its regulatory factors can also be widely expressed in various organs of the digestive system and play an extremely important role in the physiological and pathological processes of the digestive system.
1.2.1 The Physiological Role of Hypoxia in the Digestive System
Hypoxia can be used as a physiological signal that can affect many physiological processes in the digestive system (Hubbi and Semenza, 2015). For example, as an important digestive organ, the liver performs various functions necessary for maintaining systemic homeostasis. The liver is the central metabolic organ responsible for maintaining blood glucose levels, ammonia metabolism, endogenous metabolic by-products of biotransformation and metabolism of xenobiotics, and bile synthesis. All of these processes require many pathways and enzymatic reactions to run in parallel in the most efficient manner. To achieve this, the liver parenchyma exhibits a functional organization called metabolic zonation. It is currently recognized that both Wnt/β-catenin pathway and Hedgehog (Hh) signaling play a decisive role in metabolic partitioning (Benhamouche et al., 2006; Matz-Soja et al., 2013). In hypoxic environment, hif-1a and HIF-β promote the expression of Wnt/β-catenin target genes including Dkk-4, Lef-1, and Tcf-1. And HIF-1α can directly bind to the promoters of Lef1 and TCF1 genes, thereby greatly enhancing the transcriptional activity of β-catenin (Mazumdar et al., 2010). At the same time, hypoxia can induce the expression of Hh ligand SHh and the pathway activity marker Patched1, thereby inducing a systemic Hh response (Bijlsma et al., 2009). Through these two pathways, hypoxia and its regulators promote the formation of hepatic metabolic zonation (Kietzmann, 2017). Induced hepatic stem cells (iHepSCs) are lineage-reprogrammed cells derived from murine embryonic fibroblasts with self-renewal and bipotential differentiation properties, which hold great potential as hepatocyte therapy donors. Physiological hypoxia can accelerate the G1/S transition through the p53-p21 signaling pathway, thereby enhancing stemness characteristics and promoting the proliferative capacity of iHepSCs. In addition, short-term hypoxia preconditioning enhances the hepatic differentiation efficiency of iHepSCs, and long-term hypoxia promotes cholangiocyte differentiation but inhibits hepatic differentiation of iHepSCs (Zhi et al., 2018). Studies have shown that physiological hypoxia can assist the formation of the barrier function of the gastrointestinal tract. Tight junctions are the backbone that form the upper intestinal barrier, and claudins are integral membrane proteins in the intestinal barrier responsible for the selective permeability of tight junctions (Ivanov et al., 2010). The hypoxic intestinal environment induces the production of HIF-1β, which maintains CLDN1 expression by binding to the hypoxia-responsive element sequence in the gene promoter (Saeedi et al., 2015). Xenobiotic clearance is an important function of the intestinal epithelial barrier. P-glycoprotein, also known as multidrug resistance protein 1, has broad substrate specificity. In the process of xenobiotic clearance, P-glycoprotein is the main effector of xenobiotic transport into the lumen. P-glycoprotein is transcriptionally regulated by HIF-1 (Comerford et al., 2002; Zheng et al., 2015).
1.2.2 The Pathological Role of Hypoxia in the Digestive System
As a common environmental stress factor, hypoxia is more involved in the pathological process of the digestive system. First, in the liver, hypoxia-induced overexpression of HIF-2α can inhibit fatty acid β-oxidation, thereby activating peroxisome proliferators to activate the receptor PPARα, which, in turn, induces fat formation in the liver and exacerbates nonalcoholic fatty liver disease (NAFLD) (J. Chen et al., 2019). Hypoxia is a common phenomenon in hepatocellular carcinoma (HCC). Hypoxia stabilizes the transcription factor hypoxia-inducible factor (HIF), and the expression of HIF is closely related to the metastasis of liver cancer. The metastasis of liver cancer requires the support of metastasis-promoting genes, and hypoxia/hypoxia-inducible factor-1α has been shown to be a central regulator of many metastasis-promoting genes that can activate metastasis-promoting genes; thus, it ultimately participates in every step of liver cancer metastasis (Wong et al., 2014). In the pancreas, pancreatic ductal adenocarcinoma (PDAC) is characterized by fewer blood vessels, strong invasiveness, and a very poor prognosis. PDAC has strong invasion and migration ability because under hypoxic conditions, pancreatic cancer-associated fibroblasts (CAFs) are stimulated by hypoxia to produce insulin-like growth Factor 1 (IGF1), and IGF1/IGF1R signaling can stimulate the invasion and migration activity of PDAC cells (Hirakawa et al., 2016). In the esophagus, hypoxia can promote the growth and metastasis of esophageal squamous cell carcinoma (ESCC). Under hypoxic conditions, hypoxia stimulates the production of HIF-1α, and HIF-1α can induce the expression of microRNA (miRNA), a posttranscriptional regulatory factor. MicroRNAs can stimulate the growth and metastasis of esophageal squamous cell carcinoma (ESCC) (Zhang et al., 2019). In the stomach, a key factor underlying gastric cancer invasion and metastasis is the epithelial-mesenchymal transition (EMT). Hypoxia-inducible factor-1α not only is an independent prognostic factor of gastric cancer but also, under hypoxic conditions, can stimulate the overexpression of prostate cancer gene expression marker 1 (PCGEM1). The overexpression of prostate cancer gene expression marker 1 (PCGEM1) can affect the epithelial-mesenchymal transition (EMT), thereby promoting the invasion and metastasis of gastric cancer cells (Zhang J et al., 2019). In the intestine, the severity of inflammatory bowel disease was found to be positively correlated with the expression of HIF-1a (Cummins and Crean, 2017). In colorectal cancer (CRC), the Hippo signaling pathway is a central pathway that regulates intestinal growth. Related protein 1 (YAP1) is a downstream effector of the Hippo signaling pathway. YAP1 is an important regulator of proliferation, organ size, and cell differentiation. HIF-2α can directly increase the activity of cancer cells by upregulating YAP1 activity to promote the growth of colorectal cancer cells (Ma et al., 2017).
Overall, numerous studies have shown that hypoxia can play an important role in the physiological processes and diseases of the digestive system. However, to date, how hypoxia specifically affects the digestive system, especially in some diseases and tumors, its mechanism, and its function have not been fully elucidated. A deeper understanding of the mechanism of hypoxia and its regulatory factors in the digestive system could help us further understand the development, changes, and prognosis of the disease and discover new drug treatment targets.
1.3 Correlation Between Ion-Transporting Proteins and Hypoxia
In recent years, through the exploration of the mechanism of hypoxia and its regulatory factors, it has been discovered that hypoxia can affect various human systems by regulating the expression of ion-transporting proteins and interacting with various ion-transporting proteins. First, for human cells, ion-transporting proteins constitute an extremely important class of proteins that are distributed on the cell membrane and form pores in the cell membrane, strictly controlling the inflow or outflow of ions in the cell or organelle (Keynes, 1975). Ions are important cell signals in the human body. Changes in ions in cells are often closely related to extensive physiological and pathological processes in the human body. In addition, there is amazing molecular diversity in ion-transporting proteins. Ion transporters comprise a wide range of ion channels, exchangers, pumps, and ionotropic receptors. They are based on ion selectivity (sodium channel, potassium channel, chloride channel, calcium channel, proton channel, and nonselective channel), gating mechanism (voltage gating, ligand gating, cyclic nucleotide gating, light gate, and mechanically sensitive), or localization (plasma membrane and intracellular) for classification (Venter et al., 2001), Kv, Kca, Ki, NCX, and TRP channel families (Venkatachalam and Montell, 2007), STIM, NHE, etc. Because of their diversity, limited tissue distribution, and important role in regulating key cell functions, they are very attractive therapeutic or diagnostic targets in many diseases (Kaczorowski et al., 2008).
However, numerous studies have proven that under normal circumstances, hypoxia and its regulatory factor HIF-1 can regulate the transcription and expression of ion-transporting protein-related genes such that the related ion-transporting proteins are overexpressed or inhibited. Sometimes, activated ion-transporting proteins will in turn affect the expression of HIF-1, causing it to activate or silence. When the various systems of the human body are under hypoxic stress, the regulation and interaction of hypoxia on ion-transporting proteins often leads to the occurrence of related diseases and even tumors. This article aims to review the effects of ion-transporting proteins on the digestive system under hypoxia to provide new ideas for clinical treatment (Figure 2).
2 The Role of Ion-Transporting Proteins in the Digestive System Under Hypoxia
2.1 The Effect of Vacuolar H+-ATPase on Energy Metabolism of Esophageal Carcinoma Cells Under Hypoxia
2.1.1 Physiological Role of V-ATPase and Energy Metabolism of Esophageal Cells
The vacuolar (H+)-ATPase (V-ATPase) is an ATP-dependent proton pump. V-ATPase is composed of V0 and V1 domains. The V0 domain consists of five distinct subunits involved in proton transport (Forgac, 2007). On the other hand, the V1 domain consists of 8 different subunits responsible for the hydrolysis of ATP (Forgac, 2007). V-ATPase is mainly responsible for the maintenance of PH in human plasma and cells, the transport and secretion of intracellular and intracellular membranes, the processing and degradation of some macromolecules, and the coupled transport of some small molecules such as neurotransmitters and ATP (Cipriano et al., 2008). Like cells in other parts of the body, under normal circumstances, esophageal cells usually use glycolysis to convert glucose in the cytoplasm into pyruvate. Pyruvate is further metabolized in mitochondria through the tricarboxylic acid (TCA) cycle and the electron transport chain (ETC) through oxidative phosphorylation (OxPhos) to generate energy storage adenosine triphosphate (ATP) (Pelicano et al., 2006).
But we were surprised to find that V-ATPase plays a completely different role in esophageal cancer cells, and the energy metabolism of esophageal cancer cells is also completely different from the tricarboxylic acid cycle of normal cells.
2.1.2 V-ATPase can Positively Regulate HIF-1, Thereby Enhancing the Expression of Key Genes of Glycolysis and Promoting Glycolysis in Esophageal Cancer Cells
Esophageal cancer is one of the deadliest malignant tumors, and the prognosis is often poor (Nassri et al., 2018). Although there have been advances in chemotherapy and radiotherapy in recent years, due to its high mortality rate, the 5-year survival rate of patients with esophageal squamous cell carcinoma (ESCC) is very low (up to 30%–45%) (Russo and Franceschi, 1996). One of the main reasons is that, in contrast to normal cells, tumor cells heavily rely on glycolysis to produce energy, even with sufficient oxygen levels, which allows them to maintain a high proliferation rate and resist apoptosis signals (Liu et al., 2001).
Studies have shown that in the process of glycolysis, the key genes of glycolysis, glucose transporter 1 (Glut1), hexokinase II (HK2), and lactate dehydrogenase A (LDHA) activation are often dependent on HIF-1 (Nagao et al., 2019). In esophageal cancer cells, vacuolar H+-adenosyl triphosphate (V-ATPase) is highly expressed. V-ATPase is particularly important for maintaining the pH environment required for tumor growth. At the same time, V-ATPase can promote the expression of mammalian target of rapamycin (MTOR) (Buller et al., 2011). MTOR increases glucose uptake and expression of glucose transporter 1 (GLUT1). In addition, experiments showed that V-ATPase can also promote HIF-1 and other glycolytic genes such as HK2, phosphofructokinase-1 (PFK1), enolase 1 (ENO1), PKM2, LDHA, and pyruvate dehydrogenase thiamine kinase isoenzyme (PDK1) expression (Son et al., 2019). Therefore, in the process of glycolysis of esophageal cancer cells, V-ATPase itself can activate the transport of glucose and positively regulate HIF-1, so as to enhance the expression of key genes of glycolysis, promote glycolysis, and provide energy for the proliferation, growth, and metastasis of esophageal cancer cells. For the treatment of esophageal cancer, how to inhibit its glycolytic pathway seems to provide a new idea for the development of new targeted anticancer drugs, and HIF-1 and V-ATPase may also be potential targets for inhibiting glycolysis (Son et al., 2016).
2.2 The Regulatory Role of Ion Transporters in Gastric Diseases Under Hypoxia
2.2.1 Under Hypoxia, Vacuolar H+-ATPase can Promote the Multidrug Resistance of Gastric Cancer Cells
2.2.1.1 The Emergence of Multidrug Resistance in Gastric Cancer
Gastric cancer is the second leading cause of death worldwide (Ferro et al., 2014). Sixty-five percent of gastric cancer patients are often in the advanced or metastatic stage at the time of diagnosis (Hundahl et al., 2000). The combination of chemotherapy and targeted therapy provides hope of survival for patients with advanced gastric cancer. However, tumor cells sometimes develop resistance to multiple cytotoxic drugs that reduces the effectiveness of chemotherapy, known as multidrug resistance (Ozben, 2006). The main mechanisms of MDR production include reducing drug accumulation in tumor cells, altering intracellular drug distribution, increasing detoxification, reducing drug-target interactions, increasing DNA repair, altering cell cycle regulation, and uncoupling pathways that link cell damage to apoptosis (Martínez-Lacaci et al., 2007; Gillet and Gottesman, 2010). The most dominant form of resistance to chemotherapy has been correlated with two MDR transporters including P-glycoprotein (P-gp), multidrug resistant protein1 (MRP1) (Szakács et al., 2006). They can often be overexpressed in malignant cells and can pump a variety of anticancer drugs out of the cell, resulting in lower levels of the intracellular drugs needed for effective treatment (Rees et al., 2009). Therefore, it is necessary to explore the regulatory pathways of these transporters.
2.2.1.2 Vacuolar H+-ATPase Increases HIF-1α Translation to Promote P-Gp and MRP1 Expression
As we described above, the main physiological role of V-ATPases in the human body is to maintain the homeostasis of cellular pH. But in gastric cancer cells, we surprisingly found that the activity of V-ATPase was greatly increased. Activated V-ATPase can promote the expression of mTOR. mTOR, a master regulator of protein synthesis and cell growth, increases the translation of hypoxia-inducible factor 1α (HIF-1α) (Advani, 2010). In the hypoxic environment formed by gastric cancer cells, hypoxia-inducible factor-1α (HIF-1α) can also regulate the transcription of a large number of hypoxia-related genes including multidrug resistance genes (Semenza, 2002; Lin et al., 2014). Among them, the expression of HIF-1α can induce a significant increase in the translation of P-gp and MRP1 (L. Liu et al., 2008), thereby reducing intracellular drug accumulation and enhancing the drug resistance of esophageal cancer cells. Therefore, both V-ATPase and HIF-1α provide effective therapeutic targets, and reducing the expression of V-ATPase and HIF-1α can increase the effectiveness of chemotherapeutic drugs and even reverse the drug resistance of gastric cancer cells (Chen et al., 2012).
2.2.2 Mitochondrial Calcium Uniporter Regulates Migration, Invasion, Angiogenesis, and Growth of Gastric Cancer Cells Under Hypoxic Conditions
The mitochondrial calcium uniporter (MCU) is a highly selective calcium channel and the primary pathway for calcium entry into mitochondria. In the hypoxic microenvironment of gastric cancer tissue, MCU is highly expressed. The overexpressed MCU can significantly increase the mitochondrial membrane potential level, and after the mitochondrial membrane potential level is increased, the invasive ability of gastric cancer cells is significantly improved. MCU may promote the proliferation of gastric cancer cells by increasing the level of mitochondrial membrane potential. The extracellular matrix (ECM) plays an important role in the invasion and metastasis of gastric cancer. Overexpression of MCU can cause calcium disturbance in gastric cancer cells. Matrix metalloproteinases are zinc- and calcium-dependent proteases that degrade ECM components, promote angiogenesis, and regulate cell adhesion. MCU can regulate matrix metalloproteinases to disrupt ECM homeostasis and promote the invasion and metastasis of gastric cancer cells (Gan et al., 2018). MCU overexpression also significantly promotes the expression of HIF-1α and vimentin, and suppresses the expression of E-cadherin in gastric cancer cells, while HIF-1α can induce the proliferation, migration, and invasion of gastric cancer cells by promoting the expression of VEGF, suggesting that MCU may regulate the expression of VEGF through HIF-1α. Inhibition of E-cadherin promotes epithelial-mesenchymal transition (EMT). EMT is the process by which cells transition from a proliferative epithelial phenotype to a migratory and invasive mesenchymal phenotype. MCU can promote the migration and invasion of gastric cancer cells by regulating the EMT process in vitro and in vivo. Therefore, targeting MCU may inhibit the migration, invasion, angiogenesis, and growth of gastric cancer cells, providing a new idea for the treatment of gastric cancer (Wang et al., 2020).
2.3 The Regulatory Role of Ion Transporters in Intestinal Diseases Under Hypoxia
2.3.1 Hypoxia and K2P Channels are Involved in the Pathogenesis of Inflammatory Bowel Disease
2.3.1.1 K2P5.1 Channel is Up-Regulated in Inflammatory Bowel Disease
Studies have shown that dysregulation of transcription, translation, and posttranslational expression of K+ channels is related to the pathogenesis of immune and inflammatory diseases (Ohya et al., 2016). Among them, K2P5.1 channel may be involved in the pathogenesis of inflammatory bowel disease. The alkaline pH-activated K+ channel (K2P5.1) belongs to the two-pore domain K+ (K2P) channel superfamily. K2P5.1 is not only involved in the resting potential of human cells, but also has various physiological functions such as maintaining cell volume and regulating renal bicarbonate reabsorption (Cid et al., 2013; López-Cayuqueo et al., 2015; Williams et al., 2013). But of most interest is its role in autoimmune and inflammatory diseases such as inflammatory bowel disease. In the study of autoimmune disease models such as inflammatory bowel disease (IBD), it was found that the expression and activity of K2P5.1 were significantly upregulated in the CD4+ T cells of the model. However, the mechanism of K2P5.1 upregulation remains unclear (Nakakura et al., 2015).
2.3.1.2 Under Hypoxia, HIF can Promote the Occurrence of Inflammatory Bowel Disease by Activating K2P5.1
Hypoxia-inducible factor-1α is strongly expressed in T cells infiltrating the inflammatory mucosa in patients with IBD (Higashiyama et al., 2012). Hypoxia-inducible factor-1α plays an important role in the pathogenesis of inflammatory diseases by promoting the expression of inflammatory genes (Taylor and Colgan, 2017). Brazier et al. found that HIF-1 dimers can bind target HIF-1 response element (HRE) regions to activate target gene transcription (Brazier et al., 2005) and clone the common binding site of the K2P5.1 promoter and Ets (E-26)-like 1 (ELK-1), whose activity is sensitive to oxygen and is necessary for K2P5.1 transcription initiation. The expression and activity of K2P5.1 increase under hypoxia (1.5% O2), and treatment with the HIF inhibitor FM19G11 instead of the selective HIF-2 inhibitor has the opposite effect. This finding indicates that under hypoxia, HIF can promote the occurrence of IBD by activating K2P5.1 (Endo et al., 2019).
2.3.2 Under Hypoxia, Transient Receptor Potential Channel 5 (TRPC5) can Promote Colon Cancer Tumor Metastasis Through the HIF-1α-Twist Signaling Pathway
Overexpression of TRPC5 in colon cancer cells promotes cancer cell migration and proliferation by inducing epithelial-mesenchymal transition (EMT). TRPC5 belongs to the TRP (Transient receptor potential) superfamily and is one of the major Ca2+ regulatory channels in the intestine. Epithelial-mesenchymal transition (EMT) is closely related to the invasive metastasis of tumor cells (Thiery et al., 2009). Several studies have shown that the occurrence of EMT is associated with calcium influx in colon cancer cells. In colon cancer, overexpressed TRPC5 leads to massive Ca2+ influx, which reduces the expression of E-cadherin. On the other hand, the expression of interstitial biomarkers N- cadherin and vimentin is significantly increased. Loss of function of the adhesion junction protein E-cadherin is regarded as a major hallmark and fundamental event of EMT (Kalluri and Weinberg, 2009). Further studies have revealed the molecular mechanism of how TRPC5 affects E-cadherin of EMT through Ca2+ influx. The major transcription factors that bind to the E-cadherin promoter and directly repress its transcription are the ZeB, Snail, and Twist families (Lee and Nelson, 2012; Perez-Moreno et al., 2001). The transcription factor Twist is highly expressed in colonic cells. The expression of Twist is regulated by HIF-1α, which can regulate the expression of Twist by directly binding to the hypoxia-responsive element (HRE) in the proximal promoter of Twist in the hypoxic environment of colon cancer cells (Yang et al., 2008). At the same time, HIF-1α itself is also a calcium-sensing factor, and the overexpression of TRPC5 can promote the increased translation of HIF-1α. Therefore, overexpression of TRPC5 promotes the migration and proliferation of colon cancer cells through the TRPC5/HIF-1α/Twist signaling pathway, and TRPC5 and HIF-1α may become potential therapeutic targets for colon cancer (Chen et al., 2017).
2.3.3 Piezo1 and MCU are Involved in Colon Cancer Metastasis in Hypoxic Environment
Colon cancer is the leading cause of cancer-related deaths worldwide and, like other tumors, is characterized by migratory, invasive, and metastatic capabilities. Tumor cell mobility is affected by multiple signaling cascades, including multiple ion channels and transporters (Schwab and Stock, 2014). Piezo1, also known as FAM38A, is a member of the PIEZO family, which includes Piezo1 and Piezo2. Piezo1 protein is a component of mechanically activated cation channels. It directly senses mechanical forces and translates environmental signals into intracellular Ca responses, and is widely expressed in a variety of cells and tissues, including tumor cells (Miyamoto et al., 2014; Liu Q et al., 2018). MCU is an evolutionarily conserved Ca2+ channel that plays a role in intracellular Ca2+ signaling in mitochondria (Ren et al., 2017). HIF-1α, a Ca2+ sensitive factor, has been shown to be involved in tumor cell metastasis. In the hypoxic environment of colon cancer, highly expressed Piezo1 activates the MCU. MCU can regulate the concentration of intracellular calcium ions to promote the expression of HIF-1α. VEGF is involved in the migration, invasion, and metastasis of tumor cells. VEGF has been identified as a downstream target activated by HIF-1. Therefore Piezo1 and MCU are likely to play a role in colon cancer cell metastasis through the Piezo1-MCU-HIF-1α-VEGF pathway (Sun et al., 2020).
2.3.4 Under Acute Hypoxia, BKca Channel can Promote Mesenteric Vasodilation, Thereby Improving Mesenteric Microcirculation
Studies have shown that acute hypoxia affects the electrophysiological properties of guinea pig mesenteric arterial smooth muscle cells. BKCa channels belong to a heterogeneous family of Ca2+-activated K+ channels. Like most cells in vivo, K+ channel is the main ion channel in the cytoplasmic membrane of smooth muscle and it contributes significantly to resting membrane potential. Activation of the channel can lead to K+ cell efflux and membrane hyperpolarization (Guntur et al., 2021). Acute hypoxia can activate the outward current mediated by the BKca channel in the guinea pig mesenteric artery, thereby significantly enhancing the outward current. The activity and current magnitude of this channel directly affect vascular hyperpolarization and vasodilation. When the mesenteric artery smooth muscle cells are acutely hypoxic, the BKca channel on the membrane is activated to cause K+ efflux. Therefore, vascular smooth muscle cells are hyperpolarized to relax the blood vessels and improve mesenteric microcirculation (Ma et al., 2011).
2.3.5 IKCa Activation Promotes Increased Colonic Permeability Under Chemical Hypoxia
Chemical hypoxia can increase colonic permeability. Studies have shown that intestinal permeability may be related to basolateral membrane K+ channel activity. Metabolic stress secondary to chemical hypoxia causes a rapid increase in the activity of Ca2+-sensitive intermediate conductance K+ (IK Ca) channels in the basolateral membrane of natural human intestinal epithelial cells. It can greatly increase the whole cell conductance on the basal side of human colonic crypts and double the permeability of colonic membrane cells. Increased mucosal permeability may lead to bacterial translocation, sepsis, and multiple organ failure. The increase in colon permeability caused by chemical hypoxia can be prevented by IKCa channel inhibition, which may be a new way to prevent the harmful effects of increased intestinal permeability (Loganathan et al., 2011).
2.3.6 Hypoxic Preconditioning Increases Ca2+-ATPase Activity and Protects Intestinal Mucosal Cells From I/R Injury
In a rat liver transplantation model, hypoxia-induced HIF-1α expression protected mitochondrial function and Ca2+-ATPase activity to prevent I/R damage. As an important Ca2+ transporter, Ca2+-ATPase tightly controls intracellular Ca2+ levels. Ionized calcium is the ubiquitous second messenger that activates the signal cascade (Berridge, 1993; Brini and Carafoli, 2000; Berridge et al., 2003). Ca2+ signaling regulates a wide range of cellular and physiological processes, but prolonged elevation of intracellular free Ca2+ is toxic and can cause damage to cells (Orrenius et al., 2015). Therefore, secondary active transporters represented by plasma membrane Na+/Ca2+ exchangers will excrete excess Ca2+ in large quantities, thereby maintaining intracellular Ca2+ homeostasis. Decreased Ca2+-ATPase activity is an early manifestation of intestinal mucosal cells during ischemia–reperfusion injury. During intestinal ischemia, decreased Ca2+-ATPase activity can lead to intracellular calcium overload. During intestinal ischemia, the massive inactivation of Ca2+-ATPase allows the influx of extracellular calcium and greatly increases the concentration of calcium ions in the cytoplasm. Intracellular calcium-activated proteolytic enzyme produces a large number of free radicals. Oxygen free radicals destroy cell membrane lipids and can also lead to the inactivation of Na+-K+-ATP ATPase and Ca2+/Na+ exchange, which can promote Ca2+ influx and cause intracellular calcium overload (Lounsbury et al., 2000). At the same time, intracellular Ca2+ imbalance can lead to mitochondrial oxygen utilization disorder, which, in turn, leads to ATP synthesis disorder and cell damage. Hypoxic pretreatment (HP) can improve the hypoxia tolerance of small intestinal mucosal cells. Under hypoxia, HIF-1 increases Ca2+-ATPase activity and reduces apoptosis and pathological damage in small intestinal cells. HP may be an excellent way to promote the recovery of bowel function after transplantation (Ji et al., 2018).
2.4 The Role of Ion Transporters in the Pathogenesis of Liver Disease Under Hypoxic Conditions
2.4.1 TRPC6 Can Induce the Expression of Hypoxia-Inducible Factor-1-α and Promote Multidrug Resistance of Hepatocellular Carcinoma Cells
TRPC6 is also a member of the transient receptor potential (TRP) channel superfamily and promotes the development of multidrug resistance in hepatocellular carcinoma (HCC). Hepatocellular carcinoma is a highly malignant tumor with low sensitivity to chemotherapy. Part of this is because it is prone to multidrug resistance (MDR). Among them, EMT, HIF1-α signaling, and DNA damage repair play important roles in the multidrug resistance of HCC (Zhang et al., 2007; Teicher, 2009; Van Zijl et al., 2009; Luo et al., 2014). Several studies have shown that MDR-related mechanisms such as EMT, hypoxia-induced HIF1-α signaling pathway, and DNA damage repair are closely related to intracellular calcium. Intracellular calcium is a multifunctional secondary messenger involved in many physiological and pathological processes. The calcium signaling pathway plays a vital role in tumor cells through apoptosis, proliferation, invasion, and metastasis. Intracellular calcium homeostasis is regulated by calcium channels/pumps. In oncology, changes in the expression of specific calcium channels and pumps are characteristic of certain cancers (Monteith et al., 2007). TRPC6 is expressed at low levels in normal hepatocytes, but is highly expressed in liver cancer samples. Studies have shown that the expression of TRPC6 is significantly increased in the hypoxic environment of liver cancer cells. The overexpression of TRPC6 causes a continuous increase in intracellular free calcium and regulates the EMT, HIF1-α signaling, and DNA damage repair mechanisms related to multidrug resistance to stimulate and enhance the resistance of liver cancer cells to multiple drugs. However, after pretreatment with the calcium chelator BAPTA-AM, TRPC6 interference was observed. Hepatocarcinoma cells show a significant decrease in drug resistance under stimulation by EMT, HIF1-α signaling, and DNA damage repair mechanisms. Mechanisms of EMT, HIF1-α signaling, and DNA damage repair have been reported to be regulated by upstream calcium-dependent protein phosphorylation, such as Erk, AKT, and STAT3 (Li and Melton, 2012; Liu et al., 2014; Pawlus et al., 2014; Xu et al., 2015). Studies have shown that calcium chelation reduces the expression of STAT3, suggesting that STAT3 activation acts as a downstream regulator in TRPC6/calcium signaling. Collectively, the TRPC6/calcium/STAT3 pathway can mediate mechanisms such as EMT, HIF1-α signaling, and DNA damage repair to promote multidrug resistance in HCC cells under hypoxia. Targeting TRPC6 in the treatment of liver cancer may be a new antitumor strategy, especially in combination with chemotherapy (Wen et al., 2016).
2.4.2 Under Hypoxia, TRPC6 Stimulates Hepatic Stellate Cell Fibrosis
2.4.2.1 During Liver Fibrosis, HIF-1α Promotes the Expression of TRPC6 Through the Notch Pathway
Hypoxia is a key factor regulating liver fibrosis, which is a self-healing and healing process for chronic liver damage and is closely related to the development of liver cirrhosis and hepatocellular carcinoma. However, it is currently known to be a dynamic process characterized by the excessive synthesis and deposition of the extracellular matrix (ECM), which destroys the normal structure of the liver and ultimately leads to organ dysfunction and failure (Bataller and Brenner, 2005). In the process of fibrosis, hepatic stellate cells (HSCs) are undoubtedly the main cells responsible for the excessive deposition of the extracellular matrix (ECM). Hypoxia acts as an environmental stress factor to activate oxygen-sensitive hepatic stellate cells, and stellate cell activation is a unique initiating factor of liver fibrosis (Shi et al., 2007). Stimulated by hypoxia, hepatic stellate cells undergo a complex activation process leading to increased ECM synthesis and deposition in fibrotic livers. Hypoxia can activate the transcription of hypoxia-inducible factor (HIF), and HIF-1α can induce the expression of key signaling components of the Notch pathway. The Notch pathway plays an extremely important role in the process of fibrosis (Kavian et al., 2012), inducing transcription factors to trigger the differentiation of fibroblasts into myofibroblasts (Gao et al., 2012). The key to the Notch pathway is the expression of the Notch intracellular domain (NICD) (Suwanjunee et al., 2008). Activated NICD can enter the nucleus to interact with transcriptional regulators, replace transcriptional co-repressors, recruit coactivators, and finally perform transcriptional activation of target genes (Lai, 2002). Qiang et al. (2012) showed that HIF1-α can promote the expression of Notch downstream genes by inducing the expression of NICD (Reya et al., 2001; Qiang et al., 2012). The transcriptional regulator NICD can activate the transcription of many ion channel genes, one of which is TRPC6 (Chigurupati et al., 2010).
2.4.2.2 TRPC6 Can Promote ECM Deposition to Form Fibrosis
Under hypoxia, HIF-1α upregulates the expression of TRPC6 by inducing the expression of NICD. It is well known that Ca2+ channels are crucial in regulating growth control processes (Kunzelmann, 2005). Ca2+ influx mediated by TRPC6 channels directly activates calcineurin A expression. Increased calcineurin A activates various processes involved in myofibroblast and fibrotic transformation through its downstream activated T cell transcription effector nuclear factor (NFAT) (Kuwahara et al., 2006). Including ECM proteins such as α-SMA and collagen, which are activated by the transcriptional effector NFAT, both α-SMA and collagen mRNA transcript levels are significantly increased under hypoxia compared with normoxia. Furthermore, a previous study found that Smad3 is a main mediator of HSC fibrosis, especially in inducing collagen expression (Inagaki et al., 2001; Schnabl et al., 2001; Furukawa et al., 2003). Hypoxia-induced TRPC6 activates Smad2/3-dependent transforming growth factor-β signaling and promotes the upregulation of α smooth muscle actin, fibronectin, and collagen, which greatly promotes the transformation of activated hepatic stellate cells into myofibroblasts (Zhao et al., 2011). Taken together, hypoxia-induced TRPC6 activation leads to ECM protein deposition, which promotes the formation of liver fibrosis. TRPC6 appears to be a potential therapeutic target in the development of targeted intervention points for liver fibrotic diseases (Iyer et al., 2015).
2.4.3 Effects of STIM on Hepatocellular Carcinoma Under Hypoxic Environment
2.4.3.1 STIM Mediates SOCE Signaling Pathway
Matrix interacting molecules (STIMs) are a specialized class of single-channel transmembrane proteins ubiquitously expressed in the endoplasmic reticulum (ER) membrane. They typically associate with Orai ion channels in the plasma membrane (PM) to form calcium release-activated calcium (CRAC) channels. An intracellular signaling pathway called store-operated calcium entry (SOCE) is heavily dependent on CRAC channels. SOCE is one of the major pathways for calcium entry in non-excitable cells. The SOCE pathway is activated by ligand-induced depletion of ER calcium stores. STIM proteins acting as calcium sensors then sense this depletion and activate Orai ion channels through direct physical interactions to allow influx of calcium ions for storage refill and downstream signaling processes. SOCE regulates a variety of biological processes. Growing evidence suggests that SOCE even plays a key role in cancer cell proliferation, metastasis, and tumor neovascularization, as well as in antitumor immunity (Xie et al., 2016).
2.4.3.2 In Hypoxic Environment, STIM Interacts With HIF-1a to Promote Hepatocarcinogenesis
Hypoxia and intracellular Ca2+ transients are the basic characteristics of tumors, signaling cascades initiated or regulated by HIF-1 are critical for the process of tumorigenesis (Neumann et al., 2005), and STIM1 mediates SOCE activation and promotes tumor invasion and migration (Van de Vijver et al., 2002; Tsai et al., 2014). However, there is no clear consensus on the relationship between HIF-1 and STIM1. We were surprised to find that some studies have shown that in the hypoxic environment of liver cancer cells, hypoxia-induced HIF-1a promotes STIM1 expression and SOCE in liver cancer cells by directly binding to the STIM1 promoter. SOCE can positively regulate the expression of Vascular endothelial growth factor (VEGF) and other growth factors to promote the proliferation and migration of hepatoma cells. Meanwhile, SOCE stabilizes HIF-1a by activating CaMKII and p300, thereby preventing HIF-1a degradation. This regulatory loop aggravates the hypoxic microenvironment and accelerates the growth of tumors. Giving the HIF-1 inhibitor YC-1 or knocking out HIF1a can significantly reduce hypoxia-enhanced STIM1 and inhibit the occurrence of tumors. These results suggest that STIM1 and HIF-1 are interdependent and regulated in controlling Ca2+ mobilization and hypoxic tumor growth and may become potential targets for early cancer intervention (Y. Li et al., 2015).
2.4.4 STIM Mediates Hepatic Ischemia-Reperfusion (I/R) Injury Under Hypoxic Conditions
Hepatic ischemia–reperfusion (I/R) injury is unavoidable during trauma, elective liver resection, shock, or liver transplantation and has adverse effects on patients’ health (Inoue et al., 2014; Li et al., 2014). Hepatic I/R injury is a complex and multifactorial pathophysiological process involving the effects of inflammatory cytokines, ROS, and apoptosis (Sun et al., 2014). Furthermore, ROS induces the activation of Kupffer cells, which, in turn, produce a large amount of inflammatory cytokines and oxygen free radicals, further aggravating liver damage (Kalogeris et al., 2014; Tao et al., 2014). Under hypoxic conditions, the levels of the STIM1 gene and protein in Kupffer cells are significantly upregulated. The STIM protein is a calcium storage sensor that mediates cell responses to various stress conditions, including elevated ROS and hypoxia (Zhou et al., 2010). STIM can release and enter the cell to cause continuous Ca2+ overload and promote the production of the transcription factor NF-κB. NF-κB plays an important role in mediating inflammation by promoting the release of proinflammatory cytokines (Haddad, 2002). In I/R injury, the activation of NF-κB is related to an increase in tumor necrosis factor-α, interleukin-1β, and interleukin-6 (Lan et al., 2013). STIM1 gene knockout can reduce inflammation, oxidative stress, and apoptosis in cells exposed to hypoxia. Therefore, we believe that STIM1 can be used as a potential therapeutic target to improve I/R injury (Y. Li et al., 2018).
2.5 Ion Transporters Affect the Occurrence of Pancreatic Diseases Under Hypoxic Conditions
2.5.1 STIM1 Promotes the Invasion and Metastasis of Pancreatic Ductal Carcinoma Under Hypoxia
Pancreatic ductal adenocarcinoma (PDAC) is among the most aggressive and refractory cancers. Numerous studies have shown that the growth, invasion, and metastasis of PDAC are related to calcium ions and calcium channels (Morrone et al., 2016; Shapovalov et al., 2016; Yeung et al., 2017). Hypoxia is a common microenvironmental feature in tumors that promotes tumor growth and metastasis, especially in PDAC. Substantial evidence shows that the expression of hypoxia-inducible factor-1α in pancreatic cancer tissue is positively correlated with the expression of STIM1. HIF-1α can bind the HER2-3 region of the STIM1 promoter to regulate the transcription of STIM1. When shRNA was used to knock out hypoxia-inducible factor-1α in pancreatic cancer cells, STIM1 mRNA and protein were significantly reduced in the pancreatic cancer cells with HIF-1α gene knockout. HIF-1 frequently upregulates the expression of STIM1 in pancreatic cancer cells. STIM1 can upregulate vimentin and decrease the expression of E-cadherin, indicating that STIM1 may play a key role in PDAC oncogenic transformation, cell growth and invasion, and epithelial-mesenchymal transition (EMT). Moreover, studies have shown that high expression of STIM1 is significantly related to the tumor grade and early recurrence, which are important clinical factors affecting the prognosis of PDAC patients. Therefore, detecting the expression level of STIM1 in PDAC tissues can be used as a new method to predict the prognosis of PDAC patients. The HIF1α/STIM1 axis may be a potential therapeutic target for PDAC (Wang et al., 2019).
2.5.2 TRPC6 Promotes Pancreatic Stellate Cell Fibrosis Under Hypoxia
Pancreatic cancer is characterized by massive fibrosis mainly caused by activated pancreatic stellate cells (PSCs). Pancreatic stellate cells are the predominant mesenchymal cell type in the PDAC stroma and are responsible for the overproduction of extracellular matrix proteins. Pancreatic stellate cells are primarily affected by transforming growth factor beta (TGF-β), tumor necrosis factor alpha (TNF-α), and other factors such as platelet-derived growth factor (PDGF) or interleukin-8 (IL- 8) Activate. In the hypoxic environment of PDAC, hypoxia can promote the secretion of matrix proteins and growth factors to activate pancreatic stellate cells, but the specific mechanism of the action is not fully understood in molecular details. In recent years, studies have found that TRPC6 and Ca2+ signaling are involved in the activation of pancreatic stellate cells. Further studies have shown that most growth factors and chemokines trigger their actions through G protein-coupled receptors (GPCRs). The TRPC6 channel is the effector protein of these G protein-coupled receptor pathways. In the hypoxic tumor microenvironment, the TRPC6 channel participates in the continuous activation of PSCs, which is a typical feature of the PDAC microenvironment. At the same time, it provides a potential target for the treatment of pancreatic ductal carcinoma (Nielsen et al., 2017).
2.6 Inhibition of NCX Expression Prevents Hypoxia-Induced Pancreatic β-cell Damage
The Na+/Ca2+ exchanger (NCX) belongs to the antiporter family and is a major Ca2+ regulatory protein, expressed in all excitable and many non-excitable cells, and transports Ca2+ across the plasma membrane (Khananshvili, 2021) (Quednau et al., 2004). Islet B cells express NCX (Van Eylen et al., 1997). It is not only involved in maintaining glucose homeostasis by regulating Ca2+-dependent insulin secretion (Hamming et al., 2010), but also in cell survival or death through its anti-apoptotic or pro-apoptotic effects, respectively (Diaz-Horta et al., 2002; Nguidjoe et al., 2011). Immediately exposed to low oxygen tension, the transplanted islets release a large amount of HMGB1, which triggers innate immune rejection and activates DCs, NKT cells, and neutrophils to produce IFN-γ, and ultimately pancreatic transplantation fails. Therefore, the release of HMGB1 plays a crucial role in this process. Experiments have demonstrated that the release of HMGB1 from transplanted islets is due to hypoxia-induced Ca2+ influx into β cells through Na+/Ca2+ exchanger (NCX), and Ca2+ stimulates islet β cells to induce the release of HMGB1. Furthermore, hypoxia-induced β-cell damage is prevented by pretreatment with NCX-specific inhibitors prior to transplantation, resulting in protection and long-term survival of transplanted islets (Mera et al., 2013).
3 Conclusion
In summary, under hypoxia, ion-transporting proteins mediate various signaling pathways in various organs of the digestive system (Table 1), thereby regulating cell functions and participating in the pathophysiological process of the development of various diseases of the digestive system. HIF and ion-transporting proteins have become a new research hotspot and may become new markers for the diagnosis, treatment, and prognosis assessment of diseases of the digestive system. The development of drugs related to ion-transporting proteins and hypoxia could also become a new direction for the treatment of digestive system diseases.
Author Contributions
YX and DF wrote the manuscript. QA, TZ, XW, JD, XX, GY, ST, and QD collected the literature. JX and RX were responsible for the collection and assembly of the articles/published data for inclusion and interpretation in this review. All authors were involved in the writing of the manuscript. All authors read and approved the final manuscript.
Funding
This study was supported by research grants from the National Natural Science Foundation of China (No.82170628; No.81970541; No.31960151; No.32160208), the Guizhou Provincial Department of Science and Technology Excellent Youth Project ((2021)5647), the Collaborative Innovation Center of Chinese Ministry of Education (2020-39), the Basic Research Key Program of Guizhou Province (ZK (2021) key 004), and the Guizhou Graduate Research Fund (No. YJSKYJJ (2021) 177).
Conflict of Interest
The authors declare that the research was conducted in the absence of any commercial or financial relationships that could be construed as a potential conflict of interest.
Publisher’s Note
All claims expressed in this article are solely those of the authors and do not necessarily represent those of their affiliated organizations, or those of the publisher, the editors, and the reviewers. Any product that may be evaluated in this article, or claim that may be made by its manufacturer, is not guaranteed or endorsed by the publisher.
Acknowledgments
The authors thank Professor Biguang Tuo (Department of Gastroenterology, the Affiliated Hospital of Zunyi Medical University, Zunyi, P. R. China) for his assistance with grammar, spelling, and formatting of the manuscript.
References
Aarts M., Iihara K., Wei W.-L., Xiong Z.-G., Arundine M., Cerwinski W., et al. (2003). A Key Role for TRPM7 Channels in Anoxic Neuronal Death. Cell 115 (7), 863–877. doi:10.1016/s0092-8674(03)01017-1
Advani S. H. (2010). Targeting mTOR Pathway: A New Concept in Cancer Therapy. Indian J. Med. Paediatr. Oncol. 31 (4), 132–136. doi:10.4103/0971-5851.76197
Bataller R., Brenner D. A. (2005). Liver Fibrosis. J. Clin. Invest 115 (2), 209–218. doi:10.1172/jci24282
Benhamouche S., Decaens T., Godard C., Chambrey R., Rickman D. S., Moinard C., et al. (2006). Apc Tumor Suppressor Gene Is the "Zonation-Keeper" of Mouse Liver. Dev. Cell 10 (6), 759–770. doi:10.1016/j.devcel.2006.03.015
Berridge M. J., Bootman M. D., Roderick H. L. (2003). Calcium Signalling: Dynamics, Homeostasis and Remodelling. Nat. Rev. Mol. Cell Biol. 4 (7), 517–529. doi:10.1038/nrm1155
Berridge M. J. (1993). Inositol Trisphosphate and Calcium Signalling. Nature 361 (6410), 315–325. doi:10.1038/361315a0
Bijlsma M. F., Groot A. P., Oduro J. P., Franken R. J., Schoenmakers S., Peppelenbosch M. P., et al. (2009). Hypoxia Induces a Hedgehog Response Mediated by HIF-1alpha. J. Cell Mol. Med. 13 (8b), 2053–2060. doi:10.1111/j.1582-4934.2008.00491.x
Brazier S. P., Mason H. S., Bateson A. N., Kemp P. J. (2005). Cloning of the Human TASK-2 (KCNK5) Promoter and its Regulation by Chronic Hypoxia. Biochem. Biophys. Res. Commun. 336 (4), 1251–1258. doi:10.1016/j.bbrc.2005.09.007
Brini M., Carafoli E. (2000). Calcium Signalling: a Historical Account, Recent Developments and Future Perspectives. Cell Mol. Life Sci. 57 (3), 354–370. doi:10.1007/pl00000698
Buller C. L., Heilig C. W., Brosius F. C. (2011). GLUT1 Enhances mTOR Activity Independently of TSC2 and AMPK. Am. J. Physiol. Ren. Physiol. 301 (3), F588–F596. doi:10.1152/ajprenal.00472.2010
Chen J., Chen J., Fu H., Li Y., Wang L., Luo S., et al. (2019). Hypoxia Exacerbates Nonalcoholic Fatty Liver Disease via the HIF-2α/PPARα Pathway. Am. J. Physiol. Endocrinol. Metab. 317 (4), E710–e722. doi:10.1152/ajpendo.00052.2019
Chen M., Huang S. L., Zhang X. Q., Zhang B., Zhu H., Yang V. W., et al. (2012). Reversal Effects of Pantoprazole on Multidrug Resistance in Human Gastric Adenocarcinoma Cells by Down-Regulating the V-ATPases/mTOR/HIF-1α/p-Gp and MRP1 Signaling Pathway In Vitro and In Vivo. J. Cell Biochem. 113 (7), 2474–2487. doi:10.1002/jcb.24122
Chen Z., Zhu Y., Dong Y., Zhang P., Han X., Jin J., et al. (2017). Overexpression of TrpC5 Promotes Tumor Metastasis via the HIF-1α-Twist Signaling Pathway in Colon Cancer. Clin. Sci. (Lond) 131 (19), 2439–2450. doi:10.1042/cs20171069
Chigurupati S., Venkataraman R., Barrera D., Naganathan A., Madan M., Paul L., et al. (2010). Receptor Channel TRPC6 Is a Key Mediator of Notch-Driven Glioblastoma Growth and Invasiveness. Cancer Res. 70 (1), 418–427. doi:10.1158/0008-5472.can-09-2654
Chu W., Wan L., Zhao D., Qu X., Cai F., Huo R., et al. (2012). Mild Hypoxia-Induced Cardiomyocyte Hypertrophy via Up-Regulation of HIF-1α-Mediated TRPC Signalling. J. Cell Mol. Med. 16 (9), 2022–2034. doi:10.1111/j.1582-4934.2011.01497.x
Cid L. P., Roa-Rojas H. A., Niemeyer M. I., González W., Araki M., Araki K., et al. (2013). TASK-2: a K2P K(+) Channel with Complex Regulation and Diverse Physiological Functions. Front. Physiol. 4, 198. doi:10.3389/fphys.2013.00198
Cipriano D. J., Wang Y., Bond S., Hinton A., Jefferies K. C., Qi J., et al. (2008). Structure and Regulation of the Vacuolar ATPases. Biochim. Biophys. Acta 1777 (7-8), 599–604. doi:10.1016/j.bbabio.2008.03.013
Comerford K. M., Wallace T. J., Karhausen J., Louis N. A., Montalto M. C., Colgan S. P. (2002). Hypoxia-inducible Factor-1-dependent Regulation of the Multidrug Resistance (MDR1) Gene. Cancer Res. 62 (12), 3387–3394.
Cummins E. P., Crean D. (2017). Hypoxia and Inflammatory Bowel Disease. Microbes Infect. 19 (3), 210–221. doi:10.1016/j.micinf.2016.09.004
Diaz-Horta O., Kamagate A., Herchuelz A., Van Eylen F. (2002). Na/Ca Exchanger Overexpression Induces Endoplasmic Reticulum-Related Apoptosis and Caspase-12 Activation in Insulin-Releasing BRIN-BD11 Cells. Diabetes 51 (6), 1815–1824. doi:10.2337/diabetes.51.6.1815
Dong Q., Zhao N., Xia C. K., Du L. L., Fu X. X., Du Y. M. (2012). Hypoxia Induces Voltage-Gated K+ (Kv) Channel Expression in Pulmonary Arterial Smooth Muscle Cells through Hypoxia-Inducible Factor-1 (HIF-1). Bosn. J. Basic Med. Sci. 12 (3), 158–163. doi:10.17305/bjbms.2012.2463
Duan C. (2016). Hypoxia-inducible Factor 3 Biology: Complexities and Emerging Themes. Am. J. Physiol. Cell Physiol. 310 (4), C260–C269. doi:10.1152/ajpcell.00315.2015
Eigel B. N., Gursahani H., Hadley R. W. (2004). Na+/Ca2+ Exchanger Plays a Key Role in Inducing Apoptosis after Hypoxia in Cultured guinea Pig Ventricular Myocytes. Am. J. Physiol. Heart Circ. Physiol. 287 (4), H1466–H1475. doi:10.1152/ajpheart.00874.2003
Endo K., Kito H., Tanaka R., Kajikuri J., Tanaka S., Elboray E. E., et al. (2019). Possible Contribution of Inflammation-Associated Hypoxia to Increased K(2P)5.1 K(+) Channel Expression in CD4(+) T Cells of the Mouse Model for Inflammatory Bowel Disease. Int. J. Mol. Sci. 21 (1), 38. doi:10.3390/ijms21010038
Ferro A., Peleteiro B., Malvezzi M., Bosetti C., Bertuccio P., Levi F., et al. (2014). Worldwide Trends in Gastric Cancer Mortality (1980-2011), with Predictions to 2015, and Incidence by Subtype. Eur. J. Cancer 50 (7), 1330–1344. doi:10.1016/j.ejca.2014.01.029
Forgac M. (2007). Vacuolar ATPases: Rotary Proton Pumps in Physiology and Pathophysiology. Nat. Rev. Mol. Cell Biol. 8 (11), 917–929. doi:10.1038/nrm2272
Fuentes I. M., Christianson J. A. (2016). Ion Channels, Ion Channel Receptors, and Visceral Hypersensitivity in Irritable Bowel Syndrome. Neurogastroenterol. Motil. 28 (11), 1613–1618. doi:10.1111/nmo.12979
Furukawa F., Matsuzaki K., Mori S., Tahashi Y., Yoshida K., Sugano Y., et al. (2003). p38 MAPK Mediates Fibrogenic Signal through Smad3 Phosphorylation in Rat Myofibroblasts. Hepatology 38 (4), 879–889. doi:10.1053/jhep.2003.5038410.1002/hep.1840380414
Gan L., Meng J., Xu M., Liu M., Qi Y., Tan C., et al. (2018). Extracellular Matrix Protein 1 Promotes Cell Metastasis and Glucose Metabolism by Inducing Integrin β4/FAK/SOX2/HIF-1α Signaling Pathway in Gastric Cancer. Oncogene 37 (6), 744–755. doi:10.1038/onc.2017.363
Gao W., Sweeney C., Connolly M., Kennedy A., Ng C. T., McCormick J., et al. (2012). Notch-1 Mediates Hypoxia-Induced Angiogenesis in Rheumatoid Arthritis. Arthritis Rheum. 64 (7), 2104–2113. doi:10.1002/art.34397
Gillet J. P., Gottesman M. M. (2010). Mechanisms of Multidrug Resistance in Cancer. Methods Mol. Biol. 596, 47–76. doi:10.1007/978-1-60761-416-6_4
Guntur D., Olschewski H., Enyedi P., Csáki R., Olschewski A., Nagaraj C. (2021). Revisiting the Large-Conductance Calcium-Activated Potassium (BKCa) Channels in the Pulmonary Circulation. Biomolecules 11 (11), 1629. doi:10.3390/biom11111629
Haddad J. J. (2002). Redox Regulation of Pro-inflammatory Cytokines and IkappaB-alpha/NF-kappaB Nuclear Translocation and Activation. Biochem. Biophys. Res. Commun. 296 (4), 847–856. doi:10.1016/s0006-291x(02)00947-6
Hamming K. S., Soliman D., Webster N. J., Searle G. J., Matemisz L. C., Liknes D. A., et al. (2010). Inhibition of Beta-Cell Sodium-Calcium Exchange Enhances Glucose-dependent Elevations in Cytoplasmic Calcium and Insulin Secretion. Diabetes 59 (7), 1686–1693. doi:10.2337/db09-0630
Higashiyama M., Hokari R., Hozumi H., Kurihara C., Ueda T., Watanabe C., et al. (2012). HIF-1 in T Cells Ameliorated Dextran Sodium Sulfate-Induced Murine Colitis. J. Leukoc. Biol. 91 (6), 901–909. doi:10.1189/jlb.1011518
Hirakawa T., Yashiro M., Doi Y., Kinoshita H., Morisaki T., Fukuoka T., et al. (2016). Pancreatic Fibroblasts Stimulate the Motility of Pancreatic Cancer Cells through IGF1/IGF1R Signaling under Hypoxia. PLoS One 11 (8), e0159912. doi:10.1371/journal.pone.0159912
Hubbi M. E., Semenza G. L. (2015). Regulation of Cell Proliferation by Hypoxia-Inducible Factors. Am. J. Physiol. Cell Physiol. 309 (12), C775–C782. doi:10.1152/ajpcell.00279.2015
Hundahl S. A., Phillips J. L., Menck H. R. (2000). The National Cancer Data Base Report on Poor Survival of U.S. Gastric Carcinoma Patients Treated with Gastrectomy: Fifth Edition American Joint Committee on Cancer Staging, Proximal Disease, and the "different Disease" Hypothesis. Cancer 88 (4), 921–932. doi:10.1002/(sici)1097-0142(20000215)88:4<921::aid-cncr24>3.0.co;2-s
Inagaki Y., Mamura M., Kanamaru Y., Greenwel P., Nemoto T., Takehara K., et al. (2001). Constitutive Phosphorylation and Nuclear Localization of Smad3 Are Correlated with Increased Collagen Gene Transcription in Activated Hepatic Stellate Cells. J. Cell Physiol. 187 (1), 117–123. doi:10.1002/1097-4652(2001)9999:9999<00::aid-jcp1059>3.0.co;2-s
Inoue Y., Shirasuna K., Kimura H., Usui F., Kawashima A., Karasawa T., et al. (2014). NLRP3 Regulates Neutrophil Functions and Contributes to Hepatic Ischemia-Reperfusion Injury Independently of Inflammasomes. J. Immunol. 192 (9), 4342–4351. doi:10.4049/jimmunol.1302039
Ivanov A. I., Parkos C. A., Nusrat A. (2010). Cytoskeletal Regulation of Epithelial Barrier Function during Inflammation. Am. J. Pathol. 177 (2), 512–524. doi:10.2353/ajpath.2010.100168
Iyer S. C., Kannan A., Gopal A., Devaraj N., Halagowder D. (2015). Receptor Channel TRPC6 Orchestrate the Activation of Human Hepatic Stellate Cell under Hypoxia Condition. Exp. Cell Res. 336 (1), 66–75. doi:10.1016/j.yexcr.2015.03.023
Ji Z. P., Li Y. X., Shi B. X., Zhuang Z. N., Yang J. Y., Guo S., et al. (2018). Hypoxia Preconditioning Protects Ca(2+)-ATPase Activation of Intestinal Mucosal Cells against R/I Injury in a Rat Liver Transplantation Model. World J. Gastroenterol. 24 (3), 360–370. doi:10.3748/wjg.v24.i3.360
Joseph J. P., Harishankar M. K., Pillai A. A., Devi A. (2018). Hypoxia Induced EMT: A Review on the Mechanism of Tumor Progression and Metastasis in OSCC. Oral Oncol. 80, 23–32. doi:10.1016/j.oraloncology.2018.03.004
Kaczorowski G. J., McManus O. B., Priest B. T., Garcia M. L. (2008). Ion Channels as Drug Targets: the Next GPCRs. J. Gen. Physiol. 131 (5), 399–405. doi:10.1085/jgp.200709946
Kalluri R., Weinberg R. A. (2009). The Basics of Epithelial-Mesenchymal Transition. J. Clin. Invest 119 (6), 1420–1428. doi:10.1172/jci39104
Kalogeris T., Bao Y., Korthuis R. J. (2014). Mitochondrial Reactive Oxygen Species: a Double Edged Sword in Ischemia/reperfusion vs Preconditioning. Redox Biol. 2, 702–714. doi:10.1016/j.redox.2014.05.006
Kavian N., Servettaz A., Weill B., Batteux F. (2012). New Insights into the Mechanism of Notch Signalling in Fibrosis. Open Rheumatol. J. 6, 96–102. doi:10.2174/1874312901206010096
Ke Q., Costa M. (2006). Hypoxia-inducible Factor-1 (HIF-1). Mol. Pharmacol. 70 (5), 1469–1480. doi:10.1124/mol.106.027029
Keynes R. D. (1975). The Ionic Channels in Excitable Membranes. Ciba Found. Symp. 1 (31), 191–203. doi:10.1002/9780470720134.ch11
Khananshvili D. (2021). The Archaeal Na(+)/Ca(2+) Exchanger (NCX_Mj) as a Model of Ion Transport for the Superfamily of Ca(2+)/CA Antiporters. Front. Chem. 9, 722336. doi:10.3389/fchem.2021.722336
Kietzmann T. (2019). Liver Zonation in Health and Disease: Hypoxia and Hypoxia-Inducible Transcription Factors as Concert Masters. Int. J. Mol. Sci. 20 (9), 2347. doi:10.3390/ijms20092347
Kietzmann T. (2017). Metabolic Zonation of the Liver: The Oxygen Gradient Revisited. Redox Biol. 11, 622–630. doi:10.1016/j.redox.2017.01.012
Kita S., Furuta A., Takano Y., Iwamoto T. (2007). The Role of Na+/Ca2+ Exchanger in Endothelin-1-Aggravated Hypoxia/reoxygenation-Induced Injury in Renal Epithelial Cells. Ann. N. Y. Acad. Sci. 1099, 473–477. doi:10.1196/annals.1387.041
Kunzelmann K. (2005). Ion Channels and Cancer. J. Membr. Biol. 205 (3), 159–173. doi:10.1007/s00232-005-0781-4
Kuwahara K., Wang Y., McAnally J., Richardson J. A., Bassel-Duby R., Hill J. A., et al. (2006). TRPC6 Fulfills a Calcineurin Signaling Circuit during Pathologic Cardiac Remodeling. J. Clin. Invest 116 (12), 3114–3126. doi:10.1172/jci27702
Lai E. C. (2002). Keeping a Good Pathway Down: Transcriptional Repression of Notch Pathway Target Genes by CSL Proteins. EMBO Rep. 3 (9), 840–845. doi:10.1093/embo-reports/kvf170
Lan L., Tao J., Chen A., Xie G., Huang J., Lin J., et al. (2013). Electroacupuncture Exerts Anti-inflammatory Effects in Cerebral Ischemia-Reperfusion Injured Rats via Suppression of the TLR4/NF-Κb Pathway. Int. J. Mol. Med. 31 (1), 75–80. doi:10.3892/ijmm.2012.1184
Lee J. W., Bae S. H., Jeong J. W., Kim S. H., Kim K. W. (2004). Hypoxia-inducible Factor (HIF-1)alpha: its Protein Stability and Biological Functions. Exp. Mol. Med. 36 (1), 1–12. doi:10.1038/emm.2004.1
Lee K., Nelson C. M. (2012). New Insights into the Regulation of Epithelial-Mesenchymal Transition and Tissue Fibrosis. Int. Rev. Cell Mol. Biol. 294, 171–221. doi:10.1016/b978-0-12-394305-7.00004-5
Li C. X., Ng K. T., Shao Y., Liu X. B., Ling C. C., Ma Y. Y., et al. (2014). The Inhibition of Aldose Reductase Attenuates Hepatic Ischemia-Reperfusion Injury through Reducing Inflammatory Response. Ann. Surg. 260 (2), 317–328. doi:10.1097/sla.0000000000000429
Li R. L., He L. Y., Zhang Q., Liu J., Lu F., Duan H. X., et al. (2020). HIF-1α Is a Potential Molecular Target for Herbal Medicine to Treat Diseases. Drug Des. Devel Ther. 14, 4915–4949. doi:10.2147/dddt.s274980
Li W., Melton D. W. (2012). Cisplatin Regulates the MAPK Kinase Pathway to Induce Increased Expression of DNA Repair Gene ERCC1 and Increase Melanoma Chemoresistance. Oncogene 31 (19), 2412–2422. doi:10.1038/onc.2011.426
Li Y., Guo B., Xie Q., Ye D., Zhang D., Zhu Y., et al. (2015). STIM1 Mediates Hypoxia-Driven Hepatocarcinogenesis via Interaction with HIF-1. Cell Rep. 12 (3), 388–395. doi:10.1016/j.celrep.2015.06.033
Li Y., Lou C., Wang W. (2018). STIM1 Deficiency Protects the Liver from Ischemia/reperfusion Injury in Mice. Biochem. Biophys. Res. Commun. 496 (2), 422–428. doi:10.1016/j.bbrc.2018.01.006
Lin S. C., Liao W. L., Lee J. C., Tsai S. J. (2014). Hypoxia-regulated Gene Network in Drug Resistance and Cancer Progression. Exp. Biol. Med. (Maywood) 239 (7), 779–792. doi:10.1177/1535370214532755
Liu A., Wu J., Yang C., Wu Y., Zhang Y., Zhao F., et al. (2018). TRPM7 in CHBP-Induced Renoprotection upon Ischemia Reperfusion-Related Injury. Sci. Rep. 8 (1), 5510. doi:10.1038/s41598-018-22852-2
Liu H., Hu Y. P., Savaraj N., Priebe W., Lampidis T. J. (2001). Hypersensitization of Tumor Cells to Glycolytic Inhibitors. Biochemistry 40 (18), 5542–5547. doi:10.1021/bi002426w
Liu L., Ning X., Sun L., Zhang H., Shi Y., Guo C., et al. (2008). Hypoxia-inducible Factor-1 Alpha Contributes to Hypoxia-Induced Chemoresistance in Gastric Cancer. Cancer Sci. 99 (1), 121–128. doi:10.1111/j.1349-7006.2007.00643.x
Liu Q., Sun B., Zhao J., Wang Q., An F., Hu X., et al. (2018). Increased Piezo1 Channel Activity in Interstitial Cajal-like Cells Induces Bladder Hyperactivity by Functionally Interacting with NCX1 in Rats with Cyclophosphamide-Induced Cystitis. Exp. Mol. Med. 50 (5), 1–16. doi:10.1038/s12276-018-0088-z
Liu W. L., Gao M., Tzen K. Y., Tsai C. L., Hsu F. M., Cheng A. L., et al. (2014). Targeting Phosphatidylinositide3-Kinase/Akt Pathway by BKM120 for Radiosensitization in Hepatocellular Carcinoma. Oncotarget 5 (11), 3662–3672. doi:10.18632/oncotarget.1978
Lo J. F., Wang Y., Li Z., Zhao Z., Hu D., Eddington D. T., et al. (2013). Quantitative and Temporal Control of Oxygen Microenvironment at the Single Islet Level. J. Vis. Exp. 1 (81), e50616. doi:10.3791/50616
Loboda A., Jozkowicz A., Dulak J. (2010). HIF-1 and HIF-2 Transcription Factors-Ssimilar but Not Identical. Mol. Cells 29 (5), 435–442. doi:10.1007/s10059-010-0067-2
Loganathan A., Linley J. E., Rajput I., Hunter M., Lodge J. P., Sandle G. I. (2011). Basolateral Potassium (IKCa) Channel Inhibition Prevents Increased Colonic Permeability Induced by Chemical Hypoxia. Am. J. Physiol. Gastrointest. Liver Physiol. 300 (1), G146–G153. doi:10.1152/ajpgi.00472.2009
López-Cayuqueo K. I., Peña-Münzenmayer G., Niemeyer M. I., Sepúlveda F. V., Cid L. P. (2015). TASK-2 K₂p K⁺ Channel: Thoughts about Gating and its Fitness to Physiological Function. Pflugers Arch. 467 (5), 1043–1053. doi:10.1007/s00424-014-1627-7
Lounsbury K. M., Hu Q., Ziegelstein R. C. (2000). Calcium Signaling and Oxidant Stress in the Vasculature. Free Radic. Biol. Med. 28 (9), 1362–1369. doi:10.1016/s0891-5849(00)00222-7
Luo D., Wang Z., Wu J., Jiang C., Wu J. (2014). The Role of Hypoxia Inducible Factor-1 in Hepatocellular Carcinoma. Biomed. Res. Int. 2014, 409272. doi:10.1155/2014/409272
Ma K. T., Li L., Guan B. C., Li X. Z., Zhu H., Zhao L., et al. (2011). [Effects of Acute Hypoxia on the Electrophysiological Properties of Vascular Smooth Muscle Cells of Mesenteric Artery in guinea Pig]. Zhonghua Yi Xue Za Zhi 91 (46), 3289–3292.
Ma X., Zhang H., Xue X., Shah Y. M. (2017). Hypoxia-inducible Factor 2α (HIF-2α) Promotes Colon Cancer Growth by Potentiating Yes-Associated Protein 1 (YAP1) Activity. J. Biol. Chem. 292 (41), 17046–17056. doi:10.1074/jbc.M117.805655
Martínez-Lacaci I., García Morales P., Soto J. L., Saceda M. (2007). Tumour Cells Resistance in Cancer Therapy. Clin. Transl. Oncol. 9 (1), 13–20. doi:10.1007/s12094-007-0004-9
Matz-Soja M., Hovhannisyan A., Gebhardt R. (2013). Hedgehog Signalling Pathway in Adult Liver: a Major New Player in Hepatocyte Metabolism and Zonation? Med. Hypotheses 80 (5), 589–594. doi:10.1016/j.mehy.2013.01.032
Mazumdar J., O'Brien W. T., Johnson R. S., LaManna J. C., Chavez J. C., Klein P. S., et al. (2010). O2 Regulates Stem Cells through Wnt/β-Catenin Signalling. Nat. Cell Biol. 12 (10), 1007–1013. doi:10.1038/ncb2102
Mera T., Itoh T., Kita S., Kodama S., Kojima D., Nishinakamura H., et al. (2013). Pretreatment of Donor Islets with the Na(+)/Ca(2+) Exchanger Inhibitor Improves the Efficiency of Islet Transplantation. Am. J. Transpl. 13 (8), 2154–2160. doi:10.1111/ajt.12306
Miyamoto T., Mochizuki T., Nakagomi H., Kira S., Watanabe M., Takayama Y., et al. (2014). Functional Role for Piezo1 in Stretch-Evoked Ca2⁺ Influx and ATP Release in Urothelial Cell Cultures. J. Biol. Chem. 289 (23), 16565–16575. doi:10.1074/jbc.M113.528638
Monteith G. R., McAndrew D., Faddy H. M., Roberts-Thomson S. J. (2007). Calcium and Cancer: Targeting Ca2+ Transport. Nat. Rev. Cancer 7 (7), 519–530. doi:10.1038/nrc2171
Morrone F. B., Gehring M. P., Nicoletti N. F. (2016). Calcium Channels and Associated Receptors in Malignant Brain Tumor Therapy. Mol. Pharmacol. 90 (3), 403–409. doi:10.1124/mol.116.103770
Nagao A., Kobayashi M., Koyasu S., Chow C. C. T., Harada H. (2019). HIF-1-Dependent Reprogramming of Glucose Metabolic Pathway of Cancer Cells and its Therapeutic Significance. Int. J. Mol. Sci. 20 (2), 238. doi:10.3390/ijms20020238
Nakakura S., Matsui M., Sato A., Ishii M., Endo K., Muragishi S., et al. (2015). Pathophysiological Significance of the Two-Pore Domain K(+) Channel K2P5.1 in Splenic CD4(+)CD25(-) T Cell Subset from a Chemically-Induced Murine Inflammatory Bowel Disease Model. Front. Physiol. 6, 299. doi:10.3389/fphys.2015.00299
Nakayama K., Kataoka N. (2019). Regulation of Gene Expression under Hypoxic Conditions. Int. J. Mol. Sci. 20 (13), 3278. doi:10.3390/ijms20133278
Nassri A., Zhu H., Muftah M., Ramzan Z. (2018). Epidemiology and Survival of Esophageal Cancer Patients in an American Cohort. Cureus 10 (4), e2507. doi:10.7759/cureus.2507
Neumann A. K., Yang J., Biju M. P., Joseph S. K., Johnson R. S., Haase V. H., et al. (2005). Hypoxia Inducible Factor 1 Alpha Regulates T Cell Receptor Signal Transduction. Proc. Natl. Acad. Sci. U. S. A. 102 (47), 17071–17076. doi:10.1073/pnas.0506070102
Nguidjoe E., Sokolow S., Bigabwa S., Pachera N., D'Amico E., Allagnat F., et al. (2011). Heterozygous Inactivation of the Na/Ca Exchanger Increases Glucose-Induced Insulin Release, β-cell Proliferation, and Mass. Diabetes 60 (8), 2076–2085. doi:10.2337/db10-0924
Nielsen N., Kondratska K., Ruck T., Hild B., Kovalenko I., Schimmelpfennig S., et al. (2017). TRPC6 Channels Modulate the Response of Pancreatic Stellate Cells to Hypoxia. Pflugers Arch. 469 (12), 1567–1577. doi:10.1007/s00424-017-2057-0
Ohya S., Kito H., Hatano N., Muraki K. (2016). Recent Advances in Therapeutic Strategies that Focus on the Regulation of Ion Channel Expression. Pharmacol. Ther. 160, 11–43. doi:10.1016/j.pharmthera.2016.02.001
Orrenius S., Gogvadze V., Zhivotovsky B. (2015). Calcium and Mitochondria in the Regulation of Cell Death. Biochem. Biophys. Res. Commun. 460 (1), 72–81. doi:10.1016/j.bbrc.2015.01.137
Ortiz-Prado E., Dunn J. F., Vasconez J., Castillo D., Viscor G. (2019). Partial Pressure of Oxygen in the Human Body: a General Review. Am. J. Blood Res. 9 (1), 1–14.
Ozben T. (2006). Mechanisms and Strategies to Overcome Multiple Drug Resistance in Cancer. FEBS Lett. 580 (12), 2903–2909. doi:10.1016/j.febslet.2006.02.020
Parpaite T., Cardouat G., Mauroux M., Gillibert-Duplantier J., Robillard P., Quignard J. F., et al. (2016). Effect of Hypoxia on TRPV1 and TRPV4 Channels in Rat Pulmonary Arterial Smooth Muscle Cells. Pflugers Arch. 468 (1), 111–130. doi:10.1007/s00424-015-1704-6
Pawlus M. R., Wang L., Hu C. J. (2014). STAT3 and HIF1α Cooperatively Activate HIF1 Target Genes in MDA-MB-231 and RCC4 Cells. Oncogene 33 (13), 1670–1679. doi:10.1038/onc.2013.115
Pelicano H., Martin D. S., Xu R. H., Huang P. (2006). Glycolysis Inhibition for Anticancer Treatment. Oncogene 25 (34), 4633–4646. doi:10.1038/sj.onc.1209597
Perez-Moreno M. A., Locascio A., Rodrigo I., Dhondt G., Portillo F., Nieto M. A., et al. (2001). A New Role for E12/E47 in the Repression of E-Cadherin Expression and Epithelial-Mesenchymal Transitions. J. Biol. Chem. 276 (29), 27424–27431. doi:10.1074/jbc.M100827200
Qiang L., Wu T., Zhang H. W., Lu N., Hu R., Wang Y. J., et al. (2012). HIF-1α Is Critical for Hypoxia-Mediated Maintenance of Glioblastoma Stem Cells by Activating Notch Signaling Pathway. Cell Death Differ. 19 (2), 284–294. doi:10.1038/cdd.2011.95
Quednau B. D., Nicoll D. A., Philipson K. D. (2004). The Sodium/calcium Exchanger Family-SLC8. Pflugers Arch. 447 (5), 543–548. doi:10.1007/s00424-003-1065-4
Rees D. C., Johnson E., Lewinson O. (2009). ABC Transporters: the Power to Change. Nat. Rev. Mol. Cell Biol. 10 (3), 218–227. doi:10.1038/nrm2646
Ren T., Zhang H., Wang J., Zhu J., Jin M., Wu Y., et al. (2017). MCU-Dependent Mitochondrial Ca(2+) Inhibits NAD(+)/SIRT3/SOD2 Pathway to Promote ROS Production and Metastasis of HCC Cells. Oncogene 36 (42), 5897–5909. doi:10.1038/onc.2017.167
Reya T., Morrison S. J., Clarke M. F., Weissman I. L. (2001). Stem Cells, Cancer, and Cancer Stem Cells. Nature 414 (6859), 105–111. doi:10.1038/35102167
Russo A., Franceschi S. (1996). The Epidemiology of Esophageal Cancer. Ann. Ist. Super. Sanita 32 (1), 65–72.
Saeedi B. J., Kao D. J., Kitzenberg D. A., Dobrinskikh E., Schwisow K. D., Masterson J. C., et al. (2015). HIF-Dependent Regulation of Claudin-1 Is Central to Intestinal Epithelial Tight Junction Integrity. Mol. Biol. Cell 26 (12), 2252–2262. doi:10.1091/mbc.E14-07-1194
Schnabl B., Kweon Y. O., Frederick J. P., Wang X. F., Rippe R. A., Brenner D. A. (2001). The Role of Smad3 in Mediating Mouse Hepatic Stellate Cell Activation. Hepatology 34 (1), 89–100. doi:10.1053/jhep.2001.25349
Schwab A., Stock C. (2014). Ion Channels and Transporters in Tumour Cell Migration and Invasion. Philos. Trans. R. Soc. Lond B Biol. Sci. 369 (1638), 20130102. doi:10.1098/rstb.2013.0102
Semenza G. L. (2002). Involvement of Hypoxia-Inducible Factor 1 in Human Cancer. Intern Med. 41 (2), 79–83. doi:10.2169/internalmedicine.41.79
Shapovalov G., Ritaine A., Skryma R., Prevarskaya N. (2016). Role of TRP Ion Channels in Cancer and Tumorigenesis. Semin. Immunopathol. 38 (3), 357–369. doi:10.1007/s00281-015-0525-1
Shi Y. F., Fong C. C., Zhang Q., Cheung P. Y., Tzang C. H., Wu R. S., et al. (2007). Hypoxia Induces the Activation of Human Hepatic Stellate Cells LX-2 through TGF-Beta Signaling Pathway. FEBS Lett. 581 (2), 203–210. doi:10.1016/j.febslet.2006.12.010
Simonneau G., Gatzoulis M. A., Adatia I., Celermajer D., Denton C., Ghofrani A., et al. (2014). Updated Clinical Classification of Pulmonary Hypertension. Turk Kardiyol. Dern. Ars 42 (Suppl. 1), 45–54. doi:10.1016/j.jacc.2013.10.029
Singhal R., Shah Y. M. (2020). Oxygen Battle in the Gut: Hypoxia and Hypoxia-Inducible Factors in Metabolic and Inflammatory Responses in the Intestine. J. Biol. Chem. 295 (30), 10493–10505. doi:10.1074/jbc.REV120.011188
Son S. W., Chau G. C., Kim S. T., Um S. H. (2019). Vacuolar H(+)-ATPase Subunit V0C Regulates Aerobic Glycolysis of Esophageal Cancer Cells via PKM2 Signaling. Cells 8 (10), 1137. doi:10.3390/cells8101137
Son S. W., Kim S. H., Moon E. Y., Kim D. H., Pyo S., Um S. H. (2016). Prognostic Significance and Function of the Vacuolar H+-ATPase Subunit V1E1 in Esophageal Squamous Cell Carcinoma. Oncotarget 7 (31), 49334–49348. doi:10.18632/oncotarget.10340
Sun Y., Li M., Liu G., Zhang X., Zhi L., Zhao J., et al. (2020). The Function of Piezo1 in Colon Cancer Metastasis and its Potential Regulatory Mechanism. J. Cancer Res. Clin. Oncol. 146 (5), 1139–1152. doi:10.1007/s00432-020-03179-w
Sun Y., Pu L. Y., Lu L., Wang X. H., Zhang F., Rao J. H. (2014). N-Acetylcysteine Attenuates Reactive-Oxygen-Species-Mediated Endoplasmic Reticulum Stress during Liver Ischemia-Reperfusion Injury. World J. Gastroenterol. 20 (41), 15289–15298. doi:10.3748/wjg.v20.i41.15289
Suwanjunee S., Wongchana W., Palaga T. (2008). Inhibition of Gamma-Secretase Affects Proliferation of Leukemia and Hepatoma Cell Lines through Notch Signaling. Anticancer Drugs 19 (5), 477–486. doi:10.1097/CAD.0b013e3282fc6cdd
Szakács G., Paterson J. K., Ludwig J. A., Booth-Genthe C., Gottesman M. M. (2006). Targeting Multidrug Resistance in Cancer. Nat. Rev. Drug Discov. 5 (3), 219–234. doi:10.1038/nrd1984
Tao X., Wan X., Xu Y., Xu L., Qi Y., Yin L., et al. (2014). Dioscin Attenuates Hepatic Ischemia-Reperfusion Injury in Rats through Inhibition of Oxidative-Nitrative Stress, Inflammation and Apoptosis. Transplantation 98 (6), 604–611. doi:10.1097/tp.0000000000000262
Taylor C. T., Colgan S. P. (2017). Regulation of Immunity and Inflammation by Hypoxia in Immunological Niches. Nat. Rev. Immunol. 17 (12), 774–785. doi:10.1038/nri.2017.103
Teicher B. A. (2009). Acute and Chronic In Vivo Therapeutic Resistance. Biochem. Pharmacol. 77 (11), 1665–1673. doi:10.1016/j.bcp.2009.01.006
Thiery J. P., Acloque H., Huang R. Y., Nieto M. A. (2009). Epithelial-mesenchymal Transitions in Development and Disease. Cell 139 (5), 871–890. doi:10.1016/j.cell.2009.11.007
Tsai F. C., Seki A., Yang H. W., Hayer A., Carrasco S., Malmersjö S., et al. (2014). A Polarized Ca2+, Diacylglycerol and STIM1 Signalling System Regulates Directed Cell Migration. Nat. Cell Biol. 16 (2), 133–144. doi:10.1038/ncb2906
Van de Vijver M. J., He Y. D., van't Veer L. J., Dai H., Hart A. A., Voskuil D. W., et al. (2002). A Gene-Expression Signature as a Predictor of Survival in Breast Cancer. N. Engl. J. Med. 347 (25), 1999–2009. doi:10.1056/NEJMoa021967
Van Eylen F., Svoboda M., Herchuelz A. (1997). Identification, Expression Pattern and Potential Activity of Na/Ca Exchanger Isoforms in Rat Pancreatic B-Cells. Cell Calcium 21 (3), 185–193. doi:10.1016/s0143-4160(97)90043-9
Van Zijl F., Zulehner G., Petz M., Schneller D., Kornauth C., Hau M., et al. (2009). Epithelial-mesenchymal Transition in Hepatocellular Carcinoma. Future Oncol. 5 (8), 1169–1179. doi:10.2217/fon.09.91
Venkatachalam K., Montell C. (2007). TRP Channels. Annu. Rev. Biochem. 76, 387–417. doi:10.1146/annurev.biochem.75.103004.142819
Venter J. C., Adams M. D., Myers E. W., Li P. W., Mural R. J., Sutton G. G., et al. (2001). The Sequence of the Human Genome. Science 291 (5507), 1304–1351. doi:10.1126/science.1058040
Wang J., Shen J., Zhao K., Hu J., Dong J., Sun J. (2019). STIM1 Overexpression in Hypoxia Microenvironment Contributes to Pancreatic Carcinoma Progression. Cancer Biol. Med. 16 (1), 100–108. doi:10.20892/j.issn.2095-3941.2018.0304
Wang X., Song X., Cheng G., Zhang J., Dong L., Bai J., et al. (2020). The Regulatory Mechanism and Biological Significance of Mitochondrial Calcium Uniporter in the Migration, Invasion, Angiogenesis and Growth of Gastric Cancer. Onco Targets Ther. 13, 11781–11794. doi:10.2147/ott.s262049
Wen L., Liang C., Chen E., Chen W., Liang F., Zhi X., et al. (2016). Regulation of Multi-Drug Resistance in Hepatocellular Carcinoma Cells Is TRPC6/Calcium Dependent. Sci. Rep. 6, 23269. doi:10.1038/srep23269
Williams S., Bateman A., O'Kelly I. (2013). Altered Expression of Two-Pore Domain Potassium (K2P) Channels in Cancer. PLoS One 8 (10), e74589. doi:10.1371/journal.pone.0074589
Wong C. C., Kai A. K., Ng I. O. (2014). The Impact of Hypoxia in Hepatocellular Carcinoma Metastasis. Front. Med. 8 (1), 33–41. doi:10.1007/s11684-013-0301-3
Xie J., Pan H., Yao J., Zhou Y., Han W. (2016). SOCE and Cancer: Recent Progress and New Perspectives. Int. J. Cancer 138 (9), 2067–2077. doi:10.1002/ijc.29840
Xu S. H., Huang J. Z., Xu M. L., Yu G., Yin X. F., Chen D., et al. (2015). ACK1 Promotes Gastric Cancer Epithelial-Mesenchymal Transition and Metastasis through AKT-Pou2f1-ECD Signalling. J. Pathol. 236 (2), 175–185. doi:10.1002/path.4515
Yang M. H., Wu M. Z., Chiou S. H., Chen P. M., Chang S. Y., Liu C. J., et al. (2008). Direct Regulation of TWIST by HIF-1alpha Promotes Metastasis. Nat. Cell Biol. 10 (3), 295–305. doi:10.1038/ncb1691
Yeung P. S., Yamashita M., Prakriya M. (2017). Pore Opening Mechanism of CRAC Channels. Cell Calcium 63, 14–19. doi:10.1016/j.ceca.2016.12.006
Zhang H., Mizumachi T., Carcel-Trullols J., Li L., Naito A., Spencer H. J., et al. (2007). Targeting Human 8-oxoguanine DNA Glycosylase (hOGG1) to Mitochondria Enhances Cisplatin Cytotoxicity in Hepatoma Cells. Carcinogenesis 28 (8), 1629–1637. doi:10.1093/carcin/bgm072
Zhang J., Jin H. Y., Wu Y., Zheng Z. C., Guo S., Wang Y., et al. (2019). Hypoxia-induced LncRNA PCGEM1 Promotes Invasion and Metastasis of Gastric Cancer through Regulating SNAI1. Clin. Transl. Oncol. 21 (9), 1142–1151. doi:10.1007/s12094-019-02035-9
Zhang Q Q., Zhang J., Fu Z., Dong L., Tang Y., Xu C., et al. (2019). Hypoxia-induced microRNA-10b-3p Promotes Esophageal Squamous Cell Carcinoma Growth and Metastasis by Targeting TSGA10. Aging (Albany NY) 11 (22), 10374–10384. doi:10.18632/aging.102462
Zhao W., Zhang L., Yin Z., Su W., Ren G., Zhou C., et al. (2011). Activated Hepatic Stellate Cells Promote Hepatocellular Carcinoma Development in Immunocompetent Mice. Int. J. Cancer 129 (11), 2651–2661. doi:10.1002/ijc.25920
Zheng L., Kelly C. J., Colgan S. P. (2015). Physiologic hypoxia and oxygen homeostasis in the healthy intestine. A Review in the Theme: Cellular Responses to Hypoxia. Am. J. Physiol. Cell Physiol. 309 (6), C350–C360. doi:10.1152/ajpcell.00191.2015
Zhi X., Xiong J., Wang M., Zhang H., Huang G., Zhao J., et al. (2018). Physiological Hypoxia Enhances Stemness Preservation, Proliferation, and Bidifferentiation of Induced Hepatic Stem Cells. Oxid. Med. Cell Longev. 2018, 7618704. doi:10.1155/2018/7618704
Keywords: hypoxia, ion, ion-transporting protein, digestive, system
Citation: Xiang Y, Fan D, An Q, Zhang T, Wu X, Ding J, Xu X, Yue G, Tang S, Du Q, Xu J and Xie R (2022) Effects of Ion-Transporting Proteins on the Digestive System Under Hypoxia. Front. Physiol. 13:870243. doi: 10.3389/fphys.2022.870243
Received: 07 February 2022; Accepted: 13 June 2022;
Published: 14 September 2022.
Edited by:
Vito De Pinto, University of Catania, ItalyReviewed by:
Marcelo Catalan, Austral University of Chile, ChileNazzareno Capitanio, University of Foggia, Italy
Copyright © 2022 Xiang, Fan, An, Zhang, Wu, Ding, Xu, Yue, Tang, Du, Xu and Xie. This is an open-access article distributed under the terms of the Creative Commons Attribution License (CC BY). The use, distribution or reproduction in other forums is permitted, provided the original author(s) and the copyright owner(s) are credited and that the original publication in this journal is cited, in accordance with accepted academic practice. No use, distribution or reproduction is permitted which does not comply with these terms.
*Correspondence: Jingyu Xu, eHVqaW5neXVfZ3p6eUAxMjYuY29t; Rui Xie, eHIxOTg0MTAyOUBhbGl5dW4uY29t
†These authors have contributed equally to this work