- 1Graduate School of Agricultural Science, Kobe University, Kobe, Japan
- 2Graduate School of Bio-Applications and Systems Engineering, Tokyo University of Agriculture and Technology, Tokyo, Japan
The photoperiodic system is concealed in the highly complex black-box, comprising four functional subunits: 1) a photo/thermo-sensitive input unit, 2) a photoperiodic clock based on a circadian system, 3) a condenser unit counting the number of inductive signals, and 4) a neuroendocrine switch that triggers a phenotypic shift. This review aims to summarize the research history and current reach of our understanding on this subject to connect it with the molecular mechanism of the circadian clock rapidly being unveiled. The review also focuses on the mode of intersubunit information transduction. It will scan the recent advancement in research on each functional subunit, but special attention will be given to the circadian clock–endocrine conjunct and the role of melatonin signaling in the regulation of insect photoperiodism. Prothoracicotropic hormone (PTTH) probably plays the most crucial role in the regulation of pupal diapause, which is the simplest model system of diapause regulation by hormones investigated so far, particularly in the Chinese oak silkmoth (Antheraea pernyi). A search for the trigger to release the PTTH found some candidates, that is, indoleamines. Indolamine metabolism is controlled by arylalkylamine N-acetyltransferase (aaNAT). Indolamine dynamics and aaNAT enzymatic activity changed according to photoperiods. aaNAT activity and melatonin content in the brain showed not only a photoperiodic response but also a circadian fluctuation. aaNAT had multiple E-boxes, suggesting that it is a clock-controlled gene (ccg), which implies that cycle (cyc, or brain–muscle Arnt-like 1 = Bmal1)/Clock (Clk) heterodimer binds to E-box and stimulates the transcription of aaNAT, which causes the synthesis of melatonin. RNAi against transcription modulators, cyc, or Clk downregulated aaNAT transcription, while RNAi against repressor of cyc/Clk, per upregulated aaNAT transcription. Immunohistochemical localization showed that the circadian neurons carry epitopes of melatonin-producing elements such as aaNAT, the precursor serotonin, HIOMT, and melatonin as well as clock gene products such as cyc-ir, Per-ir, and dbt-ir, while PTTH-producing neurons juxtaposed against the clock neurons showed hMT2-ir in A. pernyi brain. Melatonin probably binds to the putative melatonin receptor (MT) that stimulates Ca2+ influx, which in turn activates PKC. This induces Rab 8 phosphorylation and exocytosis of PTTH, leading to termination of diapause. All the PTTH-expressing neurons have PKC-ir, and Rab8-ir. When diapause is induced and maintained under short days, serotonin binding to 5HTR1B suppresses PTTH release in a yet unknown way. RNAi against this receptor knocked out photoperiodism; short day response is blocked and diapause was terminated even under the short day condition. The result showed that a relatively simple system controls both induction and termination in pupal diapause of A. pernyi: the circadian system regulates the transcription of aaNAT as a binary switch, the enzyme produces a melatonin rhythm that gates PTTH release, and 5HTR1B and MT are probably also under photoperiodic regulation. Finally, we listed the remaining riddles which need to be resolved, to fully understand this highly complex system in future studies.
Dawn of Photoperiodic Research in Insects and Mites
Phenotypic plasticity is most extensively observed in the developmental time course of species of the phylum Arthropoda. Photoperiodic determination of diapause/non-diapause shift is the culmination of life cycle adaptations in insects and mites because it determines their voltinism and increases stress tolerance. However, the regulatory mechanisms of these shifts still remain unknown (Withrow, 1959; Nelson et al., 2010).
The photoperiodic system comprises four functional subunits, each capable of being an independent target of study: 1) an input pathway, usually mediated via a photo- or thermo-sensor; 2) a photoperiodic clock based on the circadian system; 3) a photoperiodic counter of inductive cycle number; and 4) an output pathway, usually a neuroendocrine switch that induces a phenotypic shift. Studies on each subunit are valuable, but the information flow across the subunits has not received much attention.
The hormonal regulation of diapause has been studied extensively (Denlinger, 1985, Denlinger, 2022); however, our understanding of the connection between the internal clock and the endocrine system is critically lacking or limited to circadian influences on the hormone gland size, hormone titer, hormone receptor, and enzyme activities for hormone synthesis (Rensing et al., 1965; Vafopoulou and Steel, 1996, 2006; Bembenek et al., 2005a). Many types of environmental signals affect both the clock and the endocrine system, such as photoperiod, temperature, social stress, nutritional condition, and infection. Also, photoperiodism controls the manifestation of different phenotypes, not only diapause/non-diapause but also polyphenism, reproduction, behavior, and pigmentation. Alternative phenotypes are combined through some sorting mechanism to form a set of short-day type or long-day type. The former, for example, undergoes diapause, stops feeding, and becomes stress tolerant, while the latter continues feeding, reproducing, and migrating. Collectively, such a sorted collection of short-day or long-day phenotypes is called diapause syndrome (de Wilde, 1969).
Nutritional conditions are continuously monitored in terms of neural (Khan et al., 1983) or endocrine feedback (Mikani et al., 2015). For example, in the Colorado potato beetle (Leptinotarsa decemlineata), starvation neurally suppresses the corpus allatum to reduce juvenile hormone synthesis (Khan et al., 1983). Intensive cross-talks among neurotransmitters, neuropeptides, and lipid factors such as JH and ecdysteroids have been documented in various species. For example, in the American cockroach (Periplaneta americana), starvation upregulates the synthesis and secretion of short neuropeptide F (sNPF), which in turn downregulates the synthesis and secretion of crustacean cardioactive peptide (CCAP) via an autocrine loop (Mikani et al., 2015). sNPF shuts down JH synthesis, whereas CCAP upregulates it. These peptides as well as indolamines regulate two JH-synthesizing enzymes (Kamruzzaman et al., 2020). It was also shown that the indoleamines are under the control of arylalkylamine N-acetyltransferase (aaNAT), which is encoded by the clock-controlled gene (ccg) aaNAT (see later for detail). So, various factors are interacting at different levels.
Photoperiodism was first recognized in the animal kingdom in morph determination in aphids by Marcovitch (1924) and in voltinism shift in the silkworm (Bombyx mori) by Kogure (1933) and then later in reproduction and embryonic development in spider mites by Bondarenko (1950), Lees (1950), Miller (1950), and Gasser (1951). Phenomenological characterization and the basic nature of insect photoperiodism and diapause were well formulated in the iconic book “Photoperiodism and Seasonal Development in Insects” by Danilevskii (1965), first published in 1961 in Russian. The ecological aspects of diapause and seasonal adaptations in insects and mites were also collected in several textbooks and reviews (Andrewartha, 1952; Lees, 1955; Tanaka, 1950a, Tanaka, 1950b, Tanaka, 1950c, Tanaka, 1951; Beck, 1968; Veerman, 1985; Tauber et al., 1986; Danks, 1987; Bradshaw and Holzapfel, 2009; Emerson et al., 2009). Debates among scholars have focused on the nature of photoperiodic clocks, that is, endogenous oscillator-based vs. hour-glass timer, prompting the development of several empirical models for photoperiodic time measurement including the Bünning hypothesis (Bünning, 1936, Bünning, 1958, Bünning, 1960), the external coincidence model (Pittendrigh, 1966, i.e., a more elaborate form of the Bünning hypothesis), and several types of internal coincidence models (i.e., double-oscillator type: Tyshchenko, 1966; Danilevsky et al., 1970; multi-oscillator type, e.g., resonance model, Pittendrigh, 1972; a model based on interactions of multiple oscillators, Pittendrigh, 1981); this phase of studies has been well documented by Saunders (2002), Beck (1980), and Zaslavski (1988). These models are based on the assumption that photoperiodic time measurement is a function of circadian oscillations. However, Lees (1953), Lees (1973) observed the hour-glass type of responses in the European red mite [Metatetranychus (=Panonychus) ulmi] and the vetch aphid (Megoura viciae). Takeda and Masaki (1976) and Takeda (1985) also observed hourglass-type responses to non-diel photoperiods and night interruptions with various lengths of light–dark cycles in the Indian meal moth (Plodia interpunctella) and the Southwestern corn borer moth (Diatraea grandiosella), whereas in the cabbage butterfly (Pieris brassicae) and the ground cricket (Pteronemobius fascipes), both circadian and hourglass-type responses were observed (Dumortier and Brunerius, 1977; Claret, 1985; Takeda, 1986; Dumortier, 1994). Claret and Arpagaus (1994) have provided a double hour-glass model for ichneumonid wasps.
These models were formulated in rather simplistic ways to explain a set of particular circadian and photoperiodic data in a model species, but model makers have been tempted to construct more overarching models to explain a broader range of data and species. For example, Tyshchenko (1966) and Danilevsky et al. (1970) constructed a double-oscillator model that postulated that diapause incidence depended on the overlap of the active phase of two oscillators, one phase-fixed to dawn and the other to dusk. By introducing a latent period sensitive to temperature or natural selection, the model became able to explain the observed variability in photoperiodic response curves at different temperatures in different species. This model was unique because the identity of each oscillator was sought to two types of oscillators regulating eight-hour spontaneous firing in the ventral nerve cord of the pine-tree lappet moth (Dendrolimus pini). These oscillators are localized; one in the brain and the other in an unknown site posterior to the neck ligation, and one phase-locked to light-on and the other to light-off.
Subsequently, the scotonon–photonon model by Truman (1971) attempted to concurrently explain post-diapause eclosion behavior and photoperiodic activation of pupal diapause in A. pernyi on the same oscillator phased to dusk, with the kinetics called scotonon. The scotonon starts its synthetic phase upon light-off. After reaching the hypothetical peak, another decay reaction follows with different kinetics. The retention of this reaction depends on the arrival of dawn, which starts another kinetic decay called the photonon. Interactions of this oscillator and the time zone of forbidden eclosion were postulated to determine diapause/non-diapause fate. This was an endocrinological model because prothoracicotropic hormone (PTTH)/20-hydroxyecdysone, eclosion hormone (EH), and the circadian oscillator can be handled as concrete endocrine subjects that are experimentally testable. Eclosion hormone release was postulated to depend on 20-hydroxyecdysone clearance and the gate opening allowed by the circadian system. The EH release is gated in the tobacco horn worm (Manduca sexta) where the major Zeitgeber is the temperature cycle (Truman, 1984). EH release depends on the clock gate of the G-system and ecdysteroid titer that is regulated by another circadian oscillator (E-system). This system behaves like the coupled oscillator hypothesis formulated in the fruit fly (Drosophila pseudoobscura) and showed six transient cycles, although this was formulated to explain eclosion rhythm and not intended to explain photoperiodism in this species. Truman considered that the E-system probably resided in the prothoracic gland. These neuroendocrine models provided better accessibility to the physiological reality of the photoperiodic system, but “the active phases,” “inhibitory zone,” or “circadian gating” are yet to be defined.
The next model of this type, which was highly speculative, was the dual system theory (DST) proposed by Beck (1974a), Beck (1974b), Beck (1975), Beck (1976a), Beck (1976b), who postulated the interaction of two oscillators, each engaged with dawn and dusk, respectively, having photonon and scotonon kinetics. By manipulating parameters, Beck was able to explain a variety of photoperiodic response curves, temperature modifications, and thermoperiodic response curves as well as the circadian gate. This type of model making was succeeded by Vaz Nunes and Veerman (1979a), Vaz Nunes and Veerman (1979b). Here, almost everything we know about circadian and photoperiodic reactions and photoperiodic summation was explained; however, as the number of assumptions and hypotheses increased, physiological realities faded out, and critical examination via experiments became more difficult. However, revelation came from the molecular biology of the circadian clock. Here, a new model based on identified circadian parameters with respect to circadian genes is awaited. It would have been exciting if we had new Vaz Nunes–Veerman models based on the recent molecular data
Input Pathway of the Photoperiodic Clock
The locus of the photoperiodic clock and its photoreceptor were examined in L. decemlineata (de Wilde and Bonga, 1958) and A. pernyi (Williams and Adkisson, 1964) by illuminating either the head or the abdomen and in M. viciae (Lees, 1964) by illuminating small patches of the head. In these experiments, the central part of the brain was found to be important, whereas the abdomen, thorax, and compound eyes were found to be unimportant.
Transplantation is a powerful means of examining the locations of the photoperiodic clock and photoreceptors. Williams and Adkisson (1964) showed that the graft of the brain contained the clock in A. pernyi. Bowen et al. (1984) confirmed that the isolated brain allowed photoperiodic determination of diapause in M. sexta. Truman (1971) used this approach to examine the eclosion clock in two silk moths, A. pernyi and the cecropia moth (Hyalophora cecropia), which showed the peak of eclosion at dusk and dawn, respectively. The host was debrained of its proto- and tritocerebrum and transplanted with the central brain of the other species (i.e., A. pernyi host with H. cecropia central brain and vice versa), and it was found that the host emerged at the time according to the graft (Truman, 1971); these findings confirmed those of Williams (1969).
The sensory systems for perception of the photoperiod and temperature were investigated in some insect species and mites in terms of action spectra (de Wilde and Bonga, 1958; Hayes, 1971; Lees, 1981; Suzuki et al., 2008a; Ikeno et al., 2010) and, more recently, in terms of the low temperature-sensing cation channel transient receptor potential A1 (TRPA1) (Sato et al., 2014). Photoreceptor pigments, which serve as a photoperiodic input system, were studied by Veerman and his colleagues in their studies with carotenoid feeding and albino mutants in mites.
Carotenoids are a class of tetraterpenoids that have a C40 structure consisting of eight isoprene units and function not only as coloration pigments and antioxidants but also as photoreceptor pigments. The first evidence of carotenoid involvement in the photoperiodic induction of insect diapause was obtained from a dietary experiment in D. grandiosella (Takeda, 1978). Additional evidence was provided by dietary studies on the photoperiodic induction of diapause in B. mori (Shimizu et al., 1981) and the predatory mite (Amblyseius potentillae = A. andersoni) (Van Zon et al., 1981; Veerman et al., 1983). Although predatory mites lack eyes, reproductive diapause is clearly induced by long-night photoperiods experienced during the immature stages (McMurtry et al., 1976), suggesting that extraretinal photoreceptors function as an input system for photoperiodism. No induction of diapause occurs, even under long-night photoperiods when the predatory mites A. potentillae and A. cucumeris are fed on albino spider mite eggs or broad bean pollen, which are almost devoid of carotenoids (Van Zon et al., 1981; Veerman et al., 1983). However, supplementation of these carotenoid-limited diets with β-carotene, 3-hydroxyechinenone, or vitamin A acetate (retinol acetate) allows photoperiodic induction of diapause to occur, although supplementation with astaxanthin and vitamin A acid (retinoic acid) does not (Van Zon et al., 1981; Veerman et al., 1983). These studies indicate that pigments derived from vitamin A and its precursors (provitamin A) are essential for the perception of the photoperiod. This also suggests that opsin apoproteins binding the chromophore retinal, a vitamin A derivative, function as extraretinal photoreceptors in predatory mites.
The eyes in the two-spotted spider mite (Tetranychus urticae) are red due to accumulation of carotenoid pigments; however, albino mutants lack eye pigmentation (Ballantyne, 1969; Veerman, 1974). The genes responsible for albinism in spider mites include carotenoid biosynthesis genes that were horizontally transferred from fungi (Bryon et al., 2017; Wybouw et al., 2019). In T. urticae, diapause incidence in albino mutants is significantly lower than that in wild-type under long-night photoperiods (Veerman and Helle, 1978; Veerman, 1980). In addition, the albino mutants enter diapause under long-night photoperiods after feeding on diets containing vitamin A and β-carotene (Bosse and Veerman, 1996). This suggests that opsin-type photoreceptors utilizing retinal as a chromophore are required for the perception of the diapause-inducing photoperiod in this species. Photoperiodism in T. urticae is observed not only for diapause induction but also for diapause termination (Veerman, 1977a). Although this species is less responsive to visible light during diapause, it is not totally blind (Suzuki et al., 2009a, 2013), and diapause termination is enhanced under short-night photoperiods after chilling for several months compared to long-night photoperiods or continuous darkness. Hori et al. (2014) removed the eyes from diapausing adults by using a laser ablation technique and evaluated their diapause termination. Removal of all eyes that consisted of two pairs of ocelli (Mills, 1974) prevented diapause termination even under short-night photoperiods, indicating that these eyes function as a photoperiodic input system. Whole-genome sequencing of T. urticae (Grbić et al., 2011) has revealed the presence of putative genes for ultraviolet-sensitive opsin, long-wavelength–sensitive opsin, and peropsin.
In addition to opsin-type photoreceptors involved in the photoperiodic clock that most probably operates as a non-circadian hourglass for measuring the night length of photoperiods, another type of photoreceptor has been suggested in T. urticae that is required for entrainment of the circadian system involved in photoperiodic time measurement (Veerman, 2001). The Nanda–Hamner experiment (Nanda and Hamner, 1958) revealed that the internal clock involved in photoperiodic time measurement resonated with the length of one light–dark cycle (T) at an interval of 20 h (Veerman and Vaz Nunes, 1980). Under long-night photoperiods with T = 20 h (LD = 8:12-h), diapause was induced even when the illumination provided was orange-red light (>580 nm). Under long-night photoperiods with T = 24 h (LD = 12:12-h), which is a diapause-inducing condition when the illumination is provided with white light, no diapause was induced with orange-red light (Veerman, 2001). This suggests that in T. urticae, when T is identical to the internal periodicity (20 h) of the clock like an oscillator, diapause is induced only by measuring the length of long-night photoperiods via a photoperiodic clock connected to opsin-type photoreceptors, which are sensitive to a broad range of wavelengths including orange-red light. In contrast, circadian entrainment by photoreception may be required for photoperiodic time measurement at T = 24 h, but this process does not occur under orange-red light and diapause is not induced even under long-night photoperiods, suggesting that the photoreceptors involved in the circadian system are sensitive only to short-wavelength light. One of the candidate photoreceptors is Drosophila cryptochrome (Cry-d), a photoreceptor protein coupled with a flavin chromophore, which is sensitive to ultraviolet-A and blue light and insensitive to light with a wavelength greater than 500 nm (Stanewsky et al., 1998). Indeed, an orthologous gene of cry-d is present in the genome of T. urticae (Grbić et al., 2011). Reverse genetic approaches such as RNA interference (RNAi) and genome editing, which have been shown to be effective in T. urticae (Suzuki et al., 2017; Dermauw et al., 2020), will help to clarify the functions of these candidate photoreceptor genes in photoperiodic time measurement and circadian entrainment.
Overview of the Circadian System in Drosophila melanogaster
It has already been half a century since Konopka and Benzer (1971) identified period (per) as the first circadian gene from the fruit fly (D. melanogaster). per appeared to encode a transcription modulator, but the protein lacked a DNA-binding domain. Per had heterodimerization domains shared not only by Per but by aryl hydrocarbon receptor nuclear translocator (Arnt) and single-minded (Sim). They are now called Per-Arnt-Sim (PAS) domains. Searches in silico have revealed several dimerization partners including cycle (cyc)/Bmal1 (brain-muscle Arnt-like 1) and Clock (Clk). timeless (tim) was retrieved from a forward genetic mutation search (Sehgal et al., 1996), which encodes a direct partner of Per.
Clk and cyc form a heterodimer that possesses a DNA-binding domain with a basic helix-loop-helix. Per forms a heterodimer with Tim that is photosensitive for degradation by ubiquitin-mediated proteasome. Cry-d is photosensitive. Per degradation is mediated by double-time (Dbt), a casein kinase Iε, which was also identified from a mutation search (Price et al., 1998). Clk/Cyc heterodimer binds an E-box to activate transcription of per and tim. After transcription, the resulting proteins form a heterodimer, which stabilizes both proteins. After dimerization, the heterodimer translocates to the nucleus where it interrupts Clk/Cyc from binding to the E-box of per and tim. This completes a negative feedback loop.
Additional genes that contribute to the clock function and its stability have been subsequently identified. Clk and per mRNA are produced in antiphase, which indicates a coupling between Clk and PAR domain protein 1 (Pdp1)/Vrille (Vri) loop (Cyran et al., 2003). E-boxes function as a key for the circadian system to interact with a clock-controlled gene (ccg). The pdp1 and vri genes have E-boxes, and therefore they are ccg. Clk has a Pdp1/Vri box, and therefore Clk also becomes a ccg. Thus, these two loops become interlocked (i.e., become an interlocked negative feedback loop). Pdp1 and Vri regulation via Pdp1/Vri box are antagonized by one another, which could provide one of the fundamentals of circadian rhythms, that is, temperature compensation.
Organisms must consist of multiple oscillators. The phase relationship (Ψxy) among constituent oscillators (…x … y … ) may form a hierarchical system with strong or weak coupling. When the coupling is weak, each rhythm behaves in an independent manner. In some cases where the coupling is strong, the subordinate oscillator behaves as a slave and the other oscillator like the pacemaker (circadian pacemaker, CPM), as adopted in Pittendrigh’s coupled oscillator hypothesis (Pittendrigh et al., 1958; Pittendrigh, 1960). This hypothesis was formulated based on the eclosion rhythm of the fruit fly, D. pseudoobscura, particularly to explain transient cycles after reset by a brief light pulse placed at different circadian times and the difference between post-reset rhythms by light and temperature Zeitgebers, although D. melanogaster does not display transient cycles. Nevertheless, the biological clock must control several rhythms, each controlled by a slave oscillator having a different ΨrL, as shown in the pink bollworm (Pectinophora gossypiella) (Pittendrigh and Minis, 1964, Pittendrigh and Minis, 1971). To stabilize steady-state ΨrL’s, E-box–mediated transcription is a good tool to adjust different rhythms graded by a common negative regulator. The clockwork orange (CWO) protein may play this role, which competes with other transcription modulators for E-box binding, something like a general music tempo indicator like sempre piano or forte. For example, the free-running rhythm of cwo transcript of the tobacco cutworm moth (Spodoptera litura) was strong in continuous darkness, whereas per and tim transcript rhythms were quickly damped out in continuous darkness (Zhang et al., 2021). Depending on the species and situation, the leader can be either Per, Clk, or CWO (Tomiyama et al., 2020), as was nicely shown in a study of CWO in the cricket (Gryllus bimaculatus).
In the negative feedback system, two state variables rely upon each other to make oscillatory trajectories showing a limit cycle. Translated proteins must decay in terms of photo-oriented degradation or through a proteasome or some other mode. Per degradation is aided by the phosphorylation pathway by Dbt and Tim degradation by Cry, which was originally identified in Arabidopsis (Lin and Shalitin, 2003) and Shaggy, respectively, which stimulates ubiquitination and the proteasome pathway for degradation (Sehgal et al., 1996). Cry has different functions in different species: Cry-d is a blue-sensitive photoreceptor protein, whereas mammalian-type Cry (Cry-m) dimerizes with Per and makes a nuclear translocation with Dbt (Lin et al., 2002).
Variation and Conserved Structure of the Circadian System in Species Other Than D. melanogaster
D. melanogaster was used by T. H. Morgan and many other Nobel laureates, including J. C. Hall, M. Rosbash, and M. W. Young, for elucidating the intricacies of the biological clock. It is indeed surprising to see that the structure and genes are conserved between insect clocks and vertebrate clocks, including that in humans. Contrasting to such a high constancy, two Drosophila species, D. melanogaster and D. pseudoobscura, share the basic structure but have some differences. The threonine–glycine repeat that was once considered as a landscape structure in D. melanogaster Per is lacking in D. pseudoobscura Per. A similar situation has been found in the cellular structure in two closely related cricket species, Dianemobius nigrofasciatus and Allonemobius allardi (Sehadová et al., 2006; Shao et al., 2006, Shao et al., 2008a, Shao et al., 2008b). Many insects such as the honey bee (Apis mellifera) lack tim (Rubin et al., 2006). Two lark transcripts are present in B. mori (Iwai et al., 2007). Bmal1 rather than Cyc is found in the monarch butterfly, Danaus plexippus (Zhang et al., 2017), although the lepidopteran homolog is phylogenetically remote from mammals than from flies. In contrast to Drosophila, Tim does not play a role as a dimerization partner in humans where the partner is Cry-m. Many of the lepidopterans such as A. pernyi, D. plexippus, and B. mori have both cry-d and cry-m genes. The genome of T. urticae (Grbić et al., 2011) contains per, tim, cyc, Clk, cry-d, and cry-m genes, but their functions remain unclear.
Melatonin is produced by many organisms—unicellular, multicellular, vertebrates, invertebrates, fungi, and plants. However, its roles in circadian and photoperiodic regulation and metabolic pathways are basically shared but sometimes vary among taxa (Hiragaki et al., 2015 for a review focused on aaNAT). Melatonin is indispensable for generating circadian rhythmicity in locomotor rhythm in P. americana (Kamruzzaman et al., 2021) and photoperiodic regulation of pupal diapause in A. pernyi (Wang et al., 2013; Mohamed et al., 2014), but the melatonin synthetic pathway must be unique in insects because their hydroxyindole-O-methyltransferase activity is very weak (Hiragaki et al., 2009). Also, in bony fish, the salmoniform fishes lost circadian regulation of pineal secretion of melatonin, whereas the osmeriform fishes show a circadian pattern in melatonin secretion. Thus, phylogenetic modification must be extensive in photoperiodism regulation.
Ambient Temperature and Temperature Step-Up or -Down Can Affect the Circadian System and Photoperiodism
In formulating the coupled oscillator hypothesis, Pittendrigh et al. (1958) intended to explain two peculiar behaviors observed in the pupal eclosion rhythms of the fruit fly, D. pseudoobscura: 1) the first seven cycles after a light pulse placed at different circadian times before the entrained oscillator establishes a new steady-state and 2) when phase shifts are forced to occur due to changes in light cycle and temperature, the eclosion peak immediately followed the new temperature regimen but slowly caught up with the light regimen. Pittendrigh et al. (1958) concluded that the eclosion clock consists of two coupled oscillators, one behaving as a CPM (or oscillator A) and the other as a slave (or oscillator B). The phase reference point, a phase with a visible marker (such as eclosion peak), resides on the slave. The CPM immediately obtains a fixed ΨrL to the light and the slave keeps up with the CPM. The slave regains the original ΨBA to the CPM within seven cycles. In this sense, temperature is a determinant of circadian phasing. A similar behavior has been observed in the M. sexta eclosin clock (Truman, 1984) as discussed earlier.
It is not only circadian rhythms that are affected by temperature; photoperiodic response curves are also affected by ambient temperature and by temperature cycles and different thermoperiods (Saunders, 1973). Critical daylengths across that diapause incidence dramatically changes are more strongly affected by temperature (Danilevskii, 1965; Pittendrigh and Takamura, 1987) in contrast to τ, the free-running period, which is “temperature-compensated.” Thermoperiod-dependent induction of diapause is well known from the work of Veerman’s group on the predatory mite (van Houten et al., 1987; Veerman, 1992). In the predatory mite, A. andersoni, diapause is induced not only by long-night (scotophase) photoperiods but also by long-cryophase thermoperiods even under continuous darkness, with the photoperiodic response and thermoperiodic response curves showing similarities. In addition, combined treatment with photoperiods and thermoperiods revealed that the coincidence of the scotophase and cryophase most effectively induces diapause. In mites, it is well known that as temperature increases, the diapause-inducing effect of long-night photoperiods decreases (Veerman, 1985), which may be due to shortening of the period in which the mite is sensitive to the photoperiod because development is accelerated at high temperature, thereby reducing the amount of information accumulated by the photoperiod counter to induce diapause. However, the synergistic effect of the scotophase and cryophase on the induction of diapause cannot be explained only by the length of the period of sensitivity. Thus, studies are needed to investigate the dynamics of the biomolecules involved in the perception and measurement of the photoperiod and thermoperiod.
Temperature also controls the circadian rhythm by affecting the pattern of differential splicing of per transcript at the 3′ end in D. melanogaster (Collins et al., 2004). D. melanogaster is a day-active animal, and this differential splice pattern contributes to conserving this trait. However, how this aspect affects photoperiodism has not been investigated in detail.
In B. mori, temperature determines the incidence and termination of diapause more dominantly than photoperiod via a peptide, the corazonin-mediated pathway, and diapause hormone (Tsuchiya et al., 2021). The temperature signal is mediated by TRPA1. In A. pernyi, pupal diapause is terminated by long days and low temperatures (Matsumoto and Takeda, 1996, Matsumoto and Takeda, 2002; Tohno et al., 2000; Tohno and Takeda, 2001). However, at which stage of diapause the termination cascade is affected by low temperature, possibly via TRPA1, has not been investigated. Also unknown is whether the receptor resides in the clock neurons or in PTTH-secreting cells. In arthropods, the TRPA subfamily, which diverged from an ancient TRPA1 gene, has expanded (Peng et al., 2015). Although the predatory mite (Metaseiulus occidentalis = Galendromus occidentalis) also expresses TRPA1, none of the TRPA subfamily genes have been found in the genome of T. urticae (Grbić et al., 2011). This suggests that the loss of ancient TRPA1 occurred in the two-spotted spider mite and that this species is unable to directly sense temperature signals and that the rate of development, which determines the length of the stages in which photoperiodic information can be accumulated, may change with temperature and indirectly affect photoperiodic induction of diapause. Lack of TRPA1 has also been observed in the water flea (Daphnia pulex) and in Hymenopteran insects, although they have multiple other TRPA subfamily genes that may compensate for this lack (Matsuura et al., 2009).
Cross-talk partners could be involved in the circadian system, endocrine system, calcium signaling pathways, phosphoinositide pathway, or phosphorylation/dephosphorylation, or they could be endocytic elements.
Neuroendocrine Regulation of Insect Diapause and the Upstream Regulatory Structure
It was Yoshimaro Tanaka who first used non-diel photoperiodic regimens in photoperiodic analyses of pupal diapause in A. pernyi (Tanaka, 1950a; Tanaka, 1950b; Tanaka, 1950c; Tanaka, 1951). This species has since been used as a model system for examination of the hormonal regulation of diapause (Williams, 1969; Truman, 1971). A. pernyi, as well as H. cecropia, has also been used as a model for examining activation of the prothoracic gland by the brain for secretion of prothoracicotropic hormone (PTTH). Other model photoperiodic species where interactions are suggested between the endocrine system and biological clock include B. mori (Sehadová et al., 2004; Iwai et al., 2006; Iwai et al., 2007; Iwai et al., 2008; Trang et al., 2006; Tsuchiya et al., 2021), two closely related cricket species (Dianemobius nigrofasciatus and Allonemobius allardi) (Shao et al., 2006; Shao et al., 2008a; Shao et al., 2008b; Izawa et al., 2009), the bean bug (Riptortus pedestris) (Ikeno et al., 2008), and the lindenbug (Pyrrhocoris apterus) (Doležel et al., 2007), where the colocalization of clock and related gene products and neurohormones, cloning of clock and related genes, and RNAi are conducted. The interference with some of the circadian gene impaired photoperiodism but this simply implies the circadian system is a photoperiodic component but does not show how so.
Williams and Adkisson (1964) and Williams (1969) identified that the brain is the anatomical site that regulates, photoperiodically, pupal diapause of A. pernyi for both initiation and termination in such a way that short-day photoperiods initiate and maintain diapause and long-day photoperiods terminate diapause or block diapause initiation. Long-day photoperiods induce PTTH secretion in the brain, which in turn activates the prothoracic gland to secrete ecdysone. Ecdysone is mono-oxygenated in the midgut and other peripheral organs to 20-hydroxyecdysone, a molting hormone, which triggers metamorphosis in the rising phase, but in the falling phase with the circadian gate open, it determines the release of eclosion hormone to trigger eclosion behavior (Truman, 1971).
RIA measurement showed that about 10 cycles of long-day activation of diapause pupae of A. pernyi induced the secretion of ecdysone into the hemolymph (Matsumoto and Takeda, 2002; Mohamed et al., 2014). Not only long days but also a long exposure of about 2 months to a low temperature of 5°C activated the brain (Tohno et al., 2000; Tohno and Takeda, 2001). Temperature and photoperiod regulate the brain independently because an insufficient number of cycles at low temperature and long-day photoperiod produces post-diapause emergence peaks in an independent rather than additive manner (Tohno et al., 2000; Tohno and Takeda, 2001), meaning that thermal activation and photoactivation are different physiological processes.
To examine what initiates PTTH secretion, we investigated brain monoamine content during long-day activation by means of electrochemical detection coupled with high-performance liquid chromatography (Matsumoto and Takeda, 2002) and found that serotonin content rose when ecdysone content rose. The data suggested that serotonin or its downstream products caused PTTH content to increase. We then examined the indolamine pathway leading to melatonin formation by means of radioenzymatic assay, focusing on aaNAT in the activation process. We found that the enzymatic activity of aaNAT was increased both by long-day photoperiod and by low temperature (Matsumoto and Takeda, 2002). Injection of melatonin into diapause pupae terminated diapause. We also investigated the neuroanatomical structure of this system by means of immunohistochemistry targeting both PTTH-secreting neurons and circadian clock neurons. We used antibodies targeting Clk, Cyc, Per, PTTH, serotonin, aaNAT, hydroxyindole-O-methyltransferase, melatonin, and melatonin receptor both in adjacent sections and by simultaneous staining (Mohamed et al., 2014). Both examinations showed clearly that clock neurons (Sauman and Reppert, 1996a) and PTTH neurons (Sauman and Reppert, 1996b) were juxtaposed in the dorsolateral protocerebrum and that the cell bodies were physically in contact.
Circadian clock neurons showing dcyc-ir, PaPer-ir, and BmDBT-ir have melatonin-producing machinery showing serotonin-ir, PaaaNAT-ir, hydroxyindole-O-methyltransferase-ir, and melatonin-ir, while PTTH-secreting neurons have melatonin receptor (hMT2)-ir. We have documented by RIA that the melatonin content in the brain of A. pernyi showed day/night fluctuation under light–dark cycles of both LD = 16:8-h and 12:12-h. Melatonin content peaked at 4 h after light-off under both cycles. In addition, both the peak and baseline values were higher under the 16:8-h cycle than under the 12:12-h cycle, a photoperiodic response. This seems reasonable because long days terminate diapause or activate the brain. Melatonin injection and melatonin coincubation with the brain in vitro stimulate the brain in vitro to secrete PTTH in P. americana (Richter et al., 1999) and A. pernyi (Mohamed et al., 2014).
Injection of the melatonin receptor antagonist luzindole blocked diapause termination in A. pernyi under long days, and RNAi against aaNAT also blocks long-day effects (Wang et al., 2013; Mohamed et al., 2014). Melatonin-spiked water rescues arrhythmicity caused by dsRNAaaNAT in P. americana (Kamruzzaman et al., 2021) and the house cricket (Acheta domesticus) (Yamano et al., 2001). These findings strongly suggest that the melatonin system is operative in insects as it is in vertebrates, mediating the communication between the circadian clock and endocrine system (Wood and Loudon, 2014) and that aaNAT serves as a “timezyme” (Klein, 2007).
aaNAT Is a Clock-Controlled Gene
All of the known instruments to produce melatonin exist within clock neurons (Mohamed et al., 2014; Wang et al., 2013). PTTH-secreting neurons express melatonin receptor-ir. This suggests that melatonin released by clock neurons induces the release of PTTH in a clock-controlled manner. aaNAT in insects exhibits high activities and plays important roles in various physiological regulations including neurotransmitter metabolism, cuticle formation, reproduction, and midgut function (Hiragaki et al., 2015). The aaNAT gene was first cloned in vertebrates, and it was found that it is a critical component of the downstream regulatory pathway in such a way that it is a clock-controlled gene; it possesses multiple E-boxes to which Bmal1/Clk heterodimer binds to stimulate the transcription of aaNAT, which increases the activity of aaNAT enzyme, catalyzing the synthesis of melatonin (Coon et al., 1995; Borjigin et al., 1995). We cloned two types of aaNAT from P. americana (Ichihara et al., 1997; Ichihara et al., 2001; Bembenek et al., 2005a; Bembenek et al., 2005b), B. mori (Tsugehara et al., 2007), and A. pernyi (Tsugehara et al., 2013). Melatonin secreted from the clock neurons binds to the putative melatonin receptor on the cell surface of PTTH-secreting neurons, which stimulates Ca2+ influx. This influx stimulates PKC and activated PKC phosphorylates Rab8 to induce exocytosis of PTTH, leading to diapause termination (Hiragaki et al., 2009), because all PTTH-ir neurosecretory cells showed protein kinase C-ir and Rab8-ir. The latter two reactivities have a wider distribution, but all PTTH cells share these reactivities (Hiragaki et al., 2009). We confirmed this model by using luzindole and several indoleamines as well as RNAi against per, cyc (Bmal1), aaNAT, and two genes encoding 5-hydroxytryptamine (serotonin) receptor (5HTR1A and 5HTR1B) (Hiragaki et al., 2008). When entering diapause under short days, aaNAT is not expressed, and therefore, melatonin synthesis does not occur while 5HT is accumulated, which enhances serotonin-binding to 5HTR1B (Wang et al., 2013). This prevents PTTH release, resulting in diapause initiation and maintenance. 5HTR1B transcript decreased when pupae were exposed to long days, while 5HTR1A was not sensitive to the photoperiod. Also, RNAi only reversed the function of the type 1B receptor, not that of the type 1A receptor (Wang et al., 2013). Injection of dsRNA5HTR1B induced termination of diapause even under short days.
An equivalent mode of pupal diapause regulation was found in the fall webworm (Hyphantria cunea). This species has a long-day–type response both in diapause induction and termination. The injection of dsRNAper failed to maintain diapause even under short days, LD = 14:10-h (Yang et al., 2017a). Since Per is a negative regulator of E-box binding in aaNAT, knockdown of per should enhance E-box binding and therefore increase melatonin synthesis, as seen in A. pernyi. Thus, the aaNAT regulation hypothesis based on the circadian system is not valid only in A. pernyi.
Downstream processes are integrated in a rather simple mode, but several important features remain unsolved. Temperature control is an important aspect of photoperiodism, and TRPA1 may provide an important clue to this research. We have shown the presence of another aaNAT and another serotonin receptor (i.e., aaNATB and 5HTR1A, respectively) in clock neurons in the brain of A. pernyi. These may be involved in temperature regulation of diapause in some way. We propose here a possible representation of the neuroendocrine system responsible for the photoperiodic time measurement and diapause determination in A. pernyi (Figure 1). It summarizes our current understanding of photoperiodic regulation of A. pernyi pupal diapause. The circadian and photoperiodic systems are complex. Cross-talks with other neuroendocrine organs must occur to accommodate non-photic stress adaptations to social stress, nutritional condition, desiccation, and extreme temperature. Recently, we have shown extensive cross-talks between neurotransmitters, neurohormones, neuropeptides, and juvenile hormone and ecdysteroids in the regulation of reproduction in P. americana (Kamruzzaman et al., 2020). Feeding was found to induce crustacean cardioactive peptide (CCAP) synthesis, which modulates locomotor activity, allatotrophe, and ecdysiotrophe. CCAP neurons are located at the CPM locus of the ventral optic tract in P. americana. Short neuropeptide F (sNPF) is secreted from CCAP neurons when cockroaches are starved, downregulating juvenile hormone synthesis. Indolamines also control allatotrophe. Melatonin is required for locomotor rhythm; RNAi against aaNAT made the locomotor activity arrhythmic but melatonin injection rescued the rhythm (Kamruzzaman et al., 2021).
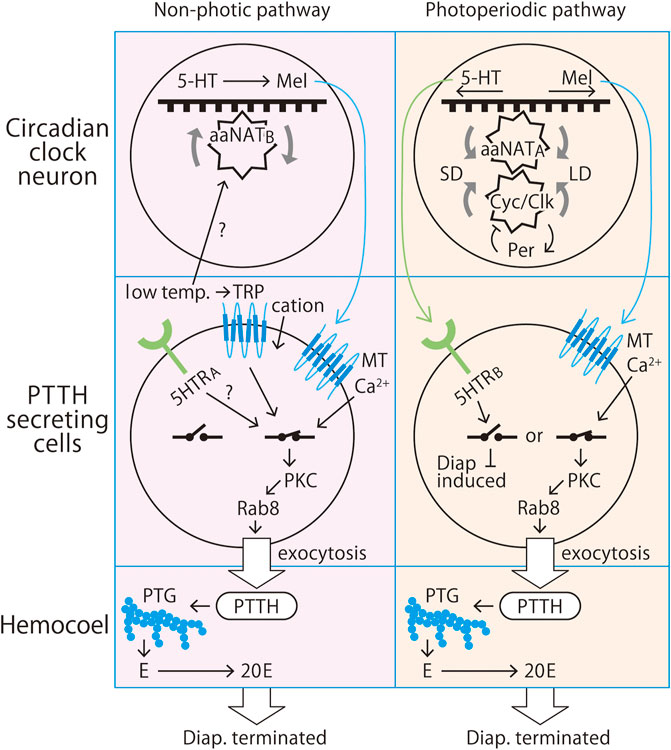
FIGURE 1. Proposed non-photic and photoperiodic pathways that control pupal diapause in the Chinese tussar moth, Antheraea pernyi (Lepidoptera: Saturniidae). Both pathways are based on circadian clock-driven regulation of arylalkylamine N-acetyltransferase (aaNAT) as an endocrine conjunct between the clock and the secretagogue prothoracicotropic hormone (PTTH). The non-photic pathway responds to temperature rather than light. In the photoperiodic pathway (right), long-day (LD) conditions prevent or terminate diapause, whereas short-day (SD) conditions induce or maintain diapause. In both the non-photic and photoperiodic pathways, metamorphosis is driven by 20-hydroxyecdysone derived from 20C monooxygenation of ecdysone (E) secreted by the prothoracic gland (PTG) under stimulation by PTTH released from dorsolateral neurosecretory cells colocalized with circadian clock neurons. The clock operates based on an interlocked transcription/translation coupled negative feedback loop consisting of the genes period, timeless, cycle, clock, vrille, PAR domain protein 1, cryptochrome, double-time, clockwork orange, sgg, and lark. The clock neurons are equipped with a melatonin-synthesizing enzyme complex such as aaNAT and hydroxyindole-O-methyltransferase as well as their substrate indoleamines. aaNAT is a clock-controlled gene that has cis element enhancer E-boxes in its upstream promotor region, which is where the cycle/Clock (cyc/Clk) heterodimer binds. This machinery synthesizes melatonin from 5-hydroxytryptamine (5HT; serotonin). In the photoperiodic pathway, aaNAT transcription under circadian control is stimulated at night and under long-day conditions. PTTH-secreting cells express melatonin receptor type 2 (MT2). Melatonin binding to this receptor opens Ca2+ channels in the cell membrane, which increases the intercellular Ca2+ concentration. The influx of Ca2+ activates protein kinase C (PKC) for the phosphorylation of Rab8, which in turn drives exocytosis of secretary granules containing PTTH to the hemocoel. The released PTTH stimulates the PTG to release ecdysone, a prohormone of the molting hormone 20-hydroxyecdysone (20E). 20E makes peripheral cells commit to metamorphosis or apoptosis. Under short-day conditions, the aaNAT transcription level is low, and therefore, melatonin synthesis is also low, which results in the activation of 5HT receptor type B, which opens the endocrine switch, resulting in the induction or maintenance of diapause. In the temperature-based pathway (left), diapause is terminated after about 2 months of exposure to low temperatures. This process is likely independent of the photoperiodic process, and it is possible that another type of aaNAT, aaNATB, is expressed in clock neurons and another type of 5HTR, 5HTRA (=5HTR1A), is expressed in PTTH-secreting cells. How this pathway increases or decreases aaNAT expression under a particular photoperiod or temperature remains unknown, and it is difficult to solve multi-oscillator equations due to the large numbers of genes and proteins involved.
We have also shown that topical application of methoprene (ZR-515), a juvenile hormone mimic, causes a phase shift in eclosion rhythm of D. grandiosella (Yin et al., 1987). In A. pernyi, the dorsolateral neurons expressing Per-ir innervate the corpus allatum (Sauman and Reppert, 1996a). Many of the circadian clock genes encode PAS proteins, including a juvenile hormone receptor, Met. It may interact with other PAS proteins among all clock proteins such as cry, Clk, and cyc, which has been shown in P. apterus (Bajar et al., 2013). The circadian gene take-out is a homolog of juvenile hormone binding protein (Sarov-Blat et al., 2000; Saito et al., 2006).
A GABA receptor subunit, RDL (resistance to dieldrin)-ir, is located at the circadian clock locus of the optic tract of P. americana (Sattele et al., 2000), and GABA is involved in diapause regulation in B. mori (Tsuchiya et al., 2021). The functions of GABA in the circadian and photoperiodic systems are yet to be investigated.
Organisms are most likely multi-oscillatory systems where weak interactions are probably more commonplace. Recent molecular elucidation is depicted as the interlocked negative feedback loops; the system is interlocked with many redundancies to stabilize the circadian system. The system is standing solid in the storms of perturbations under various environmental conditions in this way. The second loop (Pdp1/Vri loop) is to accommodate nutritional stress conditions and temperature fluctuations.
Photoperiodic Summation or Counter
How organisms accumulate measurements of day or night length is one of the most interesting questions in photoperiodic studies. As Danilevskii (1965) noted, diapause stage and sensitive stage for photoperiodic determination are, in most cases, separated in time. He even used this for the distinction of diapause from quiescence. The isolation is, in extreme cases, transgenerational, as it is in B. mori (Kogure, 1933) and M. viciae (Lees, 1959, Lees, 1960).
Gibbs (1975) has proposed that some hypothetical substance is accumulated at each photoperiodic measurement and that if the total amount accumulated (“diapause titer”) in a fixed period of sensitivity surpasses the determination threshold, the developmental fate is determined. Saunders (1981) extended this proposal to explain that the incidence of diapause depends on two parameters, the required day number (RDN) of the inductive cycle fixed by the photoperiod and the sensitive period. If the RDN is reached during the sensitive period, the diapause program is selected. The sensitive period is observable, and the RDN is derived from the days when the individuals destined for non-diapause development reach 50%. This explanation fits nicely with the obtained data, but it may be tautological because the RDN is not defined in any physiological reality. Indeed, we raised D. grandiosella larvae under mixed cycles of short days and long days on different artificial diets, and our results did not follow the above prediction; instead, the incidence of diapause depended on the larval period, even when the number of short days was less than the number of long days (Takeda, 1978).
The neuroendocrine system resembles a hydraulic system with an hourglass nature. Endocrine substances such as melatonin takes time to accumulate via synthetic pathways, and it is particularly so when the system is constructed as an interlocked negative feedback loop. In this context, it is interesting to see that the number of terminating cycles under long days and that at low temperature are not additive; the cycle number was 10 under long days but 2 months, that is, 60 cycles, at low temperatures (Tohno et al., 2000; Tohno and Takeda, 2001; Matsumoto and Takeda, 2002).
Reproduction and diapause of T. urticae are predestined by experiencing several short and long nights, respectively, during the sensitive period (Suzuki and Takeda, 2009). The rationale for measuring night length rather than day length in examining the photoperiodism of T. urticae is that diapause is induced under light–dark cycles of LD = 8:16-h and 6:16-h but not 16:8-h or 8:8-h (Veerman and Vaz Nunes, 1987). In addition, because no diapause is induced under continuous darkness (Veerman, 1977b; Veerman and Vaz Nunes, 1987; Suzuki et al., 2008a), light plays a role as a “frame” to determine the night length and to create multiple long-night photoperiods to ensure that diapause is not induced by a single long-night photoperiod, which may occur even in a season suitable for reproduction as a result of weather conditions and microhabitats.
The molecular mechanism of the photoperiodic clock and counter system in T. urticae are still largely unknown, but recent innovations in omics technology have provided a useful means of analysis. Transcriptome and proteome analyses have revealed that several neurotransmitters and their receptors, biosynthesis enzymes, and vesicular trafficking-related proteins are upregulated in association with diapause in T. urticae (Zhao et al., 2017). These include ionotropic glutamate receptors, metabotropic glutamate receptors, glutamine synthetase, glutaminase, octopamine/tyramine receptor, dopamine transporter, neuropeptide F receptor, short neuropeptide F precursor, proctolin receptor, synaptobrevin, synaptotagmin, dynamin, and frequenin. The appearance of glutamate and monoamine signaling-related factors suggests commonality with the insect photoperiodic system. Accumulation of glutamate is observed in the brain of diapause-destined larvae in the cotton bollworm (Helicoverpa armigera) (Zhang et al., 2012). In addition, Des Marteaux et al. (2022) have demonstrated functional involvement of vesicular glutamate transporter in the photoperiodic induction of adult diapause in the bean bug (Riptortus pedestris). In A. pernyi, a model has been proposed in which dopamine and melatonin function as photoperiodic counters, inducing and inhibiting the photoperiodic termination of pupal diapause, respectively (Wang et al., 2015a,Wang et al., 2015b). Dopa decarboxylase and aaNAT are rate-limiting enzymes for the synthesis of dopamine and melatonin, respectively. The genes encoding dopa decarboxylase and aaNAT have several E-box enhancer elements, which qualify them as clock-controlled genes (Adamska et al., 2016), and they may regulate photoperiodism as an output factor, a timezyme, of the circadian clock (Klein, 2007; Hiragaki et al., 2015). Genes encoding aaNAT are found in ecdysozoan genomic databases but have not been identified in those of the Acari (Hiragaki et al., 2015). Although aaNAT-like light-responsive enzyme activity has been reported in T. urticae (Suzuki et al., 2008b; 2009b), the absence of the aaNAT gene in its genome suggests that the activity of other acetyltransferases belonging to the GCN5-related N-acetyltransferase superfamily have been detected. On the other hand, the genome of T. urticae (Grbić et al., 2011) contains several genes that are annotated as encoding dopa decarboxylases. Further investigations focusing on the GCN5-related N-acetyltransferases and dopa decarboxylases are needed to clarify the timezyme in T. urticae.
Consequences of Differentiation in Circadian and Seasonal Timing in Species Status: Neuroendocrine and Clock Pleiotropy
Photoperiodism regulates the insect life cycle by seasonally separating immature stages from the reproductive stage. The timing of adult emergence and the release of sex pheromone and seasonality of the life cycle constitute a mechanism that seasonally isolates reproductive groups. Differentiation in circadian components could split reproductive population into two groups, which likely affects species status. It may split reproductive times after geographic separation as an allopatric mode or it may open for a sympatric mode to play without geographic isolation. It is tempting to assume that the latter case may lead to sympatric speciation as in H. cunea (Takeda, 2005; Yang et al., 2017a, Yang et al., 2017b) and possibly in the Japanese burrowing cricket (Velarifictorus micado) (He and Takeda, 2013; Wang et al., 2020). Indeed, pleiotropy, disruptive selection, the presence of polymorphic intermediates, and assortative mating may help sympatric speciation without allopatric segregation when the number of loci involved is <10 (Maynard Smith, 1966; Forrest and Miller-Rushing, 2010); otherwise, evolutionary novelties are not maintained in the population. Per variation perfectly fits with this need. However, the effect of mutation in clock components must be intensive on the species status.
Another example is the European corn borer (Ostrinia nubilalis). This species was accidentally introduced from Europe to North America, and geographic adaptations thereafter produced several voltinism stocks, E- and Z-pheromone types, and host preferences. The Nanda–Hamner protocol revealed different patterns including an hour-glass type in the univoltine Minnesota strain, a typical circadian type in the bivoltine Iowa and Delaware strains, and a unimodal pattern in the multivoltine Georgia strain (Takeda and Skopik, 1985). The fundamentals of the circadian system of this species have been elucidated, including the phase response curves under two different light-pulse strengths (Skopik and Takeda, 1980, Skopik and Takeda, 1986; Skopik et al., 1981, Skopik et al., 1986). Dopman’s group conducted quantitative trait locus (QTL) analyses and identified differentiations including single-nucleotide polymorphisms (SNPs) in several circadian genes, endocrine genes, pheromone genes (Glover et al., 1992; Dopman et al., 2004, 2010), and life-cycle trait genes such as cry and per (Levy et al., 2015; Kozak et al., 2017, Kozak et al., 2019). We have identified several SNPs in per between two closely related species, H. cunea and Velarifictorus crickets, which mutually interbreed and lay viable offspring but have different life cycles (He and Takeda, 2013; Wang et al., 2020). The extent of differentiations is much greater in the Asiatic corn borer, the Ostrinia species complex which allows morphologically identifiable determination such as O. furnacalis, O. scapulalis, and O. zaguliaevi; however, North American Ostrinia species have probably followed a similar differentiation path. The apple maggot (Rhagoletis pomonella) is another example for the same mode of speciation, but the timing aspect is yet to be examined in detail (Feder et al., 1993). Tauber and Tauber (1973), Tauber and Tauber (1977a), Tauber (1977b) proposed that the mode of speciation fits with the sympatric speciation in the case of lacewings (Chrysopa carnea and C. downesi) where the split of the life cycle has affected loci related to habitat selection, life cycle, and coloration, according to Maynard Smith (1966), although Henry (1979) argued against this hypothesis and supported a conventional allopatric mode based on acoustic data of mating calls.
Thus, circadian timing has various influences in living organisms for all aspects, from pigmentation, behavior, developmental patterns, polyphenism, immunity, stress adaptation, aging, and life cycle to species status. It has made and is making “endless forms most beautiful and most wonderful”, yet common mechanisms are shared among different phyla such as melatonin signaling. Melatonin may be a lubricant for cross-talking among components forming a clock–neuroendocrine axis.
Author Contributions
MT and TS wrote the manuscript.
Conflict of Interest
The authors declare that the research was conducted in the absence of any commercial or financial relationships that could be construed as a potential conflict of interest.
Publisher’s Note
All claims expressed in this article are solely those of the authors and do not necessarily represent those of their affiliated organizations, or those of the publisher, the editors, and the reviewers. Any product that may be evaluated in this article, or claim that may be made by its manufacturer, is not guaranteed or endorsed by the publisher.
Acknowledgments
Last year, we lost two great pioneers in the field. We would like to dedicate this work and express our thanks and condolences to the families of Drs. Alfred Veerman and Ivo Hodek, who contributed greatly to our scientific quest to elucidate the photoperiodic mechanism. Fred not only revealed the involvement of carotenoid in photoperiodic perception and proposed many sophisticated photoperiodic models, but he also translated a Russian book by Zaslavsky (1988) into English. The authors acknowledge the financial support of the Japan Society for the Promotion of Science (KAKENHI grant no. 21K05614 to MT) and the Cabinet Office, Government of Japan, Moonshot R&D Program for Agriculture, Forestry and Fisheries (funding agency: Bio-oriented Technology Research Advancement Institution) [JPJ009237 to TS].
Abbreviations
5HT, 5-hydroxytryptamine (serotonin); 5HTR, serotonin receptor; aaNAT, arylalkylamine N-acetyltransferase; Arnt, aryl hydrocarbon receptor nuclear translocator; Bmal1, brain-muscle Arnt-like one; CPM, Circadian Pacemaker; Clk, clock; Cry, cryptochrome; Cry-d, Drosophila-type Cry; Cry-m, mammalian-type Cry; Cwo, clockwork orange; Cyc, cycle; Dbt, double-time; Pdp1, E-box, transcriptional enhancer cis-element; PAR domain protein one; Per, period; ΨrL, phase relationship between circadian rhythm and light–dark cycle; PTTH, prothoracicotropic hormone; RDL, resistance to dieldrin, a GABA receptor subunit; RDN, required day number; τ, free-running period; tim, timeless; RNAi, RNA interference; TRPA1, transient receptor potential A1; Vri, vrille.
References
Adamska I., Marhelava K., Walkiewicz D., Kedzierska U., Markowska M., Majewski P. M. (2016). All Genes Encoding Enzymes Participating in Melatonin Biosynthesis in the Chicken Pineal Gland Are Transcribed Rhythmically. J. Physiol. Pharmacol. 67, 521–530.
Andrewartha H. G. (1952). Diapause in Relation to the Ecology of Insects. Biol. Rev. 27, 50–107. doi:10.1111/j.1469-185X.1952.tb01363.x
Bajgar A., Jindra M., Dolezel D. (2013). Autonomous Regulation of the Insect Gut by Circadian Genes Acting Downstream of Juvenile Hormone Signaling. Proc. Natl. Acad. Sci. U.S.A. 110, 4416–4421. doi:10.1073/pnas.1217060110
Ballantyne G. H. (1969). Genetic Fine Structure and Complementation at the Albino Locus in Spider Mites (Tetranychus Species: Acarina). Genetica 40, 289–323. doi:10.1007/BF01787359
Beck S. D. (1974a). Photoperiodic Determination of Insect Development and Diapause. I. Oscillators, Hourglasses, and a Determination Model. J. Comp. Physiol. 90, 275–295. doi:10.1007/BF00701477
Beck S. D. (1974b). Photoperiodic Determination of Insect Development and Diapause. II. The Determination Gate for in a Theoretical Model. J. Comp. Physiol. 90, 297–310. doi:10.1007/BF00701478
Beck S. D. (1975). Photoperiodic Determination of Insect Development and Diapause. III Effects of Nondiel Photoperiods. J. Comp. Physiol. 103, 227–245. doi:10.1007/BF00617123
Beck S. D. (1976a). Photoperiodic Determination of Insect Development and Diapause. IV. Effects of Skeleton Photoperiods. J. Comp. Physiol. 105, 267–277. doi:10.1007/BF01379287
Beck S. D. (1976b). Photoperiodic Determination of Insect Development and Diapause. V. Diapause, Circadian Rhythms, and Phase Response Curves, According to the Dual System Theory. J. Comp. Physiol. 107, 97–111. doi:10.1007/BF00663921
Bembenek J., Sehadová H., Ichihara N., Takeda M. (2005a). Day/night Fluctuations in Melatonin Content, Arylalkylamine N-Acetyltransferase Activity and Nat mRNA Expression in the CNS, Peripheral Tissues and Hemolymph of the Cockroach, Periplaneta americana. Comp. Biochem. Physiology Part B Biochem. Mol. Biol. 140, 27–36. doi:10.1016/j.cbpc.2004.03.017
Bembenek J., Sakamoto K., Takeda M. (2005b). Molecular Cloning of a cDNA Encoding arylalkylamineN-Acetyltransferase from the Testicular System ofPeriplaneta Americana: Primary Protein Structure and Expression Analysis. Arch. Insect Biochem. Physiol. 59, 219–229. doi:10.1002/arch.20070
Bondarenko N. V. (1950). The Influence of Shortened Day on the Annual Cycle of Development of the Common Spider Mite. Dokl. Akad. Nauk. SSSR 70, 1077–1080.
Borjigin J., Wang M. M., Snyder S. H. (1995). Diurnal Variation in mRNA Encoding Serotonin N-Acetyltransferase in Pineal Gland. Nature 378, 783–785. doi:10.1038/378783a0
Bosse T. C., Veerman A. (1996). Involvement of Vitamin A in the Photoperiodic Induction of Diapause in the Spider Mite Tetranychus Urticae Is Demonstrated by Rearing an Albino Mutant on a Semi-synthetic Diet with and without P-Carotene or Vitamin A. Physiol. Entomol. 21, 188–192. doi:10.1111/j.1365-3032.1996.tb00854.x
Bowen M. F., Bollenbacher W. E., Gilbert L. I. (1984). In Vitro studies on the Role of the Brain and Prothoracic Glands in the Pupal Diapause of Manduca Sexta. J. Exp. Biol. 108, 9–24. doi:10.1242/jeb.108.1.9
Bradshaw W. E., Holzapfel C. M. (2009). Evolution of Animal Photoperiodism. Annu. Rev. Ecol. Evol. Syst. 38, 1–25. doi:10.1146/annurev.ecolsys.37.091305.110115
Bryon A., Kurlovs A. H., Dermauw W., Greenhalgh R., Riga M., Grbić M., et al. (2017). Disruption of a Horizontally Transferred Phytoene Desaturase Abolishes Carotenoid Accumulation and Diapause in Tetranychus Urticae. Proc. Natl. Acad. Sci. U.S.A. 114, E5871–E5880. doi:10.1073/pnas.1706865114
Bünning E. (1936). Die endonome Tagesrhythmik als Grundlage der photoperiodischen Reaktion. Ber. Dtsch. Bot. Ges. 54, 590–607. doi:10.1111/j.1438-8677.1937.tb01941.x
Bünning E. (1958). Die Pysiologische Uhr. “The Pysiological Clock” (Engl.Translation). Heidelberg: The English Universities Press, 1973–2258pp.
Bünning E. (1960). “Circadian Rhythms and the Time Measurement in Photoperiodism,” in Cold Spring Harbor Symposia on Quantitative Biology (Cold Spring Harbor, NY: Cold Spring Harbor Laboratory Press). 249–256. doi:10.1101/SQB.1960.025.01.026
Claret J., Arpagaus M. (1994). Evidence for 'dawn' and 'dusk' Hourglasses in Pimpla Photoperiodic Clock. Biol. Rhythm Res. 25, 106–118. doi:10.1080/09291019409360279
Claret J. (1985). Two Mechanisms in the Biological Clock ofPieris Brassicae L.: an Oscillator for Diapause Induction; an Hour-Glass for Diapause Termination. Experientia 41, 1613–1615. doi:10.1007/bf01964835
Collins B. H., Rosato E., Kyriacou C. P. (2004). Seasonal Behavior in Drosophila melanogaster Requires the Photoreceptors, the Circadian Clock, and Phospholipase C. Proc. Natl. Acad. Sci. U.S.A. 101, 1945–1950. doi:10.1073/pnas.0308240100
Coon S. L., Roseboom P. H., Baler R., Weller J. L., Namboodiri M. A. A., Koonin E. V., et al. (1995). Pineal Serotonin N -Acetyltransferase: Expression Cloning and Molecular Analysis. Science 270, 1681–1683. doi:10.1126/science.270.5242.1681
Cyran S. A., Buchsbaum A. M., Reddy K. L., Lin M.-C., Glossop N. R. J., Hardin P. E., et al. (2003). Vrille, Pdp1, and dClock Form a Second Feedback Loop in the Drosophila Circadian Clock. Cell 112, 329–341. doi:10.1016/S0092-8674(03)00074-6
Danilevskii A. S. (1965). Photoperiodism and Seasonal Development of Insects, 1st English ed, Oliver & Boyd, Edinburgh, UK. 283pp
Danilevsky A. S., Goryshin N. I., Tyshchenko V. P. (1970). Biological Rhythms in Terrestrial Arthropods. Annu. Rev. Entomol. 15, 201–244. doi:10.1146/annurev.en.15.010170.001221
Danks H. V. (1987). Insect Dormancy: an Ecological Perspective. Biol. Surv. Can. Terrestrial Arthropods. 1. 1.
Denlinger D. L. (1985). “Hormonal Control of Diapause,” in Comprehensive Insect Physiology, Biochemistry and Pharmacology. Editors G. A. Kerkut, and L. I. Gilbert (New York: Pergamon), 353–412. doi:10.1016/b978-0-08-030809-8.50017-4
Dermauw W., Jonckheere W., Riga M., Livadaras I., Vontas J., Van Leeuwen T. (2020). Targeted Mutagenesis Using CRISPR-Cas9 in the Chelicerate Herbivore Tetranychus Urticae. Insect Biochem. Mol. Biol. 120, 103347. doi:10.1016/j.ibmb.2020.103347
Des Marteaux L., Xi J., Mano G., Goto S. G. (2022). Circadian Clock Outputs Regulating Insect Photoperiodism: A Potential Role for Glutamate Transporter. Biochem. Biophysical Res. Commun. 589, 100–106. doi:10.1016/j.bbrc.2021.12.014
Doležel D., Šauman I., Košt'álKost'ál V., Hodkova M. (2007). Photoperiodic and Food Signals Control Expression Pattern of the Clock Gene, Period, in the Linden Bug, Pyrrhocoris apterus. J. Biol. Rhythm. Biol. Rhythms. 22, 335–342. doi:10.1177/0748730407303624
Dopman E. B., Bogdanowicz S. M., Harrison R. G. (2004). Genetic Mapping of Sexual Isolation between E and Z Pheromone Strains of the European Corn Borer (Ostrinia Nubilalis). Genetics 167, 301–309. doi:10.1534/genetics.167.1.301
Dopman E. B., Robbins P. S., Seaman A. (2010). Components of Reproductive Isolation between North American Pheromone Strains of the European Corn Borer. Evolution 64, 881–902. doi:10.1111/j.1558-5646.2009.00883.x
Dumortier B., Brunnarius J. (1977). Existence d’une composante circadienne dans l’induction thermopériodique de la diapause chez Pieris brassicae. CR Acad. Sci. Paris 285, 361–364.
Dumortier B. (1994). The “Circadian Paradigm”: A Test of Involvement of the Circadian System in the Photoperiodic Clock. J. Theor. Biol. 166, 101–112. doi:10.1006/jtbi.1994.1008
Emerson K. J., Bradshaw W. E., Holzapfel C. M. (2009). Complications of Complexity: Integrating Environmental, Genetic and Hormonal Control of Insect Diapause. Trends Genet. 25, 217–225. doi:10.1016/j.tig.2009.03.009
Feder J. L., Hunt T. A., Bush L. (1993). The Effects of Climate, Host Plant Phenology and Host Fidelity on the Genetics of Apple and Hawthorn Infesting Races of Rhagoletis pomonella. Rhagoletis Pomonellaentomol. Exp. Appl. 69, 117–135. doi:10.1111/j.1570-7458.1993.tb01735.x
Forrest J., Miller-Rushing A. J. (2010). Toward a Synthetic Understanding of the Role of Phenology in Ecology and Evolution. Phil. Trans. R. Soc. B 365, 3101–3112. doi:10.1098/rstb.2010.0145
Gasser R. (1951). Zur Kenntnis der gemeinen Spinnmilbe Tetranychus urticae Koch. 1. Mitteilung: Morphologie, Anatomie, Biologie und Oekologie. Mitt. Schweiz. Entomol. Ges. 24, 217–262.
Gibbs D. (1975). Reversal of Pupal Diapause in Sarcophaga Argyrostoma by Temperature Shifts after Puparium Formation. J. Insect Physiology 21, 1179–1186. doi:10.1016/0022-1910(75)90085-2
Glover T. J., Robbins P. S., Eckenrode C. J., Roelofs W. L. (1992). Genetic Control of Voltinism Characteristics in European Corn Borer Races Assessed with a Marker Gene. Arch. Insect Biochem. Physiol. 20, 107–117. doi:10.1002/arch.940200203
Grbić M., Van Leeuwen T., Clark R. M., Rombauts S., Rouzé P., Grbić V., et al. (2011). The Genome of Tetranychus Urticae Reveals Herbivorous Pest Adaptations. Nature 479, 487–492. doi:10.1038/nature10640
Hayes D. K. (1971). Action Spectra for Breaking Diapause and Action Spectra of Insect Brain Tissue. In Biochronometry, M. Menaker, ed., pp 393–402. National Academy of Sciences, Washington, DC.
He Z., Takeda M. (2013). Discrete Modes of Life Cycle in Velarifictorus Micado Species Complex (Orthoptera: Gryllidae). ISRN Entomol. 2013, 1–5. 5page. doi:10.1155/2013/851581
Henry C. S. (1979). The Courtship Call of Chrysopa Downesi Banks (Neuroptera: Chrysopidae): Its Evolutionary Significance. Psyche A J. Entomology 86, 291–297. doi:10.1155/1979/53735
Hiragaki S., Kawabe Y., Takeda M. (2008). Molecular Cloning and Expression Analysis of Two Putative Serotonin Receptors in the Brain of Antheraea pernyi Pupa. Int. J. Wild Silkmoth Silk 13, 1–14.
Hiragaki S., Uno T., Takeda M. (2009). Putative Regulatory Mechanism of Prothoracicotropic Hormone (PTTH) Secretion in the American Cockroach, Periplaneta americana as Inferred from Co-localization of Rab8, PTTH, and Protein Kinase C in Neurosecretory Cells. Cell Tissue Res. 335, 607–615. doi:10.1007/s00441-008-0747-9
Hiragaki S., Suzuki T., Mohamed A. A. M., Takeda M. (2015). Structures and Functions of Insect Arylalkylamine N-Acetyltransferase (iaaNAT): a Key Enzyme for Physiological and Behavioral Switch in Arthropods. Front. Physiology. 6. 113. doi:10.3389/2015.0011310.3389/fphys.2015.00113
Hori Y., Numata H., Shiga S., Goto S. G. (2014). Both the Anterior and Posterior Eyes Function as Photoreceptors for Photoperiodic Termination of Diapause in the Two-Spotted Spider Mite. J. Comp. Physiol. A 200, 161–167. doi:10.1007/s00359-013-0872-0
Ichihara N., Okada M., Nakagawa H., Takeda M. (1997). Purification and Characterization of Arylalkylamine N-Acetyltransferase from Cockroach Testicular Organs. Insect Biochem. Mol. Biol. 27, 241–246. doi:10.1016/S0965-1748(96)00091-4
Ichihara N., Okada M., Takeda M. (2001). Characterization and Purification of Polymorphic Arylalkylamine N-Acetyltransferase from the American Cockroach, Periplaneta americana. Insect Biochem. Mol. Biol. 32, 15–22. doi:10.1016/S0965-1748(01)00075-3
Ikeno T., Numata H., Goto S. G. (2008). Molecular Characterization of the Circadian Clock Genes in the Bean Bug, Riptortus Pedestris, and Their Expression Patterns under Long- and Short-Day Conditions. Gene 419, 56–61. doi:10.1016/j.gene.2008.05.002
Ikeno T., Tanaka S. I., Numata H., Goto S. G. (2010). Photoperiodic Diapause Under the Control of Circadian Clock Genes in an Insect. BMC Biol. 8, 116.
Iwai S., Takeda M. (2007). Expression Analysis of Two Types of Transcripts from Circadian Output Gene Lark in Bombyx mori. Comp. Biochem. Physiology Part B Biochem. Mol. Biol. 146, 470–476. doi:10.10161/;cbpb.11,01510.1016/j.cbpb.2006.11.015
Iwai S., Fukui Y., Fujiwara Y., Takeda M. (2006). Structure and Expressions of Two Circadian Clock Genes, Period and Timeless in the Commercial Silkmoth, Bombyx mori. J. Insect PhysiologyJ. Insect Physiol. 52, 625–637. doi:10.1016/j.jinsphys.2006.03.001
Iwai S., Thi Dieu Trang L., Sehadova H., Takeda M. (2008). Expression Analyses of Casein Kinase 2α and Casein Kinase 2β in the Silkmoth, Bombyx mori. Comp. Biochem. Physiology Part B Biochem. Mol. Biol. 149, 38–46. doi:10.1016/j.cbpb.2007.08.004
Izawa N., Suzuki T., Watanabe M., Takeda M. (2009). Characterization of Arylalkylamine N-Acetyltransferase (AANAT) Activities and Action Spectrum for Suppression in the Band-Legged Cricket, Dianemobius Nigrofasciatus (Orthoptera: Gryllidae). Comp. Biochem. Physiology Part B Biochem. Mol. Biol. 152, 346–351. doi:10.1016/j.cbpb.2008.12.016
Kamruzzaman A. S. M., Mikani A., Mohamed A. A., Elgendy A. M., Takeda M. (2020). Crosstalk Among Indoleamines, Neuropeptides and JH/20E in Regulation of Reproduction in the American Cockroach, Periplaneta americana. Insects 11, 155. doi:10.3390/insects11030155
Kamruzzaman A. S. M., Hiragaki S., Watari Y., Natsukawa T., Yasuhara A., Ichihara N., et al. (2021). Clock-controlled Arylalkylamine N-Acetyltransferase (aaNAT) Regulates Circadian Rhythms of Locomotor Activity in the American Cockroach, Periplaneta americana, via melatonin/MT2-like Receptor. J. Pineal Res. 71, e12751. doi:10.1111/jpi.12751
Khan M. A., Koopmanschap A. B., De Kort C. A. D. (1983). The Relative Importance of Nervous and Humoral Pathways for Control of Corpus Allatum Activity in the Adult Colorado Potato Beetle, Leptinotarsa decemlineata (Say). General Comp. Endocrinol. 52, 214–221. doi:10.1016/0016-6480(83)90115-6
Klein D. C. (2007). Arylalkylamine N-Acetyltransferase: "the Timezyme". J. Biol. Chem. 282, 4233–4237. doi:10.1074/jbc.R600036200
Kogure M. (1933).The Influence of Light and Temperature on Certain Characters of the Silkworm, Bombyx MoriBombyx mori, Dept. Agric. Kushyu Imp. Univ. 4, 1–93. doi:10.5109/22568
Konopka R. J., Benzer S. (1971). Clock Mutants of Drosophila melanogaster. Proc. Natl. Acad. Sci. U.S.A. 68, 2112–2116. doi:10.1073/pnas.68.9.2112
Kozak G. M., Wadsworth C. B., Kahne S. C., Bogdanowicz S. M., Harrison R. G., Coates B. S., et al. (2017). A Combination of Sexual and Ecological Divergence Contributes to Rearrangement Spread during Initial Stages of Speciation. Mol. Ecol. 26, 2331–2347. doi:10.111/mwc.1403610.1111/mec.14036
Kozak G. M., Wadsworth C. B., Kahne S. C., Bogdanowicz S. M., Harrison R. G., Coates B. S., et al. (2019). Genomic Basis of Circannual Rhythm in the European Corn Borer Moth. Curr. Biol. 29, 3501–3509. doi:10.1016/j.cub.2019.08.053
Lees A. D. (1950). Diapause and Photoperiodism in the Fruit Tree Red Spider Mite (Metatetranychus Ulmi Koch). Nature 166, 874–875. doi:10.1038/166874a0
Lees A. D. (1953). The Significance of the Light and Dark Phases in the Photoperiodic Control of Diapause in Metatetranychus Ulmi Koch. Ann. Appl. Biol. 40, 487–497. doi:10.1111/j.1744-7348.1953.tb02388.x
Lees A. D. (1959). The Role of Photoperiod and Temperature in the Determination of Parthenogenetic and Sexual Forms in the Aphid Megoura Viciae Buckton-I. J. Insect Physiology 3, 92–117. doi:10.1016/0022-1910(60)90078-010
Lees A. D. (1960). The Role of Photoperiod and Temperature in the Determination of Parthogenetic and Sexual Forms in the Aphid Megoura Viciae Buckton-II. The Operation of the 'interval Timer' in Young Clones. J. Insect Physiology 4, 154–175. doi:10.1016/0022-1910(60)90078-0
Lees A. D. (1964). The Location of the Photoperiodic Receptors in the Aphid Megoura Viciae Buckton. J. Exp. Biol. 41, 119–133. doi:10.1242/jeb.41.1.119
Lees A. D. (1973). Photoperiodic Time Measurement in the Aphid Megoura Viciae. J. Insect Physiology 19, 2279–2316. doi:10.1016/0022-1910(73)90237-0
Lees A. D. (1981). Action Spectra for the Photoperiodic Control of Polymorphism in the Aphid Megoura Viciae. J. Insect PhysiologyJ. Insect. Physiol. 27, 761–771. doi:10.1016/0022-1910(81)90066-4
Levy R. C., Kozak G. M., Wadsworth C. B., Coates B. S., Dopman E. B. (2015). Explaining the Sawtooth: Latitudinal Periodicity in a Circadian Gene Correlates with Shifts in Generation Number. J. Evol. Biol. 28, 40–53. doi:10.1111/jeb.12562
Lin C., Shalitin D. (2003). Cryptochrome Structure and Signal Transduction. Annu. Rev. Plant Biol. 54, 469–496. doi:10.1146/annurev.arplant.54.110901.160901
Lin J.-M., Kilman V. L., Keegan K., Paddock B., Emery-Le M., Rosbash M., et al. (2002). A Role for Casein Kinase 2α in the Drosophila Circadian Clock. Nature 420, 816–820. doi:10.1038/nature01235
Marcovitch S. (1924). The Migration of the Aphididae and the Appearance of the Sexual Forms as Affected by the Relative Length of Daily Light Exposure. J. Agric. Res. 27, 51 3–522.
Matsumoto M., Takeda M. (1996). Photoperiodic Regulation of Summer Diapause and Ecdysiotroph in Anteraea Yamamai (Lepidoptera, Saturniidae). Intern. J. Wild Silkmoth Silk. 2, 1–4.
Matsumoto M., Takeda M. (2002). Changes in Brain Monoamine Contents in Diapause Pupae of Antheraea pernyi when Activated under Long-Day and by Chilling. J. Insect Physiology 48, 765–771. doi:10.1016/S0022-1910(02)00102-6
Matsuura H., Sokabe T., Kohno K., Tominaga M., Kadowaki T. (2009). Evolutionary Conservation and Changes in Insect TRP Channels. BMC Evol. Biol. 9, 1–11. doi:10.1186/1471-2148-9-228
McMurtry J. A., Mahr D. L., Johnson H. G. (1976). Geographic Races in the Predaceous mite,Amblyseius potentillae(Acari: Phytoseiidae). Int. J. Acarol. 2, 23–28. doi:10.1080/01647957608683752
Mikani A., Watari Y., Takeda M. (2015). Brain-midgut Cross-Talk and Autocrine Metabolastat via the sNPF/CCAP Negative Feed-Back Loop in the American Cockroach, Periplaneta americana. Cell Tissue Res. 362, 481–496. doi:10.1007/s00441-015-2242-4
Miller L. W. (1950). Factors Influencing Diapause in the European Red Mite. Nature 166, 875. doi:10.1038/166875a0
Mills L. R. (1974). Structure of the Visual System of the Two-Spotted Spider-Mite, Tetranychus Urticae. J. Insect Physiology 20, 795–808. doi:10.1016/0022-1910(74)90171-1
Mohamed A. A. M., Wang Q., Bembenek J., Ichihara N., Hiragaki S., Suzuki T., et al. (2014). N-acetyltransferase (Nat) Is a Critical Conjunct of Photoperiodism between the Circadian System and Endocrine axis in Antheraea pernyi. PLoS One 9, e92680. doi:10.1371/journal.pone.0092680
Nanda K. K., Hamner K. C. (1958). Studies on the Nature of the Endogenous Rhythm Affecting Photoperiodic Response of Biloxi Soybean. Bot. Gaz. 120, 14–25. doi:10.1086/335992
Nelson R. J., Denlinger D. L., Somers D. E. (2010). Photoperiodism. New York: Oxford Univ. Press, 581 pp.
Peng G., Shi X., Kadowaki T. (2015). Evolution of TRP Channels Inferred by Their Classification in Diverse Animal Species. Mol. Phylogenetics Evol. 84, 145–157. doi:10.1016/j.ympev.2014.06.016
Pittendrigh C. S., Minis D. H. (1964). The Entrainment of Circadian Oscillations by Light and Their Role as Photoperiodic Clocks. Am. Nat. 98, 261–294. doi:10.1086/282327
Pittendrigh C. S., Minis D. H. (1971). The Photoperiodic Time Measurement in Pectinophora Gossypiella and its Relation to the Circadian System in that Species. In Biochronometry, M. Menaker, ed, pp 212–250. National Academy of Sciences, Washington, DC.
Pittendrigh C. S., Takamura T. (1987). Temperature Dependence and Evolutionary Adjustment of Critical Night Length in Insect Photoperiodism. Proc. Natl. Acad. Sci. U.S.A. 84, 7169–7173. doi:10.1073/pnas.84.20.7169
Pittendrigh C., Bruce V., Kaus P. (1958). On the Significance of Transients in Daily Rhythms. Proc. Natl. Acad. Sci. U.S.A. 44, 965–973. doi:10.1073/pnas.44.9.965
Pittendrigh C. S. (1960). Circadian Rhythms and the Circadian Organization of Living Systems. Cold Spring Harb. Symposia Quantitative Biol. 25, 159–184. doi:10.1101/SQB.1960.025.01.015
Pittendrigh C. S. (1966). The Circadian Oscillation in Drosophila pseudoobscura Pupae: A Model for the Photoperiodic Clock. Z. Pflanzenphysiol 54, 275–307.
Pittendrigh C. S. (1972). Circadian Surfaces and the Diversity of Possible Roles of Circadian Organization in Photoperiodic Induction. Proc. Natl. Acad. Sci. U.S.A. 69, 2734–2737. doi:10.1073/pnas.69.9.2734
Pittendrigh C. S. (1981). Circadian Organization and the Photoperiodic Phenomena. In Biological Clocks in Seasonal Reproductive Cycles, B. K. Follett, and D. E. Follett, eds, pp 1–35. Scientechnica, Bristol, UK.
Price J. L., Blau J., Rothenfluh A., Abodeely M., Kloss B., Young M. W. (1998). Double-Time is a Novel Drosophila Clock Gene That Regulates PERIOD Protein Accumulation. Cell 94 (1), 83–95.
Rensing L., Thach B., Bruce V. (1965). Daily Rhythms in the Endocrine Glands of Drosophila Larvae. Experientia 21, 103–104. doi:10.1007/BF02144769
Richter K., Peschke E., Peschke D. (1999). Effect of Melatonin on the Release of Prothoracicotropic Hormone from the Brain of Periplaneta americana (Blattodea: Blattidea). Eur. J. Entomol. 96, 341–345.
Rubin E. B., Shemesh Y., Cohen M., Elgavish S., Robertson H. M., Bloch G. (2006). Molecular and Phylogenetic Analyses Reveal Mammalian-like Clockwork in the Honey Bee (Apis mellifera) and Shed New Light on the Molecular Evolution of the Circadian Clock. Genome Res. 16, 1352–1365. doi:10.1101/gr.5094806
Saito K., Su Z.-H., Emi A., Mita K., Takeda M., Fujiwara Y. (2006). Cloning and Expression Analysis of Takeout/JHBP Family Genes of Silkworm, Bombyx mori. Insect Mol. BiolInsect Mol. Biol. 15, 245–251. doi:10.1111/j.1365-2583.2006.00612.x
Sarov-Blat L., So W. V., Liu L., Rosbash M. (2000). The Drosophila Takeout Gene Is a Novel Molecular Link between Circadian Rhythms and Feeding Behavior. Cell 101, 647–656. doi:10.1016/S0092-8674(00)80876-4
Sato A., Sokabe T., Kashio M., Yasukochi Y., Tominaga M., Shiomi K. (2014). Embryonic Thermosensitive TRPA1 Determines Transgenerational Diapause Phenotype of the Silkworm, Bombyx mori. Proc. Natl. Acad. Sci. U.S.A. 111, E1249–E1255. doi:10.1073/pnas.1322134111
Sattelle D. B., Harrison J. B., Chen H. H., Bai D., Takeda M. (2000). Immunocytochemical Localization of Putative γ-aminobutyric Acid Receptor Subunits in the Head Ganglia of Periplaneta americana Using an Anti-RDL C-Terminal Antibody. Neurosci. Lett. 289, 197–200. doi:10.1016/s0304-3940(00)01292-1
Sauman I., Reppert S. M. (1996a). Circadian Clock Neurons in the Silkmoth Antheraea pernyi: Novel Mechanisms of Period Protein Regulation. Neuron 17, 889–900. doi:10.1016/S0896-6273(00)80220-2
Sauman I., Reppert S. M. (1996b). Molecular Characterization of Prothoracicotropic Hormone (PTTH) from the Giant SilkmothAntheraea pernyi:Developmental Appearance of PTTH-Expressing Cells and Relationship to Circadian Clock Cells in Central Brain. Dev. Biol. 178, 418–429. doi:10.1006/dbio.1996.0228
Saunders D. S. (1973). Thermoperiodic Control of Diapause in an Insect: Theory of Internal Coincidence. Science 181, 358–360. doi:10.1126/science.181.4097.358
Saunders D. S. (1981). Insect Photoperiodism - the Clock and the Counter: a Review. Physiol. Entomol. 6, 99–116. doi:10.1111/j.1365-3032.1981.tb00264.x
Sehadová H., Markova E. P., Sehnal F., Takeda M. (2004). Distribution of Circadian Clock-Related Proteins in the Cephalic Nervous System of the Silkworm, Bombyx mori. J. Biol. Rhythms 19, 466–482. doi:10.1177/0748730404269153
Sehadová H., Shao Q.-M., Sehnal F., Takeda M. (2006). Neurohormones as Putative Circadian Clock Output Signals in the Central Nervous System of Two Cricket Species. Cell Tissue Res. 328, 239–255. doi:10.1007/s00441-006-0339-5
Sehgal A., Ousley A., Hunter-Ensor M. (1996). Control of Circadian Rhythms by a Two-Component Clock. Mol. Cell. Neurosci. 7, 165–172. doi:10.1006/mcne.1996.0013
Shao Q.-M., Sehadová H., Ichihara N., Sehnal F., Takeda M. (2006). Immunoreactivities to Three Circadian Clock Proteins in Two Ground Crickets Suggest Interspecific Diversity of the Circadian Clock Structure. J. Biol. Rhythms 21, 118–131. doi:10.1177/0748730405283660
Shao Q.-M., Hiragaki S., Takeda M. (2008a). Co-localization and Unique Distributions of Two Clock Proteins CYCLE and CLOCK in the Cephalic Ganglia of the Ground Cricket, Allonemobius Allardi. Cell Tissue Res. 331, 435–446. doi:10.1007/s00441-007-0534-z
Shao Q.-M., Bembenek J., Trang L. T. D., Hiragaki S., Takeda M. (2008b). Molecular Structure, Expression Patterns, and Localization of the Circadian Transcription Modulator CYCLE in the Cricket, Dianemobius Nigrofasciatus. J. Insect Physiology 54, 403–413. doi:10.1016/j.jinsphys.2007.10.013
Shimizu I., Kitabatake S., Kato M. (1981). Effect of Carotenoid Deficiency on Photosensitivities in the Silkworm, Bombyx mori. J. Insect Physiology 27, 593–599. doi:10.1016/0022-1910(81)90106-2
Skopik S. D., Takeda M. (1980). Circadian Control of Oviposition Activity in Ostrinia Nubilalis. Am. J. Physiology-Regulatory, Integr. Comp. Physiology 239, R259–R264. doi:10.1152/ajpregu.1980.239.3.R259
Skopik S. D., Takeda M. (1986). Photoperiodic Control of Diapause Induction and Termination in Ostrinia Nubilalis: Two Different Clocks? J. Biol. Rhythms. 1, 137–143. doi:10.1177/074873048600100204
Skopik S. D., Takeda M., Holyoke C. W. (1981). A Critical Examination of the Dual System Theory in Ostrinia Nubilalis. Am. J. Physiology-Regulatory, Integr. Comp. Physiology 241, R322–R329. doi:10.1152/ajpregu.1981.241.5.R322
Skopik S. D., Takeda M., Cain W. J., Patel N. G. (1986). Insect Photoperiodism: Diversity of Results in Night-Break Experiments, Including Nonresponsiveness to Light. J. Biol. Rhythms. 1, 243–249. doi:10.1177/074873048600100306
Stanewsky R., Kaneko M., Emery P., Beretta B., Wager-Smith K., Kay S. A., et al. (1998). The Cryb Mutation Identifies Cryptochrome as a Circadian Photoreceptor in Drosophila. Cell 95, 681–692. doi:10.1016/S0092-8674(00)81638-4
Suzuki T., Takeda M. (2009). Diapause-inducing Signals Prolong Nymphal Development in the Two-Spotted Spider miteTetranychus Urticae. Tetranychus Urticae. Physiol. Entomol. 34, 278–283. doi:10.1111/j.1365-3032.2009.00688.x
Suzuki T., Fukunaga Y., Amano H., Takeda M., Goto E. (2008a). Effects of Light Quality and Intensity on Diapause Induction in the Two-Spotted Spider Mite, Tetranychus Urticae. Tetranychus Urticaeappl. Entomol. Zool. 43, 213–218. doi:10.1303/aez.2008.213
Suzuki T., Takashima T., Izawa N., Watanabe M., Takeda M. (2008b). UV Radiation Elevates Arylalkylamine N-Acetyltransferase Activity and Melatonin Content in the Two-Spotted Spider Mite, Tetranychus Urticae. J. Insect Physiology 54, 1168–1174. doi:10.1016/j.jinsphys.2008.06.005
Suzuki T., Watanabe M., Takeda M. (2009a). UV Tolerance in the Two-Spotted Spider Mite, Tetranychus Urticae. J. Insect PhysiologyJ. Insect Physiol. 55, 649–654. doi:10.1016/j.jinsphys.2009.04.005
Suzuki T., Izawa N., Takashima T., Watanabe M., Takeda M. (2009b). Action Spectrum for the Suppression of ArylalkylamineN-Acetyltransferase Activity in the Two-Spotted Spider MiteTetranychus Urticae. Photochem. Photobiol. 85, 214–219. doi:10.1111/j.1751-1097.2008.00419.x
Suzuki T., Kojima T., Takeda M., Sakuma M. (2013). Photo-orientation Regulates Seasonal Habitat Selection in the Two-Spotted Spider miteTetranychus Urticae. J. Exp. Biol. 216, 977–983. doi:10.1242/jeb.079582
Suzuki T., Nunes M. A., España M. U., Namin H. H., Jin P., Bensoussan N., et al. (2017). RNAi-Based Reverse Genetics in the Chelicerate Model Tetranychus Urticae: A Comparative Analysis of Five Methods for Gene Silencing. PLoS ONE 12, e0180654. doi:10.1371/journal.pone.0180654
Takeda M., Masaki S. (1976). Photoperiodic Control of Larval Development in Plodia Interpunctella. Proc. Jt. U.S.-Jap. Sem. Stor. Prod. Insect. Manhattan, Ks. 1. pp 186-201.
Takeda M., Skopik S. D. (1985). Geographic Variation in the Circadian System Controlling Photoperiodism inOstrinia Nubilalis. J. Comp. Physiol. 156, 653–658. doi:10.1007/BF00619114
Takeda M. (1978). Photoperiodic Time Measurement and Seasonal Adaptation of the South-Western Corn Borer, Diatraea Grandiosella Dyar (Lepidoptera: Pyralidae). Columbia, MO: University of Missouri. PhD Thesis, 164.
Takeda M. (1985). An “Hourglass-like” Feature in the Photoperiodic Time Measurement in Diatraea Grandiosella (Pyralidae). J. Insect Physiology 31, 397–406. doi:10.1016/0022-1910(85)90085-X
Takeda M. (1986). A Circadian Clock Controlling Cricket Photoperiodism: A Resonance Effect? J. Insect Physiology 32, 557–560. doi:10.1016/0022-1910(86)90071-5
Takeda M. (2005). Differentiation in Life Cycle of Sympatric Populations of Two Forms of Hyphantria Moth in Central Missouri. Entomological Sci. 8, 211–218. doi:10.1111/j.1479-8298.2005.00117.x
Tanaka Y. (1950a). Studies on Hibernation with Special Reference to Photoperiodicity and Breeding of Chinese Tussar-Silkworm. I. . J. Serc. Sci. Jpn. 19, 358–371. doi:10.11416/kontyushigen1930.19.358
Tanaka Y. (1950b). Studies on Hibernation with Special Reference to Photoperiodicity and Breeding of Chinese Tussar-Silkworm. II. J. Serc. Sci. Jpn. 19, 429–446. doi:10.11416/kontyushigen1930.19.429
Tanaka Y. (1950c). Studies on Hibernation with Special Reference to Photoperiodicity and Breeding of Chinese Tussar-Silkworm. III. J. Serc. Sci. Jpn. 19, 580–590.
Tanaka N., Kobayashi M. (1951). Effect of Gelatin on the Polarographic Waves Ofp- Ando-Nitroanilines. Bcsj 24, 132–135. doi:10.11416/kontyushigen1930.20.13210.1246/bcsj.24.132
Tauber M. J., Tauber C. A. (1973). Quantitative Response to Daylength during Diapause in Insects. Nature 244, 296–297. doi:10.1038/244296a0
Tauber C. A., Tauber M. J. (1977a). Sympatric Speciation Based on Allelic Changes at Three Loci: Evidence from Natural Populations in Two Habitats. Science 197, 1298–1299. doi:10.1126/science.197.4310.1298
Tauber C. A., Tauber M. J. (1977b). A Genetic Model for Sympatric Speciation through Habitat Diversification and Seasonal Isolation. Nature 268 (5622), 702–705. doi:10.1038/268702a0
Tauber M. J., Tauber C. A., Masaki S. (1986). Seasonal Adaptations of Insects. New York: Oxford University Press on Demand.
Tohno Y., Takeda M. (2001). Sex-specific Circadian Dynamics of Cyclic Nucleotides in the Cerebral Ganglia of a Univoltine Strain of Antheraea pernyi during Post-diapause Development Internat. J. Wild Silkmoth Silk. 6, 17–24.
Tohno Y., Li K.-Z., Takeda M. (2000). Two Types of Diapause Development in the Chinese Oak Silkmoth, Antheraea pernyi. Int. J. Wild Silkmoth Silk. 5, 31–39.
Tomiyama Y., Shinohara T., Matsuka M., Bando T., Mito T., Tomioka K. (2020). The Role of Clockwork Orange in the Circadian Clock of the Cricket Gryllus Bimaculatus. Zool. LettZool. Lett. 6, 1–15. doi:10.1186/s40851-020-00166-4
Trang L. T. D., Sehadova H., Ichihara N., Iwai S., Mita K., Takeda M. (2006). Casein Kinases I of the Silkworm, Bombyx mori: Their Possible Roles in Circadian Timing and Developmental Determination. J. Biol. Rhythms 21, 335–349. doi:10.1177/0748730406291734
Truman J. W. (1971). “The Role of the Brain in the Ecdysis Rhythm of Silkmoths: Comparison with the Photoperiodic Termination of Diapause,” in “Biochhronometry” Nat Acad Sci. Editor M. Menaker, (Washington, DC), 483–504. 662pp.
Truman J. W. (1984). Physiological Aspects of the Two Oscillators that Regulate the Timing of Eclosion in Moths. “Photoperiodic Regul. insect molluscan hormone” CIBA Fdn Symp 104, pp221–239.
Tsuchiya R., Kaneshima A., Kobayashi M., Yamazaki M., Takasu Y., Sezutsu H., et al. (2021). Maternal GABAergic and GnRH/corazonin Pathway Modulates Egg Diapause Phenotype of the Silkworm Bombyx mori. Proc. Natl. Acad. Sci. U.S.A. 118, e2020028118. doi:10.1073/pnas.2020028118
Tsugehara T., Iwai S., Fujiwara Y., Mita K., Takeda M. (2007). Cloning and Characterization of Insect Arylalkylamine N-Acetyltransferase from Bombyx mori. Comp. Biochem. Physiology Part B Biochem. Mol. Biol. 147, 358–366. doi:10.1016/j.cbpb.2006.10.112
Tsugehara T., Imai T., Takeda M. (2013). Characterization of Arylalkylamine N-Acetyltransferase from Silkmoth (Antheraea pernyi) and Pesticidal Drug Design Based on the Baculovirus-Expressed Enzyme. Comp. Biochem. Physiology Part C Toxicol. Pharmacol. 157, 93–102. doi:10.1016/j.cbpc.2012.10.003
Tyshchenko V. P. (1966). Two-oscillatory Model of the Physiological Mechanism of Insect Photoperiodic Reaction. Zh. Obsch. Biol. 27, 209–222.
Vafopoulou X., Steel C. G. (1996). The Insect Neuropeptide Prothoracicotropic Hormone Is Released with a Daily Rhythm: Re-evaluation of its Role in Development. Proc. Natl. Acad. Sci. U.S.A. 93, 3368–3372. doi:10.1073/pnas.93.8.3368
Vafopoulou X., Steel C. G. H. (2006). Hormone Nuclear Receptor (EcR) Exhibits Circadian Cycling in Certain Tissues, but Not Others, during Development in Rhodnius prolixus (Hemiptera). Cell Tissue Res. 323, 443–455. doi:10.1007/s00441-005-0076-1
van Houten Y. M., Overmeer W. P. J., Veerman A. (1987). Thermoperiodically Induced Diapause in a Mite in Constant Darkness Is Vitamin A Dependent. Experientia 43, 933–935. doi:10.1007/BF01951676
Van Zon A. Q., Overmeer W. P. J., Veerman A. (1981). Carotenoids Function in Photoperiodic Induction of Diapause in a Predacious Mite. Science 213, 1131–1133. doi:10.1126/science.213.4512.1131
Vaz Nunes M., Veerman A. (1979a). Photoperiodic Time Measurement in Spider Mites. I. Development of Two Interval Timers Model. J. Comp. Physiol. 134, 203–217. doi:10.1007/BF00610395
Vaz Nunes M., Veerman A. (1979b). Photoperiodic Time Measurement in Spider Mites. II. Effects of Skeleton Photoperiods. J. Comp. Physiol. 134, 219–226. doi:10.1007/BF00610396
Veerman A., Helle W. (1978). Evidence for the Functional Involvement of Carotenoids in the Photoperiodic Reaction of Spider Mites. Nature 275, 234. doi:10.1038/275234a0
Veerman A., Nunes M. V. (1980). Circadian Rhythmicity Participates in the Photoperiodic Determination of Diapause in Spider Mites. Nature 287, 140–141. doi:10.1038/287140a0
Veerman A., Nunes M. V. (1987). Analysis of the Operation of the Photoperiodic Counter Provides Evidence for Hourglass Time Measurement in the Spider miteTetranychus Urticae. J. Comp. Physiol. 160, 421–430. doi:10.1007/BF00615076
Veerman A., Overmeer W. P. J., Van Zon A. Q., De Boer J. M., De Waard E. R., Huisman H. O. (1983). Vitamin A Is Essential for Photoperiodic Induction of Diapause in an Eyeless Mite. Nature 302, 248–249. doi:10.1038/302248a0
Veerman A. (1974). Carotenoid Metabolism in Tetranychus Urticae Koch (Acari: Tetranychidae). Comp. Biochem. Physiology Part B Comp. Biochem. 47, 101–116. doi:10.1016/0305-0491(74)90095-9
Veerman A. (1977a). Photoperiodic Termination of Diapause in Spider Mites. Nature 266, 526–527. doi:10.1038/266526b0
Veerman A. (1977b). Aspects of the Induction of Diapause in a Laboratory Strain of the Mite Tetranychus Urticae. J. Insect Physiology 23, 703–711. doi:10.1016/0022-1910(77)90087-7
Veerman A. (1980). Functional Involvement of Carotenoids in Photoperiodic Induction of Diapause in the Spider Mite, Tetranychus Urticae. Physiol. Entomol. 5, 291–300. doi:10.1111/j.1365-3032.1980.tb00237.x
Veerman A. (1992). Diapause in Phytoseiid Mites: a Review. Exp. Appl. Acarol. 14, 1–60. doi:10.1007/BF01205351
Veerman A. (2001). Photoperiodic Time Measurement in Insects and Mites: a Critical Evaluation of the Oscillator-Clock Hypothesis. J. Insect Physiology 47, 1097–1109. doi:10.1016/S0022-1910(01)00106-8
Wang Q., Mohamed A. A. M., Takeda M. (2013). Serotonin Receptor B May Lock the Gate of PTTH Release/synthesis in the Chinese Silk Moth, Antheraea pernyi; a Diapause Initiation/maintenance Mechanism? PLoS One 8, e79381. doi:10.1371/journal.pone.0079381
Wang Q., Egi Y., Takeda M., Oishi K., Sakamoto K. (2015a). Melatonin Pathway Transmits Information to Terminate Pupal Diapause in the Chinese Oak silkmothAntheraea Pernyiand through Reciprocated Inhibition of Dopamine Pathway Functions as a Photoperiodic Counter. Entomological Sci. 18, 74–84. doi:10.1111/ens.12083
Wang Q., Hanatani I., Takeda M., Oishi K., Sakamoto K. (2015b). D2-like Dopamine Receptors Mediate Regulation of Pupal Diapause in Chinese Oak silkmothAntheraea Pernyi. Entomological Sci. 18, 193–198. doi:10.1111/ens.12099
Wang B., Li K., He Z. Q., Takeda M. (2020). The Genetic Differentiation of a Cricket (Velarifictorus Micado) with Two Modes of Life Cycle in East Asia after the Middle Pleistocene and the Invasion Origin of the United States of America. Ecol. Evol. 10, 13767–13786. doi:10.1002/ece3.6967
Wilde J., Bonga H. (1958). Observations on Threshold Intensity and Sensitivity to Different Wave Lengths of Photoperiodic Responses in the Colorado Beetle (Leptinotarsa Decemlineata Say). Entomol. Exp. Appl. 1, 301–307. doi:10.1111/j.1570-7458.1958.tb00034.x
Williams C. M., Adkisson P. L. (1964). Physiology of Insect Diapause. XIV. An Endocrine Mechanism for the Photoperiodic Control of Pupal Diapause in the Oak Silkworm, Antheraea pernyi. Biol. Bull. 127, 511–525. doi:10.2307/1539252
Williams C. M. (1969). Photoperiodism and the Endocrine Aspects of Insect Diapause. Symp. Soc. Exp. Biol. 23, 285–300. doi:10.1039/qr9692300001
Withrow R. B. (1959). Photoperiodism and Related Phenomena in Plants and Animals. Wash. D.C. Amer. Assoc. Adv. Sci. 1. 1.
Wood S., Loudon A. (2014). Clocks for all Seasons : Unwinding the Roles and Mechanisms of Circadian and Interval Timers in the Hypothalamus and Pituitary. J. Endocrinol. 222. R39–R59.
Wybouw N., Kurlovs A. H., Greenhalgh R., Bryon A., Kosterlitz O., Manabe Y., et al. (2019). Convergent Evolution of Cytochrome P450s Underlies Independent Origins of Keto-Carotenoid Pigmentation in Animals. Proc. R. Soc. B 286, 20191039. doi:10.1098/rspb.2019.1039
Yamano H., Watari Y., Arai T., Takeda M. (2001). Melatonin in Drinking Water Influences a Circadian Rhythm of Locomotor Activity in the House Cricket, Acheta domesticus. J. Insect Physiology 47, 943–949. doi:10.1016/S0022-1910(01)00067-1
Yang F., Hiragaki S., Kamruzzaman A. S. M., Takeda M. (2017a). The Involvement of Circadian Clock Gene Period in Photoperiodic Time Measurement of the Fall Webworm, Hyphantria cunea. Glob. J. Adv. Study 3 (11), 985–991.
Yang F., Kawabata E., Tufail M., Brown J. J., Takeda M. (2017b). r/K-like Trade-Off and Voltinism Discreteness: The Implication to Allochronic Speciation in the Fall Webworm,Hyphantria Cuneacomplex (Arctiidae). Ecol. Evol. 7, 10592–10603. doi:10.1002/ece3.3334
Yin C.-M., Takeda M., Wang Z.-S. (1987). A Juvenile Hormone Analogue, Methoprene as a Circadian and Developmental Modulator in Diatraea Grandiosella (Pyralidae). J. Insect Physiology 33, 95–102. doi:10.1016/0022-1910(87)90080-1
Zaslavski V. A. (1988). “Quantitative Perception of Photoperiod,” in Insect Development: Photoperiodic and Temperature Control. Editor A. Veerman translated (Berlin: Springer-Verlag), 91–99. 187pp.
Zhang Q., Lu Y.-X., Xu W.-H. (2012). Integrated Proteomic and Metabolomic Analysis of Larval Brain Associated with Diapause Induction and Preparation in the Cotton Bollworm, Helicoverpa Armigera. Helicoverpa armigeraJ. Proteome Res. 11, 1042–1053. doi:10.1021/pr200796a
Zhang Y., Markert M. J., Groves S. C., Hardin P. E., Merlin C. (2017). Vertebrate-Like CRYPTOCHROME 2 From Monarch Regulates Circadian Transcription via Independent Repression of CLOCK and BMAL1 Activity. PNAS 114, E7516–E7525.
Zhang J., Li S., Li W., Chen Z., Guo H., Liu J., et al. (2021). Circadian Regulation of Night Feeding and Daytime Detoxification in a Formidable Asian Pest Spodoptera Litura. Commun. Biol. 4, 286. doi:10.1038/s42003-021-01816-9
Keywords: arylalkylamine N-acetyltransferase, circadian oscillation, E-box, prothoracicotropic hormone, melatonin, photoperiodic time measurement, serotonin receptor, melatonin receptors
Citation: Takeda M and Suzuki T (2022) Circadian and Neuroendocrine Basis of Photoperiodism Controlling Diapause in Insects and Mites: A Review. Front. Physiol. 13:867621. doi: 10.3389/fphys.2022.867621
Received: 01 February 2022; Accepted: 02 May 2022;
Published: 22 June 2022.
Edited by:
Wen Liu, Huazhong Agricultural University, ChinaReviewed by:
Joanna Kotwica-Rolinska, Academy of Sciences of the Czech Republic (ASCR), CzechiaJ. Joe Hull, U.S. Arid Land Agricultural Research Center (USDA ARS), United States
Copyright © 2022 Takeda and Suzuki. This is an open-access article distributed under the terms of the Creative Commons Attribution License (CC BY). The use, distribution or reproduction in other forums is permitted, provided the original author(s) and the copyright owner(s) are credited and that the original publication in this journal is cited, in accordance with accepted academic practice. No use, distribution or reproduction is permitted which does not comply with these terms.
*Correspondence: Makio Takeda, bXRha2VkYUBrb2JlLXUuYWMuanA=