- Department of Kinesiology, Iowa State University, Ames, IA, United States
Age-related chronic diseases are among the most common causes of mortality and account for a majority of global disease burden. Preventative lifestyle behaviors, such as regular exercise, play a critical role in attenuating chronic disease burden. However, the exact mechanism behind exercise as a form of preventative medicine remains poorly defined. Interestingly, many of the physiological responses to exercise are comparable to aging. This paper explores an overarching hypothesis that exercise protects against aging/age-related chronic disease because the physiological stress of exercise mimics aging. Acute exercise transiently disrupts cardiovascular, musculoskeletal, and brain function and triggers a substantial inflammatory response in a manner that mimics aging/age-related chronic disease. Data indicate that select acute exercise responses may be similar in magnitude to changes seen with +10–50 years of aging. The initial insult of the age-mimicking effects of exercise induces beneficial adaptations that serve to attenuate disruption to successive “aging” stimuli (i.e., exercise). Ultimately, these exercise-induced adaptations reduce the subsequent physiological stress incurred from aging and protect against age-related chronic disease. To further examine this hypothesis, future work should more intricately describe the physiological signature of different types/intensities of acute exercise in order to better predict the subsequent adaptation and chronic disease prevention with exercise training in healthy and at-risk populations.
Introduction
Age-related chronic diseases (e.g., cardiovascular disease, chronic kidney disease, Alzheimer’s Dementia, Type II Diabetes, etc.) are among the most common causes of mortality and account for a majority of global disease burden (Yach et al., 2004; Kennedy et al., 2014; Murphy et al., 2018). Preventive lifestyle strategies such as exercise have emerged as potent, cost-effective means of reducing chronic disease risk (Sallis, 2009; Sepanlou et al., 2011; Bauer et al., 2014). Exercise has a critical role in disease prevention (Booth et al., 2012; Pedersen and Saltin, 2015; Sallis, 2015; Bennie et al., 2020) and has been proposed by the American College of Sports Medicine as a form of “medicine” (Church and Blair, 2009; Sallis, 2009, 2015). The protective effects of exercise on chronic disease risk are ultimately accumulated over time through physiological adaptations to the stress of exercise.
Acute exercise causes widespread physiological disruptions that require a complex, integrated response from the major physiological systems (autonomic, cardiovascular, metabolic, musculoskeletal, etc.) to meet the substantial requirements of human locomotion (Hawley et al., 2014, 2018). Repeated exposure to the physiological disruptions incurred by acute exercise (through exercise training) stimulate physiological adaptations that act to attenuate stress during subsequent exercise bouts (Małkiewicz et al., 2019). These exercise adaptations provide the foundation through which individuals can adapt and improve their ability to perform physical work (e.g., increase muscular power, endurance, aerobic capacity, etc.) and also prevent development of age-related chronic disease (Hawley et al., 2014, 2018). Thus, physiologic adaptations to exercise are the latent mechanisms through which exercise acts as medicine and reduces chronic disease risk. Despite seminal work that has identified several key mechanisms underlying the protective effects of exercise, there has yet to be an overarching hypothesis that explains broadly why or how it is that exercise protects against age-related chronic disease. We posit that exercise prevents age-related chronic disease because it acutely elicits physiological responses that mimic physiological changes seen with aging, the greatest contributing risk factor to all chronic disease (Bauer et al., 2014; Kennedy et al., 2014). Thus, we propose the hypothesis that exercise is “medicine” that protects against age-related chronic diseases because exercise can effectively simulate “aging.” This paper is not intended to comprehensively review the physiological adaptations to exercise or their specific benefits on health/disease (see prior reviews; (Hawley et al., 2014, 2018; Green et al., 2017; Tanaka, 2019; McGee and Hargreaves, 2020), rather, we will examine this hypothesis by comparing age-related physiological changes with those induced during acute exercise and integrate these responses within the context and implications of stress-induced adaptation. This is not a systematic review, rather, we conducted a literature search of original data and reviews (when appropriate) examining the physiological effects of acute exercise on the brain (cognitive, brain-blood-barrier), cardiovascular, neuroendocrine, inflammation/oxidative stress, metabolic, and musculoskeletal systems and then aligned those observations with literature describing changes seen with aging and age-related chronic disease.
Effects of Aging and Exercise on the Brain
Aging is accompanied by natural reductions across multiple domains of cognitive function (memory, reasoning abilities, executive function, and processing speed) (Carlson et al., 1995; Hayden and Welsh-Bohmer, 2012; Salthouse, 2012; Harada et al., 2013). Increasing age is also associated with inflammation and oxidative stress that damages the cerebral microvasculature and decreases blood-brain-barrier integrity (Verheggen et al., 2020). Ultimately, reductions in higher-order cognitive processing (memory/executive function) and blood-brain-barrier permeability are implicated in the underlying pathology and presentation of dementia and Alzheimer’s disease (Salthouse, 2012; Harada et al., 2013; Gamba et al., 2015; Kirova et al., 2015).
Acute exercise imposes substantial stress on brain function and blood-brain-barrier integrity that parallel changes observed with age and cognitive disease. Acute exercise (particularly high intensity exercise) can impair higher order cognitive processing (e.g., executive function) through reallocation of mental resources (Audiffren et al., 2009) in an exercise intensity-dependent fashion (Lambourne and Tomporowski, 2010; Wohlwend et al., 2017). Exercise also acutely disrupts blood-brain-barrier integrity, with increased blood-brain-barrier permeability immediately following intense exercise (Sharma et al., 1991; Roh et al., 2017). This acute disruption in blood-brain-barrier integrity may be related to the effects of exercise on 1) oxidative-nitrosative stress (the origins of which are discussed further in subsequent sections) at the blood-brain-barrier interface that damages cells, reorganizes cytoskeletons, and increases inflammation (Sharma et al., 1991; Roh et al., 2017), 2) vasoactive effects of serotonin (Sharma et al., 1991), and 3) changes in cerebral blood flow patterns during exercise (e.g. increased pulsatile hemodynamics) (Armentano et al., 1991; Ogoh et al., 2005; Alwatban et al., 2020) which are linked with blood-brain-barrier damage and disruption (Jufri et al., 2015; Garcia-Polite et al., 2017; de Montgolfier et al., 2019).
Effects of Aging and Exercise on the Cardiovascular System
Aging is associated with an increase in mean blood pressure, resulting from a steady rise in systolic blood pressure and a slight decline in diastolic blood pressure (Franklin et al., 1997). Age-related increases in blood pressure may stem from, and simultaneously promote, large artery stiffening (Henskens et al., 2008; Najjar et al., 2008; Kaess et al., 2012; Mitchell, 2014; Tarumi et al., 2014; Zhou et al., 2018), which amplifies the magnitude of forward traveling energy waves and increases pulsatile blood pressure and flow (Mitchell, 2014; Tarumi et al., 2014; Lefferts et al., 2020). Age-related increases in large artery stiffness may be due, in part, to endothelial dysfunction wrought by oxidative stress and subsequent reductions in nitric oxide bioavailability (Donato et al., 2015; LaRocca et al., 2017). Ultimately, age-related vascular dysfunction increases cardiac work (i.e., afterload) and results in left ventricular hypertrophic remodeling (Lovic et al., 2017; Yildiz et al., 2020) and diastolic dysfunction (Strait and Lakatta, 2012; Abdellatif et al., 2018). Cumulatively, age-related vascular and cardiac dysfunction are intrinsically linked with the risk and development of cardiovascular disease (Lakatta and Levy, 2003; Abdellatif et al., 2018).
The cardiovascular response during acute exercise is markedly similar to the detrimental, chronic changes in cardiovascular function seen with aging. Exercise produces a substantial blood pressure response [systolic pressures >190 mmHg in young adults (Sabbahi et al., 2018)] and increase in heart rate that stiffens the large arteries (Armentano et al., 1991; Studinger et al., 2003; Townsend et al., 2015). Increases in large artery stiffness during exercise (Studinger et al., 2003; Sharman et al., 2005; Pomella et al., 2018) are accompanied by increased forward wave energy (Jiang et al., 1995; Heckmann et al., 2000; Stock et al., 2021) and decreased wave reflection (Stock et al., 2021), ultimately contributing to greater pulsatile hemodynamics (Armentano et al., 1991; Ogoh et al., 2005; Alwatban et al., 2020). Additionally, exercise-induced acute increases in blood pressure may transiently impair endothelial function through a combination of mechanical distension/dilation of the artery, reductions in nitric oxide bioavailability, and endothelin-1 release during exercise (Millgård and Lind, 1998; Jurva et al., 2006; Gonzales et al., 2011; Morishima et al., 2020). This acute vascular response during exercise is further accompanied by a substantial (2–5-fold) increase in cardiac work (Channer and Jones, 1989; Rowland et al., 2002; Vega et al., 2017) that over time can stimulate ventricular remodeling in a similar manner to aging.
Effects of Aging and Exercise on Neuroendocrine System
Aging impacts various neuro/endocrine regulatory systems throughout the body. Serum cortisol increases 20–50% throughout the adult lifespan (Chahal and Drake, 2007; Feller et al., 2014) owing to hormonal changes in the hypothalamic-pituitary-adrenal axis (Corazza et al., 2014). Aging is also associated with autonomic nervous system dysfunction manifesting as increased sympathetic and decreased parasympathetic nervous system activity (Pfeifer et al., 1983; Jandackova et al., 2016). Higher cortisol levels over time are associated with increased cardiometabolic disease risk and may compromise immune function in older adults (Corazza et al., 2014; Feller et al., 2014), whereas shifts in autonomic balance favoring sympathetic activity is an independent risk factor for cardiovascular disease (de Jonge et al., 2010; de Lucia et al., 2018). Both of these neuroendocrine responses to aging are mimicked by acute exercise. Cortisol levels increase during acute exercise in an intensity-dependent manner (Brandenberger and Follenius, 1975; Kanaley et al., 2001). Similarly, sympathetic nerve activity increases in exercising muscle and cardiac autonomic balance shifts to favor sympathetic over parasympathetic activity (Rowell, 1997; Michael et al., 2017).
Effects of Aging and Exercise on Inflammation and Oxidative Stress
There is a well-established relationship between age and chronic low-level systemic inflammation (Ferrucci et al., 2010; Liberale et al., 2020). Circulating inflammatory markers increase with age in-part owing to increased chronic activation of the immune system (Liberale et al., 2020). Chronic inflammation with aging increases production of reactive oxygen (ROS)/nitrogen species (RNS) (Sergiev et al., 2015; Davalli et al., 2016). Higher levels of ROS/RNS promote cellular oxidative damage (cell membrane breakdown, protein modification, DNA damage) (Davalli et al., 2016) which can be further exaggerated by additional oxidative stress independent of ROS/RNS (Kudryavtseva et al., 2016). Ultimately, elevated markers of oxidative stress and systemic inflammation are strongly associated with increased risk of neurodegenerative, cardiovascular, and kidney disease, cancer, and dementia (Verbon et al., 2012; Marseglia et al., 2014; Kudryavtseva et al., 2016; Coen et al., 2018; Ferrucci and Fabbri, 2018; Senoner and Dichtl, 2019; Liberale et al., 2020).
Circulating inflammatory markers and oxidative stress also increase with acute exercise (Peake et al., 2005; Tsao et al., 2021). Acute exercise has been shown to increase pro-inflammatory cytokines such as interleukin (IL)-6 (Fischer, 2006), IL-7 (Małkiewicz et al., 2019), IL-10, C-reactive protein, and tumor necrosis-factor alpha (TNF-α) (Bernecker et al., 2013; Cerqueira et al., 2019; Fonseca et al., 2021) and initiate an inflammatory cascade (Powers and Jackson, 2008; McDonagh et al., 2014; Luca and Luca, 2019; Powers et al., 2020; Aragón-Vela et al., 2021). Additionally, exercise increases skeletal muscle ROS/RNS production via 1) electron leakage during oxidative phosphorylation within the mitochondria and NAD(P)H oxidase, 2) nitric oxide synthase activity within the skeletal muscle, 3) catecholamine and prostanoid release, and 4) ischemia/reperfusion-induced changes in xanthine oxidase activity, which ultimately contributes to oxidative stress, and subsequent cellular damage (Fisher-Wellman and Bloomer, 2009; McDonagh et al., 2014; Bouzid et al., 2015; Davalli et al., 2016; Powers et al., 2016; Petriz et al., 2017). As such, acute exercise can act as a pro-inflammatory stimulus that increases oxidative stress and damage in a manner similar to aging.
Effects of Aging and Exercise on Metabolism
Advancing age is accompanied by alterations in both the metabolic pathways of energy production and mitochondrial function. Aging results in a steady rise in blood glucose concentration (Ko et al., 2006), driven in part by insulin resistance, exaggerated hepatic glucose production, and increasing cortisol levels (Satrústegui et al., 1986; Magnusson et al., 1992; Ko et al., 2006; Rizza, 2010). Similarly, aging and insulin resistance promote unrestrained lipolysis, which could contribute to systemic inflammation by increasing circulating free fatty acids (Reaven et al., 1989; Wende et al., 2012). Mitochondrial function is also impaired with aging, resulting in 1) increased sensitivity to ROS, 2) impaired oxidative metabolism, and 3) compromised mitochondrial membrane integrity (Shigenaga et al., 1994; Balaban et al., 2005). Mitochondrial dysfunction increases generation of oxidative byproducts (e.g. ROS/RNS) within the electron transport chain (Bratic and Larsson, 2013; Quinlan et al., 2013; Davalli et al., 2016) and thus accelerates age-related cellular damage (Genova and Lenaz, 2015). Taken together, these aspects of metabolic and mitochondrial dysfunction are associated with obesity, type II diabetes mellitus, obesity, fatty liver disease, cancer, sarcopenia and Alzheimer’s disease (Kim et al., 2001; Wende et al., 2012; Girousse et al., 2018; Yoo et al., 2019; Spitler and Davies, 2020; Paliwal et al., 2021).
Acute exercise also perturbs metabolic pathways, increases mitochondrial ROS production, and alters mitochondrial membrane permeability (Tonkonogi and Sahlin, 2002; Powers and Jackson, 2008). Acute exercise stimulates adipose tissue lipolysis, with low/moderate exercise eliciting a 2 to 5-fold increase in circulating free fatty acids for use in substrate metabolism (Havel et al., 1963; Ahlborg et al., 1974; Wolfe et al., 1990; Romijn et al., 1993; Ranallo and Rhodes, 1998; Burguera et al., 2000). Moreover, acute exercise also increases liver gluconeogenesis and hepatic glucose output via catecholamine release and sympathetic activity (Dibe et al., 2020) which aligns with changes in glucose production with aging.
Effects of Aging and Exercise on the Musculoskeletal System
Skeletal integrity begins to decrease around 35–40 years of age, with postmenopausal women experiencing bone loss at a rate of approximately 1–2% annually (Riggs et al., 2008; Curtis et al., 2015) owing to disproportionate increases in bone breakdown versus buildup. Similarly, aging is also often accompanied by 1) muscle atrophy from imbalances between muscle protein synthesis and degradation in response to anabolic stimuli (Reynolds et al., 2002; Koopman and van Loon, 2009; Wall et al., 2015) and 2) reduced muscular force production (Zizzo, 2021). Age-related shifts in protein synthesis/degradation and reductions in force production may stem from free radical accumulation/oxidative stress and inflammation that activate proteolytic pathways, damage the muscle, and impair mitochondrial function (Guo et al., 2013; McDonagh et al., 2014; Fernando et al., 2019; Zizzo, 2021). Taken together, these musculoskeletal changes contribute to dyna-/sarco-penia and osteoporosis which have a profound impact on health and longevity with aging (Tagliaferri et al., 2015; Prawiradilaga et al., 2020).
Though exercise has long been known to stimulate bone mineralization and promote increased bone density, the initial response following any mechanical stimulus such as exercise is the resorption/breakdown of bone (Feng and McDonald, 2011). Similarly, exercise may acutely suppress muscle protein synthesis and increase protein degradation (Tipton and Wolfe, 1998; Kumar et al., 2009). Muscle force production also decreases following a bout of acute exercise (Howatson and van Someren, 2008) owing to, 1) inflammatory damage via increased mitochondrial reactive oxygen/nitrogen species within the working muscle (Powers and Jackson, 2008), and 2) structural damage (i.e., filament disintegration/misalignment, z-band streaming, excitation-coupling failure) incurred within the straining muscle (Fridén and Lieber, 1992). These effects of acute exercise ultimately contribute to initial reductions in voluntary force production following exercise (Howatson and van Someren, 2008).
Implications of Adaptations to Exercise as an “Aging Stimulus”
As outlined above, there is substantial evidence that the acute physiological response to exercise mimics physiological responses that occur with aging and age-related chronic disease (Figure 1). As such, acute exercise could be conceptualized as a transient bout of “aging.” The body naturally adapts to any stress (such as exercise) that disrupts homeostasis (Figure 2A). Proper adaptation to transient stimuli reduces stress during subsequent stressors (e.g., the next bout of exercise; see diminishing size of exercise-induced dysfunction in Figures 2A,B). For example, 1) exercise-induced increases in inflammation are attenuated following exercise training (Orlander et al., 1977; Fonseca et al., 2021), 2) increases in cardiac work are attenuated (e.g., lower heart rate) at a given workload following exercise training (Orlander et al., 1977), and 3) exercise training enhances antioxidant defense against exercise-induced oxidative stress (Bouzid et al., 2015). Parallels between the physiological stress of acute exercise and age-related chronic disease support the notion that repeated exposure to an exercise stimulus and the subsequent adaptations would protect against the physiological stress of aging and age-related chronic disease (Figure 2B).
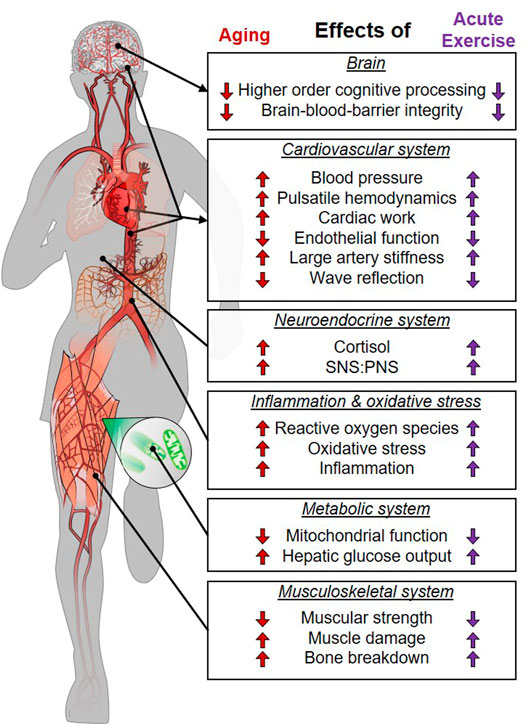
FIGURE 1. Parallels between the stress of aging/chronic disease and acute physical exercise. SNS, sympathetic nervous system; PNS, parasympathetic nervous system.
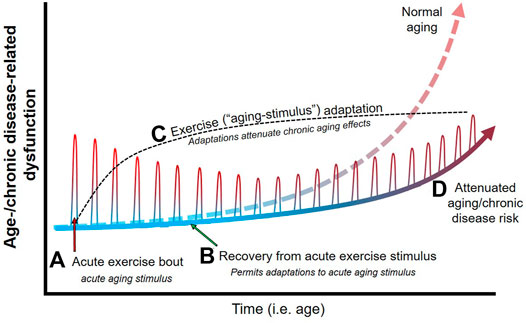
FIGURE 2. Theoretical effects of physical exercise as an aging stimulus on age-/chronic disease-related physiological dysfunction. Age-related dysfunction (e.g., cardiovascular, metabolic, muscular) generally increases steeply around middle-age into older age, and results in an increase in chronic disease risk. An acute bout of exercise (A) acts as an aging stimulus and elicits responses during exercise that mimic that of age-related dysfunction (e.g., increased large artery stiffness, inflammation, etc.). Cessation of exercise (i.e., removal of the acute aging stimulus) and proper recovery between exercise bouts/stimuli (B) permits adaptations (C) that serve to reduce the physiological stress during successive exercise (i.e., aging) bouts. Since acute exercise elicits physiological responses that parallel aging, exercise adaptations essentially prepare the body to endure less physiological stress and dysfunction when exposed to the effects of aging over time. As such, regular exposure to transient aging stimuli (i.e., regular physical exercise) elicits physiological adaptations that attenuate age-/chronic disease-related dysfunction (D), and thus attenuates many of the detrimental physiological effects of age and protects against chronic disease development.
If exercise is viewed as an aging mimetic, then more intense exercise should elicit a larger “aging” stressor and subsequent adaptation and protection against age-related chronic disease. Indeed, observational data suggest a dose-response relationship between exercise and physiological/health benefits, such that larger doses of exercise generally elicit greater protection (Ekelund et al., 2019; Shi et al., 2020; Aune et al., 2021). The protection afforded by exercise and the stress-adaptation cycle are maximized when the stress is transient and adequate recovery is allowed for adaptation (Figure 2C) (Booth and Laye, 2009). In the case of exercise, some data indicate extreme exercise volumes (e.g., marathons, ultramarathons) may be accompanied by pathological changes and a loss of health benefits, although this remains an area of debate (Eijsvogels et al., 2018; O’Keefe et al., 2020). Indeed, the line between physiological and pathological adaptations become blurred with high volumes of exercise being linked with risk of arrhythmias (Claessen et al., 2011; Andersen et al., 2013), cardiac dysfunction (O’Keefe et al., 2012; Rajanayagam and Alsabri, 2021), and myocardial injury (Neilan et al., 2006). Our hypothesis links to these observations since exposure to 1) extreme aging stimuli or 2) too frequent of exposure to an aging stimulus (preventing adequate recovery and adaptation) could contribute to negative (i.e., pathological) adaptations, accelerate physiological “aging,” and attenuate health benefits (Eijsvogels et al., 2018; O’Keefe et al., 2020). As such, the notion that exercise mimics aging provides insight into how exercise can both protect against age-related chronic disease and potentially give way to pathological changes under extreme exercise volumes.
Alternative Perspectives and Limitations
We openly acknowledge that the actual cellular/molecular mechanisms driving acute and training responses to exercise may differ from those contributing to physiological changes with aging/age-related chronic disease (e.g., exercise and the cardiovascular demands required to meet metabolic output for musculoskeletal movement are fundamentally different mechanisms than those governing increases in blood pressure with aging such as degradation of elastin, microvascular rarefaction, endothelial dysfunction etc.). Many examples demonstrate the phenomenon of cross-tolerance, in which, despite diverse mechanisms, one stressor [e.g., exercise, environment (heat stress)] can confer protective benefits across other different stressors (Bond et al., 1999; Heled et al., 2012; Corbett et al., 2014; White et al., 2014; Wang et al., 2021). Consistent with this concept, our hypothesis is that the stimulus (e.g., an increase in blood pressure) for adaptation is similar between acute exercise and aging/age-related chronic disease and thus exercise adaptations may be mutually beneficial for both reducing the stress of subsequent exercise stimuli and aging/chronic disease pathways that involve that particular signal (e.g., blood pressure and cardio-/cerebro-vascular/cognitive disease).
Data indicate that lower intensity exercise/physical activity (e.g., walking) can confer mortality benefits in the absence of detectable physiological adaptations (Wasfy and Baggish, 2016). This raises the possibility that acute low intensity exercise 1) offers protection without adequately disrupting homeostasis and subsequent physiologic adaptations (contrary to our hypothesis), or 2) benefits age-related chronic disease burden through accumulation of diffuse, modest physiological adaptations that reflect a more modest exercise stimulus. Indeed, activities of daily living often viewed as “low” intensity (e.g., walking) are actually considered moderate intensity among older/deconditions populations (Sundquist et al., 2004; McPhee et al., 2016) and result in modest increases in energy expenditure (Maciejczyk et al., 2016), ventilation (Fusi et al., 2005), and cardiovascular stress (Renzi et al., 2010; Sugawara et al., 2015; Carter et al., 2018). Thus, even low-intensity exercise/physical activity may elicit similar directional physiological changes as “aging” and moderate-to-vigorous intensity exercise (as discussed above), albeit of smaller magnitude. This supports the idea that lower intensity activity patterns may need to be continued for longer periods of time to accumulate physiological benefits and reduce chronic disease risk (Carnethon, 2009). In the context of our hypothesis low-intensity exercise/physical activity likely elicits a smaller homeostatic disruption that represents a smaller “aging” stimulus, and thus more modest adaptations and benefits (in line with the dose-response literature). It is not surprising to see more sizeable benefits wrought from moderate and vigorous exercise intensities since these intensities can acutely elicit physiological responses comparable in magnitude to +10–50 years of aging (see Table 1) (Franklin et al., 1997; Hilbert et al., 2003; Ogoh et al., 2005; Ferrucci et al., 2012; Keith et al., 2013; Alwatban et al., 2020; Lefferts et al., 2020), and that exercise-trained older adults can be phenotypically similar to adults 40 years younger (Bhella et al., 2014; Fragala et al., 2019).
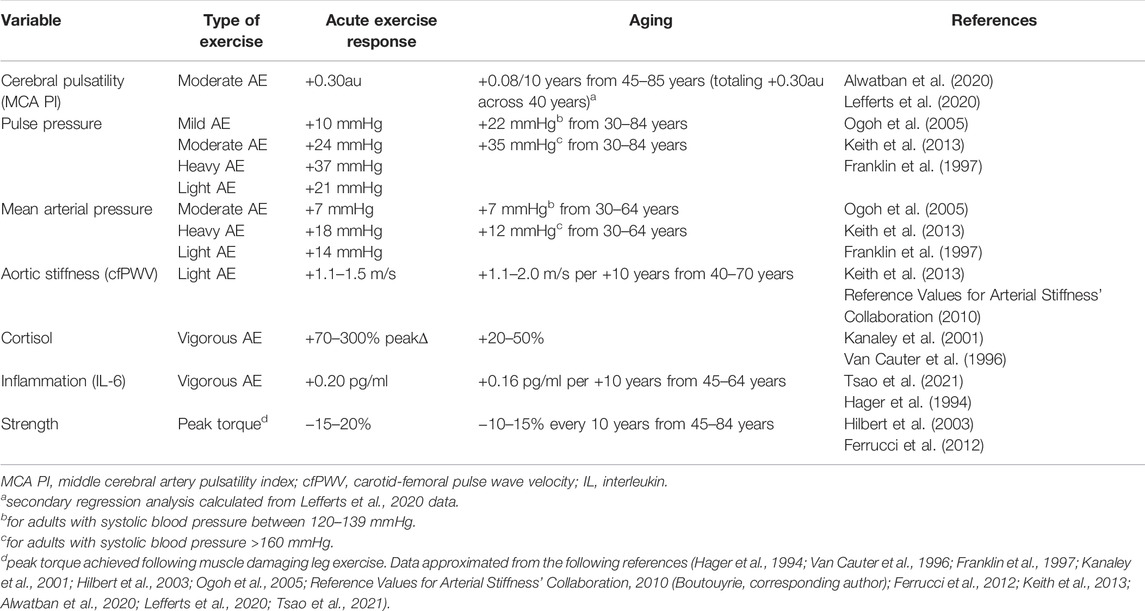
TABLE 1. Comparison of magnitude of acute exercise response with observed changes in the context of aging from select available literature.
In this paper we presented an amalgam of acute exercise literature, including aerobic and resistance exercise across a spectrum of exercise intensities. It is currently unclear whether one specific type of exercise is a better “aging-mimetic” and thus more protective against age-related disease. This gap in understanding reflects methodological limitations [challenges of assessing outcomes during discontinuous exercise (resistance/high-intensity exercise)], and greater attention paid to continuous aerobic over discontinuous aerobic/resistance exercise in the literature. We posit that the exact exercise type is less important than the response it elicits since 1) epidemiological evidence indicates both aerobic and resistance exercise are associated with reduced disease risk (Ross et al., 2016; Bennie et al., 2020) and 2) all forms of exercise disrupt homeostasis (e.g., running, resistance, and high-intensity interval exercise can induce inflammation, increase blood pressure, increase artery stiffness, load bones, damage muscles etc.), and thus may contribute to beneficial adaptations that attenuate physiological aging and reduce disease risk.
The acute effects of exercise are highly variable and may depend, in part, on age. Data indicate that the given response to acute exercise may be preserved (Hogan et al., 2013; Lavin et al., 2020a, 2020b; Luttrell et al., 2020; Rosenberg et al., 2020; MacNeil et al., 2021), exaggerated (Fleg et al., 1985; Fragala et al., 2019; Rosenberg et al., 2020), or blunted (Nordin et al., 2014; Jakovljevic, 2018; Fragala et al., 2019; Rosenberg et al., 2020) with aging, and that these conflicting responses could occur simultaneously depending on the physiological systems in question. This variable effects of age on acute exercise responses may alter the physiological stimulus that elicits adaptations to repeated exercise in older adults. It is possible that either 1) the attenuated physiological response to exercise (e.g., blunted stimulus), or 2) reduced plasticity/sensitivity (Slivka et al., 2008; Greig et al., 2011; Haran et al., 2012) to a similar or exaggerated exercise response could render exercise somewhat less potent or less beneficial among older adults. Indeed, it appears that greater exercise stimuli is required to elicit measurable physiological adaptations among older adults (Fujimoto et al., 2010). Despite reductions in plasticity and altered exercise responses among older adults, exercise training can elicit physiological adaptations in aged individuals (improved muscular, metabolic, cardiovascular function) (Fujimoto et al., 2010; Carrick-Ranson et al., 2014; Vigorito and Giallauria, 2014; Fragala et al., 2019; Green et al., 2021; Grevendonk et al., 2021) that may even be similar to benefits in young adults (Stratton et al., 1994) and ultimately increase cardiorespiratory fitness (Stratton et al., 1994; Woo et al., 2006; Fujimoto et al., 2010). Taken together, data are clear that despite potentially different acute responses and degree of adaptation to exercise, the cumulative effects of exercise are beneficial in older adults and contribute to reduced disease/mortality risk (Bijnen et al., 1998; Sundquist et al., 2004; Carrick-Ranson et al., 2014; Osawa et al., 2021). It should be underscored that the benefits of exercise are wrought over a lifetime of repeated exposure and thus engaging in regular exercise throughout life elicits greater physiological adaptations and health benefits than exercise initiated only later in life (Russ and Kent-Braun, 2004; Fujimoto et al., 2010; Seals et al., 2019; Lavin et al., 2020a).
Future Directions, Applications, and Conclusion
Future work should seek to leverage technological advances and innovative methods to further explore acute cellular/physiological responses during exercise. The following recommendations are suggested to fill knowledge gaps surrounding the idea of exercise as an aging mimetic and the protective effects of exercise on age-related chronic disease risk: 1) examine mechanisms behind the beneficial effects of low-intensity exercise on age-related chronic disease, which remains under explored owing to more optimal signal-to-noise ratio observed with moderate-to-vigorous intensity exercise; 2) better identify and understand the phenotypic “signature” and physiological disruption caused by discontinuous exercise types (e.g., resistance, high-intensity interval) compared to continuous aerobic exercise; and 3) better understand the role of individual characteristics (age, sex, health status) in governing acute exercise responses and subsequent exercise-induced adaptation. Additionally, research often interrogates acute exercise to gain insight into training-induced adaptations under the guise that responses following acute exercise should be positive and contribute to beneficial long-term adaptations (Dawson et al., 2018; Voss et al., 2020). In reality, it is important to recognize that exercise is a potent disruption of homeostasis that mimics responses seen with aging and age-related chronic disease (i.e., exercise is disruptive and not necessarily immediately beneficial for physiological systems). It is this insult to homeostasis that primes adaptations to protect against chronic, age-related changes and reduce disease risk over time (Figure 2D). If research shifts to focus on the homeostatic disruption incurred during exercise, we may better understand the stimulus for adaptation and thus the mechanisms that govern adaptations to exercise and prevent age-related chronic disease.
Ultimately, we posit that regular exercise protects against aging and age-related chronic disease because each bout of exercise is, at its essence, an aging mimetic. The resilience and plasticity of the human body permit adaptations to these repeated exercise-induced “aging” stimuli and ultimately prepares the body’s defenses against the stress of aging and age-related chronic disease.
Data Availability Statement
The original contributions presented in the study are included in the article/Supplementary Material, further inquiries can be directed to the corresponding author.
Author Contributions
WL conceived, drafted, edited, and revised the paper/figures; and MD and RV drafted, edited, and revised the paper. All authors approved the final version of the paper.
Conflict of Interest
The authors declare that the research was conducted in the absence of any commercial or financial relationships that could be construed as a potential conflict of interest.
Publisher’s Note
All claims expressed in this article are solely those of the authors and do not necessarily represent those of their affiliated organizations, or those of the publisher, the editors and the reviewers. Any product that may be evaluated in this article, or claim that may be made by its manufacturer, is not guaranteed or endorsed by the publisher.
References
Abdellatif M., Sedej S., Carmona-Gutierrez D., Madeo F., Kroemer G. (2018). Autophagy in Cardiovascular Aging. Circ. Res. 123, 803–824. doi:10.1161/CIRCRESAHA.118.312208
Ahlborg G., Felig P., Hagenfeldt L., Hendler R., Wahren J. (1974). Substrate Turnover during Prolonged Exercise in Man. J. Clin. Invest. 53, 1080–1090. doi:10.1172/JCI107645
Alwatban M. R., Liu Y., Perdomo S. J., Ward J. L., Vidoni E. D., Burns J. M., et al. (2020). TCD Cerebral Hemodynamic Changes during Moderate‐Intensity Exercise in Older Adults. J. Neuroimaging 30, 76–81. doi:10.1111/jon.12675
Andersen K., Farahmand B., Ahlbom A., Held C., Ljunghall S., Michaëlsson K., et al. (2013). Risk of Arrhythmias in 52 755 Long-Distance Cross-Country Skiers: a Cohort Study. Eur. Heart J. 34, 3624–3631. doi:10.1093/eurheartj/eht188
Aragón-Vela J., Fontana L., Casuso R. A., Plaza-Díaz J., R. Huertas J. (2021). Differential Inflammatory Response of Men and Women Subjected to an Acute Resistance Exercise. Biomed. J. 44, 338–345. doi:10.1016/j.bj.2020.02.005
Armentano R. L., Levenson J., Barra J. G., Fischer E. I., Breitbart G. J., Pichel R. H., et al. (1991). Assessment of Elastin and Collagen Contribution to Aortic Elasticity in Conscious Dogs. Am. J. Physiology-Heart Circulatory Physiology 260, H1870–H1877. doi:10.1152/ajpheart.1991.260.6.H1870
Audiffren M., Tomporowski P. D., Zagrodnik J. (2009). Acute Aerobic Exercise and Information Processing: Modulation of Executive Control in a Random Number Generation Task. Acta Psychol. 132, 85–95. doi:10.1016/j.actpsy.2009.06.008
Aune D., Schlesinger S., Leitzmann M. F., Tonstad S., Norat T., Riboli E., et al. (2021). Physical Activity and the Risk of Heart Failure: a Systematic Review and Dose-Response Meta-Analysis of Prospective Studies. Eur. J. Epidemiol. 36, 367–381. doi:10.1007/s10654-020-00693-6
Balaban R. S., Nemoto S., Finkel T. (2005). Mitochondria, Oxidants, and Aging. Cell 120, 483–495. doi:10.1016/j.cell.2005.02.001
Bauer U. E., Briss P. A., Goodman R. A., Bowman B. A. (2014). Prevention of Chronic Disease in the 21st Century: Elimination of the Leading Preventable Causes of Premature Death and Disability in the USA. Lancet 384, 45–52. doi:10.1016/S0140-6736(14)60648-6
Bennie J. A., Shakespear-Druery J., De Cocker K. (2020). Muscle-strengthening Exercise Epidemiology: a New Frontier in Chronic Disease Prevention. Sports Med. - Open 6, 40. doi:10.1186/s40798-020-00271-w
Bernecker C., Scherr J., Schinner S., Braun S., Scherbaum W. A., Halle M. (2013). Evidence for an Exercise Induced Increase of TNF-α and IL-6 in Marathon Runners. Scand. J. Med. Sci. Sports 23, 207–214. doi:10.1111/j.1600-0838.2011.01372.x
Bhella P. S., Hastings J. L., Fujimoto N., Shibata S., Carrick-Ranson G., Palmer M. D., et al. (2014). Impact of Lifelong Exercise “Dose” on Left Ventricular Compliance and Distensibility. J. Am. Coll. Cardiol. 64, 1257–1266. doi:10.1016/j.jacc.2014.03.062
Bijnen F. C. H., Caspersen C. J., Feskens E. J. M., Saris W. H. M., Mosterd W. L., Kromhout D. (1998). Physical Activity and 10-Year Mortality from Cardiovascular Diseases and All Causes. Arch. Intern. Med. 158, 1499–1505. doi:10.1001/archinte.158.14.1499
Bond V., Mills R. M., Caprarola M., Vaccaro P., Adams R. G., Blakely R., et al. (1999). Aerobic Exercise Attenuates Blood Pressure Reactivity to Cold Pressor Test in Normotensive, Young Adult African-American Women. Ethn. Dis. 9, 104–110.
Booth F. W., Laye M. J. (2009). Lack of Adequate Appreciation of Physical Exercise's Complexities Can Pre-empt Appropriate Design and Interpretation in Scientific Discovery. J. Physiol. 587, 5527–5539. doi:10.1113/jphysiol.2009.179507
Booth F. W., Roberts C. K., Laye M. J. (2012). Lack of Exercise Is a Major Cause of Chronic Diseases. Compr. Physiol. 2, 1143–1211. doi:10.1002/cphy.c110025
Bouzid M. A., Filaire E., McCall A., Fabre C. (2015). Radical Oxygen Species, Exercise and Aging: An Update. Sports Med. 45, 1245–1261. doi:10.1007/s40279-015-0348-1
Brandenberger G., Follenius M. (1975). Influence of Timing and Intensity of Muscular Exercise on Temporal Patterns of Plasma Cortisol Levels. J. Clin. Endocrinol. Metabolism 40, 845–849. doi:10.1210/jcem-40-5-845
Bratic A., Larsson N.-G. (2013). The Role of Mitochondria in Aging. J. Clin. Invest. 123, 951–957. doi:10.1172/JCI64125
Burguera B., Proctor D., Dietz N., Guo Z., Joyner M., Jensen M. D. (2000). Leg Free Fatty Acid Kinetics during Exercise in Men and Women. Am. J. Physiology-Endocrinology Metabolism 278, E113–E117. doi:10.1152/ajpendo.2000.278.1.E113
Carlson M. C., Hasher L., Connelly S. L., Zacks R. T. (1995). Aging, Distraction, and the Benefits of Predictable Location. Psychol. Aging 10, 427–436. doi:10.1037//0882-7974.10.3.42710.1037/0882-7974.10.3.427
Carnethon M. R. (2009). Physical Activity and Cardiovascular Disease: How Much Is Enough? Am. J. Lifestyle Med. 3, 44S–49S. doi:10.1177/1559827609332737
Carrick-Ranson G., Hastings J. L., Bhella P. S., Fujimoto N., Shibata S., Palmer M. D., et al. (2014). The Effect of Lifelong Exercise Dose on Cardiovascular Function during Exercise. J. Appl. Physiology (1985) 116, 736–745. doi:10.1152/japplphysiol.00342.2013
Carter S. E., Draijer R., Holder S. M., Brown L., Thijssen D. H. J., Hopkins N. D. (2018). Regular Walking Breaks Prevent the Decline in Cerebral Blood Flow Associated with Prolonged Sitting. J. Appl. Physiology (1985) 125, 790–798. doi:10.1152/japplphysiol.00310.2018
Cerqueira É., Marinho D. A., Neiva H. P., Lourenço O. (2019). Inflammatory Effects of High and Moderate Intensity Exercise-A Systematic Review. Front. Physiol. 10, 1550. doi:10.3389/fphys.2019.01550
Chahal H., Drake W. (2007). The Endocrine System and Ageing. J. Pathol. 211, 173–180. doi:10.1002/path.2110
Channer K. S., Jones J. V. (1989). The Contribution of Atrial Systole to Mitral Diastolic Blood Flow Increases during Exercise in Humans. J. Physiol. 411, 53–61. doi:10.1113/jphysiol.1989.sp017559
Church T. S., Blair S. N. (2009). When Will We Treat Physical Activity as a Legitimate Medical therapy.Even Though it Does Not Come in a Pill? Br. J. Sports Med. 43, 80–81. doi:10.1136/bjsm.2008.053850
Claessen G., Colyn E., La Gerche A., Koopman P., Alzand B., Garweg C., et al. (2011). Long-term Endurance Sport Is a Risk Factor for Development of Lone Atrial Flutter. Heart 97, 918–922. doi:10.1136/hrt.2010.216150
Coen P. M., Musci R. V., Hinkley J. M., Miller B. F. (2018). Mitochondria as a Target for Mitigating Sarcopenia. Front. Physiol. 9, 1883. doi:10.3389/fphys.2018.01883
Corazza D. I., Sebastião É., Pedroso R. V., Andreatto C. A. A., de Melo Coelho F. G., Gobbi S., et al. (2014). Influence of Chronic Exercise on Serum Cortisol Levels in Older Adults. Eur. Rev. Aging Phys. Act. 11, 25–34. doi:10.1007/s11556-013-0126-8
Corbett J., Neal R. A., Lunt H. C., Tipton M. J. (2014). Adaptation to Heat and Exercise Performance under Cooler Conditions: a New Hot Topic. Sports Med. 44, 1323–1331. doi:10.1007/s40279-014-0212-8
Curtis E., Litwic A., Cooper C., Dennison E. (2015). Determinants of Muscle and Bone Aging. J. Cell. Physiol. 230, 2618–2625. doi:10.1002/jcp.25001
Davalli P., Mitic T., Caporali A., Lauriola A., D’Arca D. (2016). ROS, Cell Senescence, and Novel Molecular Mechanisms in Aging and Age-Related Diseases. Oxidative Med. Cell. Longev. 2016, 3565127. doi:10.1155/2016/3565127
Dawson E. A., Cable N. T., Green D. J., Thijssen D. H. J. (2018). Do acute Effects of Exercise on Vascular Function Predict Adaptation to Training? Eur. J. Appl. Physiol. 118, 523–530. doi:10.1007/s00421-017-3724-8
de Jonge L., Moreira E. A. M., Martin C. K., Ravussin E.Pennington CALERIE Team (2010). Impact of 6-month Caloric Restriction on Autonomic Nervous System Activity in Healthy, Overweight, Individuals. Obes. Silver Spring Md 18, 414–416. doi:10.1038/oby.2009.408
de Montgolfier O., Pinçon A., Pouliot P., Gillis M.-A., Bishop J., Sled J. G., et al. (2019). High Systolic Blood Pressure Induces Cerebral Microvascular Endothelial Dysfunction, Neurovascular Unit Damage, and Cognitive Decline in Mice. Hypertension 73, 217–228. doi:10.1161/HYPERTENSIONAHA.118.12048
Dibe H. A., Townsend L. K., McKie G. L., Wright D. C. (2020). Epinephrine Responsiveness Is Reduced in Livers from Trained Mice. Physiol. Rep. 8, e14370. doi:10.14814/phy2.14370
Donato A. J., Morgan R. G., Walker A. E., Lesniewski L. A. (2015). Cellular and Molecular Biology of Aging Endothelial Cells. J. Mol. Cell. Cardiol. 89, 122–135. doi:10.1016/j.yjmcc.2015.01.021
Eijsvogels T. M. H., Thompson P. D., Franklin B. A. (2018). The “Extreme Exercise Hypothesis”: Recent Findings and Cardiovascular Health Implications. Curr. Treat. Options Cardio Med. 20, 84. doi:10.1007/s11936-018-0674-3
Ekelund U., Tarp J., Steene-Johannessen J., Hansen B. H., Jefferis B., Fagerland M. W., et al. (2019). Dose-response Associations between Accelerometry Measured Physical Activity and Sedentary Time and All Cause Mortality: Systematic Review and Harmonised Meta-Analysis. BMJ 366, l4570. doi:10.1136/bmj.l4570
Feller S., Vigl M., Bergmann M. M., Boeing H., Kirschbaum C., Stalder T. (2014). Predictors of Hair Cortisol Concentrations in Older Adults. Psychoneuroendocrinology 39, 132–140. doi:10.1016/j.psyneuen.2013.10.007
Feng X., McDonald J. M. (2011). Disorders of Bone Remodeling. Annu. Rev. Pathol. Mech. Dis. 6, 121–145. doi:10.1146/annurev-pathol-011110-130203
Fernando R., Drescher C., Nowotny K., Grune T., Castro J. P. (2019). Impaired Proteostasis during Skeletal Muscle Aging. Free Radic. Biol. Med. 132, 58–66. doi:10.1016/j.freeradbiomed.2018.08.037
Ferrucci L., de Cabo R., Knuth N. D., Studenski S. (2012). Of Greek Heroes, Wiggling Worms, Mighty Mice, and Old Body Builders. Journals Gerontology Ser. A Biol. Sci. Med. Sci. 67A, 13–16. doi:10.1093/gerona/glr046
Ferrucci L., Fabbri E. (2018). Inflammageing: Chronic Inflammation in Ageing, Cardiovascular Disease, and Frailty. Nat. Rev. Cardiol. 15, 505–522. doi:10.1038/s41569-018-0064-2
Ferrucci L., Semba R. D., Guralnik J. M., Ershler W. B., Bandinelli S., Patel K. V., et al. (2010). Proinflammatory State, Hepcidin, and Anemia in Older Persons. Blood 115, 3810–3816. doi:10.1182/blood-2009-02-201087
Fischer C. P. (2006). Interleukin-6 in Acute Exercise and Training: what Is the Biological Relevance? Exerc. Immunol. Rev. 12, 6–33.
Fisher-Wellman K., Bloomer R. J. (2009). Acute Exercise and Oxidative Stress: a 30 Year History. Dyn. Med. 8, 1. doi:10.1186/1476-5918-8-1
Fleg J. L., Tzankoff S. P., Lakatta E. G. (1985). Age-related Augmentation of Plasma Catecholamines during Dynamic Exercise in Healthy Males. J. Appl. Physiology (1985) 59, 1033–1039. doi:10.1152/jappl.1985.59.4.1033
Fonseca T. R., Mendes T. T., Ramos G. P., Cabido C. E. T., Morandi R. F., Ferraz F. O., et al. (2021). Aerobic Training Modulates the Increase in Plasma Concentrations of Cytokines in Response to a Session of Exercise. J. Environ. Public Health 2021, 1304139. doi:10.1155/2021/1304139
Fragala M. S., Cadore E. L., Dorgo S., Izquierdo M., Kraemer W. J., Peterson M. D., et al. (2019). Resistance Training for Older Adults. J. Strength Cond. Res. 33, 2019–2052. doi:10.1519/JSC.0000000000003230
Franklin S. S., Gustin W., Wong N. D., Larson M. G., Weber M. A., Kannel W. B., et al. (1997). Hemodynamic Patterns of Age-Related Changes in Blood Pressure. Circulation 96, 308–315. doi:10.1161/01.cir.96.1.308
Fridén J., Lieber R. L. (1992). Structural and Mechanical Basis of Exercise-Induced Muscle Injury. Med. Sci. Sports Exerc. 24, 521–530. doi:10.1249/00005768-199205000-00005
Fujimoto N., Prasad A., Hastings J. L., Arbab-Zadeh A., Bhella P. S., Shibata S., et al. (2010). Cardiovascular Effects of 1 Year of Progressive and Vigorous Exercise Training in Previously Sedentary Individuals Older Than 65 Years of Age. Circulation 122, 1797–1805. doi:10.1161/CIRCULATIONAHA.110.973784
Fusi S., Cutuli D., Valente M. R., Bergonzi P., Porro C. A., Di Prampero P. E. (2005). Cardioventilatory Responses during Real or Imagined Walking at Low Speed. Arch. Ital. Biol. 143, 223–228.
Gamba P., Testa G., Gargiulo S., Staurenghi E., Poli G., Leonarduzzi G. (2015). Oxidized Cholesterol as the Driving Force behind the Development of Alzheimer's Disease. Front. Aging Neurosci. 7, 119. doi:10.3389/fnagi.2015.00119
Garcia-Polite F., Martorell J., Del Rey-Puech P., Melgar-Lesmes P., O’Brien C. C., Roquer J., et al. (2017). Pulsatility and High Shear Stress Deteriorate Barrier Phenotype in Brain Microvascular Endothelium. J. Cereb. Blood Flow. Metab. 37, 2614–2625. doi:10.1177/0271678X16672482
Genova M. L., Lenaz G. (2015). The Interplay between Respiratory Supercomplexes and ROS in Aging. Antioxidants Redox Signal. 23, 208–238. doi:10.1089/ars.2014.6214
Girousse A., Virtue S., Hart D., Vidal-Puig A., Murgatroyd P. R., Mouisel E., et al. (2018). Surplus Fat Rapidly Increases Fat Oxidation and Insulin Resistance in Lipodystrophic Mice. Mol. Metab. 13, 24–29. doi:10.1016/j.molmet.2018.05.006
Gonzales J. U., Thompson B. C., Thistlethwaite J. R., Scheuermann B. W. (2011). Association between Exercise Hemodynamics and Changes in Local Vascular Function Following Acute Exercise. Appl. Physiol. Nutr. Metab. 36, 137–144. doi:10.1139/H10-097
Green D. J., Hopman M. T. E., Padilla J., Laughlin M. H., Thijssen D. H. J. (2017). Vascular Adaptation to Exercise in Humans: Role of Hemodynamic Stimuli. Physiol. Rev. 97, 495–528. doi:10.1152/physrev.00014.2016
Green D. J., Smith K., Maslen B. A., Cox K. L., Lautenschlager N. T., Pestell C. F., et al. (2021). The Impact of 6-Month Land versus Water Walking on Cerebrovascular Function in the Aging Brain. Med. Sci. Sports Exerc. 53, 2093–2100. doi:10.1249/MSS.0000000000002685
Greig C. A., Gray C., Rankin D., Young A., Mann V., Noble B., et al. (2011). Blunting of Adaptive Responses to Resistance Exercise Training in Women over 75y. Exp. Gerontol. 46, 884–890. doi:10.1016/j.exger.2011.07.010
Grevendonk L., Connell N. J., McCrum C., Fealy C. E., Bilet L., Bruls Y. M. H., et al. (2021). Impact of Aging and Exercise on Skeletal Muscle Mitochondrial Capacity, Energy Metabolism, and Physical Function. Nat. Commun. 12, 4773. doi:10.1038/s41467-021-24956-2
Guo C., Sun L., Chen X., Zhang D. (2013). Oxidative Stress, Mitochondrial Damage and Neurodegenerative Diseases. Neural Regen. Res. 8, 2003–2014. doi:10.3969/j.issn.1673-5374.2013.21.009
Hager K., Machein U., Krieger S., Platt D., Seefried G., Bauer J. (1994). Interleukin-6 and Selected Plasma Proteins in Healthy Persons of Different Ages. Neurobiol. Aging 15, 771–772. doi:10.1016/0197-4580(94)90066-3
Harada C. N., Natelson Love M. C., Triebel K. L. (2013). Normal Cognitive Aging. Clin. Geriatric Med. 29, 737–752. doi:10.1016/j.cger.2013.07.002
Haran P. H., Rivas D. A., Fielding R. A. (2012). Role and Potential Mechanisms of Anabolic Resistance in Sarcopenia. J. Cachexia Sarcopenia Muscle 3, 157–162. doi:10.1007/s13539-012-0068-4
Havel R. J., Naimark A., Borchgrevink C. F. (1963). Turnover Rate and Oxidation of Free Fatty Acids of Blood Plasma in Man during Exercise: Studies during Continuous Infusion of Palmitate-1-C14*. J. Clin. Invest. 42, 1054–1063. doi:10.1172/JCI104791
Hawley J. A., Hargreaves M., Joyner M. J., Zierath J. R. (2014). Integrative Biology of Exercise. Cell 159, 738–749. doi:10.1016/j.cell.2014.10.029
Hawley J. A., Lundby C., Cotter J. D., Burke L. M. (2018). Maximizing Cellular Adaptation to Endurance Exercise in Skeletal Muscle. Cell Metab. 27, 962–976. doi:10.1016/j.cmet.2018.04.014
Hayden K. M., Welsh-Bohmer K. A. (2011). Epidemiology of Cognitive Aging and Alzheimer's Disease: Contributions of the Cache County Utah Study of Memory, Health and Aging. Curr. Top. Behav. Neurosci. 10, 3–31. doi:10.1007/7854_2011_152
Heckmann J. G., Hilz M. J., Mück-Weymann M., Neundörfer B. (2000). Transcranial Doppler Sonography-Ergometer Test for the Non-invasive Assessment of Cerebrovascular Autoregulation in Humans. J. Neurological Sci. 177, 41–47. doi:10.1016/s0022-510x(00)00330-0
Heled Y., Peled A., Yanovich R., Shargal E., Pilz-Burstein R., Epstein Y., et al. (2012). Heat Acclimation and Performance in Hypoxic Conditions. Aviat. Space Environ. Med. 83, 649–653. doi:10.3357/asem.3241.2012
Henskens L. H. G., Kroon A. A., van Oostenbrugge R. J., Gronenschild E. H. B. M., Fuss-Lejeune M. M. J. J., Hofman P. A. M., et al. (2008). Increased Aortic Pulse Wave Velocity Is Associated with Silent Cerebral Small-Vessel Disease in Hypertensive Patients. Hypertension 52, 1120–1126. doi:10.1161/HYPERTENSIONAHA.108.119024
Hilbert J. E., Sforzo G. A., Swensen T. (2003). The Effects of Massage on Delayed Onset Muscle Soreness. Br. J. Sports Med. 37, 72–75. doi:10.1136/bjsm.37.1.72
Hogan C. L., Mata J., Carstensen L. L. (2013). Exercise Holds Immediate Benefits for Affect and Cognition in Younger and Older Adults. Psychol. Aging 28, 587–594. doi:10.1037/a0032634
Howatson G., van Someren K. A. (2008). The Prevention and Treatment of Exercise-Induced Muscle Damage. Sports Med. 38, 483–503. doi:10.2165/00007256-200838060-00004
Jakovljevic D. G. (2018). Physical Activity and Cardiovascular Aging: Physiological and Molecular Insights. Exp. Gerontol. 109, 67–74. doi:10.1016/j.exger.2017.05.016
Jandackova V. K., Scholes S., Britton A., Steptoe A. (2016). Are Changes in Heart Rate Variability in Middle‐Aged and Older People Normative or Caused by Pathological Conditions? Findings from a Large Population‐Based Longitudinal Cohort Study. Jaha 5, e002365. doi:10.1161/JAHA.115.002365
Jiang Z.-L., Yamaguchi H., Takahashi A., Tanabe S., Utsuyama N., Ikehara T., et al. (1995). Blood Flow Velocity in the Common Carotid Artery in Humans during Graded Exercise on a Treadmill. Eur. J. Appl. Physiol. 70, 234–239. doi:10.1007/BF00238569
Jufri N. F., Mohamedali A., Avolio A., Baker M. S. (2015). Mechanical Stretch: Physiological and Pathological Implications for Human Vascular Endothelial Cells. Vasc. Cell 7, 8. doi:10.1186/s13221-015-0033-z
Jurva J. W., Phillips S. A., Syed A. Q., Syed A. Y., Pitt S., Weaver A., et al. (2006). The Effect of Exertional Hypertension Evoked by Weight Lifting on Vascular Endothelial Function. J. Am. Coll. Cardiol. 48, 588–589. doi:10.1016/j.jacc.2006.05.004
Kaess B. M., Rong J., Larson M. G., Hamburg N. M., Vita J. A., Levy D., et al. (2012). Aortic Stiffness, Blood Pressure Progression, and Incident Hypertension. JAMA 308, 875–881. doi:10.1001/2012.jama.10503
Kanaley J. A., Weltman J. Y., Pieper K. S., Weltman A., Hartman M. L. (2001). Cortisol and Growth Hormone Responses to Exercise at Different Times of Day1. J. Clin. Endocrinol. Metabolism 86, 2881–2889. doi:10.1210/jcem.86.6.7566
Keith L. J., Rattigan S., Keske M. A., Jose M., Sharman J. E. (2013). Exercise Aortic Stiffness: Reproducibility and Relation to End-Organ Damage in Men. J. Hum. Hypertens. 27, 516–522. doi:10.1038/jhh.2013.5
Kennedy B. K., Berger S. L., Brunet A., Campisi J., Cuervo A. M., Epel E. S., et al. (2014). Geroscience: Linking Aging to Chronic Disease. Cell 159, 709–713. doi:10.1016/j.cell.2014.10.039
Kim J. K., Fillmore J. J., Chen Y., Yu C., Moore I. K., Pypaert M., et al. (2001). Tissue-specific Overexpression of Lipoprotein Lipase Causes Tissue-specific Insulin Resistance. Proc. Natl. Acad. Sci. U.S.A. 98, 7522–7527. doi:10.1073/pnas.121164498
Kirova A.-M., Bays R. B., Lagalwar S. (2015). Working Memory and Executive Function Decline across Normal Aging, Mild Cognitive Impairment, and Alzheimer's Disease. BioMed Res. Int. 2015, 748212. doi:10.1155/2015/748212
Ko G. T., Wai H. P., Tang J. S. (2006). Effects of Age on Plasma Glucose Levels in Non-diabetic Hong Kong Chinese. Croat. Med. J. 47, 709–713.
Koopman R., van Loon L. J. C. (2009). Aging, Exercise, and Muscle Protein Metabolism. J. Appl. Physiology (1985) 106, 2040–2048. doi:10.1152/japplphysiol.91551.2008
Kudryavtseva A. V., Krasnov G. S., Dmitriev A. A., Alekseev B. Y., Kardymon O. L., Sadritdinova A. F., et al. (2016). Mitochondrial Dysfunction and Oxidative Stress in Aging and Cancer. Oncotarget 7, 44879–44905. doi:10.18632/oncotarget.9821
Kumar V., Atherton P., Smith K., Rennie M. J. (2009). Human Muscle Protein Synthesis and Breakdown during and after Exercise. J. Appl. Physiology (1985) 106, 2026–2039. doi:10.1152/japplphysiol.91481.2008
Lakatta E. G., Levy D. (2003). Arterial and Cardiac Aging: Major Shareholders in Cardiovascular Disease Enterprises. Circulation 107, 139–146. doi:10.1161/01.cir.0000048892.83521.58
Lambourne K., Tomporowski P. (2010). The Effect of Exercise-Induced Arousal on Cognitive Task Performance: a Meta-Regression Analysis. Brain Res. 1341, 12–24. doi:10.1016/j.brainres.2010.03.091
LaRocca T. J., Martens C. R., Seals D. R. (2017). Nutrition and Other Lifestyle Influences on Arterial Aging. Ageing Res. Rev. 39, 106–119. doi:10.1016/j.arr.2016.09.002
Lavin K. M., Perkins R. K., Jemiolo B., Raue U., Trappe S. W., Trappe T. A. (2020a). Effects of Aging and Lifelong Aerobic Exercise on Basal and Exercise-Induced Inflammation. J. Appl. Physiology (1985) 128, 87–99. doi:10.1152/japplphysiol.00495.2019
Lavin K. M., Perkins R. K., Jemiolo B., Raue U., Trappe S. W., Trappe T. A. (2020b). Effects of Aging and Lifelong Aerobic Exercise on Basal and Exercise-Induced Inflammation in Women. J. Appl. Physiology (1985) 129, 1493–1504. doi:10.1152/japplphysiol.00655.2020
Lefferts W. K., DeBlois J. P., Augustine J. A., Keller A. P., Heffernan K. S. (2020). Age, Sex, and the Vascular Contributors to Cerebral Pulsatility and Pulsatile Damping. J. Appl. Physiology (1985) 129, 1092–1101. doi:10.1152/japplphysiol.00500.2020
Liberale L., Montecucco F., Tardif J.-C., Libby P., Camici G. G. (2020). Inflamm-ageing: the Role of Inflammation in Age-dependent Cardiovascular Disease. Eur. Heart J. 41, 2974–2982. doi:10.1093/eurheartj/ehz961
Lovic D., Narayan P., Pittaras A., Faselis C., Doumas M., Kokkinos P. (2017). Left Ventricular Hypertrophy in Athletes and Hypertensive Patients. J. Clin. Hypertens. 19, 413–417. doi:10.1111/jch.12977
Luca M., Luca A. (2019). Oxidative Stress-Related Endothelial Damage in Vascular Depression and Vascular Cognitive Impairment: Beneficial Effects of Aerobic Physical Exercise. Oxidative Med. Cell. Longev. 2019, 8067045. doi:10.1155/2019/8067045
Lucia C. d., Eguchi A., Koch W. J. (2018). New Insights in Cardiac β-Adrenergic Signaling during Heart Failure and Aging. Front. Pharmacol. 9, 904. doi:10.3389/fphar.2018.00904
Luttrell M. J., Mardis B. R., Bock J. M., Iwamoto E., Hanada S., Ueda K., et al. (2021). Effect of Age and Acute Exercise on Circulating Angioregulatory Factors. J. Aging Phys. Act. 29, 423–430. doi:10.1123/japa.2020-0024
Maciejczyk M., Wiecek M., Szymura J., Szygula Z. (2016). Energy Expenditure and Physiological Responses during Walking on a Treadmill and Moving on the Torqway Vehicle. Acta Bioeng. Biomech. 18, 137–143.
MacNeil L. G., Tarnopolsky M. A., Crane J. D. (2021). Acute, Exercise-Induced Alterations in Cytokines and Chemokines in the Blood Distinguish Physically Active and Sedentary Aging. J. Gerontol. A. Biol. Sci. Med. Sci. 76, 811–818. doi:10.1093/gerona/glaa310
Magnusson I., Rothman D. L., Katz L. D., Shulman R. G., Shulman G. I. (1992). Increased Rate of Gluconeogenesis in Type II Diabetes Mellitus. A 13C Nuclear Magnetic Resonance Study. J. Clin. Invest. 90, 1323–1327. doi:10.1172/JCI115997
Małkiewicz M. A., Szarmach A., Sabisz A., Cubała W. J., Szurowska E., Winklewski P. J. (2019). Blood-brain Barrier Permeability and Physical Exercise. J. Neuroinflammation 16, 15. doi:10.1186/s12974-019-1403-x
Marseglia L., Manti S., D’Angelo G., Nicotera A., Parisi E., Di Rosa G., et al. (2014). Oxidative Stress in Obesity: a Critical Component in Human Diseases. Ijms 16, 378–400. doi:10.3390/ijms16010378
McDonagh B., Sakellariou G. K., Jackson M. J. (2014). Application of Redox Proteomics to Skeletal Muscle Aging and Exercise. Biochem. Soc. Trans. 42, 965–970. doi:10.1042/BST20140085
McGee S. L., Hargreaves M. (2020). Exercise Adaptations: Molecular Mechanisms and Potential Targets for Therapeutic Benefit. Nat. Rev. Endocrinol. 16, 495–505. doi:10.1038/s41574-020-0377-1
McPhee J. S., French D. P., Jackson D., Nazroo J., Pendleton N., Degens H. (2016). Physical Activity in Older Age: Perspectives for Healthy Ageing and Frailty. Biogerontology 17, 567–580. doi:10.1007/s10522-016-9641-0
Michael S., Graham K. S., Davis G. M. (2017). Cardiac Autonomic Responses during Exercise and Post-exercise Recovery Using Heart Rate Variability and Systolic Time Intervals-A Review. Front. Physiol. 8, 301. doi:10.3389/fphys.2017.00301
Millgård J., Lind L. (1998). Acute Hypertension Impairs Endothelium-dependent Vasodilatation. Clin. Sci. Lond. Engl. 94, 601–607. doi:10.1042/cs0940601
Mitchell G. F. (2014). Arterial Stiffness and Hypertension. Hypertension 64, 210–214. doi:10.1161/HYPERTENSIONAHA.114.03449
Morishima T., Padilla J., Tsuchiya Y., Ochi E. (2020). Maintenance of Endothelial Function Following Acute Resistance Exercise in Females Is Associated with a Tempered Blood Pressure Response. J. Appl. Physiology 1985 129, 792–799. doi:10.1152/japplphysiol.00378.2020
Murphy S. L., Xu J., Kochanek K. D., Arias E. (2018). Mortality in the United States, 2017. NCHS Data Brief., 1–8.
Najjar S. S., Scuteri A., Shetty V., Wright J. G., Muller D. C., Fleg J. L., et al. (2008). Pulse Wave Velocity Is an Independent Predictor of the Longitudinal Increase in Systolic Blood Pressure and of Incident Hypertension in the Baltimore Longitudinal Study of Aging. J. Am. Coll. Cardiol. 51, 1377–1383. doi:10.1016/j.jacc.2007.10.065
Neilan T. G., Januzzi J. L., Lee-Lewandrowski E., Ton-Nu T.-T., Yoerger D. M., Jassal D. S., et al. (2006). Myocardial Injury and Ventricular Dysfunction Related to Training Levels Among Nonelite Participants in the Boston Marathon. Circulation 114, 2325–2333. doi:10.1161/CIRCULATIONAHA.106.647461
Nordin T. C., Done A. J., Traustadóttir T. (2014). Acute Exercise Increases Resistance to Oxidative Stress in Young but Not Older Adults. Age 36, 9727. doi:10.1007/s11357-014-9727-z
O'Keefe J. H., Patil H. R., Lavie C. J., Magalski A., Vogel R. A., McCullough P. A. (2012). Potential Adverse Cardiovascular Effects from Excessive Endurance Exercise. Mayo Clin. Proc. 87, 587–595. doi:10.1016/j.mayocp.2012.04.005
Ogoh S., Fadel P. J., Zhang R., Selmer C., Jans Ø., Secher N. H., et al. (2005). Middle Cerebral Artery Flow Velocity and Pulse Pressure during Dynamic Exercise in Humans. Am. J. Physiology-Heart Circulatory Physiology 288, H1526–H1531. doi:10.1152/ajpheart.00979.2004
O’Keefe E. L., Torres-Acosta N., O’Keefe J. H., Lavie C. J. (2020). Training for Longevity: The Reverse J-Curve for Exercise. Mo. Med. 117, 355–361.
Örlander J., Kiessling K.-H., Karlsson J., Ekblom B. (1977). Low Intensity Training, Inactivity and Resumed Training in Sedentary Men. Acta Physiol. Scand. 101, 351–362. doi:10.1111/j.1748-1716.1977.tb06017.x
Osawa Y., Abe Y., Takayama M., Oguma Y., Arai Y. (2021). Physical Activity and All-Cause Mortality and Mediators of the Association in the Very Old. Exp. Gerontol. 150, 111374. doi:10.1016/j.exger.2021.111374
Paliwal D., McInerney T. W., Pa J., Swerdlow R. H., Easteal S., Andrews S. J., et al. (2021). Mitochondrial Pathway Polygenic Risk Scores Are Associated with Alzheimer's Disease. Neurobiol. Aging 108, 213–222. doi:10.1016/j.neurobiolaging.2021.08.005
Peake J., Nosaka K., Suzuki K. (2005). Characterization of Inflammatory Responses to Eccentric Exercise in Humans. Exerc. Immunol. Rev. 11, 64–85.
Pedersen B. K., Saltin B. (2015). Exercise as Medicine - Evidence for Prescribing Exercise as Therapy in 26 Different Chronic Diseases. Scand. J. Med. Sci. Sports 25 (Suppl. 3), 1–72. doi:10.1111/sms.12581
Petriz B. A., Gomes C. P. C., Almeida J. A., de Oliveira G. P., Ribeiro F. M., Pereira R. W., et al. (2017). The Effects of Acute and Chronic Exercise on Skeletal Muscle Proteome. J. Cell. Physiol. 232, 257–269. doi:10.1002/jcp.25477
Pfeifer M. A., Weinberg C. R., Cook D., Best J. D., Reenan A., Halter J. B. (1983). Differential Changes of Autonomic Nervous System Function with Age in Man. Am. J. Med. 75, 249–258. doi:10.1016/0002-9343(83)91201-9
Pomella N., Wilhelm E. N., Kolyva C., González-Alonso J., Rakobowchuk M., Khir A. W. (2018). Noninvasive Assessment of the Common Carotid Artery Hemodynamics with Increasing Exercise Work Rate Using Wave Intensity Analysis. Am. J. Physiology-Heart Circulatory Physiology 315, H233–H241. doi:10.1152/ajpheart.00667.2017
Powers S. K., Deminice R., Ozdemir M., Yoshihara T., Bomkamp M. P., Hyatt H. (2020). Exercise-induced Oxidative Stress: Friend or Foe? J. Sport Health Sci. 9, 415–425. doi:10.1016/j.jshs.2020.04.001
Powers S. K., Jackson M. J. (2008). Exercise-induced Oxidative Stress: Cellular Mechanisms and Impact on Muscle Force Production. Physiol. Rev. 88, 1243–1276. doi:10.1152/physrev.00031.2007
Powers S. K., Radak Z., Ji L. L. (2016). Exercise-induced Oxidative Stress: Past, Present and Future. J. Physiol. 594, 5081–5092. doi:10.1113/JP270646
Prawiradilaga R., Madsen A., Jørgensen N., Helge E. (2020). Acute Response of Biochemical Bone Turnover Markers and the Associated Ground Reaction Forces to High-Impact Exercise in Postmenopausal Women. bs 37, 41–48. doi:10.5114/biolsport.2020.91497
Quinlan C. L., Perevoshchikova I. V., Hey-Mogensen M., Orr A. L., Brand M. D. (2013). Sites of Reactive Oxygen Species Generation by Mitochondria Oxidizing Different Substrates. Redox Biol. 1, 304–312. doi:10.1016/j.redox.2013.04.005
Rajanayagam J., Alsabri M. (2021). Intense Endurance Exercise: A Potential Risk Factor in the Development of Heart Disease. Cureus 13, e12608. doi:10.7759/cureus.12608
Ranallo R. F., Rhodes E. C. (1998). Lipid Metabolism during Exercise. Sports Med. 26, 29–42. doi:10.2165/00007256-199826010-00003
Reaven G., Chang H., Hoffman B. (1989). Impaired Insulin-Mediated Inhibition of Lipolysis and Glucose Transport with Aging. Horm. Metab. Res. 21, 168–171. doi:10.1055/s-2007-1009183
Reference Values for Arterial Stiffness’ Collaboration (2010). Determinants of Pulse Wave Velocity in Healthy People and in the Presence of Cardiovascular Risk Factors: 'establishing Normal and Reference Values'. Eur. Heart J. 31, 2338–2350. doi:10.1093/eurheartj/ehq165
Renzi C. P., Tanaka H., Sugawara J. (2010). Effects of Leg Blood Flow Restriction during Walking on Cardiovascular Function. Med. Sci. Sports Exerc. 42, 726–732. doi:10.1249/MSS.0b013e3181bdb454
Reynolds T. H., Krajewski K. M., Larkin L. M., Reid P., Halter J. B., Supiano M. A., et al. (2002). Effect of Age on Skeletal Muscle Proteolysis in Extensor Digitorum Longus Muscles of B6C3F1 Mice. Journals Gerontology Ser. A Biol. Sci. Med. Sci. 57, B198–B201. doi:10.1093/gerona/57.5.b198
Riggs B. L., Melton L. J., Robb R. A., Camp J. J., Atkinson E. J., McDaniel L., et al. (2008). A Population-Based Assessment of Rates of Bone Loss at Multiple Skeletal Sites: Evidence for Substantial Trabecular Bone Loss in Young Adult Women and Men. J. Bone Min. Res. 23, 205–214. doi:10.1359/jbmr.071020
Rizza R. A. (2010). Pathogenesis of Fasting and Postprandial Hyperglycemia in Type 2 Diabetes: Implications for Therapy. Diabetes 59, 2697–2707. doi:10.2337/db10-1032
Roh H.-T., Cho S.-Y., Yoon H.-G., So W.-Y. (2017). Effect of Exercise Intensity on Neurotrophic Factors and Blood-Brain Barrier Permeability Induced by Oxidative-Nitrosative Stress in Male College Students. Int. J. Sport Nutr. Exerc. Metab. 27, 239–246. doi:10.1123/ijsnem.2016-0009
Romijn J. A., Coyle E. F., Sidossis L. S., Gastaldelli A., Horowitz J. F., Endert E., et al. (1993). Regulation of Endogenous Fat and Carbohydrate Metabolism in Relation to Exercise Intensity and Duration. Am. J. Physiology-Endocrinology Metabolism 265, E380–E391. doi:10.1152/ajpendo.1993.265.3.E380
Rosenberg A. J., Schroeder E. C., Grigoriadis G., Wee S. O., Bunsawat K., Heffernan K. S., et al. (2020). Aging Reduces Cerebral Blood Flow Regulation Following an Acute Hypertensive Stimulus. J. Appl. Physiology 1985 128, 1186–1195. doi:10.1152/japplphysiol.00137.2019
Ross R., Blair S. N., Arena R., Church T. S., Després J.-P., Franklin B. A., et al. (2016). Importance of Assessing Cardiorespiratory Fitness in Clinical Practice: A Case for Fitness as a Clinical Vital Sign: A Scientific Statement from the American Heart Association. Circulation 134, e653–e699. doi:10.1161/CIR.0000000000000461
Rowell L. B. (1997). Neural Control of Muscle Blood Flow: Importance during Dynamic Exercise. Clin. Exp. Pharmacol. Physiol. 24, 117–125. doi:10.1111/j.1440-1681.1997.tb01793.x
Rowland T., Unnithan V., Fernhall B., Baynard T., Lange C. (2002). Left Ventricular Response to Dynamic Exercise in Young Cyclists. Med. Sci. Sports Exerc. 34, 637–642. doi:10.1097/00005768-200204000-00012
Russ D. W., Kent-Braun J. A. (2004). Is Skeletal Muscle Oxidative Capacity Decreased in Old Age? Sports Med. 34, 221–229. doi:10.2165/00007256-200434040-00002
Sabbahi A., Arena R., Kaminsky L. A., Myers J., Phillips S. A. (2018). Peak Blood Pressure Responses during Maximum Cardiopulmonary Exercise Testing. Hypertension 71, 229–236. doi:10.1161/HYPERTENSIONAHA.117.10116
Sallis R. E. (2009). Exercise Is Medicine and Physicians Need to Prescribe it!. Br. J. Sports Med. 43, 3–4. doi:10.1136/bjsm.2008.054825
Sallis R. (2015). Exercise Is Medicine: a Call to Action for Physicians to Assess and Prescribe Exercise. Physician Sportsmed. 43, 22–26. doi:10.1080/00913847.2015.1001938
Salthouse T. (2012). Consequences of Age-Related Cognitive Declines. Annu. Rev. Psychol. 63, 201–226. doi:10.1146/annurev-psych-120710-100328
Satrústegui J., Cuezva J. M., Machado A. (1986). Increased Basal Gluconeogenesis in the Aged Rat. FEBS Lett. 197, 159–163. doi:10.1016/0014-5793(86)80318-0
Seals D. R., Nagy E. E., Moreau K. L. (2019). Aerobic Exercise Training and Vascular Function with Ageing in Healthy Men and Women. J. Physiol. 597, 4901–4914. doi:10.1113/JP277764
Senoner T., Dichtl W. (2019). Oxidative Stress in Cardiovascular Diseases: Still a Therapeutic Target? Nutrients 11, 2090. doi:10.3390/nu11092090
Sepanlou S. G., Poustchi H., Kamangar F., Malekzadeh R. (2011). Effectiveness and Feasibility of Lifestyle and Low-Cost Pharmacologic Interventions in the Prevention of Chronic Diseases: a Review. Arch. Iran. Med. 14, 46–53.
Sergiev P. V., Dontsova O. A., Berezkin G. V. (2015). Theories of Aging: an Ever-Evolving Field. Acta Naturae 7, 9–18. doi:10.32607/20758251-2015-7-1-9-18
Shanker Sharma H., Cervós-Navarro J., Kumar Dey P. (1991). Increased Blood-Brain Barrier Permeability Following Acute Short-Term Swimming Exercise in Conscious Normotensive Young Rats. Neurosci. Res. 10, 211–221. doi:10.1016/0168-0102(91)90058-7
Sharman J. E., McEniery C. M., Campbell R. I., Coombes J. S., Wilkinson I. B., Cockcroft J. R. (2005). The Effect of Exercise on Large Artery Haemodynamics in Healthy Young Men. Eur. J. Clin. Invest. 35, 738–744. doi:10.1111/j.1365-2362.2005.01578.x
Shi R., Cai Y., Qin R., Yan Y., Yu D. (2020). Dose-response Association between Physical Activity and Clustering of Modifiable Cardiovascular Risk Factors Among 26,093 Chinese Adults. BMC Cardiovasc. Disord. 20, 347. doi:10.1186/s12872-020-01627-6
Shigenaga M. K., Hagen T. M., Ames B. N. (1994). Oxidative Damage and Mitochondrial Decay in Aging. Proc. Natl. Acad. Sci. U.S.A. 91, 10771–10778. doi:10.1073/pnas.91.23.10771
Slivka D., Raue U., Hollon C., Minchev K., Trappe S. (2008). Single Muscle Fiber Adaptations to Resistance Training in Old (>80 Yr) Men: Evidence for Limited Skeletal Muscle Plasticity. Am. J. Physiology-Regulatory, Integr. Comp. Physiology 295, R273–R280. doi:10.1152/ajpregu.00093.2008
Spitler K. M., Davies B. S. J. (2020). Aging and Plasma Triglyceride Metabolism. J. Lipid Res. 61, 1161–1167. doi:10.1194/jlr.R120000922
Stock J. M., Chirinos J. A., Edwards D. G. (2021). Lower‐body Dynamic Exercise Reduces Wave Reflection in Healthy Young Adults. Exp. Physiol. 106, 1720–1730. doi:10.1113/EP089581
Strait J. B., Lakatta E. G. (2012). Aging-associated Cardiovascular Changes and Their Relationship to Heart Failure. Heart Fail. Clin. 8, 143–164. doi:10.1016/j.hfc.2011.08.011
Stratton J. R., Levy W. C., Cerqueira M. D., Schwartz R. S., Abrass I. B. (1994). Cardiovascular Responses to Exercise. Effects of Aging and Exercise Training in Healthy Men. Circulation 89, 1648–1655. doi:10.1161/01.cir.89.4.1648
Studinger P., Lénárd Z., Kováts Z., Kocsis L., Kollai M. (2003). Static and Dynamic Changes in Carotid Artery Diameter in Humans during and after Strenuous Exercise. J. Physiology 550, 575–583. doi:10.1113/jphysiol.2003.040147
Sugawara J., Tomoto T., Tanaka H. (2015). Impact of Leg Blood Flow Restriction during Walking on Central Arterial Hemodynamics. Am. J. Physiology-Regulatory, Integr. Comp. Physiology 309, R732–R739. doi:10.1152/ajpregu.00095.2015
Sundquist K., Qvist J., Sundquist J., Johansson S.-E. (2004). Frequent and Occasional Physical Activity in the Elderly. Am. J. Prev. Med. 27, 22–27. doi:10.1016/j.amepre.2004.03.011
Tagliaferri C., Wittrant Y., Davicco M.-J., Walrand S., Coxam V. (2015). Muscle and Bone, Two Interconnected Tissues. Ageing Res. Rev. 21, 55–70. doi:10.1016/j.arr.2015.03.002
Tanaka H. (20191979). Antiaging Effects of Aerobic Exercise on Systemic Arteries. Hypertension 74, 237–243. doi:10.1161/HYPERTENSIONAHA.119.13179
Tarumi T., Khan M. A., Liu J., Tseng B. M., Parker R., Riley J., et al. (2014). Cerebral Hemodynamics in Normal Aging: Central Artery Stiffness, Wave Reflection, and Pressure Pulsatility. J. Cereb. Blood Flow. Metab. 34, 971–978. doi:10.1038/jcbfm.2014.44
Tipton K. D., Wolfe R. R. (1998). Exercise-induced Changes in Protein Metabolism. Acta Physiol. Scand. 162, 377–387. doi:10.1046/j.1365-201X.1998.00306.x
Tonkonogi M., Sahlin K. (2002). Physical Exercise and Mitochondrial Function in Human Skeletal Muscle. Exerc. Sport Sci. Rev. 30, 129–137. doi:10.1097/00003677-200207000-00007
Townsend R. R., Wilkinson I. B., Schiffrin E. L., Avolio A. P., Chirinos J. A., Cockcroft J. R., et al. (2015). Recommendations for Improving and Standardizing Vascular Research on Arterial Stiffness. Hypertension 66, 698–722. doi:10.1161/HYP.0000000000000033
Tsao J.-P., Liu C.-C., Wang H.-F., Bernard J. R., Huang C.-C., Cheng I.-S. (2021). Oral Resveratrol Supplementation Attenuates Exercise-Induced Interleukin-6 but Not Oxidative Stress after a High Intensity Cycling Challenge in Adults. Int. J. Med. Sci. 18, 2137–2145. doi:10.7150/ijms.55633
Van Cauter E., Leproult R., Kupfer D. J. (1996). Effects of Gender and Age on the Levels and Circadian Rhythmicity of Plasma Cortisol. J. Clin. Endocrinol. Metabolism 81, 2468–2473. doi:10.1210/jcem.81.7.8675562
Vega R. B., Konhilas J. P., Kelly D. P., Leinwand L. A. (2017). Molecular Mechanisms Underlying Cardiac Adaptation to Exercise. Cell Metab. 25, 1012–1026. doi:10.1016/j.cmet.2017.04.025
Verbon E. H., Post J. A., Boonstra J. (2012). The Influence of Reactive Oxygen Species on Cell Cycle Progression in Mammalian Cells. Gene 511, 1–6. doi:10.1016/j.gene.2012.08.038
Verheggen I. C. M., de Jong J. J. A., van Boxtel M. P. J., Postma A. A., Jansen J. F. A., Verhey F. R. J., et al. (2020). Imaging the Role of Blood-Brain Barrier Disruption in Normal Cognitive Ageing. GeroScience 42, 1751–1764. doi:10.1007/s11357-020-00282-1
Vigorito C., Giallauria F. (2014). Effects of Exercise on Cardiovascular Performance in the Elderly. Front. Physiol. 5, 51. doi:10.3389/fphys.2014.00051
Voss M. W., Weng T. B., Narayana-Kumanan K., Cole R. C., Wharff C., Reist L., et al. (2020). Acute Exercise Effects Predict Training Change in Cognition and Connectivity. Med. Sci. Sports Exerc. 52, 131–140. doi:10.1249/MSS.0000000000002115
Wall B. T., Gorissen S. H., Pennings B., Koopman R., Groen B. B. L., Verdijk L. B., et al. (2015). Aging Is Accompanied by a Blunted Muscle Protein Synthetic Response to Protein Ingestion. PloS One 10, e0140903. doi:10.1371/journal.pone.0140903
Wang L., Lavier J., Hua W., Wang Y., Gong L., Wei H., et al. (2021). High-Intensity Interval Training and Moderate-Intensity Continuous Training Attenuate Oxidative Damage and Promote Myokine Response in the Skeletal Muscle of ApoE KO Mice on High-Fat Diet. Antioxidants 10, 992. doi:10.3390/antiox10070992
Wasfy M. M., Baggish A. L. (2016). Exercise Dose in Clinical Practice. Circulation 133, 2297–2313. doi:10.1161/CIRCULATIONAHA.116.018093
Wende A. R., Symons J. D., Abel E. D. (2012). Mechanisms of Lipotoxicity in the Cardiovascular System. Curr. Hypertens. Rep. 14, 517–531. doi:10.1007/s11906-012-0307-2
White A., Salgado R., Schneider S., Loeppky J., Astorino T., Mermier C. (2014). Does Heat Acclimation Improve Exercise Capacity at Altitude? A Cross-Tolerance Model. Int. J. Sports Med. 35, 975–981. doi:10.1055/s-0034-1368724
Wohlwend M., Olsen A., Håberg A. K., Palmer H. S. (2017). Exercise Intensity-dependent Effects on Cognitive Control Function during and after Acute Treadmill Running in Young Healthy Adults. Front. Psychol. 8, 406. doi:10.3389/fpsyg.2017.00406
Wolfe R. R., Klein S., Carraro F., Weber J. M. (1990). Role of Triglyceride-Fatty Acid Cycle in Controlling Fat Metabolism in Humans during and after Exercise. Am. J. Physiology-Endocrinology Metabolism 258, E382–E389. doi:10.1152/ajpendo.1990.258.2.E382
Woo J. S., Derleth C., Stratton J. R., Levy W. C. (2006). The Influence of Age, Gender, and Training on Exercise Efficiency. J. Am. Coll. Cardiol. 47, 1049–1057. doi:10.1016/j.jacc.2005.09.066
Yach D., Hawkes C., Gould C. L., Hofman K. J. (2004). The Global Burden of Chronic Diseases. JAMA 291, 2616–2622. doi:10.1001/jama.291.21.2616
Yildiz M., Oktay A. A., Stewart M. H., Milani R. V., Ventura H. O., Lavie C. J. (2020). Left Ventricular Hypertrophy and Hypertension. Prog. Cardiovasc. Dis. 63, 10–21. doi:10.1016/j.pcad.2019.11.009
Yoo S.-Z., No M.-H., Heo J.-W., Park D.-H., Kang J.-H., Kim J.-H., et al. (2019). Effects of Acute Exercise on Mitochondrial Function, Dynamics, and Mitophagy in Rat Cardiac and Skeletal Muscles. Int. Neurourol. J. 23, S22–S31. doi:10.5213/inj.1938038.019
Zhou T. L., Henry R. M. A., Stehouwer C. D. A., van Sloten T. T., Reesink K. D., Kroon A. A. (2018). Blood Pressure Variability, Arterial Stiffness, and Arterial Remodeling. Hypertension 72, 1002–1010. doi:10.1161/HYPERTENSIONAHA.118.11325
Keywords: preventive medicine, exercise physiology, physiological mechanisms, stress adaptation, aging
Citation: Lefferts WK, Davis MM and Valentine RJ (2022) Exercise as an Aging Mimetic: A New Perspective on the Mechanisms Behind Exercise as Preventive Medicine Against Age-Related Chronic Disease. Front. Physiol. 13:866792. doi: 10.3389/fphys.2022.866792
Received: 31 January 2022; Accepted: 06 June 2022;
Published: 15 August 2022.
Edited by:
Hassane Zouhal, University of Rennes 2, FranceReviewed by:
Lorenzo Iovino, Fred Hutchinson Cancer Research Center, United StatesCopyright © 2022 Lefferts, Davis and Valentine. This is an open-access article distributed under the terms of the Creative Commons Attribution License (CC BY). The use, distribution or reproduction in other forums is permitted, provided the original author(s) and the copyright owner(s) are credited and that the original publication in this journal is cited, in accordance with accepted academic practice. No use, distribution or reproduction is permitted which does not comply with these terms.
*Correspondence: Wesley K. Lefferts, d2xlZmZlcnRAaWFzdGF0ZS5lZHU=