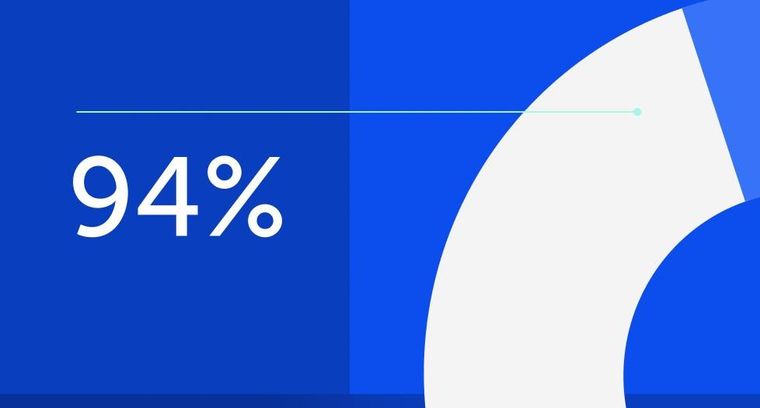
94% of researchers rate our articles as excellent or good
Learn more about the work of our research integrity team to safeguard the quality of each article we publish.
Find out more
MINI REVIEW article
Front. Physiol., 29 April 2022
Sec. Clinical and Translational Physiology
Volume 13 - 2022 | https://doi.org/10.3389/fphys.2022.866617
This article is part of the Research TopicModel Organisms and Experimental Models: Opportunities and Challenges in Clinical and Translational PhysiologyView all 6 articles
The urgency to investigate trauma in a controlled and reproducible environment rises since multiple trauma still account for the most deaths for people under the age of 45. The most common multiple trauma include head as well as blunt thorax trauma along with fractures. However, these trauma remain difficult to treat, partially because the molecular mechanisms that trigger the immediate immune response are not fully elucidated. To illuminate these mechanisms, investigators have used animal models, primarily mice as research subjects. This mini review aims to 1) emphasize the importance of the development of clinically relevant murine trauma research, 2) highlight and discuss the existing conflict between simulating clinically relevant situations and elucidating molecular mechanisms, 3) describe the advantages and disadvantages of established mouse trauma models developed to simulate clinically relevant situations, 4) summarize and list established mouse models in the field of trauma research developed to simulate clinically relevant situations.
Although advances were made in therapeutic treatment of patients suffering multiple trauma, it still remains one of the main causes for death in the population under 45 years (Kung et al., 2008; Norton and Kobusingye 2013). These kinds of injuries can be caused by falls, car accidents, explosions and most frequently result in thorax trauma, extremity fractures, muscle trauma and head injuries (Bardenheuer et al., 2000) affecting functionality of substantial organs like lung, heart, brain, and kidneys but also the hematologic system, the immune system and the endocrine system, potentially leading to multiple organ dysfunction syndrome (MODS) (Marshall, 2001). Regarding the lung, trauma could result in acute respiratory distress syndrome (ARDS), characterized by dyspnea, hypoxemia, cellular infiltrates as well as alveolar degradation (Bakowitz et al., 2012). Cardiovascular dysfunction is characterized by lower cardiac output and stroke volume (Wall et al., 2019), dysrhythmias including ventricular fibrillation, cardiac arrest, and wall motion disorders as well as ongoing hypotension (B. Weber et al., 2021a; B. Weber et al., 2021b). Neurological disorders are described by altered level of consciousness assessed by the Glasgow coma score (Villeneuve et al., 2016) and most likely caused by impaired cerebral blood flow, vasospasm or cerebral edema (Vella et al., 2017; Jha et al., 2019). Furthermore, the kidneys can be affected by trauma resulting in acute kidney injury (AKI) characterized by decreased renal perfusion and glomerular filtration rate (GFR) (Lai et al., 2016; Makris and Spanou 2016). Apart from that, trauma can also affect the hematological system leading to trauma-induced coagulopathy (TIC) specified by hypocoagulation in early-stage and hypercoagulation in later-stage, platelet dysfunction, and dysregulated fibrinolysis (Hayakawa 2017; Peng and Su 2017; Moore et al., 2021). Especially traumatic brain injury is highly associated to dysfunctions concerning the endocrine hormonal system manifesting by adrenal insufficiency, diabetes insipidus or hyponatremia (Bollerslev et al., 2013). Many aspects of the patient care remain heavily debated due to unknown underlying mechanisms with regard to the immediate immune response. This instant response can be described as systemic inflammatory response syndrome (SIRS) and the compensatory anti-inflammatory response syndrome (CARS), where both require attention to avoid secondary consequences of multiple organ failure (Osuchowski et al., 2006; Novotny et al., 2012), persistent inflammation, immunosuppression, and/or protein catabolism syndrome (PICS) (Mira et al., 2018). Even though the basic principles behind the triggered immune response are fairly known, molecular mechanisms after multiple trauma are extremely complex and not fully elucidated yet.
Within the last years, more trauma models including hemorrhagic shock as an additional factor were established as the handling of these patients is still complex and the underlying mechanisms are not fully elucidated yet (Bouglé et al., 2013). Hypovolemic hemorrhagic shock leads to insufficient oxygen delivery and consequently to cellular death. The subsequent release of damage-associated molecular patterns (DAMPs) triggers the innate immune response (Pantalone et al., 2021). In a murine polytrauma model, additional hemorrhagic shock strongly influenced the innate immune response by upregulation of myeloid leukocyte activation and differentiation, upregulation of cytokine secretion of interleukin 6 (IL-6), interleukin 1β (IL-1β) and tumor necrosis factor α (TNF-α), upregulation of genes involved neutrophil chemotaxis and cell adhesion as well as upregulation of toll-like receptor signaling pathway. Apart from that a downregulation of pathways involved in B- and T-cell activation was observed indicating a dysfunction of adaptive immune activation (Debler et al., 2021). Consequently, hemorrhagic shock further promotes uncontrolled innate immune response potentially leading to disbalanced cascade systems such as acute trauma-induced complementopathy or coagulopathy (Huber-Lang and Ward 2018).
The acute response to trauma-hemorrhage involves a complex interplay between the brain and the peripheral visceral organs. Trauma often leads to a disruption of the endocrine brain function including pituitary dysfunction, impaired antidiuretic hormone secretion, adrenal hormone reduction and altered secretion of thyrotropin consequently influencing the catecholamine surge, inflammatory response as well as sympathetic tone (Rachfalska et al., 2020). Apart from that, gut barrier integrity and loss of mucus layer caused by metabolic alterations related to glycolysis, amino acid biosynthesis, pento-phosphate pathway and mitochondrial ATP synthesis seem to be highly involved in the development of MODS after trauma-hemorrhage (Z. Li et al., 2019). The epithelial barrier failure of the gut leads to the systemic release of tissue injury factors via the mesenteric lymph (toxic mesenteric lymph) affecting and damaging multiple organs including spleenic and thymic immune cell apoptosis (Tiesi et al., 2013), shift of Treg to Th17 cell ratio in the mesenteric lymph node (Morishita et al., 2015), induction of cardiac contractile dysfunction (Lee et al., 2008) as well as promoting lung injury (Levy et al., 2012). Furthermore, it was shown that hemorrhagic shock leads to apoptosis of bone-marrow hematopoietic progenitor cells (Kumar et al., 2016). Remarkably, it was shown that stimulation of the vagus nerve, which represents the longest parasympathetic nerve connecting the central nervous system with visceral organs and the immune system, mitigates gut barrier dysfunction and prevents systemic organ damage (Levy et al., 2012; Levy et al., 2013; Morishita et al., 2015).
In this context the call for models to investigate the ongoing mechanisms after trauma increases. This need can be filled by utilizing animal models, which offer options for a reproducible and controllable environment.
However, the choice of a suitable trauma model highly depends on hypothesis and scientific questions and should be carefully revised as later modifications or adaptions regarding the trauma model might be difficult to implement and are often related to high effort or flawed data.
Generally, research has utilized animal models that fall within one of the two categories: clinical relevance or elucidation of molecular mechanisms. Therefore, researchers should clarify whether the aim of the study is on illuminating a molecular mechanism possibly by using genetically modified organisms or on simulating a clinically relevant situation. Both approaches show an interdependency and choosing one approach often goes at the expense of the other one (Figure 1).
FIGURE 1. Advantages (+) and disadvantages (−) of clinically or mechanistically relevant animal trauma models.
Nevertheless, either approach show drawbacks with the focus on translatability of the findings to the human situation. With the main focus in trauma research in mind, which inevitably is on developing animal models that simulate the clinical situation of complex response after trauma as close as possible, this might seem contradicting, since this represents the fundamental requirements for a successful transfer to the human. To overcome these shortcomings a third group of models is necessary, dealing with complex trauma settings, since in this research field relevant mechanisms can only be elucidated when the underlying trauma model was proven to be clinically relevant.
Complex trauma models, for example the combination of multiple trauma with hemorrhagic shock or infection, allow a systemic evaluation of the inflammatory response that is more important for the clinical progress but often lack the possibility to elucidate direct cause-effect relations and detailed molecular mechanisms due to multifactorial design (Figure 1). Although related to high additional effort, one approach to overcome these limitations might be the independent investigation of the single trauma as well as all possible trauma combinations as exemplarily performed by Denk et al. (2015) and Relja et al. (2020). The investigation of monotrauma should ideally be performed within the same study or at least under same conditions as the polytrauma. This strategy will greatly enhance the understanding of the complex trauma models and clarify how monotrauma contribute to the overall outcome. The establishment of models and the design of studies that combine both, clinical relevance, and the possibility to describe molecular mechanisms, should be the focus of further investigations.
Within recent years, this has been in the focus of researchers, who have developed new models and refined existing models by increasing complexity as well as severity of trauma with the aim to improve clinical translatability, which will be discussed in a later chapter (Table 1). This will include various murine animal models, that aim to add additional depth to their trauma model by advanced modifications closer to human conditions. These models try to diminish the negative aspects mentioned in Figure 1 as they are developed based on previous studies that investigated the effect of the respective single trauma (when applying multiple trauma) or they even address this issue in the presented study. Apart from that, the shown studies first describe and elucidate the impact of trauma on molecular, cellular, tissue, and organ level helping to evaluate whether the approach is useful to simulate a human clinical situation, illustrating an important aspect of the “reverse translation” approach introduced by Efron et al. (2015), discussed more detailed in chapter 3.
TABLE 1. Summary of trauma mouse models with a specific focus on clinical relevance by implementing additional aspects to add to translational clinical value.
Various mouse models were defined and established over the last century. One of the first animal models in scientific literature was established by R. L. Noble and J. B. Collip in 1942 focusing on graded levels of trauma and their association with mortality curves, with a focus on complications of hemorrhage, infections as well as anesthesia (Noble and Collip 1942). In the context of this research the Noble-Collip drum was established, which has been used in trauma models since then (Moulton et al., 1962; Li et al., 2017; Hayakawa et al., 2015). Based on this, researchers came up with their own ways to set reproducible trauma in animal models including single as well as a combination of trauma. Some of the most recent mouse models with the aim to mimic the human condition of a traumatic injury are listed in Table 1, including one of our own murine models consisting of a combined blunt trauma (muscle + lung) using 16 ± 1 week old C57BL/6 mice (Gihring et al., 2020). The PubMed® and the Web of ScienceTM databases were searched in January and February 2022 for original mouse research reports published between 01/2018 and 02/2022; focused on monotrauma involving the head, bone, abdomen, and thorax or monotrauma in combination with hemorrhagic shock (polytrauma); involved murine models that represent improvements in clinical relevancy compared to previous model versions; and provided clear methods to enhance reproducibility.
Generally, mice are the animal model of choice to investigate underlying mechanisms in diseases or various immunological settings and are responsible for important breakthroughs in understanding the human immune system. Reasons are cost-effective keeping, easy implementation, ethically acceptance by the public, genetic manipulation in the form of knockout models as well as high gene homology between mice and human (approximately 80%) (Tsukamoto and Pape 2009).
However, it needs to be mentioned that criticism surrounds murine models with regards to translational research. In this context, the “Inflammation and Host Response to Injury” (Xiao et al., 2011; Cuschieri et al., 2012) as well as the Mouse ENCODE Consortium (Yue et al., 2014) listed the response of mice and humans on the transcriptomic level. These datasets have been assessed and evaluated by separate working groups, resulting in differing conclusions with regards to the genomic response to inflammatory settings (Seok et al., 2013; Takao and Miyakawa 2015).
The choice of the mouse strain also seems to be an issue for trauma research as different strains might exhibit different properties, relevant for the trauma response. First, different inbred (e.g., C57BL/6) and outbred (e.g., ICR) strains are available. While inbred strains show a high genetic stability and therefore potentially increase experimental reproducibility, outbred strains are genetically heterogeneous and therefore better simulate the human population (Tuttle et al., 2018; Spenlingwimmer et al., 2019) thereby presenting an important factor when establishing clinically relevant models. Moreover, Tuttle et al. could not find evidence for greater trait stability in inbred mice compared to outbred mice and therefore suggested the use of outbred mice for biomedical research (Tuttle et al., 2018). However, mouse strains not only differ regarding their breeding method but also in physiological properties like heart and skeletal muscle masses (Avila et al. 2017) and their immune response to injury or infection. In a sepsis model, BALB/c mice were shown to respond in a Th2-dominant manner compared to C57BL/6 mice, which respond in a Th-1 dominant manner, probably caused by different innate immune response of macrophages (Watanabe et al., 2004). Furthermore, the airways response to injury in C57BL/6 was comparable to that in outbred strains and humans, which was not the case for BALB/c mice (Busch et al., 2016). Additionally, BALB/c mice showed higher levels of circulating regulatory T-cells and MHC-2-positive lymphocytes compared to CD-1 mice in response to polytrauma, whereas overall immune response was comparable (Spenlingwimmer et al., 2019). With regard to trauma-hemorrhage C57BL/6 mice showed significant differences in splenocyte and bone-marrow functions as well as in release of immune mediators compared to C3H/HeN mice (Matsutani et al., 2005).
Apart from that, one issue often discussed in literature concerns the age of the mice that are used in experiments as it influences the immune system, organ function as well as metabolites (Nikolich-Žugich 2014; Petr et al., 2021). As shown in Table 1, all but one (pediatric study) of the mentioned studies used mice within the age of 8–17 weeks representing the age range most often used for murine models, although the term “adult” seems to be inconsistently defined across studies (Jackson et al., 2017). For C57BL/6J mice, three life phases, namely mature adult (3–6 months corresponds to 20–30 years in human), middle-aged (10–14 months corresponds to 38–47 years in human), and old (18–24 months corresponds to 56–69 years in human) are described (Flurkey et al., 2007). The use of mature adult mice has several biological advantages as they are considered as fully developed but not yet affected by senescence, although one of the main reasons seemed to be reduced costs as well as historical data comparability (Jackson et al., 2017). However, the gold standard for age might not exist and should depend on the scientific question; for example in some mouse strains the peak bone mass is not reached within the age span of 3–6 months (Jilka 2013). Obviously, the age of the human counterpart should always serve as a reference.
Furthermore, it is criticized that one of the advantages of murine trauma models namely the controlled environment is limiting research with regards to underlying inflammatory processes and mechanisms due to the missing multifactorial nature of a traumatic injury. All models listed in Table 1 use mechanical, blunt trauma induction with the intention to create a reproducible, multifactorial nature of trauma, imitating the course of injury (accident, fall, hit, explosion, bone fracture) in a best possible way and overcoming the limitations existing for injuries induced via chemical induction like bleomycin-induced lung injury (Orlando et al., 2019), BaCl2-induced muscle injury (Hardy et al., 2016) or glutamate-induced spinal cord injury (Cheriyan et al., 2014) more relevant for a highly controlled injury induction.
Additionally, no drug or therapeutic reagent that showed success to some degree in murine trauma models was able to show comparable results in clinical trials (Efron et al., 2015; Stortz et al., 2017; Mira et al., 2018). Therefore, an alternative approach described as “reverse translation” (Efron et al., 2015) was proposed and should be taken into account when establishing new trauma models. It requires the verification of the murine model based on observations (specific molecules, phenotypes) made in the clinic and simplifies the final retranslation to the patient. Due to the development of high-resolution methods like next generation sequencing, single-cell RNA-Seq or mass cytometry but also the progress made in imaging techniques the data situation in humans improved within recent years making this approach even more applicable. On the other hand, these methods should be also applied in the respective murine model to confirm and verify its suitability. Although associated with higher effort, costs and need of expertise, we recommend using the “reverse translation” approach for the development of murine models as it might greatly enhance the translatability of murine research to human.
After reviewing various aspects that clearly indicate some disadvantages of murine models for investigating the immune response to multiple trauma, the questions arise if these models should be replaced in trauma research. Even though no clinical success was achieved with mouse models in trauma research, most innovations in human research were based on mouse models, due to the unethically nature of immediate human trials (Mira et al., 2018; Efron et al., 2015; Stortz et al., 2017). Furthermore, mouse models have proven themselves as an important tool in other translational research fields including immunology (Osuchowski et al., 2014). The solution should be to revise existing models instead of abandoning them. Each of the presented trauma models in Table 1 was chosen because it added some modification to an established trauma model to get closer towards the human situation. Either by adding more trauma to resemble the multifactorial side of a human trauma or by adding specific ways to set a trauma to be more consistent with the circumstances of human trauma (brain contusion by explosion modeled through blast wave). Clinical relevance should always be the center point of the murine mouse model. If the main population of patients suffering multiple trauma are adults, there is no need to set up a model with 10-week-old mice (Wang et al., 2020), although several advantages are associated with this age range. If blunt trauma is investigated, chemical induction might not be the best choice due to a different nature of the trauma.
In conclusion, we are of the opinion that the represented models serve as a good starting point for further development of animal models under consideration of the “reverse translational” approach, keeping the focus on the important aspect of clinical relevance and clinical translatability. As this approach requires a profound knowledge about the complex trauma model, the investigation of the respective monotrauma should always be included although, at first sight, increasing effort and animal numbers. Nevertheless, a well investigated and described model proven to be reliable and clinically relevant enhances reproducibility and has the potential to become a standard model in this research field simultaneously compensating effort and animal numbers.
When developing models with the intention to describe a molecular mechanism, the underlying trauma model should be proven to be clinically relevant as described before. Within their work, researchers should clearly state whether and how the used model addresses a clinically relevant situation, intends to elucidate a molecular mechanism, or even tries to combine both aspects. However, the downsides of using murine models in such a complex field of research, as represented within this review, should always be borne in mind. Apart from focusing on clinical relevance and clinical translatability, further improvement of existing models should also consider the importance of social determinants of health as they are relevant for the outcome and mortality after trauma (Phelos et al., 2022), but are often overlooked within current studies.
All authors have been involved in writing and editing the manuscript. Figures have been done by FG and AG. All authors contributed to the article and approved the submitted version.
MW and UK were supported by the Deutsche Forschungsgemeinschaft (DFG) as part of the SFB1149 “Danger Response, Disturbance Factors and Regenerative Potential after Acute Trauma” (251293561, project B04). MS holds a GEROK position funded by the DFG (SFB1149) and AG participates in the International PhD Programme of the International Graduate School in Molecular Medicine Ulm (GSC270).
The authors declare that the research was conducted in the absence of any commercial or financial relationships that could be construed as a potential conflict of interest.
All claims expressed in this article are solely those of the authors and do not necessarily represent those of their affiliated organizations, or those of the publisher, the editors and the reviewers. Any product that may be evaluated in this article, or claim that may be made by its manufacturer, is not guaranteed or endorsed by the publisher.
Anandasivam N. S., Russo G. S. B. A., Samuel A. M., Ondeck N. T., Chung S. H., et al. (2017). Tibial Shaft Fracture: A Large-Scale Study Defining the Injured Population and Associated Injuries. J. Clin. Orthopaedics Trauma 8 (3), 225–231. doi:10.1016/j.jcot.2017.07.012
Avila J. J., Kim S. K., Massett M. P. (2017). Differences in Exercise Capacity and Responses to Training in 24 Inbred Mouse Strains. Front. Physiol. 8, 974. doi:10.3389/fphys.2017.00974
Bakowitz M., Bruns B., McCunn M. (2012). Acute Lung Injury and the Acute Respiratory Distress Syndrome in the Injured Patient. Scand. J. Trauma Resusc. Emerg. Med. 20, 54. doi:10.1186/1757-7241-20-54
Bardenheuer M., Obertacke U., Obertacke U., Waydhas C., Nast-Kolb D. (2000). Epidemiologie des Schwerverletzten Eine prospektive Erfassung der präklinischen und klinischen Versorgung. Unfallchirurg 103 (5), 355–363. doi:10.1007/s001130050550
Bernick C., Banks S. J., Shin W., Obuchowski N., Butler S., Noback M., et al. (2015). Repeated Head Trauma Is Associated with Smaller Thalamic Volumes and Slower Processing Speed: The Professional Fighters' Brain Health Study. Br. J. Sports Med. 49 (15), 1007–1011. doi:10.1136/bjsports-2014-093877
Bhoi S., Kumar M., Mohanty S., Kamal V., Rao D., Mishra P., et al. (2016). Bone Marrow Hematopoietic Stem Cells Behavior with or without Growth Factors in Trauma Hemorrhagic Shock. Int. J. Crit. Illn Inj. Sci. 6 (3), 119–126. doi:10.4103/2229-5151.190654
Bollerslev J., Klibanski A., Tritos N. (2013). Traumatic Brain Injury: Effects on the Endocrine System. J. Clin. Endocrinol. Metab. 98 (3), 27A–28A. doi:10.1210/jcem.98.3.zeg27a
Bouglé A., Harrois A., Duranteau J. (2013). Resuscitative Strategies in Traumatic Hemorrhagic Shock. Ann. Intensive Care 3 (1), 1. doi:10.1186/2110-5820-3-1
Busch R. A., Jonker M. A., Pierre J. F., Heneghan A. F., Kudsk K. A. (2016). Innate Mucosal Immune System Response of BALB/c vs C57BL/6 Mice to Injury in the Setting of Enteral and Parenteral Feeding. JPEN J. Parenter. Enteral Nutr. 40 (2), 256–263. doi:10.1177/0148607114558489
Chen K., Gu H., Zhu L., Feng D.-F. (2019). A New Model of Repetitive Traumatic Brain Injury in Mice. Front. Neurosci. 13, 1417. doi:10.3389/fnins.2019.01417
Cheriyan T., Ryan D. J., Weinreb J. H., Cheriyan J., Paul J. C., Lafage V., et al. (2014). Spinal Cord Injury Models: A Review. Spinal Cord 52 (8), 588–595. doi:10.1038/sc.2014.91
Chrysou K., Halat G., Hoksch B., Schmid R. A., Kocher G. J. (2017). Lessons from a Large Trauma Center: Impact of Blunt Chest Trauma in Polytrauma Patients-Still a Relevant Problem? Scand. J. Trauma Resusc. Emerg. Med. 25 (1), 42. doi:10.1186/s13049-017-0384-y
Clark A. L., Merritt V. C., BiglerBangen E. D., Bangen K. J., Sorg S. F., Bondi M. W., et al. (2018). Blast-Exposed Veterans with Mild Traumatic Brain Injury Show Greater Frontal Cortical Thinning and Poorer Executive Functioning. Front. Neurol. 9, 873. doi:10.3389/fneur.2018.00873
Corradi F., Brusasco C., Vezzani A., Palermo S., Altomonte F., Moscatelli P., et al. (2011). Hemorrhagic Shock in Polytrauma Patients: Early Detection with Renal Doppler Resistive Index Measurements. Radiology 260 (1), 112–118. doi:10.1148/radiol.11102338
Cuschieri J., Johnson J. L., Sperry J., West M. A., Moore E., Bankey J. P. P. E., et al. (2012). Benchmarking Outcomes in the Critically Injured Trauma Patient and the Effect of Implementing Standard Operating Procedures. Ann. Surg. 255 (5), 993–999. doi:10.1097/SLA.0b013e31824f1ebc
Debler L., Palmer A., Braumüller S., Kloh B., Mollnes T. E., Holzmann K., et al. (2021). Hemorrhagic Shock Induces a Rapid Transcriptomic Shift of the Immune Balance in Leukocytes after Experimental Multiple Injury. Mediators Inflamm. 2021, 1–9. doi:10.1155/2021/6654318
Denk S., Weckbach S., Eisele P., Braun C. K., Wiegner R., Ohmann J. J., et al. (2018). Role of Hemorrhagic Shock in Experimental Polytrauma. Shock (Augusta, Ga.) 49 (2), 154–163. doi:10.1097/SHK.0000000000000925
Denk S., Wiegner R., Hönes F. M., Messerer D. A. C., Radermacher P., Weiss M., et al. (2015). Early Detection of Junctional Adhesion Molecule-1 (JAM-1) in the Circulation After Experimental and Clinical Polytrauma. Mediators Inflamm. 2015, 1–7. doi:10.1155/2015/463950
Efron P. A., Mohr A. M., Moore F. A., Moldawer L. L. (2015). The Future of Murine Sepsis and Trauma Research Models. J. Leukoc. Biol. 98 (6), 945–952. doi:10.1189/jlb.5MR0315-127R
Flurkey K., Mcurrer J., Harrison D. (2007). Mouse Models in Aging Research. Mouse Biomed. Res. III, 637–672. doi:10.1016/b978-012369454-6/50074-1
Gihring A., Gärtner F., Liu C., Hoenicka M., Wabitsch M., Knippschild U., et al. (2020). Influence of Obesity on the Organization of the Extracellular Matrix and Satellite Cell Functions after Combined Muscle and Thorax Trauma in C57BL/6J Mice. Front. Physiol. 11, 849. doi:10.3389/fphys.2020.00849
Hardy D., Besnard A., Latil M., Jouvion G., Briand D., Thépenier C., et al. (2016). Comparative Study of Injury Models for Studying Muscle Regeneration in Mice. PloS One 11 (1), e0147198. doi:10.1371/journal.pone.0147198
Hayakawa M., Gando S., Ono Y., Wada T., Yanagida Y., Sawamura A., et al. (2015). Noble-Collip Drum Trauma Induces Disseminated Intravascular Coagulation but Not Acute Coagulopathy of Trauma-Shock. Shock (Augusta, Ga.) 43 (3), 261–267. doi:10.1097/SHK.0000000000000281
Hayakawa M. (2017). Pathophysiology of Trauma-Induced Coagulopathy: Disseminated Intravascular Coagulation with the Fibrinolytic Phenotype. J. Intensive Care 5, 14. doi:10.1186/s40560-016-0200-1
Huber-Lang M., Lambris J. D., Ward P. A. (2018). Innate Immune Responses to Trauma. Nat. Immunol. 19 (4), 327–341. doi:10.1038/s41590-018-0064-8
Jackson S. J., Andrews N., Ball D., Bellantuono I., Gray J., Hachoumi L., et al. (2017). Does Age Matter? The Impact of Rodent Age on Study Outcomes. Lab. Anim. 51 (2), 160–169. doi:10.1177/0023677216653984
Jha R. M., Kochanek P. M., Simard J. M. (2019). Pathophysiology and Treatment of Cerebral Edema in Traumatic Brain Injury. Neuropharmacology 145 (Pt B), 230–246. doi:10.1016/j.neuropharm.2018.08.004
Jilka R. L. (2013). The Relevance of Mouse Models for Investigating Age-Related Bone Loss in Humans. Journals Gerontol. Ser. A: Biol. Sci. Med. Sci. 68 (10), 1209–1217. doi:10.1093/gerona/glt046
Kung H. C., Hoyert D. L., Xu J., Murphy S. L. (2008). Deaths: Final Data for 2005. Natl. Vital Stat. Rep. 56 (10), 1.
Lai W.-H., Rau C.-S., Wu S.-C., Chen Y.-C., Kuo P.-J., Hsu S.-Y., et al. (2016). Post-traumatic Acute Kidney Injury: A Cross-Sectional Study of Trauma Patients. Scand. J. Trauma Resusc. Emerg. Med. 24 (1), 136. doi:10.1186/s13049-016-0330-4
Lee M. A., YataniSambol A., Sambol J. T., Deitch E. A. (2008). Role of Gut-Lymph Factors in the Induction of Burn-Induced and Trauma-Shock-Induced Acute Heart Failure. Int. J. Clin. Exp. Med. 1 (2), 171.
Levy G., Fishman J. E., Xu D.-z., Dong W., Palange D., Vida G., et al. (2012). Vagal Nerve Stimulation Modulates Gut Injury and Lung Permeability in Trauma-Hemorrhagic Shock. J. Trauma Acute Care Surg. 73 (2), 338–342. doi:10.1097/TA.0b013e31825debd3
Levy G., Fishman J. E., Xu D., Chandler B. T. J., Feketova E., Dong W., et al. (2013). Parasympathetic Stimulation via the Vagus Nerve Prevents Systemic Organ Dysfunction by Abrogating Gut Injury and Lymph Toxicity in Trauma and Hemorrhagic Shock. Shock (Augusta, Ga.) 39 (1), 39–44. doi:10.1097/SHK.0b013e31827b450d
Li X., Cao T., Ma S., Jing Z., Bi Y., Zhou J., et al. (2017). Curcumin Ameliorates Cardiac Dysfunction Induced by Mechanical Trauma. Eur. J. Pharmacol. 814, 73–80. doi:10.1016/j.ejphar.2017.07.048
Li Z., Li J., Zhang S., Chen G., Chi S., Li X., et al. (2019). Metabolomics Analysis of Gut Barrier Dysfunction in a Trauma-Hemorrhagic Shock Rat Model. Biosci. Rep. 39 (1). doi:10.1042/BSR20181215
Liang T., Ma Y.-F., Zhu J., Wang D.-X., Liu Y. (2016). A Clinical Study of Multiple Trauma Combined with Acute Lung Injury. J. Acute Dis. 5 (6), 450–453. doi:10.1016/j.joad.2016.07.001
Maitz A., Haussner F., Braumüller S., Hoffmann A., Lupu L., Wachter U., et al. (2021). Temporal-Spatial Organ Response After Blast‐Induced Experimental Blunt Abdominal Trauma. FASEB J. 35 (12), e22038. doi:10.1096/fj.202100995R
Makris K., Spanou L. (2016). Acute Kidney Injury: Definition, Pathophysiology and Clinical Phenotypes. Clin. Biochem. Rev. 37 (2), 85.
Matsutani T., Ananthasamy T., Kang S., Bland K., Chaudry I. (2005). Mouse Genetic Background Influences Severity of Immune Responses Following Trauma-Hemorrhage. Cytokine 30 (4), 168–176. doi:10.1016/j.cyto.2004.12.019
Mira J. C., Nacionales D. C., Loftus T. J., Ungaro R., Mathias B., Mohr A. M., et al. (2018). Mouse Injury Model of Polytrauma and Shock. Methods Mol. Biol. (Clifton, N.J.), 1–15. doi:10.1007/978-1-4939-7526-6_1
Moore E. E., Moore H. B. Lucy. Z., Hoffman M., Mutch N. J., Schöchl H., Hunt B. J., et al. (2021). Trauma-Induced Coagulopathy. Nat. Rev. Dis. Primers 7 (1), 30. doi:10.1038/s41572-021-00264-3
Morishita K., Coimbra R., Langness S., Eliceiri B. P., Costantini T. W. (2015). Neuroenteric Axis Modulates the Balance of Regulatory T Cells and T-Helper 17 Cells in the Mesenteric Lymph Node Following Trauma/Hemorrhagic Shock. Am. J. Physiology-Gastrointestinal Liver Physiol. 309 (3), G202–G208. doi:10.1152/ajpgi.00097.2015
Moulton G. A., Esmond W. G., Michaelis M. (1962). Effect of Hyperbaric Oxygenation on Noble Collip Drum Shock in the Rat, 47. Baltimore, Md: Bulletin of the School of Medicine, 42.
Nikolich-Žugich J. (2014). Aging of the T Cell Compartment in Mice and Humans: From No Naive Expectations to Foggy Memories. J. Immunol. 193, 2622–2629. doi:10.4049/jimmunol.14011746
Noble R. L., Collip J. B. (1942). A Quantitative Method for the Production of Experimental Traumatic Shock without Haemorrhage in Unanaesthetized Animals. Exp. Physiol. 31 (3), 187–199. doi:10.1113/expphysiol.1942.sp000856
Norton R., Kobusingye O. (2013). Injuries. N. Engl. J. Med. 368 (18), 1723–1730. doi:10.1056/NEJMra1109343
Novotny A. R., Reim D., Assfalg V., Altmayr F., Friess H. M., Emmanuel K., et al. (2012). Mixed Antagonist Response and Sepsis Severity-Dependent Dysbalance of Pro- and Anti-Inflammatory Responses at the Onset of Postoperative Sepsis. Immunobiology 217 (6), 616–621. doi:10.1016/j.imbio.2011.10.019
Ntundu S. H., Herman A. M., Kishe A., Babu H., Jahanpour O. F., Msuya D., et al. (2019). Patterns and Outcomes of Patients with Abdominal Trauma on Operative Management from Northern Tanzania: A Prospective Single Centre Observational Study. BMC Surg. 19 (1), 69. doi:10.1186/s12893-019-0530-8
Orlando F., Paolini C., Agarbati S., Tonnini C., Grieco A., Capelli C., et al. (2019). Induction of Mouse Lung Injury by Endotracheal Injection of Bleomycin. JoVE 146, 58922. doi:10.3791/58922
Osuchowski M. F., Remick D. G., Lederer J. A., Aasen A. O., Azevedo M. L. C., Bahrami S., et al. (2014). Abandon the Mouse Research Ship? Not Just Yet! Shock (Augusta, Ga.) 41 (6), 463–475. doi:10.1097/SHK.0000000000000153
Osuchowski M. F., Welch K., Siddiqui J., Remick D. G. (2006). Circulating Cytokine/Inhibitor Profiles Reshape the Understanding of the SIRS/CARS Continuum in Sepsis and Predict Mortality. J. Immunol. 177, 1967–1974. doi:10.4049/jimmunol.177.3.1967
Pantalone D., Bergamini C., Martellucci J., Alemanno G., Bruscino A., Maltinti G., et al. (2021). The Role of DAMPS in Burns and Hemorrhagic Shock Immune Response: Pathophysiology and Clinical Issues. Review. Ijms 22 (13), 7020. doi:10.3390/ijms22137020
Peng N., Su L. (2017). Progresses in Understanding Trauma-Induced Coagulopathy and the Underlying Mechanism. Chin. J. Traumatol. 20 (3), 133–136. doi:10.1016/j.cjtee.2017.03.002
Petr M. A., Alfaras I., Krawcyzk M., Bair W.-N., MitchellMorrell S. J., Studenski C. H. S. A., et al. (2021). A Cross-Sectional Study of Functional and Metabolic Changes during Aging Through the Lifespan in Male Mice. eLife 10. doi:10.7554/eLife.62952
Phelos H. M., Kass N. M., Deeb A.-P., Brown J. B. (2022). Social Determinants of Health and Patient-Level Mortality Prediction After Trauma. J. Trauma Acute Care Surg. 92 (2), 287–295. doi:10.1097/TA.0000000000003454
Rachfalska N., Putowski Z., Krzych Ł. (2020). Distant Organ Damage in Acute Brain Injury. Brain Sci. 10 (12), 1019. doi:10.3390/brainsci10121019
Ratliff W. A., Mervis R. F., Citron B. A., Schwartz B., Rubovitch V., Schreiber S., et al. (2020). Effect of Mild Blast-Induced TBI on Dendritic Architecture of the Cortex and Hippocampus in the Mouse. Sci. Rep. 10 (1), 2206. doi:10.1038/s41598-020-59252-4
Relja B., Yang B., Bundkirchen K., Xu B., Köhler K., Neunaber C. (2020). Different Experimental Multiple Trauma Models Induce Comparable Inflammation and Organ Injury. Sci. Rep. 10 (1), 20185. doi:10.1038/s41598-020-76499-z
Seok J., Warren H. S., Cuenca A. G., Mindrinos M. N., Baker H. V., Xu W., et al. (2013). Genomic Responses in Mouse Models Poorly Mimic Human Inflammatory Diseases. Proc. Natl. Acad. Sci. U S A. 110 (9), 3507–3512. doi:10.1073/pnas.1222878110
Spenlingwimmer T., Zipperle J., Jafarmadar M., Osuchowski M. F., Drechsler S. (2019). Comparison of Post-Traumatic Changes in Circulating and Bone Marrow Leukocytes Between BALB/c and CD-1 Mouse Strains. PloS One 14 (9), e0222594. doi:10.1371/journal.pone.0222594
Stortz J. A., Raymond S. L., MiraMohr J. C., Moldawer L. L., Mohr A. M., Efron P. A. (2017). Murine Models of Sepsis and Trauma: Can We Bridge the Gap? ILAR J. 58 (1), 90–105. doi:10.1093/ilar/ilx007
Takao K., Miyakawa T. (2015). Genomic Responses in Mouse Models Greatly Mimic Human Inflammatory Diseases. Proc. Natl. Acad. Sci. U.S.A. 112 (4), 1167–1172. doi:10.1073/pnas.1401965111
Tawfik V. L., Quarta M., Paine P., Forman T. E., Pajarinen J., Takemura Y., et al. (2020). Angiotensin Receptor Blockade Mimics the Effect of Exercise on Recovery After Orthopaedic Trauma by Decreasing Pain and Improving Muscle Regeneration. J. Physiol. 598 (2), 317–329. doi:10.1113/JP278991
Tiesi G., Reino D., Mason L., Palange D., Tomaio J. N., Deitch E. A. (2013). Early Trauma-Hemorrhage-Induced Splenic and Thymic Apoptosis Is Gut-Mediated and Toll-Like Receptor 4-Dependent. Shock (Augusta, Ga.) 39 (6), 507–513. doi:10.1097/SHK.0b013e318293d020
Tsukamoto T., Pape H. C. (2009). Animal Models for Trauma Research. Shock (Augusta, Ga.) 31 (1), 3–10. doi:10.1097/SHK.0b013e31817fdabf
Tuttle A. H., PhilipPhilip V. M., Chesler E. J., Mogil J. S. (2018). Comparing Phenotypic Variation Between Inbred and Outbred Mice. Nat. Methods 15 (12), 994–996. doi:10.1038/s41592-018-0224-7
Vella M. A., Crandall M. L., Patel M. B. (2017). Acute Management of Traumatic Brain Injury. Surg. Clin. North America 97 (5), 1015–1030. doi:10.1016/j.suc.2017.06.003
Villeneuve A., Joyal J.-S., Proulx F., Ducruet T., Poitras N., Lacroix J. (2016). Multiple Organ Dysfunction Syndrome in Critically Ill Children: Clinical Value of Two Lists of Diagnostic Criteria. Ann. Intensive Care 6 (1), 40. doi:10.1186/s13613-016-0144-6
Wall J., Naganathar S., Praditsuktavorn B., Bugg O. F., McArthur S., Thiemermann C., et al. (2019). Modeling Cardiac Dysfunction Following Traumatic Hemorrhage Injury: Impact on Myocardial Integrity. Front. Immunol. 10, 2774. doi:10.3389/fimmu.2019.02774
Wang S., Lai X., Deng Y., Song Y. (2020). Correlation Between Mouse Age and Human Age in Anti-Tumor Research: Significance and Method Establishment. Life Sci. 242, 117242. doi:10.1016/j.lfs.2019.117242
Watanabe H., Numata K., Ito T., Takagi K., Matsukawa A. (2004). Innate Immune Response in Th1- and Th2-Dominant Mouse Strains. Shock 22 (5), 460–466. doi:10.1097/01.shk.0000142249.08135.e9
Weber B., Lackner I., Baur M., Gebhard F., Pfeifer R., Cinelli P., et al. (2021a). Early Myocardial Damage (EMD) and Valvular Insufficiency Result in Impaired Cardiac Function After Multiple Trauma in Pigs. Sci. Rep. 11 (1), 1151. doi:10.1038/s41598-020-80409-8
Weber B., Lackner I., Gebhard F., Miclau T., Kalbitz M. (2021b). Trauma, a Matter of the Heart-Molecular Mechanism of Post-Traumatic Cardiac Dysfunction. Int. J. Mol. Sci. 22 (2), 737. doi:10.3390/ijms22020737
Weber C. D., Lefering R., Dienstknecht T., Kobbe P., Sellei R. M., Hildebrand F., et al. (2016). Classification of Soft-Tissue Injuries in Open Femur Fractures. J. Trauma Acute Care Surg. 81 (5), 824–833. doi:10.1097/TA.0000000000001216
Xiao W., Mindrinos M. N., Seok J., Cuschieri J., Cuenca A. G., Gao H., et al. (2011). A Genomic Storm in Critically Injured Humans. J. Exp. Med. 208 (13), 2581–2590. doi:10.1084/jem.20111354
Keywords: trauma, mouse model, clinical relevance, immune response, translatability
Citation: Gihring A, Gärtner F, Schirmer M, Wabitsch M and Knippschild U (2022) Recent Developments in Mouse Trauma Research Models: A Mini-Review. Front. Physiol. 13:866617. doi: 10.3389/fphys.2022.866617
Received: 31 January 2022; Accepted: 04 April 2022;
Published: 29 April 2022.
Edited by:
Meijing Wang, Indiana University Bloomington, United StatesReviewed by:
Barbara St. Pierre Schneider, University of Texas at Arlington, United StatesCopyright © 2022 Gihring, Gärtner, Schirmer, Wabitsch and Knippschild. This is an open-access article distributed under the terms of the Creative Commons Attribution License (CC BY). The use, distribution or reproduction in other forums is permitted, provided the original author(s) and the copyright owner(s) are credited and that the original publication in this journal is cited, in accordance with accepted academic practice. No use, distribution or reproduction is permitted which does not comply with these terms.
*Correspondence: Uwe Knippschild, dXdlLmtuaXBwc2NoaWxkQHVuaWtsaW5pay11bG0uZGU=
Disclaimer: All claims expressed in this article are solely those of the authors and do not necessarily represent those of their affiliated organizations, or those of the publisher, the editors and the reviewers. Any product that may be evaluated in this article or claim that may be made by its manufacturer is not guaranteed or endorsed by the publisher.
Research integrity at Frontiers
Learn more about the work of our research integrity team to safeguard the quality of each article we publish.