- 1Biomedical Engineering Program, University of North Dakota, Grand Forks, ND, United States
- 2Parkinson Disease Research Laboratory, Department of Neurology, Sanford Health, Fargo, ND, United States
- 3Department of Biomedical Physiology and Kinesiology, Simon Fraser University, Burnaby, BC, Canada
- 4School of Medicine and Health Sciences, Department of Neurology, University of North Dakota, Grand Forks, ND, United States
Cardiac baroreflex and leg muscles activation are two important mechanisms for blood pressure regulation, failure of which could result in syncope and falls. Parkinson’s disease is known to be associated with cardiac baroreflex impairment and skeletal muscle dysfunction contributing to falls. However, the mechanical effect of leg muscles contractions on blood pressure (muscle-pump) and the baroreflex-like responses of leg muscles to blood pressure changes is yet to be comprehensively investigated. In this study, we examined the involvement of the cardiac baroreflex and this hypothesized reflex muscle-pump function (cardio-postural coupling) to maintain blood pressure in Parkinson’s patients and healthy controls during an orthostatic challenge induced via a head-up tilt test. We also studied the mechanical effect of the heart and leg muscles contractions on blood pressure. We recorded electrocardiogram, blood pressure and electromyogram from 21 patients with Parkinson’s disease and 18 age-matched healthy controls during supine, head-up tilt at 70°, and standing positions with eyes open. The interaction and bidirectional causalities between the cardiovascular and musculoskeletal signals were studied using wavelet transform coherence and convergent cross mapping techniques, respectively. Parkinson’s patients displayed an impaired cardiac baroreflex and a reduced mechanical effect of the heart on blood pressure during supine, tilt and standing positions. However, the effectiveness of the cardiac baroreflex decreased in both Parkinson’s patients and healthy controls during standing as compared to supine. In addition, Parkinson’s patients demonstrated cardio-postural coupling impairment along with a mechanical muscle pump dysfunction which both could lead to dizziness and falls. Moreover, the cardiac baroreflex had a limited effect on blood pressure during standing while lower limb muscles continued to contract and maintain blood pressure via the muscle-pump mechanism. The study findings highlighted altered bidirectional coupling between heart rate and blood pressure, as well as between muscle activity and blood pressure in Parkinson’s disease. The outcomes of this study could assist in the development of appropriate physical exercise programs to reduce falls in Parkinson’s disease by monitoring the cardiac baroreflex and cardio-postural coupling effect on maintaining blood pressure.
Introduction
Parkinson’s disease (PD) is a neurodegenerative disorder characterized by tremors, bradykinesia, rigidity, and postural instability (Mazzoni et al., 2012). When transitioning from supine to stand or sitting to stand, a transient decrease in blood pressure occurs due to the sudden change in the hydrostatic pressure gradient between the feet and the heart. In healthy persons, homeostatic mechanisms quickly compensate. However, people with Parkinson’s disease may experience impaired reflexes and the drop in blood pressure persists (orthostatic hypotension, OH) (Fanciulli et al., 2020), leading to postural instability, visual disturbances, and loss of consciousness (Mar and Raj 2018), all of which contribute to falls in Parkinson’s disease (Allen et al., 2013). Falls can lead to injury, decreased activity due to fear of falling, poor quality of life, and caregiver stress (LeWitt et al., 2019). Studies have shown that OH can happen in the early stages of the disease and may occur with or without symptoms (Palma et al., 2015; Cutsforth-Gregory and Low 2019; Yoo et al., 2021). In this study, we investigated the involvement of the cardiac baroreflex and cardio-postural coupling (hypothesized reflex muscle-pump function) in maintaining blood pressure in Parkinson’s patients and healthy controls during an orthostatic challenge induced via a head-up tilt test. We also studied the mechanical effect of the heart and leg muscles contractions on blood pressure.
Preventing falls through maintenance of blood pressure and postural stability during standing depends on a series of reflex mechanisms, including the cardiac baroreflex, which is initiated by the autonomic nervous system via efferent neural pathways causing an elevation in heart rate, systemic vascular resistance, and cardiac contractility (Armstrong et al., 2021). Parkinson’s disease can result in damage to the nerve endings that secrete norepinephrine, the main neurotransmitter of the postganglionic sympathetic neurons (Ziemssen and Reichmann 2010; Espay et al., 2014), making blood pressure regulation challenging while standing. Norepinephrine deficiency resulting from sympathetic nerve degeneration contributes to reduced cardiac contractility and the inability to increase vascular resistance via sympathetic outflow (Foulon and De Backer 2018). In addition, previous research suggested that patients with PD have cardiac parasympathetic impairment along with sympathetic denervation (Awerbuch and Sandyk 1992; Shibata et al., 2009; Kim et al., 2014). Parkinson’s disease has been associated with autonomic dysfunction (Visser et al., 2004; Goldstein 2006; Velseboer et al., 2011; Goldstein 2014), and cardiovascular autonomic control deficiencies have been suggested as possible underlying reasons for the drop in blood pressure upon standing (Barbic et al., 2007). PD affects not only the autonomic control of blood pressure but also skeletal muscle function (Inkster et al., 2003; Lavin et al., 2020). During standing, skeletal muscles contract and compress the underlying veins, which leads to the pumping of venous blood pooled in the lower limbs back to the heart (skeletal muscle pump), increasing venous return and blood pressure (Verma et al., 2017; Verma et al., 2019). Xu et al. (2017, 2020), and Verma et al. (2019) found that leg muscles were activated in response to variations in blood pressure, thus preventing blood pooling, and maintaining blood pressure. They referred to this mechanism as the “muscle-pump baroreflex” (Xu et al., 2017; Verma et al., 2019; Xu et al., 2020). Therefore, blood pressure regulation during standing involves feedback from cardiovascular, and musculoskeletal systems. Due to the involvement of multiple physiological systems in the regulation of blood pressure, assessment of blood pressure exclusively through cardiovascular control may be inadequate. In addition to cardiovascular control, measurements of the musculoskeletal system can provide supplemental information on an individual’s ability to maintain blood pressure during an orthostatic challenge.
Previous research has been limited to independently studying cardiovascular or postural control of blood pressure in Parkinson’s disease (Pérez et al., 2015; Miyasato et al., 2018; Kamieniarz et al., 2021). However, evidence of the interplay between these control systems in young and older adults suggests that they should not be studied in isolation (Verma et al., 2017; Verma et al., 2019). Our previous work assessed the physiological interactions between the cardiovascular, postural, and musculoskeletal systems in young and older adults (Blaber et al., 2009; Verma et al., 2017; Xu et al., 2017; Verma et al., 2019). However, the relationship between these systems for blood pressure regulation and postural control in PD patients has yet to be comprehensively investigated. In this regard, our work strives to fill in the gaps of previous Parkinson’s research by monitoring cardiac and muscle pump baroreflexes and their role in blood pressure regulation and postural control in Parkinson’s disease. The outcomes of this work will provide insights in designing appropriate physical exercise programs that can increase the ability of leg muscles to generate force and improve muscle strength to maintain blood pressure and postural stability during standing, hence reducing unexpected falls and associated injuries.
Blood pressure, heart rate, and muscle activity variations are generated by complex control systems. It is consequently important to understand the behavior of these systems, such as trends, periodicities, as well as the coherence between the representative signals of such systems. To characterize these systems and assess their dynamic change, methods based on Fourier analysis have been developed. However, these methods are often based on the assumption that changes in cardiovascular and postural hemodynamics are stationary by assuming the statistical properties of these systems do not change with time (Tian et al., 2016). Hence, more appropriate analysis methods are required to characterize the natural non-stationary aspects of the cardiovascular and postural control systems. Continuous wavelet transform (CWT) has been used to analyze physiological signals in time-frequency space (Garg et al., 2013; Tian et al., 2016; de Boer and Karemaker 2019; Verma et al., 2019). While CWT is a common tool for analyzing localized oscillations in a time series, wavelet transform coherence (WTC) can be used to study the existence and strength of the coherence between two time series in the time-frequency domain.
This work aimed to investigate the involvement of the cardiac baroreflex and a hypothesized reflex muscle-pump function (cardio-postural coupling) in maintaining blood pressure in Parkinson’s patients and healthy controls during an orthostatic challenge. The mechanical effect of the heart and leg muscles contractions on blood pressure was also examined. We recorded EMG activity from the medial and lateral gastrocnemius (MG, LG), tibialis anterior (TA), and soleus (SOL) muscles. Wavelet transform coherence (WTC) was utilized to study the interdependency between the respective signals of the cardiovascular and musculoskeletal systems (Xu et al., 2017; Verma et al., 2019). Convergent cross-mapping (CCM) was used to calculate the degree and the direction of information flow (causality) between the representative signals of cardiovascular and musculoskeletal systems (Verma et al., 2017; Verma et al., 2019; Xu et al., 2020).
We hypothesized that: 1) The cardio-postural coupling is impaired in Parkinson’s disease along with inefficient lower limb muscles contractions to maintain blood pressure. 2) The cardiac baroreflex effect on blood pressure control is reduced during standing as leg muscles contractions also contribute to increased cardiac output and blood pressure.
The study’s findings are expected to reveal the effect of Parkinson’s disease on cardiac baroreflex and cardio-postural coupling, both of which are important mechanisms to maintain blood pressure and ensure a stable upright stance.
Materials and Methods
Experimental Protocol
We recruited PD patients from the Movement Disorders Clinic at Sanford Health in Fargo, ND. All PD patients met at least two of the four diagnostic criteria for PD: bradykinesia, tremor, rigidity, and postural instability, and were DOPA responders. We also recruited healthy controls from the community. PD patients and healthy controls were excluded if they had severe neurological or medical conditions, such as multiple sclerosis, stroke, epilepsy, COPD, congestive heart failure, or renal failure. Participants were also excluded if they had any implanted devices (e.g., deep brain stimulation, pacemaker). We excluded PD patients if they had a Montreal Cognitive Assessment [MOCA (Nasreddine et al., 2005)] score <21. Healthy controls were excluded if they met the criteria for mild cognitive impairment (MOCA <26). The IRB at Sanford Health and the University of North Dakota approved the protocol (IRB #1445). We obtained written informed consent from all participants. The study was conducted following the declaration of Helsinki.
All PD patients were administered the Movement Disorder Society–Unified Parkinson’s Disease Rating Scale (MDS–UPDRS) Part III (Goetz et al., 2007) to assess motor symptom severity. Participants maintained their regular schedule for PD medications. We calculated the levodopa equivalent daily dosage (LEDD) using the conversion formula proposed by (Tomlinson et al., 2010) (e.g., Sinemet: 1 *600 mg L-dopa = 600 LEDD; Selegiline 10 *10 mg = 100 LEDD). To evaluate general autonomic dysfunction, we administered the Scales for Outcomes in Parkinson’s disease–Autonomic Dysfunction [SCOPA–AUT (Visser et al., 2004)]. We measured symptom severity related to low blood pressure using the first 6-items of the Orthostatic Hypotension Questionnaire [OHQ (Kaufmann et al., 2012)]. To evaluate fatigue symptoms, we administered the Multidimensional Fatigue Inventory [MFI (Smets et al., 1995)]. The sample consisted of 24 individuals diagnosed with idiopathic PD by a neurologist and 22 healthy controls. Three PD patients were excluded, one due to a low MOCA score and the others due to noisy data. Four healthy controls were excluded due to a low MOCA score.
The protocol required participants to lie supine on a tilt table for 5 min of baseline recording. After 5 min, the table was tilted to 70° for 15 min to induce orthostatic challenge. After which, participants were asked to step off the tilt table into a force platform, where they stood upright with eyes open for 6 min. The procedure was conducted in a reduced sensory environment. The experiment was terminated immediately if the participant showed signs of dizziness, nausea, discomfort, risk of syncope (rapid and drastic drop in BP), or upon request. No participants requested early termination nor demonstrated adverse symptoms requiring withdrawal from the study.
Data Acquisition
We recorded electrocardiogram (ECG) in the lead I electrode configuration from BIOPAC systems, continuous non-invasive blood pressure (BP) using a finger photoplethysmography cuff (Finapres Nova, FMS, Netherlands), and electromyogram (BIOPAC EMG system) from 21 PD patients (males: 13; females: 8) and 18 healthy controls (males: 4; females: 14) in supine, head-up tilt at 70° and standing positions with eyes open. The electromyogram (EMG) of four different leg muscles on each leg, bilateral tibialis anterior (TA), lateral and medial gastrocnemius (LG, MG), and soleus (SOL) were acquired following the SENIAM recommendations for sensor placement (Hermens et al., 1999). Data were acquired at a sampling rate of 2,000 Hz through BIOPAC Systems.
Data Processing
Due to the presence of movement artifacts during the transition phase between tilt and standing, the first minute of data was not included in the analyses. Therefore, minutes two through six of supine, tilt, and standing data were analyzed. RR intervals were obtained from the ECG signal. Beat-to-beat systolic blood pressure (SBP) and diastolic blood pressure (DBP) were calculated as the maximum and the minimum value in the blood pressure waveform within a heartbeat. Mean arterial pressure (MAP) was obtained from SBP and DBP as:
In this study, we were interested in investigating blood pressure control through lower limb muscles contraction, thus EMG signals from all individual muscles were added to represent the overall muscle activity (Garg et al., 2013; Garg et al., 2014; Xu et al., 2020). EMG data was rectified (absolute value), transformed to have zero-mean, and low pass filtered (cutoff frequency of 20 Hz). The EMG envelope was then captured by a moving average filter whose cutoff frequency was recommended by the SENIAM project to be within 5–20 Hz (Hermens et al., 1999). Since the cardiopostural control response occurs in the low-frequency (<0.5 Hz) (Borst and Karemaker 1983; Wallin et al., 1994; Blaber et al., 2009; Xu et al., 2017), a cutoff frequency of 5 Hz was used for the filter in EMG envelope extraction. Finally, the area under the EMG envelope within each heartbeat [EMG impulse (
In this work, we used wavelet transform coherence (Grinsted et al., 2004; Garg et al., 2013; Garg et al., 2014; Tian et al., 2016) to study the interaction in time and frequency between the following signal pairs
1) Fraction time active (FTA) was calculated as the portion of time during which the squared cross-wavelet coherence
2) The gain reflects the relative amplitude between the signals of each pair over a specified frequency range and it was calculated as the mean value of gain at each time scale over the periods where
3) The active gain was calculated as (FTA × Gain) to study the effectiveness of the cardiac baroreflex and cardio-postural coupling (Xu et al., 2020).
Convergent cross-mapping (CCM) (Sugihara et al., 2012) technique was used to study the bidirectional causalities between the following signal pairs:
Statistical Analysis
Since data were recorded on supine, tilt, and standing positions and not all response variables were normally distributed, we used a nonparametric Anova-type statistic (nparLD, F1-LD-F1 design) suggested by (Brunner et al., 2002). The F1-LD-F1 design refers to an experimental design with one between-subjects factor (Parkinson’s patients and healthy controls) and one within-subjects factor (supine, tilt and stand). This design is employed to study the differences between PD and HC during supine, tilt, and stand, in addition, to investigate the interaction between the two factors on the calculated response variables. To investigate the pairwise differences between supine, tilt, and standing (time main effect), we applied multiple comparisons (LD-F1 design) with Bonferroni adjustment. Wilcoxon rank-sum tests were used to study the differences between Parkinson’s patients and healthy controls (treatment main effects) in supine, tilt, and standing positions. The test results were considered significant at
Results
Parkinson’s patients varied in disease severity [Hoehn and Yahr Stage I: 12 (57%), Stage II: 7 (33%), Stage III: 2 (10%)]. All patients were on either Carbidopa/Levodopa alone or Carbidopa/Levodopa with dopamine agonists or Monoamine Oxidase Type B (MAO-B) inhibitors. Table 1 shows participants’ characteristics.
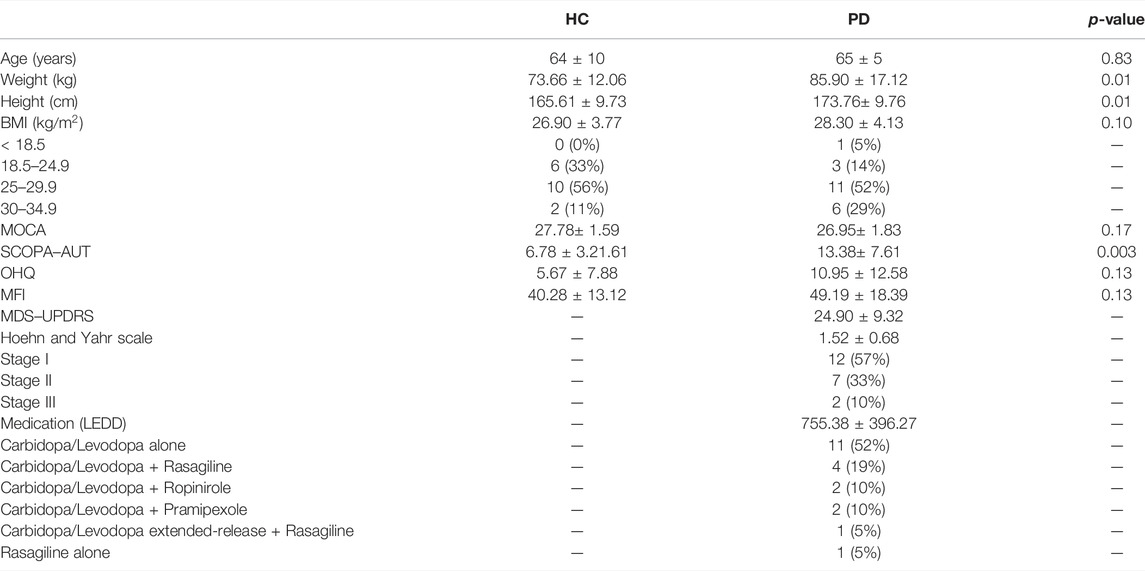
TABLE 1. Characteristics of Parkinson’s disease patients and healthy controls considered in this study. Body mass index (BMI), Montreal Cognitive Assessment (MOCA), Scales for Outcomes in Parkinson’s disease–Autonomic Dysfunction (SCOPA–AUT), Orthostatic Hypotension Questionnaire (OHQ), Multidimensional Fatigue Inventory (MFI), Movement Disorder Society–Unified Parkinson’s Disease Rating Scale (MDS–UPDRS), Levodopa Equivalent Daily Dosage (LEDD). The table lists the Wilcoxon rank sum comparison p-value between Parkinson’s disease patients (PD) and healthy controls (HC).
Cardiovascular and Musculoskeletal Variables
Averaged values (over 5 min period) of cardiovascular and musculoskeletal variables are shown in Table 2. No difference in heart rate
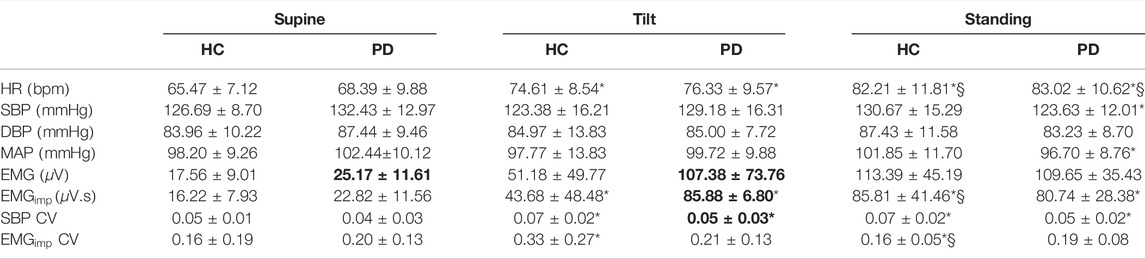
TABLE 2. Cardiovascular and musculoskeletal parameters (mean ± SD) for Parkinson’s disease patients and healthy controls during supine, tilt, and standing positions. HR: heart rate; SBP: systolic blood pressure; DBP: diastolic blood pressure; MAP: mean arterial pressure; EMG: electromyogram;
SBP coefficient of variation was lower
Cardiac Baroreflex
No difference in the cardiac baroreflex
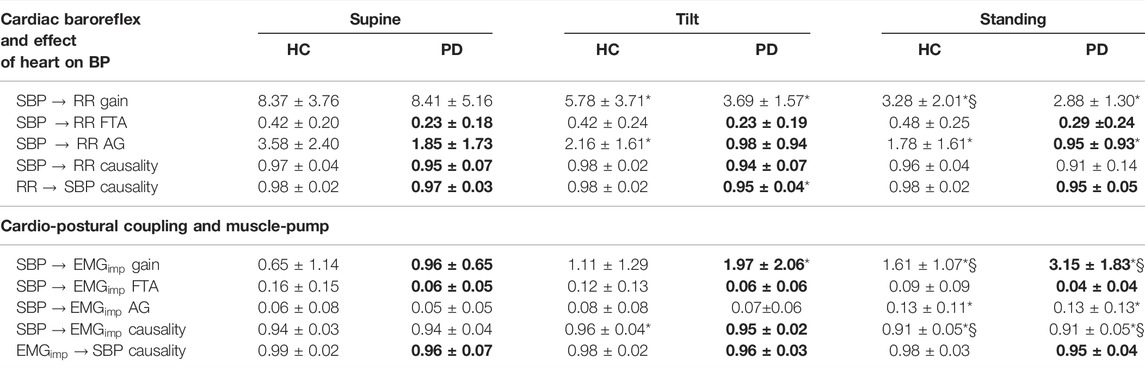
TABLE 3. Comparison of cardiac baroreflex, and cardio-postural coupling between healthy controls and Parkinson’s disease patients during supine, tilt, and standing positions. Table lists mean ± SD of gain, active gain (AG), fraction time active (FTA), and causality values. The Bolded values indicate a significant difference (Wilcoxon rank sum test) between Parkinson’s patients (PD) and healthy controls (HC). *, § represent a significant difference (post-hoc analysis with Bonferroni adjustment) from supine, tilt, respectively.
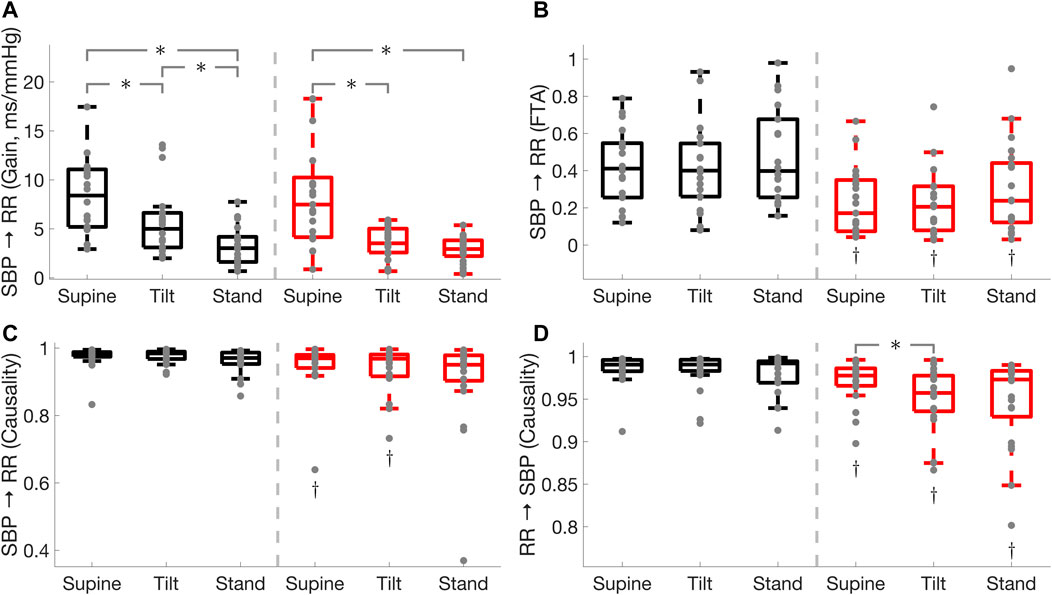
FIGURE 1. Comparison of cardiac baroreflex
Cardiac baroreflex fraction time active was lower in PD during supine (
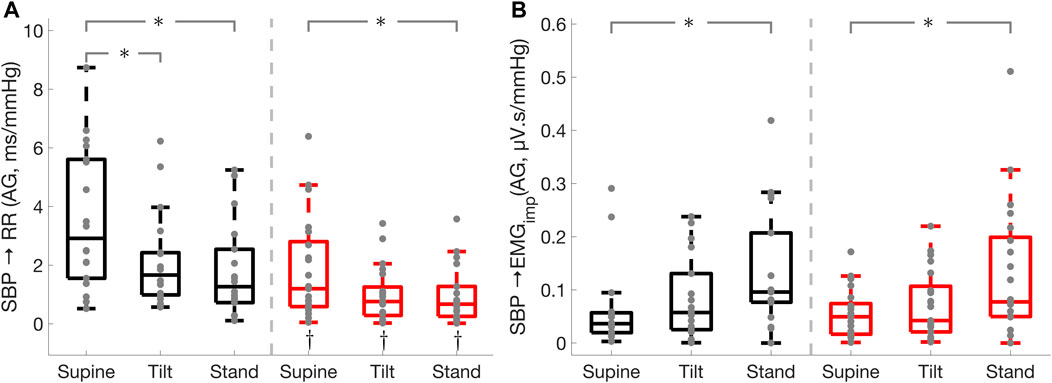
FIGURE 2. Effect of Parkinson’s disease on the effectiveness of the cardiac baroreflex and cardio-postural coupling. Cardiac baroreflex active gain (A), cardio-postural coupling active gain (B). * illustrates a significance difference for multiple comparison (post-hoc analysis with Bonferroni adjustment) between supine, tilt, and standing in Parkinson’s disease patients (red) and healthy controls (black), while † represents a significant difference (Wilcoxon rank sum test) between Parkinson’s disease patients and healthy controls.
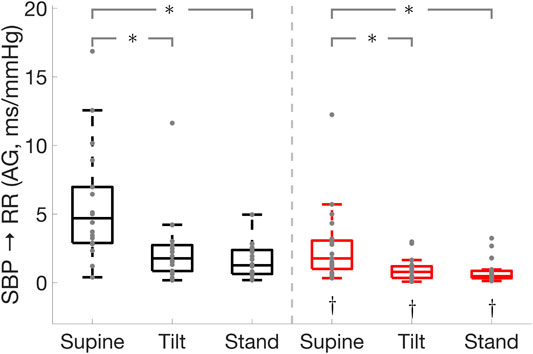
FIGURE 3. Effect of Parkinson’s disease on the cardiac baroreflex
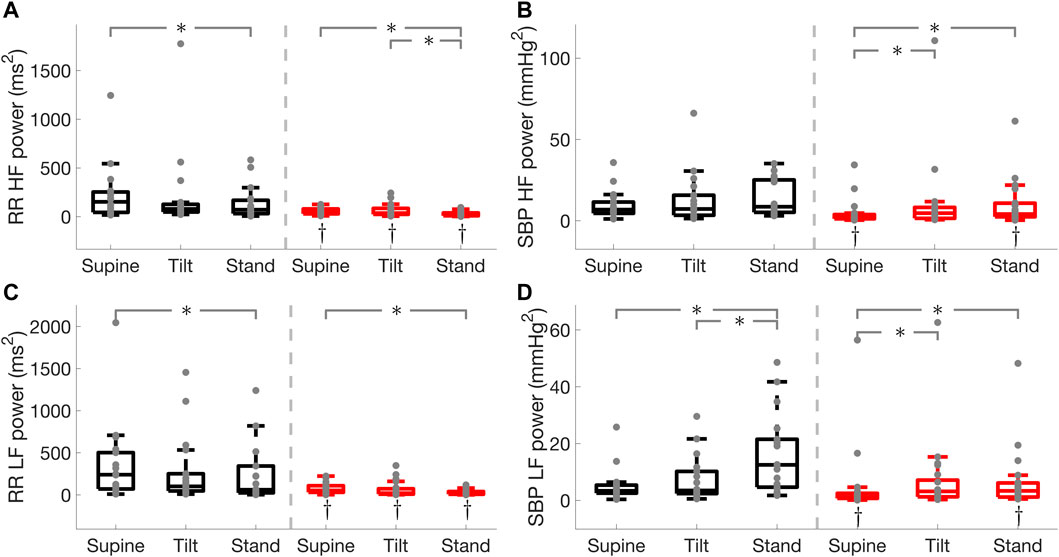
FIGURE 4. RR interval and systolic blood pressure (SBP) spectral power in the high (HF) and low (LF) frequency bands in Parkinson’s disease and healthy controls. RR interval HF power (A), SBP HF power (B), RR interval LF power (C), SBP LF power (D). * illustrates a significance difference for multiple comparison (post-hoc analysis with Bonferroni adjustment) between supine, tilt, and standing in Parkinson’s disease patients (red) and healthy controls (black), while † represents a significant difference (Wilcoxon rank sum test) between Parkinson’s disease patients and healthy controls.
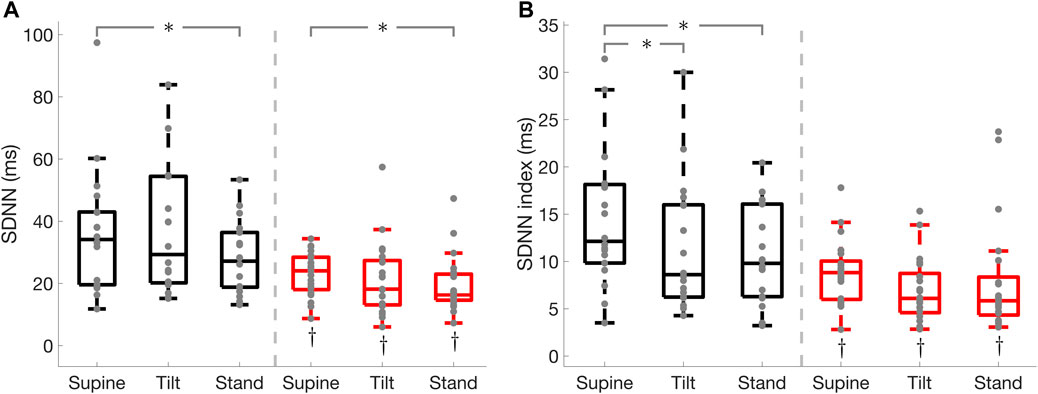
FIGURE 5. Standard deviation of normal RR interval (SDNN), and SDNN index in Parkinson’s disease and healthy controls. SDNN (A), SDNN index (B). * illustrates a significance difference for multiple comparison (post-hoc analysis with Bonferroni adjustment) between supine, tilt, and standing in Parkinson’s disease patients (red) and healthy controls (black), while † represents a significant difference (Wilcoxon rank sum test) between Parkinson’s disease patients and healthy controls.
The cardiac baroreflex
Cardio-Postural Coupling and Muscle-Pump
Cardio-postural coupling (
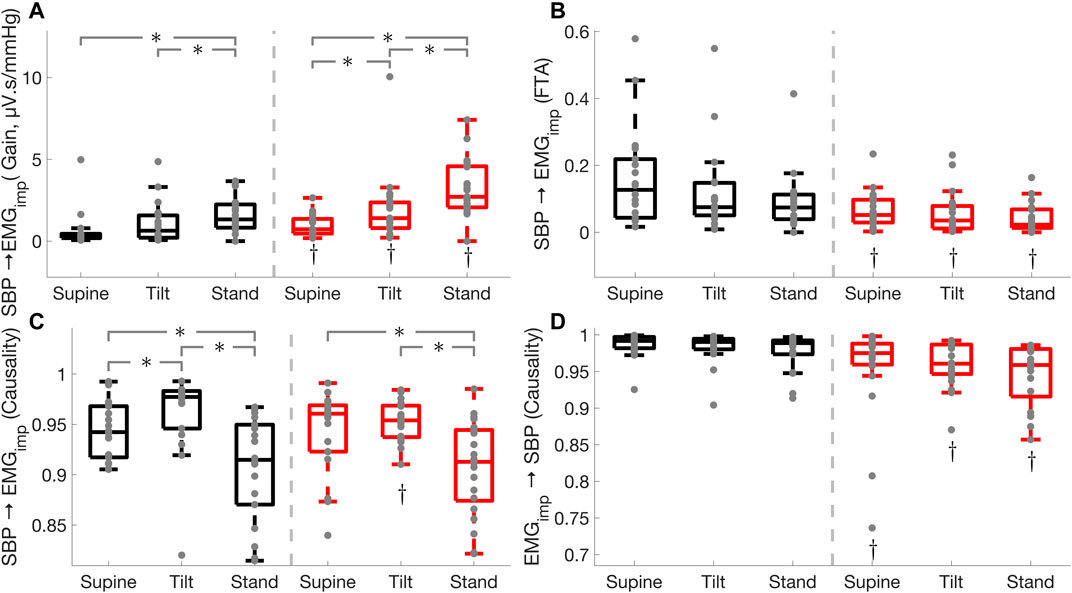
FIGURE 6. Effect of Parkinson’s disease on cardio-postural coupling (
Cardio-postural coupling fraction time active was lower in Parkinson’s patients during supine
Figure 7 shows the different parameters calculated in this study for one Parkinson’s patient and an age-matched healthy control participant during standing. The Parkinson’s patient presented with lower values for all the response variables compared to the healthy control participant, except heart rate,
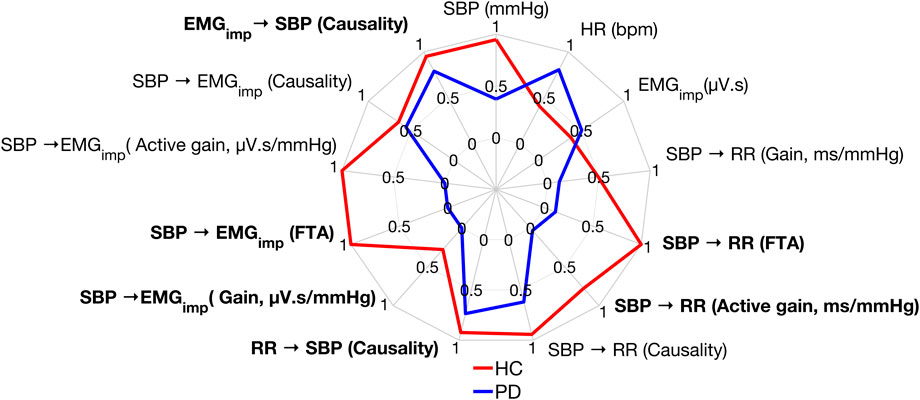
FIGURE 7. Comparison of the response variables calculated in this work between a Parkinson’s patient and a healthy control participant during standing. Data were normalized between 0 and 1 for better visualization of the results. A value of 0, 1 signifies the worst, best performance, respectively. Bolded text indicates a significant difference between Parkinson’s patients and healthy controls during standing.
Discussion
The current study assessed blood pressure regulation in Parkinson’s disease patients and healthy controls through the cardiac baroreflex and a hypothesized reflex muscle-pump function (cardio-postural coupling) during supine, head-up tilt at 70°, and standing. In addition to cardiac baroreflex dysfunction, we found that Parkinson’s disease patients experience reduced mechanical muscle pump (the mechanical effect of leg muscles contractions on blood pressure) and cardio-postural coupling (reflex activity). Impairments in these orthostatic reflexes could result in dizziness and an unexpected fall. We found that adjustments of blood pressure through leg muscles contractions during standing may compensate for, or limit the effect of, the cardiac baroreflex on blood pressure since the muscle-pump had already counteracted changes in blood pressure.
Parkinson’s disease is known to be associated with cardiac baroreflex dysfunction, however, the effect of Parkinson’s disease on cardio-postural coupling has not been previously investigated. Upon standing, 500–1,000 ml of blood shift from the central body compartments to the lower extremities and splanchnic circulation (Lanier et al., 2011), leading to a decreased venous return, cardiac output, and a consequent decline in blood pressure. The drop in blood pressure triggers reflex responses through a hypothesized cardio-postural control center, including cardiac baroreflex and the hypothesized reflex muscle-pump function. The reflex muscle-pump responds to changes in blood pressure by activating leg muscles contractions (muscle-pump) to prevent blood pooling and maintain blood pressure (Xu et al., 2017; Verma et al., 2019; Xu et al., 2020)
Muscle strength deficits and impaired cardiac baroreflex control are responsible for postural instability and contribute to falls in Parkinson’s disease (De Pablo-Fernandez et al., 2017; Gandolfi et al., 2018; Chen et al., 2020). As a result, a thorough understanding of alterations in cardiac baroreflex and cardio-postural coupling might help comprehend the physiology of blood pressure regulation in Parkinson’s disease. Such knowledge can aid in the development of appropriate measures to prevent falls in Parkinson’s disease patients.
Cardiac Baroreflex
Parkinson’s disease patients displayed impaired cardiac baroreflex as shown by the reduced active gain, fraction time active, and RR interval spectral power in the low-frequency band which is thought to be mediated by both sympathetic and parasympathetic activities with a parasympathetic dominance (Shaffer and Ginsberg 2017). In addition, Parkinson’s patients exhibited a lower active gain and RR interval spectral power in the high-frequency band (Figure 3) which reflects parasympathetic modulation of the heart (Shaffer and Ginsberg 2017). This suggests that Parkinson’s patients have impaired sympathetic and vagal modulations. Moreover, Parkinson’s disease patients had lower SBP spectral power in the LF band which is associated with sympathetic vasomotor control (Pagani et al., 1996; Porta et al., 2012; Barbic et al., 2014). While the cardiac baroreflex was lower in Parkinson’s patients compared to healthy controls, its effectiveness was reduced in both groups during standing as compared to the supine position.
Patients with Parkinson’s disease exhibit varying degrees of autonomic dysfunction, which can occur in the early stages of the disease, and symptoms worsen as the Hoehn and Yahr PD stage progresses (Ziemssen and Reichmann 2010; Postuma et al., 2013; De Pablo-Fernandez et al., 2017). The effect of the heart on maintaining blood pressure (
The cardiac baroreflex gain and active gain were reduced from supine to standing in both groups. Upon standing, lower leg muscles contract and maintain blood pressure via the muscle pump mechanism, and thus the effect of the cardiac baroreflex on blood pressure control was lessened in Parkinson’s patients and healthy controls during standing. This is supported by the increased leg muscle activity, muscle-pump gain, and muscle-pump active gain during standing in both groups as compared to supine position.
Cardio-Postural Coupling and Muscle-Pump
Research have shown that the cerebellar fastigial nucleus (FN) which innervates antigravity muscles to maintain postural balance has heavy branches to the nucleus tractus solitarius (NTS), the primary integrative center for respiration and cardiovascular control (Andrezik et al., 1984; Lutherer et al., 1989; Rector et al., 2006; Zhang et al., 2016; Fujita et al., 2020). NTS receives afferent information from the arterial baroreceptors and produces autonomic adjustments to maintain blood pressure. Moreover, fastigial activity has been shown to vary as a function of arterial blood pressure (Lutherer et al., 1989; Rector et al., 2006). In addition to postural control, FN has a profound influence on cardiovascular control and respiration (Andrezik et al., 1984), while FN activity might be a tonic contribution to the autonomic response to hypotension rather than an integral part of the autonomic reflex (Chen et al., 1994). Furthermore, Andrezik et al. (1984) found that neurons that regulate posture in the rostral fastigial nucleus (rFN) are mixed in with neurons that influence the autonomic nervous system in beagles. Moreover, rFN neurons that respond to passive movement also showed changes in firing rate to cardiovascular and respiratory challenges (Lutherer et al., 1989). This suggests that rFN contributes to adaptive autonomic reflex modifications in response to changes in body posture (Rector et al., 2006; Fujita et al., 2020). However, there are certain discrepancies between investigators regarding the role of the FN area on cardiovascular and respiratory control. Some studies have suggested that the effect of the FN on cardiovascular function may result from activation of fibers of passage in autonomic control (Chida et al., 1986; Bradley et al., 1987; Miura and Takayama 1988). Moreover, NTS has been shown to have projections to the paragigantocellularis nucleus (PGi) that sends excitatory fibers to the locus coeruleus (LC) (Williams et al., 2000; Mello-Carpes and Izquierdo 2013; Lopes et al., 2016), which has been shown to modulate neuronal activity of fastigial nucleus (Somana and Walberg 1978; Tao et al., 1998; Zhang et al., 2016; Yu and Wang 2022).
Based on the above information, we suggest that the neurophysiological pathways of the hypothesized blood pressure reflex (cardio-postural coupling) could be as follows: In the supine position, gravitational forces are similar on the thorax, abdomen, and legs as these compartments lie in the same horizontal axis. Consequently, arterial and venous pressures are distributed evenly throughout the body. Upon tilting, gravity acts on the vascular volume causing blood to pool in the lower extremities leading to greater postural variations in blood pressure as compared to the supine position (Table 2). These changes in blood pressure lead to the decrease of baroreceptors discharge to the NTS which relays that information to the rFN (NTS
During the head-up tilt test, participants’ feet were not in contact with any support, suggesting that the increase of muscle activity (
Parkinson’s patients exhibited higher cardio-postural coupling (
Muscle pump
Limitations and Future Work
Parkinson’s patients studied in this research were in the early stages of the disease; therefore, the role of the cardiac baroreflex and cardio-postural coupling towards blood pressure regulation and the mechanical effect of the heart and leg muscles contractions on blood pressure is still to be explored in the advanced stages of Parkinson’s disease. Parkinson’s patients in different disease stages show varying degrees of tremor and bilateral desynchronization which can significantly interfere with the muscle pump’s function to maintain blood pressure. A direct comparison of the proposed aggregated EMG marker between healthy subjects and PD patients in different stages may thus be affected from this fact. Further work will be focused on the proposal of non-aggregated EMG markers, allowing for a more detailed analysis of the muscle pump function in PD patients. Moreover, the effect of respiration on blood pressure was not investigated in this study. Respiration influences both blood pressure and postural stability (Rodrigues et al., 2018; Nuckowska et al., 2019); therefore, future studies addressing the physiology of blood pressure regulation should include respiration as well. Furthermore, the sample size of each group studied was limited; additional research with larger cohorts is needed to fully confirm the effect of Parkinson’s disease on muscle-pump and cardio-postural coupling during the orthostatic challenge. Finally, the analysis of the first minute of data was not possible, mainly due to the noise observed in the signals during this period. However, further analysis of this segment of data could provide additional information on the underlying mechanisms of the hypothesized reflex-dependent muscle activation.
Conclusion
In this study, we investigated the involvement of the cardiac baroreflex and cardio-postural coupling—a hypothesized blood pressure reflex involving the skeletal muscle-pump–in maintaining blood pressure in Parkinson’s disease patients and healthy controls. We also studied the mechanical effect of the heart and leg muscles contractions on blood pressure. The results support the previous research suggesting that the cardiac baroreflex is impaired in PD patients and is likely driven by sympathetic and parasympathetic dysfunction. Furthermore, our study demonstrated novel findings that Parkinson’s disease patients exhibited higher cardio-postural coupling gain but lower cardio-postural coupling active time during supine, tilt, and standing. In addition, the effect of leg muscle activation to maintain blood pressure was lower in Parkinson’s patients during supine, tilt, and standing. We argue that PD-related rigidity, muscle weakness, and delayed muscle responses contribute to PD patients engaging dyssynchronous and ineffective leg muscles contractions without seeing an impactful benefit on blood pressure control. This would suggest PD patients experience a mechanical muscle pump impairment and cardio-postural coupling dysfunction which both could lead to dizziness resulting in an unexpected fall. We have also found that the cardiac baroreflex has a limited effect on blood pressure during standing as lower limb muscles contract to pump blood back to the venous circulation, increasing cardiac output and blood pressure via the muscle-pump mechanism. In conclusion, we found several factors that contribute to poor blood pressure regulation in PD. These factors have meaningful interaction that can contribute to dizziness, fainting, and falls in PD patients. It is imperative to recognize that the findings in this study are not limited to cardiac autonomic dysfunction alone but reflect a greater system entailing lower leg involvement in blood pressure regulation. Finally, causality analysis and wavelet-derived metrics of fraction time active (FTA), gain, and active gain for quantitative evaluation of blood pressure control in Parkinson’s disease indicate a potential to use this methodology for the development of effective interventions to reduce falls in Parkinson’s disease by monitoring the cardiac baroreflex and cardio-postural coupling and their role in maintaining blood pressure and postural stability.
Data Availability Statement
The datasets presented in this article are not readily available because data may only be shared for the use under which it was ethically approved. Requests to access the datasets should be directed to the corresponding author.
Ethics Statement
The studies involving human participants were reviewed and approved by The IRB at Sanford Health and the University of North Dakota approved the protocol (IRB #1445). The patients/participants provided their written informed consent to participate in this study.
Author Contributions
AB and KT conceived research. RF, AH, AB, JL, and KT designed the experiment. RF, AH, RB, and KT performed data acquisition. RF preprocessed and analyzed data. RF performed statistical analysis, created figures and tables and drafted the manuscript. RF, AH, AV, AB, JL, and KT, interpreted results. All authors read, edited, and approved the final manuscript for publication.
Conflict of Interest
The authors declare that the research was conducted in the absence of any commercial or financial relationships that could be construed as a potential conflict of interest.
Publisher’s Note
All claims expressed in this article are solely those of the authors and do not necessarily represent those of their affiliated organizations, or those of the publisher, the editors and the reviewers. Any product that may be evaluated in this article, or claim that may be made by its manufacturer, is not guaranteed or endorsed by the publisher.
Acknowledgments
The authors would like to thank Mr. and Mrs. Joseph Peltier for their endowment to Sanford Parkinson’s disease laboratory. The authors appreciate the valuable help of the participants to complete this study.
Supplementary Material
The Supplementary Material for this article can be found online at: https://www.frontiersin.org/articles/10.3389/fphys.2022.863877/full#supplementary-material
References
Allen N. E., Schwarzel A. K., Canning C. G. (2013). Recurrent Falls in Parkinson's Disease: A Systematic Review. Parkinson's Dis. 2013, 1–16. doi:10.1155/2013/906274
Andrezik J. A., Dormer K. J., Foreman R. D., Person R. J. (1984). Fastigial Nucleus Projections to the Brain Stem in Beagles: Pathways for Autonomic Regulation. Neuroscience 11 (2), 497–507. doi:10.1016/0306-4522(84)90040-x
Armstrong M., Kerndt C. C., Moore R. A. (2021). “Physiology, Baroreceptors,” in StatPearls. Treasure Island (FL). Available at: http://www.ncbi.nlm.nih.gov/books/NBK538172/.
Awerbuch G. I., Sandyk R. (1992). Autonomic Functions in the Early Stages of Parkinson's Disease. Int. J. Neurosci. 64 (1–4), 7–14. doi:10.3109/00207459209000530
Barbic F., Galli M., Dalla Vecchia L., Canesi M., Cimolin V., Porta A., et al. (2014). Effects of Mechanical Stimulation of the Feet on Gait and Cardiovascular Autonomic Control in Parkinson's Disease. J. Appl. Physiology. 116 (5), 495–503. doi:10.1152/japplphysiol.01160.2013
Barbic F., Perego F., Canesi M., Gianni M., Biagiotti S., Costantino G., et al. (2007). Early Abnormalities of Vascular and Cardiac Autonomic Control in Parkinson's Disease Without Orthostatic Hypotension. Hypertension. 49 (1), 120–126. doi:10.1161/01.HYP.0000250939.71343.7c
Barraquand F., Picoche C., Detto M., Hartig F. (2021). Inferring Species Interactions Using Granger Causality and Convergent Cross Mapping. Theor. Ecol. 14 (1), 87–105. doi:10.1007/s12080-020-00482-7
Blaber A. P., Landrock C. K., Souvestre P. A. (2009). Cardio-postural Deconditioning: A Model for Post-flight Orthostatic Intolerance. Respir. Physiology Neurobiol. 169 (Suppl. 1), S21–S25. doi:10.1016/j.resp.2009.04.007
Borst C., Karemaker J. M. (1983). Time Delays in the Human Baroreceptor Reflex. J. Auton. Nerv. Syst. 9 (2–3), 399–409. doi:10.1016/0165-1838(83)90004-8
Bradley D. J., Pascoe J. P., Paton J. F., Spyer K. M. (1987). Cardiovascular and Respiratory Responses Evoked from the Posterior Cerebellar Cortex and Fastigial Nucleus in the Cat. J. Physiology 393, 107–121. doi:10.1113/jphysiol.1987.sp016813
Chen C. H., Williams J. L., Lutherer L. O. (1994). Cerebellar Lesions Alter Autonomic Responses to Transient Isovolaemic Changes in Arterial Pressure in Anaesthetized Cats. Clin. Aut. Res. 4 (5), 263–272. doi:10.1007/BF01827432
Chen Z., Li G., Liu J. (2020). Autonomic Dysfunction in Parkinson's Disease: Implications for Pathophysiology, Diagnosis, and Treatment. Neurobiol. Dis. 134, 104700. doi:10.1016/j.nbd.2019.104700
Chida K., Iadecola C., Underwood M. D., Reis D. J. (1986). A Novel Vasodepressor Response Elicited from the Rat Cerebellar Fastigial Nucleus: the Fastigial Depressor Response. Brain Res. 370 (2), 378–382. doi:10.1016/0006-8993(86)90498-1
Comi C., Magistrelli L., Oggioni G. D., Carecchio M., Fleetwood T., Cantello R., et al. (2014). Peripheral Nervous System Involvement in Parkinson's Disease: Evidence and Controversies. Park. Relat. Disord. 20 (12), 1329–1334. doi:10.1016/j.parkreldis.2014.10.010
Cutsforth-Gregory J. K., Low P. A. (2019). Neurogenic Orthostatic Hypotension in Parkinson Disease: A Primer. Neurol. Ther. 8 (2), 307–324. doi:10.1007/s40120-019-00152-9
de Boer R. W., Karemaker J. M. (2019). Cross-Wavelet Time-Frequency Analysis Reveals Sympathetic Contribution to Baroreflex Sensitivity as Cause of Variable Phase Delay Between Blood Pressure and Heart Rate. Front. Neurosci. 13, 694. doi:10.3389/fnins.2019.00694
De Pablo-Fernandez E., Tur C., Revesz T., Lees A. J., Holton J. L., Warner T. T. (2017). Association of Autonomic Dysfunction With Disease Progression and Survival in Parkinson Disease. JAMA Neurol. 74 (8), 970–976. doi:10.1001/jamaneurol.2017.1125
Espay A. J., LeWitt P. A., Kaufmann H. (2014). Norepinephrine Deficiency in Parkinson's Disease: The Case for Noradrenergic Enhancement. Mov. Disord. 29 (14), 1710–1719. doi:10.1002/mds.26048
Fanciulli A., Leys F., Falup-Pecurariu C., Thijs R., Wenning G. K. (2020). Management of Orthostatic Hypotension in Parkinson's Disease. Jpd 10 (s1), S57–S64. doi:10.3233/JPD-202036
Foulon P., De Backer D. (2018). The Hemodynamic Effects of Norepinephrine: Far More Than an Increase in Blood Pressure!. Ann. Transl. Med. 6 (Suppl. 1), S25. doi:10.21037/atm.2018.09.27
Fujita H., Kodama T., du Lac S. (2020). Modular Output Circuits of the Fastigial Nucleus for Diverse Motor and Nonmotor Functions of the Cerebellar Vermis. eLife 9, e58613. doi:10.7554/eLife.58613
Gandolfi M., Valè N., Filippetti M., Kirilova Dimitrova E., Geroin C., Picelli A., et al. (2018). “Postural Control in Individuals with Parkinson's Disease,” in Different Areas of Physiotherapy. doi:10.5772/intechopen.81098
Garg A., Xu D., Blaber A. P. (2013). Statistical Validation of Wavelet Transform Coherence Method to Assess the Transfer of Calf Muscle Activation to Blood Pressure during Quiet Standing. Biomed. Eng. OnLine 12, 132. doi:10.1186/1475-925X-12-132
Garg A., Xu D., Laurin A., Blaber A. P. (2014). Physiological Interdependence of the Cardiovascular and Postural Control Systems under Orthostatic Stress. Am. J. Physiology-Heart Circulatory Physiology 307 (2), H259–H264. doi:10.1152/ajpheart.00171.2014
Goetz C. G., Fahn S., Martinez-Martin P., Poewe W., Sampaio C., Stebbins G. T., et al. (2007). Movement Disorder Society-Sponsored Revision of the Unified Parkinson's Disease Rating Scale (MDS-UPDRS): Process, Format, and Clinimetric Testing Plan. Mov. Disord. 22 (1), 41–47. doi:10.1002/mds.21198
Goldstein D. S. (2014). Dysautonomia in Parkinson Disease. Compr. Physiol. 4 (2), 805–826. doi:10.1002/cphy.c130026
Goldstein D. S. (2006). Orthostatic Hypotension as an Early Finding in Parkinson's Disease. Clin. Auton. Res. 16 (1), 46–54. doi:10.1007/s10286-006-0317-8
Grasso M., Mazzini L., Schieppati M. (1996). Muscle Relaxation in Parkinson's Disease: A Reaction Time Study. Mov. Disord. 11 (4), 411–420. doi:10.1002/mds.870110410
Grinsted A., Moore J. C., Jevrejeva S. (2004). Application of the Cross Wavelet Transform and Wavelet Coherence to Geophysical Time Series. Nonlin. Process. Geophys. 11 (5/6), 561–566. doi:10.5194/npg-11-561-2004
Hermens H., Bart F., Roberto M., Dick S., Blok J., Rau G., et al. (1999). SENIAM 8: European Recommendations for Surface Electromyography. Roessingh Res. Dev.
Horsager J. (2021). Vagus Nerve Cross-Sectional Area in Patients With Parkinson’s Disease—An Ultrasound Case-Control Study. Front. Neurology 12, 681413. doi:10.3389/fneur.2021.681413
Inkster L. M., Eng J. J., MacIntyre D. L., Stoessl A. J. (2003). Leg Muscle Strength Is Reduced in Parkinson's Disease and Relates to the Ability to Rise from a Chair. Mov. Disord. 18 (2), 157–162. doi:10.1002/mds.10299
Kamieniarz A., Michalska J., Marszałek W., Stania M., Słomka K. J., Gorzkowska A., et al. (2021). Detection of Postural Control in Early Parkinson's Disease: Clinical Testing vs. Modulation of Center of Pressure. PLOS ONE 16 (1), e0245353. doi:10.1371/journal.pone.0245353
Kang G. A., Bronstein J. M., Masterman D. L., Redelings M., Crum J. A., Ritz B. (2005). Clinical Characteristics in Early Parkinson's Disease in a Central California Population-Based Study. Mov. Disord. 20 (9), 1133–1142. doi:10.1002/mds.20513
Kato K., Kanosue K. (2015). “Muscle Relaxation and Sports,” in Sports Performance. Editors K. Kanosue, T. Nagami, and J. Tsuchiya (Tokyo: Springer Japan), 67–78. doi:10.1007/978-4-431-55315-1_7
Kato K., Vogt T., Kanosue K. (2019). Brain Activity Underlying Muscle Relaxation. Frontiers In Physiology 10. doi:10.3389/fphys.2019.01457
Kaufmann H., Malamut R., Norcliffe-Kaufmann L., Rosa K., Freeman R. (2012). The Orthostatic Hypotension Questionnaire (OHQ): Validation of a Novel Symptom Assessment Scale. Clin. Auton. Res. 22 (2), 79–90. doi:10.1007/s10286-011-0146-2
Kim J. B., Kim B.-J., Koh S.-B., Park K.-W. (2014). Autonomic Dysfunction According to Disease Progression in Parkinson's Disease. Park. Relat. Disord. 20 (3), 303–307. doi:10.1016/j.parkreldis.2013.12.001
Lanier J. B., Mote M. B., Clay E. C. (2011). Evaluation and Management of Orthostatic Hypotension. Am. Fam. Physician 84 (5), 527–536.
Lavin K. M., Sealfon S. C., McDonald M.-L. N., Roberts B. M., Wilk K., Nair V. D., et al. (2020). Skeletal Muscle Transcriptional Networks Linked to Type I Myofiber Grouping in Parkinson's Disease. J. Appl. Physiology 128 (2), 229–240. doi:10.1152/japplphysiol.00702.2019
LeWitt P. A., Kymes S., Hauser R. A. (2020). Parkinson Disease and Orthostatic Hypotension in the Elderly: Recognition and Management of Risk Factors for Falls. Aging Dis. 11 (3), 679–691. doi:10.14336/AD.2019.0805
Lopes L. T., Patrone L. G. A., Li K.-Y., Imber A. N., Graham C. D., Gargaglioni L. H., et al. (2016). Anatomical and Functional Connections between the Locus Coeruleus and the Nucleus Tractus Solitarius in Neonatal Rats. Neuroscience 324, 446–468. doi:10.1016/j.neuroscience.2016.03.036
Lutherer L. O., Williams J. L., Everse S. J. (1989). Neurons of the Rostral Fastigial Nucleus Are Responsive to Cardiovascular and Respiratory Challenges. J. Aut. Nerv. Syst. 27 (2), 101–111. doi:10.1016/0165-1838(89)90092-1
Mar P. L., Raj S. R. (2018). Orthostatic Hypotension for the Cardiologist. Curr. Opin. Cardiol. 33 (1), 66–72. doi:10.1097/HCO.0000000000000467
Mazzoni P., Shabbott B., Cortés J. C. (2012). Motor Control Abnormalities in Parkinson's Disease. Cold Spring Harb. Perspect. Med. 2 (6), a009282. doi:10.1101/cshperspect.a009282
Mello-Carpes P. B., Izquierdo I. (2013). The Nucleus of the Solitary Tract→Nucleus Paragigantocellularis→Locus Coeruleus→CA1 Region of Dorsal hippocampus Pathway Is Important for Consolidation of Object Recognition Memory. Neurobiol. Learn. Mem. 100, 56–63. doi:10.1016/j.nlm.2012.12.002
Miura M., Takayama K. (1988). The Site of the Origin of the So-Called Fastigial Pressor Response. Brain Res. 473 (2), 352–358. doi:10.1016/0006-8993(88)90865-7
Miyasato R. S., Silva-Batista C., Peçanha T., Low D. A., de Mello M. T., Piemonte M. E. P., et al. (2018). Cardiovascular Responses During Resistance Exercise in Patients With Parkinson Disease. PM&R 10 (11), 1145–1152. doi:10.1016/j.pmrj.2018.04.009
Nasreddine Z. S., Phillips N. A., Bã©dirian V. r., Charbonneau S., Whitehead V., Collin I., et al. (2005). The Montreal Cognitive Assessment, MoCA: a Brief Screening Tool for Mild Cognitive Impairment. J. Am. Geriatrics Soc. 53 (4), 695–699. doi:10.1111/j.1532-5415.2005.53221.x
Noguchi K., Gel Y. R., Brunner E., Konietschke F. (2002). nparLD: AnRSoftware Package for the Nonparametric Analysis of Longitudinal Data in Factorial Experiments. J. Stat. Soft. 50. doi:10.18637/JSS.V050.I12
Nuckowska M. K., Gruszecki M., Kot J., Wolf J., Guminski W., Frydrychowski A. F., et al. (2019). Impact of Slow Breathing on the Blood Pressure and Subarachnoid Space Width Oscillations in Humans. Sci. Rep. 9 (1), 6232. doi:10.1038/s41598-019-42552-9
Pagani M., Lucini D., Rimoldi O., Furlan R., Piazza S., Porta A., et al. (1996). Low and High Frequency Components of Blood Pressure Variability. Ann. N. Y. Acad. Sci. 783 (1), 10–23. doi:10.1111/j.1749-6632.1996.tb26704.x
Palma J.-A., Gomez-Esteban J. C., Norcliffe-Kaufmann L., Martinez J., Tijero B., Berganzo K., et al. (2015). Orthostatic Hypotension in Parkinson Disease: How Much You Fall or How Low You Go? Mov. Disord. 30 (5), 639–645. doi:10.1002/mds.26079
Pérez T., Tijero B., Gabilondo I., Luna A., Llorens V., Berganzo K., et al. (2015). Cardiocirculatory Manifestations in Parkinson's Disease Patients without Orthostatic Hypotension. J. Hum. Hypertens. 29 (10), 604–609. doi:10.1038/jhh.2014.131
Porta A., Castiglioni P., Rienzo M. D., Bari V., Bassani T., Marchi A., et al. (2012). Short-term Complexity Indexes of Heart Period and Systolic Arterial Pressure Variabilities Provide Complementary Information. J. Appl. Physiology 113 (12), 1810–1820. doi:10.1152/japplphysiol.00755.2012
Postuma R. B., Gagnon J.-F., Pelletier A., Montplaisir J. (2013). Prodromal Autonomic Symptoms and Signs in Parkinson's Disease and Dementia with Lewy Bodies. Mov. Disord. 28 (5), 597–604. doi:10.1002/mds.25445
R Development Core Team (2010). A Language and Environment for Statistical Computing: Reference Index. Vienna: R Foundation for Statistical Computing. Available at: http://www.polsci.wvu.edu/duval/PS603/Notes/R/fullrefman.pdf.
Rector D. M., Richard C. A., Harper R. M. (2006). Cerebellar Fastigial Nuclei Activity during Blood Pressure Challenges. J. Appl. Physiology 101 (2), 549–555. doi:10.1152/japplphysiol.00044.2006
Rodrigues G. D., Gurgel J. L., Gonçalves T. R., Porto F., Soaresda P. P. d. S. S. (2018). Influence of Breathing Patterns and Orthostatic Stress on Postural Control in Older Adults. Geriatr. Gerontol. Int. 18 (5), 692–697. doi:10.1111/ggi.13231
Shaffer F., Ginsberg J. P. (2017). An Overview of Heart Rate Variability Metrics and Norms. Front. Public Health 5, 258. doi:10.3389/fpubh.2017.00258
Shibata M., Morita Y., Shimizu T., Takahashi K., Suzuki N. (2009). Cardiac Parasympathetic Dysfunction Concurrent with Cardiac Sympathetic Denervation in Parkinson's Disease. J. Neurological Sci. 276 (1–2), 79–83. doi:10.1016/j.jns.2008.09.005
Smets E. M. A., Garssen B., Bonke B., De Haes J. C. J. M. (1995). The Multidimensional Fatigue Inventory (MFI) Psychometric Qualities of an Instrument to Assess Fatigue. J. Psychosomatic Res. 39 (3), 315–325. doi:10.1016/0022-3999(94)00125-o
Somana R., Walberg F. (1979). The Cerebellar Projection from Locus Coeruleus as Studied with Retrograde Transport of Horseradish Peroxidase in the Cat. Anat. Embryol. 155 (1), 87–94. doi:10.1007/BF00315733
Sugihara G., May R. M. (1990). Nonlinear Forecasting as a Way of Distinguishing Chaos from Measurement Error in Time Series. Nature 344 (6268), 734–741. doi:10.1038/344734a0
Sugihara G., May R., Ye H., Hsieh C.-h., Deyle E., Fogarty M., et al. (2012). Detecting Causality in Complex Ecosystems. Science 338 (6106), 496–500. doi:10.1126/science.1227079
Tao W., Jianjun W., Hong C., Hongzhao L., Qixiang Y. (1998). Modulation of Neuronal Activity of Cerebellar Fastigial Nucleus by Locus Coeruleus Stimulation in the Rat. Chin. Sci. Bull. 43 (11), 940–944. doi:10.1007/BF02884618
Tian F., Tarumi T., Liu H., Zhang R., Chalak L. (2016). Wavelet Coherence Analysis of Dynamic Cerebral Autoregulation in Neonatal Hypoxic-Ischemic Encephalopathy. NeuroImage Clin. 11, 124–132. doi:10.1016/j.nicl.2016.01.020
Tomlinson C. L., Stowe R., Patel S., Rick C., Gray R., Clarke C. E. (2010). Systematic Review of Levodopa Dose Equivalency Reporting in Parkinson's Disease. Mov. Disord. 25 (15), 2649–2653. doi:10.1002/mds.23429
Torrence C., Compo G. P. (1998). A Practical Guide to Wavelet Analysis. Bull. Amer. Meteor. Soc. 79 (1), 61–78. doi:10.1175/1520-0477(1998)079<0061
Tsonis A. A., Deyle E. R., Ye H., Sugihara G. (2018). “Convergent Cross Mapping: Theory and an Example,” in Advances in Nonlinear Geosciences. Editor A. A. Tsonis (Cham: Springer International Publishing), 587–600. doi:10.1007/978-3-319-58895-7_27
Velseboer D. C., de Haan R. J., Wieling W., Goldstein D. S., de Bie R. M. A. (2011). Prevalence of Orthostatic Hypotension in Parkinson's Disease: A Systematic Review and Meta-Analysis. Park. Relat. Disord. 17 (10), 724–729. doi:10.1016/j.parkreldis.2011.04.016
Verma A. K., Garg A., Xu D., Bruner M., Fazel-Rezai R., Blaber A. P., et al. (2017). Skeletal Muscle Pump Drives Control of Cardiovascular and Postural Systems. Sci. Rep. 7 (1), 45301. doi:10.1038/srep45301
Verma A. K., Xu D., Garg A., Blaber A. P., Tavakolian K. (2019). Effect of Aging on Muscle-Pump Baroreflex of Individual Leg Muscles During Standing. Frontiers In Physiology 10. doi:10.3389/fphys.2019.00845
Visser M., Marinus J., Stiggelbout A. M., Van Hilten J. J. (2004). Assessment of Autonomic Dysfunction in Parkinson's Disease: The SCOPA-AUT. Mov. Disord. 19 (11), 1306–1312. doi:10.1002/mds.20153
Wallin B. G., Burke D., Gandevia S. (1994). Coupling between Variations in Strength and Baroreflex Latency of Sympathetic Discharges in Human Muscle Nerves. J. Physiology 474 (2), 331–338. doi:10.1113/jphysiol.1994.sp020025
Wallot S., Mønster D. (2018). Calculation of Average Mutual Information (AMI) and False-Nearest Neighbors (FNN) for the Estimation of Embedding Parameters of Multidimensional Time Series in Matlab. Front. Psychol. 9, 1679. doi:10.3389/fpsyg.2018.01679
Walter U., Tsiberidou P., Kersten M., Storch A., Löhle M. (2018). Atrophy of the Vagus Nerve in Parkinson’s Disease Revealed by High-Resolution Ultrasonography. Frontiers In Neurology 9. doi:10.3389/fneur.2018.00805
Williams C. L., Men D., Clayton E. C. (2000). The Effects of Noradrenergic Activation of the Nucleus Tractus Solitarius on Memory and in Potentiating Norepinephrine Release in the Amygdala. Behav. Neurosci. 114 (6), 1131–1144. doi:10.1037/0735-7044.114.6.1131
Xu D., Tremblay M. F., Verma A. K., Tavakolian K., Goswami N., Blaber A. P. (2020). Cardio-postural Interactions and Muscle-Pump Baroreflex Are Severely Impacted by 60-day Bedrest Immobilization. Sci. Rep. 10 (1), 12042. doi:10.1038/s41598-020-68962-8
Xu D., Verma A. K., Garg A., Bruner M., Fazel-Rezai R., Blaber A. P., et al. (2017). Significant Role of the Cardiopostural Interaction in Blood Pressure Regulation during Standing. Am. J. Physiology-Heart Circulatory Physiology 313 (3), H568–H577. doi:10.1152/ajpheart.00836.2016
Yoo S.-W., Kim J.-S., Yoo J.-Y., Yun E., Yoon U., Shin N.-Y., et al. (2021). Delayed Orthostatic Hypotension in Parkinson's Disease. npj Park. Dis. 7 (1), 1–8. doi:10.1038/s41531-021-00181-y
Yu M., Wang S.-M. (2022). “Neuroanatomy, Nucleus Fastigial,” in StatPearls. Treasure Island (FL). Available at: http://www.ncbi.nlm.nih.gov/books/NBK547738/(Accessed May 2, 2022).
Zhang X.-Y., Wang J.-J., Zhu J.-N. (2016). Cerebellar Fastigial Nucleus: from Anatomic Construction to Physiological Functions. cerebellum ataxias 3 (1), 9. doi:10.1186/s40673-016-0047-1
Keywords: Parkinson’s disease, falls, muscle-pump, cardiac baroreflex, blood pressure regulation, cardio-postural coupling
Citation: Fadil R, Huether AXA, Verma AK, Brunnemer R, Blaber AP, Lou J-S and Tavakolian K (2022) Effect of Parkinson’s Disease on Cardio-postural Coupling During Orthostatic Challenge. Front. Physiol. 13:863877. doi: 10.3389/fphys.2022.863877
Received: 27 January 2022; Accepted: 06 May 2022;
Published: 03 June 2022.
Edited by:
Alessandro Silvani, University of Bologna, ItalyReviewed by:
Vlasta Bari, IRCCS San Donato Polyclinic, ItalyAlfredo Hernandez, Institut National de la Santé et de la Recherche Médicale (INSERM), France
David Cristóbal Andrade, University of Antofagasta, Chile
Copyright © 2022 Fadil, Huether, Verma, Brunnemer, Blaber, Lou and Tavakolian. This is an open-access article distributed under the terms of the Creative Commons Attribution License (CC BY). The use, distribution or reproduction in other forums is permitted, provided the original author(s) and the copyright owner(s) are credited and that the original publication in this journal is cited, in accordance with accepted academic practice. No use, distribution or reproduction is permitted which does not comply with these terms.
*Correspondence: Kouhyar Tavakolian, a291aHlhckB1bmQuZWR1
†These authors have contributed equally to this work