- 1Anesthesia and Critical Care Research Group, Faculty of Health Sciences, Department of Clinical Medicine, UiT The Arctic University of Norway, Tromsø, Norway
- 2Division of Surgical Medicine and Intensive Care, University Hospital of North Norway, Tromsø, Norway
- 3Emergency Medical Services, University Hospital of North Norway, Tromsø, Norway
- 4Cardiothoracic Research Group, Faculty of Health Sciences, Department of Clinical Medicine, UiT The Arctic University of Norway, Tromsø, Norway
- 5Cardiothoracic and Respiratory Medicine, University Hospital of North Norway, Tromsø, Norway
- 6Department of Physiology and Biomedical Engineering, Mayo Clinic College of Medicine & Science, Rochester, MN, United States
Introduction: Cooling by cardiopulmonary bypass (CPB) to deep hypothermic cardiac arrest (HCA) for cardiac surgical interventions, followed by CPB-rewarming is performed on a routine basis with relatively low mortality. In contrast, victims of deep accidental hypothermia rewarmed with CPB generally have a much worse prognosis. Thus, we have developed an intact pig model to compare effects on perfusion pressures and global oxygen delivery (DO2) during immersion cooling versus cooling by CPB. Further, we compared the effects of CPB-rewarming between groups, to restitute cardiovascular function, brain blood flow, and brain metabolism.
Materials and Methods: Total sixteen healthy, anesthetized juvenile (2–3 months) castrated male pigs were randomized in a prospective, open placebo-controlled experimental study to immersion cooling (IMMc, n = 8), or cooling by CPB (CPBc, n = 8). After 75 minutes of deep HCA in both groups, pigs were rewarmed by CPB. After weaning from CPB surviving animals were observed for 2 h before euthanasia.
Results: Survival rates at 2 h after completed rewarming were 4 out of 8 in the IMMc group, and 8 out of 8 in the CPBc group. Compared with the CPBc-group, IMMc animals showed significant reduction in DO2, mean arterial pressure (MAP), cerebral perfusion pressure, and blood flow during cooling below 25°C as well as after weaning from CPB after rewarming. After rewarming, brain blood flow returned to control in CPBc animals only, and brain micro dialysate-data showed a significantly increase in the lactate/pyruvate ratio in IMMc vs. CPBc animals.
Conclusion: Our data indicate that, although global O2 consumption was independent of DO2, regional ischemic damage may have taken place during cooling in the brain of IMMc animals below 25°C. The need for prolonged extracorporeal membrane oxygenation (ECMO) should be considered in all victims of accidental hypothermic arrest that cannot be weaned from CPB immediately after rewarming.
Introduction
Cooling by cardiopulmonary bypass (CPB) until deep hypothermic cardiac arrest (HCA) at 15–18°C to perform complex aortic surgery followed by rewarming has been proven to be relatively safe over the last 10–15 years. Even in patients with serious co-morbidity, it has been reported that the overall hospital mortality associated with CPB is only around 5% (Bakaeen et al., 2010; Fehrenbacher et al., 2010). These results contrast with the reported hospital mortality of 70–87% after extracorporeal rewarming of victims of accidental hypothermia (Kornberger and Mair, 1996; Farstad et al., 2001). In many of these cases, hypothermia is secondary and preceded by asphyxia, as during submersion (drowning) (Farstad et al., 2001), or by burying in avalanches (Boyd et al., 2010). Successful CPB rewarming from accidental circulatory arrest at low body core temperatures has proven to be demanding, even in fit young survivors. Cardiopulmonary failure as well as renal insufficiency occurred early during intensive care treatment, irrespective if circulatory arrest was caused by primary hypothermia, and/or the interval between cardiac arrest and qualified cardiopulmonary resuscitation was relatively short (Gilbert et al., 2000; Oberhammer et al., 2008).
Fortunately, accidental hypothermia has received a lot of attention over the past 15 years (Paal et al., 2013), which has contributed to the creation of new treatment algorithms (Paal et al., 2016, 2022) that can be used in trauma centers. Progress has also been made after introduction of new techniques (Winkler et al., 2016), like extracorporeal membrane oxygenation (ECMO), for rewarming accidental hypothermia victims in cardiac arrest. The use of ECMO may be associated with higher survival rates and more favorable neurological outcomes in these patients than after rewarming using traditional CPB (Ruttmann et al., 2007; Morita et al., 2011).
Experimental data support the notion that the process of accidental surface cooling is more detrimental to the organism than the controlled clinical cooling by invasive or non-invasive methods. One apparent difference is the lack of sedation in accidental hypothermia. Experiments on newborn piglets demonstrated that the neuroprotective effect of mild hypothermia (35°C) after hypoxic brain damage was lost, if cooling was performed without deep sedation (Thoresen et al., 2001). Clinically it has been observed that surviving victims of accidental hypothermia influenced by sedative drugs or ethanol tolerated hypothermia and rewarming are better than victims unaffected by these substances (Locher et al., 1991; Vassal et al., 2001). If surface cooling in an anesthetized animal proceeds to deep hypothermia, preclinical studies have demonstrated that a hypothermia-induced, non-ischemic heart failure evolves, depending on the level of hypothermia and exposure time (Fedor et al., 1958; Popovic and Kent, 1965; Kondratiev et al., 2008; Filseth et al., 2010).
On the other hand, cooling by CPB and maintained CPB at low temperatures also have negative side effects. Hypothermic CPB is pro-inflammatory (Tassani et al., 2002), leads to post-hypothermic impaired cardiac ventricle function (Schultz et al., 2006), and invariably causes increased extravasation of fluid and general edema (Heltne et al., 2001; Farstad et al., 2003).
The goal of the present study was to isolate the effects of immersion cooling per se from cooling by CPB by keeping important factors like deep sedation and controlled ventilation similar in both groups. Thus, the immersion cooling protocol in the present study differed from the situation in accidental hypothermia, but we hypothesized that there would be fundamental differences in physiological responses to the two cooling techniques that would have translational therapeutic value for victims of accidental HCA.
Materials and Methods
There are several alternative nomenclatures for hypothermia (Popovic and Popovic, 1974; Moss, 1986). We have chosen the definition by the American Heart Association (Vanden Hoek et al., 2010), which designates 30°C as a “watershed” marker between moderate (30–34°C) and potentially life-threatening (below 30°C) hypothermia, which is referred to as deep hypothermia if applied clinically and severe, if caused accidentally.
Animals and Overall Experimental Protocol
Animals received human care in accordance with The Norwegian Animal Welfare Act and the study (reference number: 37/04) was approved by The Norwegian Animal Research Authority (Forsøksdyrutvalget). Total sixteen castrated male juvenile pigs (24–37 kg, 2–3 months of age) from a hybrid crossing of native Norwegian (norsk landsvin) and British Yorkshire breeds were placed in pens for 2–5 days after arrival to the laboratory animal unit. They were fed twice daily and had free access to water at all the times. The animals were anesthetized and randomized to immersion cooling (IMMc, n = 8) or cooling by CPB (CPBc, n = 8) groups. Cooling continued until hypothermic circulatory arrest (HCA). After 75 minutes of HCA animals in both the groups were rewarmed by CPB. Following weaning from CPB surviving animals were observed for 2 h before euthanasia by an intravenous bolus of 20 mmol potassium chloride during ongoing anesthesia. The overall protocol layout is presented in Figures 1, 2. Figure 1 shows temperature variations in different body locations, time-points for sampling, administration of anesthesia, and cooling/rewarming methods in IMMc (Figure 1A) and CPBc (Figure 1B) groups. Figure 2 shows the time-course of experiments and compares time-dependent temperatures in esophagus (Figure 2A) and urinary bladder (Figure 2B) in the two groups.
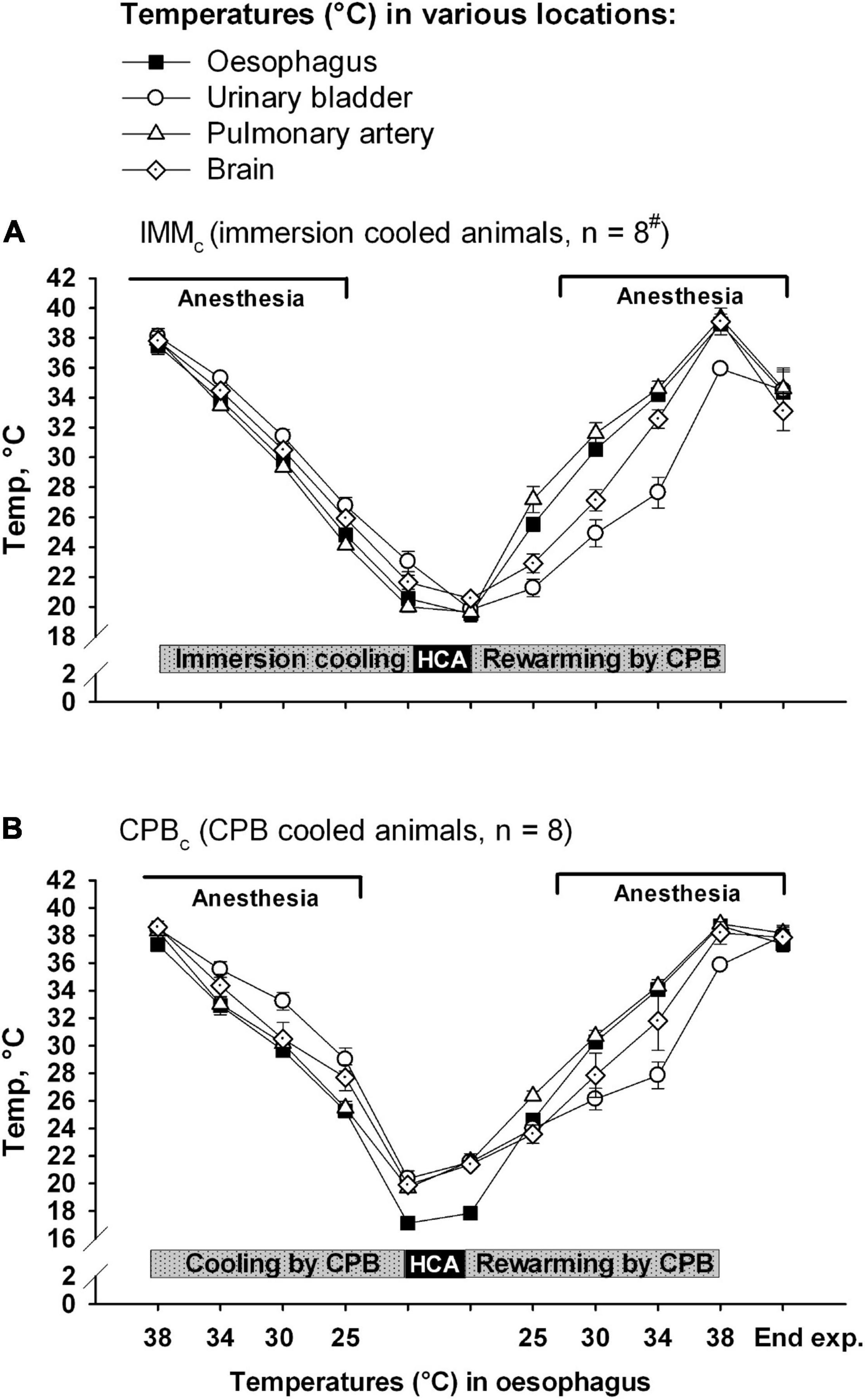
Figure 1. Temperatures at various body locations. (A) Immersion cooled animals (IMMc group), and (B) in animals cooled by CPB (CPBc group). Data presented as mean and SEM. #n was reduced to 4 animals at the end of experiments (End exp.) in IMMc group.
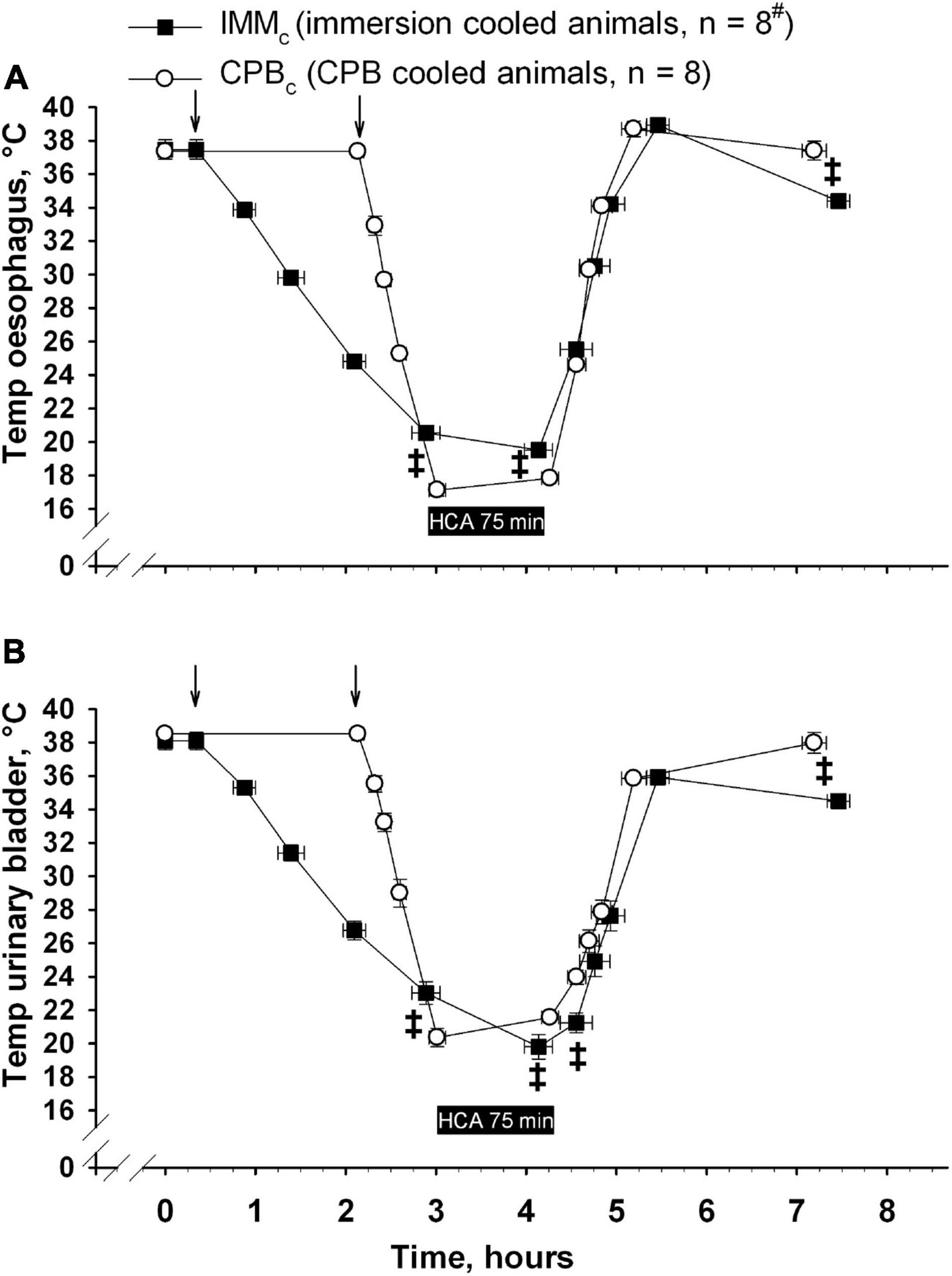
Figure 2. Time-dependent changes in temperature in esophagus (A) and urinary bladder (B). ↓ denotes start of cooling in either group. Data presented as mean and SEM. ‡Statistically significant difference between groups (p < 0.05). #n was reduced to 4 animals at end of the experiments (End exp.) in IMMc group.
Anesthesia and Instrumentation
The animals were fasted overnight before premedication was induced in the pen by a bolus intramuscular injection of ketamine hydrochloride 20 mg/kg, midazolam 25 mg, and atropine 1.0 mg. After weighing and transferring the animals to the operation theater, anesthesia was induced by a bolus infusion of intravenous fentanyl 10 μg/kg, and pentobarbital-sodium 10 mg/kg. Following tracheostomy, the right external jugular vein was catheterized for infusion of fentanyl 20 μg/kg/h, pentobarbital-sodium 4 mg/kg/h, and midazolam 0.3 mg/kg/h together with Ringer‘s acetate 9 ml/kg/h. This infusion was maintained throughout the experiment, except for the period when brain temperature was below 25°C.
Animals were ventilated with a PEEP of 4 cm H2O (Siemens Servo 900 D, Solna, Sweden). The fractional concentration of O2 (FiO2) was adjusted to maintain PaO2 > 10 kPa, and alveolar ventilation was adjusted to keep PaCO2 at 4.5–6.0 kPa, uncorrected for temperature (alpha-stat management).
An arterial catheter was positioned into the left femoral artery for monitoring mean arterial pressure (MAP) and blood sampling. A 5F thermodilution catheter (Edwards Lifesciences, Irvine, California, United States) was advanced via the right external jugular vein for monitoring of central venous pressure (CVP), pulmonary artery pressure (PAP), blood temperature; cardiac output (CO), and central venous saturation. A 6F pigtail high-volume-flush catheter (Cordis, Miami, Florida, United States) was positioned into the left ventricle of the heart through the left carotid artery for monitoring left ventricular pressure. At the end of experiments, the animals were re-weighed.
Recording and Calculation of Hemodynamic Variables
Electrocardiogram (ECG) (from standard leads), heart rate (HR), CVP, MAP, and PAP were continuously displayed on a data monitor and intermittently recorded using the software package LabVIEW™ v.6.0 (National Instruments, Austin, TX, United States). In addition, left ventricular end diastolic pressure (LVEDP) was recorded and maximum and minimum values of the first derivate of ventricular pressure over time (dP/dtmax and dP/dtmin) were calculated by the LabVIEW software.
Cardiac output (CO) was measured by a thermodilution computer (Vigilance, Edwards Lifesciences, Irvine, California, United States), by injecting 5 ml precooled saline in the pulmonary artery catheter. Systemic vascular (SV) and systemic vascular resistance (SVR) were calculated as: SV = CO/HR; SVR = (MAP – CVP) × 80/CO. DO2 and VO2 were calculated as O2 content in arterial blood × CO, and the difference of arterial and mixed venous O2 content × CO, respectively.
Brain Microdialysis and Intracerebral Monitoring
An area of approximately 2 × 5 cm of the skull was exposed over the right hemisphere. A microdialysis catheter (CMA 70, CMA/Microdialysis, Stockholm, Sweden) was positioned at a depth of 10 mm below the duramater through a cranial hole positioned 1 cm right of the sagittal suture and 2 cm rostral to the coronal suture. The catheter was connected to a 1.0 ml syringe placed in a microinfusion pump (CMA102, CMA/Microdialysis) for perfusion of Ringer’s solution at a rate of 2.0 μL/min (Perfusion Fluid CNS, CMA/Microdialysis). Five samples were obtained during the experiment at 30 minutes intervals: at baseline at 30°C and 20°C during cooling, at 30°C during rewarming, and at 2 h after rewarming. The microvials containing the dialysate fluid were immediately frozen at –70°C and concentrations of cerebral tissue glucose, lactate, pyruvate, glutamate, and glycerol were measured using a microdialysis analyzer (CMA 600, CMA/Microdialysis).
A catheter for monitoring of intracerebral pressure (ICP) (Codman MicroSensor ICP Transducer, Codman & Shutleff, Raynham, Massachutes, United States/Millar transducer control unit TC-510, Houston, Texas, United States) and a temperature probe for monitoring intracerebral temperature were placed in the brain parenchyma just below the dura mater through a second cranial hole 1 cm to the right of the sagittal suture and 1 cm caudal to the coronal suture. ICP was displayed continuously on a monitor and recorded manually at different time intervals. Cerebral perfusion pressure (CCP) was calculated as MAP – ICP. Finally, a catheter was retrogradely placed through the left internal jugular vein to sample blood for determination of jugular venous O2 saturation.
Use of Fluorescent Microspheres to Determine Regional Cerebral Blood Flow
Microsphere Injection
For measurements, 15 μm polystyrene fluorescent microspheres (FM), (FluoSpheres, Molecular Probes, Eugene, OR, United States) (Glenny, Bernard, and Brinkley 2585-97) of four different colors (orange, yellow-green, crimson, and red) were injected as follows; at baseline (38°C), at start and at the end of stable hypothermia (25°C), and after rewarming to (38°C). Before each injection, FM were resuspended using a Sonorex Digital 10P ultrasonic bath (Badelin electronic GmbH, Berlin, Germany) for 1 minute and vortexed for 1 minute before 1 ml of the FM suspension (1 × 106 FM) was injected into the left ventricle through the 6F pigtail catheter followed by washing with 10 ml saline. Reference blood samples were drawn, using a withdrawal pump (Harvard Apparatus, Holliston, MA, United States) at a constant rate of 4.12 ml/min, into a preheparinized 20 ml syringe via a catheter inserted into the right femoral artery and advanced to the abdominal part of the aorta. The collection of the reference blood sample began 15 seconds before the FM injection and continued for 2 minutes. After sampling, the syringe and sampling line were rinsed twice with 5 ml saline and placed in the vial. After each experiment, brain biopsies were obtained from the frontal lobes, cerebellum (2–3 g), and hippocampus (0.5–1 g) were placed into sampling vials and processed later for regional blood flow analysis.
Sample Processing
Tissue and blood samples were processed (digesting, filtration, rinsing, and dye extraction) using sample processing units (SPU) (Perkin-Elmer Analytical, Shelton, CT, United States) as described in detail by Raab et al. (1999). The SPU consists of three main parts: filter unit, filter holder, and sample tube with a screw-top cap. All tissue samples were weighed immediately after harvesting. To recover FM from tissue or blood, all samples were digested using 4 N aqueous KOH solution (224.4 g/l) with 2% Tween 80 (20 ml per 1,000 ml of KOH solution, provided by Sigma-Aldrich Norway AS, Oslo, Norway. Each tissue or reference blood sample was placed in a separate filter unit, which was then placed in high-grade steel beaker filled with 25 ml digesting solution, and 2.5 ml 100% isopropanol alcohol, covered with tight caps, and kept in a heating oven for 24 h at 60°C. After the digestion process, filter units were carefully removed from the steel beakers, and the liquid was suctioned through the wire mesh of the filter with a maximum negative pressure of 400 hPa. To neutralize residual KOH, the filter unit wall and the filter mesh were rinsed using 20 ml phosphate buffer (5.88 g KH2PO4 in 200 ml H2O + 29.9 g K2HPO4 in 800 ml H2O) before the filter units were immersed in a beaker filled with phosphate buffer to clean residual KOH from the outside. To remove remaining liquid from the filter units, they were placed in 50 ml plastic tubes and centrifuged for 3 minutes at 4,000 rpm. After digestion, suction, washing, and centrifuging, all FM recovered from tissue or blood were collected on a filter mesh inside the filter units. Then each filter unit was placed into the filter holder with the sample tube connected to the lower end of the filter holder. To extract dye from microspheres, 1 ml of organic solvent (Cellosolve, 2-ethoxyethyl acetate) was pipetted into the filter unit, and the assembly with filter unit inside was vortexed softly for 30 seconds. After 1 minute, a second extraction of dye using 1 ml of Cellosolve was performed. The assembly with filter unit inside was then centrifuged at 4,000 rpm for 30 seconds, and all Cellosolve- containing fluorescent dye samples were collected in a sample tube. The concentration of fluorescent dye dissolved in Cellosolve was measured using a microplate reader (Wallac Victor2TM 1,420 Mulitlabel Counter, Perkin-Elmer Life Sciencis, Turku, Finland). Organ blood flow (OBF) was calculated based on the following formula:
Where, R is the withdrawal rate of the reference blood sample (4.12 ml/min), IT is the fluorescence intensity of the tissue sample, IR is the fluorescence intensity of the reference blood sample, and Wt is the weight of the tissue sample (g).
Cooling and Rewarming Protocols
Temperature Monitoring
Body temperature was continuously monitored at four locations: brain (Licox temperature probe/Licox MCB Universal oxygen and temperature monitor, Mielkendorf, Germany); esophagus; urinary bladder (Kone temperature probes, Espoo, Finland); and pulmonary arterial blood (via the thermodilution catheter). During CPB, temperatures were also monitored in the venous and arterial lines and displayed on the centrifugal pump machine. Esophageal temperature was used as reference temperature for determining sampling points throughout experiments and the end point of cooling, whereas the point of weaning from CPB during rewarming was determined by urinary bladder temperature. At temperatures below 25°C, brain temperature was used to withdraw anesthetics.
Immersion Cooling (IMMc Group Only)
Animals in the IMMc group were placed in a right lateral recumbent position on the operating table. Using a centrifugal pump (Bio-Medicus, Eden Prairie, Minnesota, United States) and heat-exchanger (Stöckert Normo/hypothermie, Munich, Germany), cold water (5°C) was circulated in the hollow operating table and a tarpaulin tub surrounding the animal. The upper left side of the animal was covered with ice slush and irrigated with cold water, submerging 2/3 of the animals. The head was placed on a cushion and not immersed or covered with ice slush. As esophageal temperature fell below 24°C and serious bradycardia (< 20 beats/min) developed, circulation of cold water was discontinued, and the tub was drained of water and ice slush. Invariably, esophageal temperature subsequently dropped further, and with the onset of asystole preparations for sternotomy and attachment to CPB were started.
Cardiopulmonary Bypass
Initiating and Maintaining Cardiopulmonary Bypass
A heparin-coated CPB circuit consisting of a membrane oxygenator (Jostra Quadrox, Maquet Cardiopulmonary, Hirrlingen, Germany), a hosing system without venous reservoir and a centrifugal pump head (Bio-Medicus, Eden Prairie, Minnesota, United States) was primed with approximately 600 ml of Ringer acetate. The heart was exposed via a median sternotomy. The ascending aorta was cannulated with a 16 F arterial cannula (Jostra), and a single stage 24 F venous cannula (Medtronic, Cardiac Surgical Products, Grand Rapids, Michigan United States) was placed in the right atrium. A 12 F intracardiac catheter was positioned into the left ventricle through the apex of the heart for decompression of the left ventricle. Blood coagulability was monitored using activated clotting time (ACT) (Hemochron 801, Hemochron whole blood coagulation system, Edison, New Jersey, United States). Heparin was given to keep ACT around 200 seconds. Non-pulsatile CPB was started, and pump flow adjusted to reach a global perfusion pressure above 50 mm Hg and a central venous saturation above 60%. If these endpoints were not reached by increasing the pump head rotation speed, Ringer acetate was added to the circuit. According to α-stat management, fresh gas administration was adjusted to maintain PaO2 supranormal and PaCO2 within normal limits.
Cooling and Rewarming by Cardiopulmonary Bypass
Cooling and rewarming while on CPB were performed using the heat-exchanger attached to the oxygenator to achieve a temperature gradient of 5°C maximum between drained venous blood and inflowing arterial blood. However, during rewarming maximum temperature in the inflowing arterial line was set at 39°C and the temperature gradient between the urinary bladder and blood in the arterial line was not allowed to exceed 10°C. To ensure the heart surgery resemblance; after cooling animals in the CPBc group to18°C (in esophagus), CPB was stopped, the aorta was cross-clamped distal to the aortic cannula and a cold (4°C) crystalloid hyperkalemic cardioplegic solution (St. Thomas cardioplegic solution, 13 ml/kg) was added via the aortic cannula to produce cardiac arrest.
During rewarming internal electroconversion of ventricular fibrillation was initiated at an esophageal temperature of 25°C. If three attempts at electro-conversion were unsuccessful up to an esophageal temperature of 28°C, a bolus of 150 mg amiodarone was administered in the arterial cannula before additional electro-conversions.
Weaning from CPB was performed when the temperature measured in the urinary bladder reached 36°C, accomplished by use of dopamine (DA) infusions to keep MAP > 60 mmHg.
Biochemical Analyses
Analyses of arterial, mixed venous, and jugular vein blood gases along with hemoglobin (Hb) measurements were performed using a blood gas analyzer (Rapid lab, Chiron Diagnostics, Emeryville, CA, United States) uncorrected for temperature. Blood samples for plasma analysis were put on ice, quickly centrifuged and frozen, and kept at –80°C before analysis. Troponin T (TnT), ASAT, ALAT, and albumin were analyzed using the sandwich method of electrochemical luminescence, UV-test with pyridoxal phosphate activation, and a colorimetric end point method (Modular, Roche Diagnostics, Rotkreuz, Switzerland).
Postmortem Wet/Dry Organ Weight Ratios
A postmortem autopsy was performed, and representative samples of various organs were excised, weighed, and stored overnight in an incubator at 60°C, before weighing was repeated followed by calculation of wet/dry ratios.
Statistical Analyses
Statistical analyses were performed using SigmaPlot statistical software version 11 (Systat Software Inc. (SSI), Richmond, California, United States). Intragroup comparisons were performed by one-way repeated measures analysis of variance, followed by post hoc pairwise comparisons using the Holm-Sidak or Student-Newman-Keuls tests when appropriate. For within group single comparisons a paired sample Student’s t test was used. For comparisons between groups, a two sample Student’s t test was used if data showed normal distribution, otherwise Mann-Whitney rank sum test was used. For comparisons of survival between groups the two-sided Barnard’s unconditional test was used. Level of significance was set at p ≤ 0.05. Data are presented as mean ± standard error of mean (SEM).
Results
No statistically significant differences in any of the variables were observed in baseline measurements.
Survival
Out of eight animals in the IMMc group, four died at the time of weaning from CPB after rewarming was completed, or shortly thereafter. Death appeared to result from electro-mechanical dissociation since the heart had no measurable output in spite of electrical activity. In contrast, all animals in the CPBc group survived for the 2-hour period post CPB. Thus, intergroup difference in survival was statistically significant (p = 0.02).
Flow, Pressures, and O2 Variables
Cooling
Except from the initial reduction in global blood flow delivered by the extracorporeal circuit compared with the flow generated by spontaneous circulation, animals in CPBc group had a significantly higher blood flow below 34°C than IMMc animals (Figure 3A). However, as Hb content was significantly lower in the CPBc group after initiating CPB, and throughout cooling (Figure 4A), DO2 (Figure 4D) was significantly higher in IMMc animals until 25°C. Below 25°C, CPBc animals had significantly higher DO2 than IMMc animals. Global extraction of O2, measured by SvO2 (Figure 4B), matched variations in DO2 so that VO2 (Figure 4E) was independent of DO2. Brain O2 extraction, measured by SvjO2, followed the pattern of changes in SvO2, but the difference between groups was more profound for SvjO2 than for SvO2 at 20°C (Figure 4C).
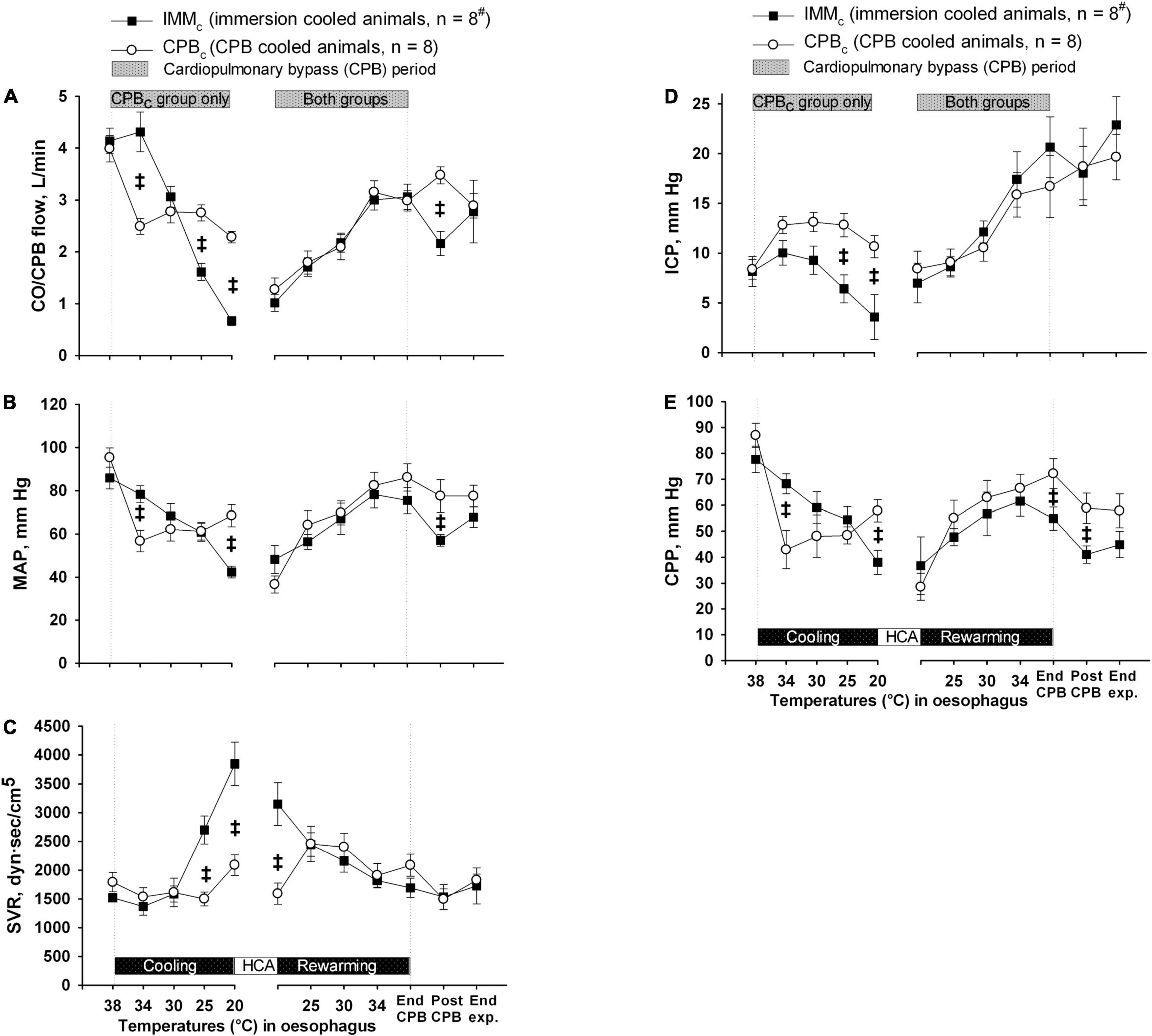
Figure 3. Blood flow and pressures. (A) Cardiac output and CPB flow (CO/CPB flow). (B) Mean arterial pressure (MAP). (C) Systemic vascular resistance (SVR). (D) Intracranial pressure (ICP). (E) Cerebral perfusion pressure (CPP). Data presented as mean and SEM. ‡Statistically significant difference between groups (p < 0.05). #n was reduced to 5 animals after termination of CPB (Post CPB) and to 4 animals at end of experiments (End exp.) in IMMc group.
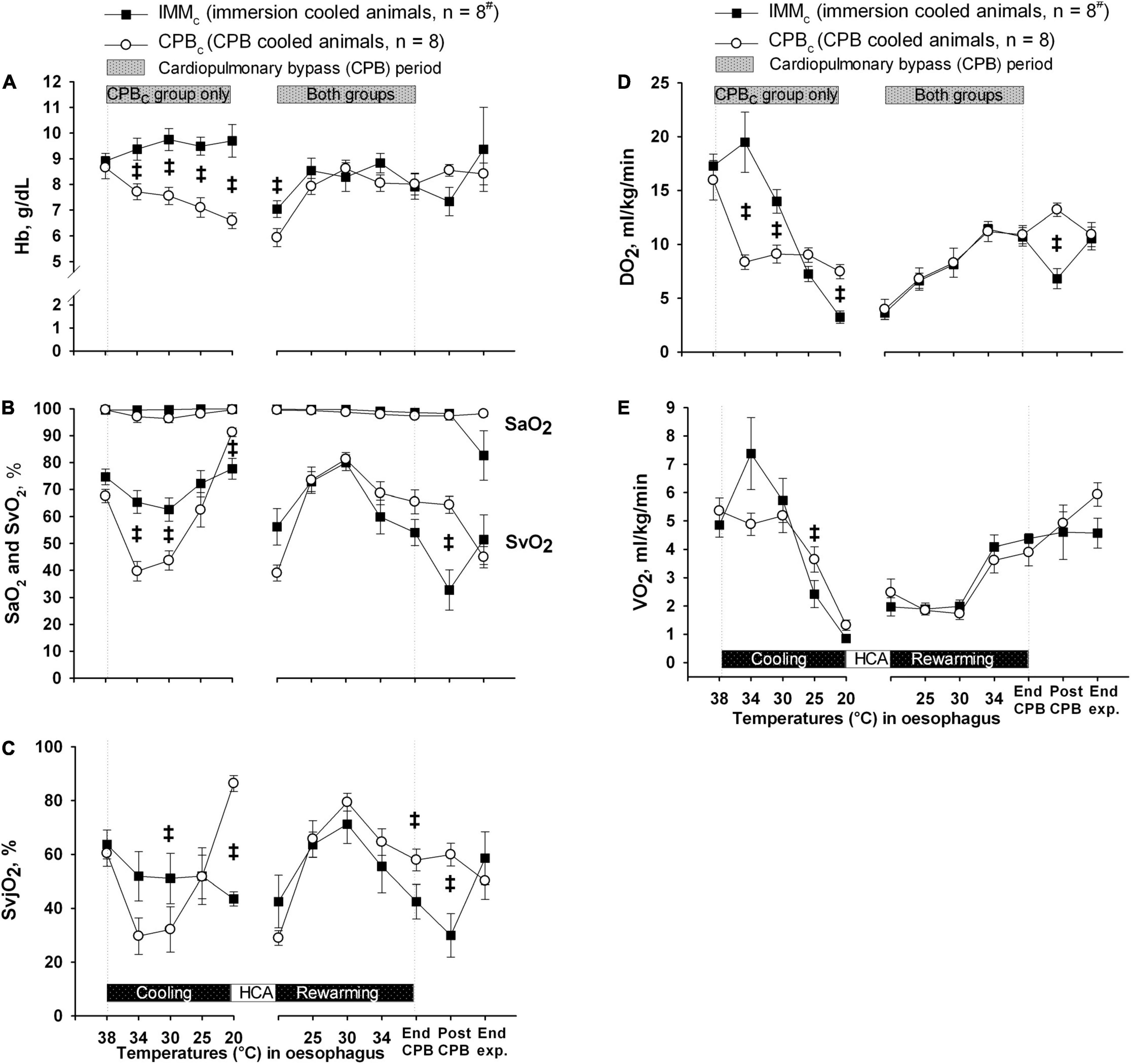
Figure 4. Oxygen variables. (A) Blood hemoglobin (Hb) concentration. (B) Arterial and mixed venous blood oxygen saturation (SaO2 and SVO2). (C) Internal jugular venous blood oxygen saturation (SvjO2). (D) Global delivery of oxygen (DO2). (E) Global consumption of oxygen (VO2). Data presented as mean and SEM. ‡Statistically significant difference between groups (p < 0.05). #n was reduced to 5 animals after termination of CPB (Post CPB) and to 4 animals at end of experiments (End exp.) in IMMc group.
Mean arterial pressure (Figure 3B) declined steadily in IMMc group, while SVR (Figure 3C) increased throughout cooling. In CPBc group MAP stabilized after an initial drop at the onset of CPB, while SVR was unaffected by cooling. Consequently, at 20°C MAP was significantly lower in IMMc group than in CPBc group, while the opposite was the case for SVR.
During cooling, ICP declined in IMMc group, while it was stable in CPBc group, resulting in significantly higher values in CPBc group at severe hypothermia (Figure 3D). However, with the decline in MAP in the IMMc group, CPP in this group was significantly lower than in the CPBc group at 20°C (Figure 3E).
Rewarming. Except for the initial increase in SVR and Hb in the IMMc group immediately after the start of rewarming by CPB, there were no intergroup differences in CPB flow, MAP, SVR, ICP, CPP, or O2 variables during rewarming. However, after completion of rewarming, just before weaning from CPB, CPP and SvjO were significantly higher in the CPBc group.
Post-hypothermic period: In order to preserve statistical power, comparisons of variables between the groups after rewarming were made immediately after weaning from CPB, when five animals in the IMMc group were still alive (Table 1). At this timepoint, animals in the IMMc group had significantly lower CO, HR, MAP, CPP, SvO2, SvjO2, and DO2 than CPB-cooled animals (Table 1). SV did not differ in between the groups. Animals in the CPBc group maintained CO at prehypothermic levels by increasing HR (Table 1). Further, CVP and average DA dose (7.4 ± 1.6 μg/kg/min in the IMMc group vs. 2.1 ± 1.2 μg/kg/min in the CPBc group) to support circulation were both significantly higher in IMMc animals (Table 1).
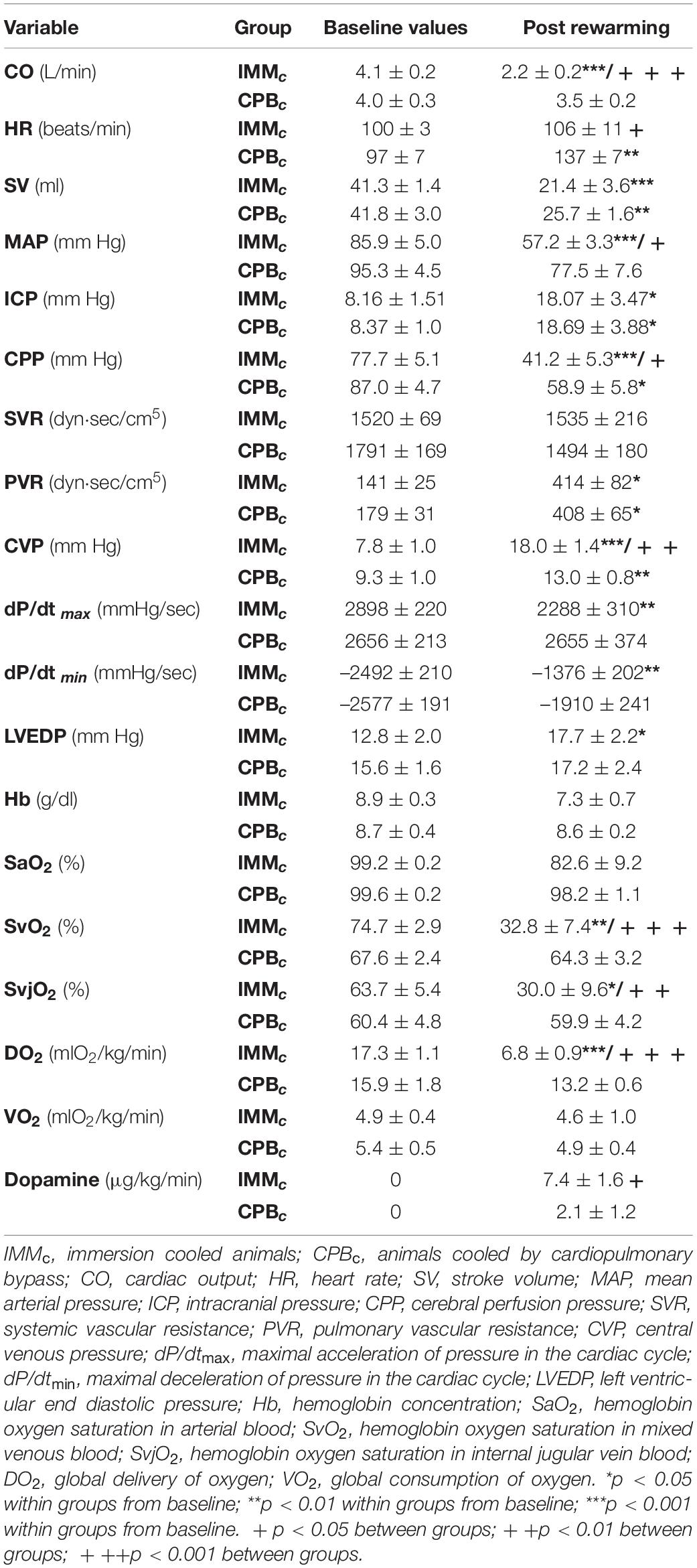
Table 1. Flow- and pressure-derived variables, oxygen variables and dopamine administration before and after cooling.
Brain Metabolism
Brain lactate and glycerol were significantly increased in both the groups at the end of experiment, while the brain glucose/lactate ratio was decreased (Figure 5). At end of experiment, brain pyruvate was significantly lower in the IMMc than in the CPBc group, while the brain lactate/pyruvate ratio was significantly depressed in the IMMc group compared to CPBc animals.
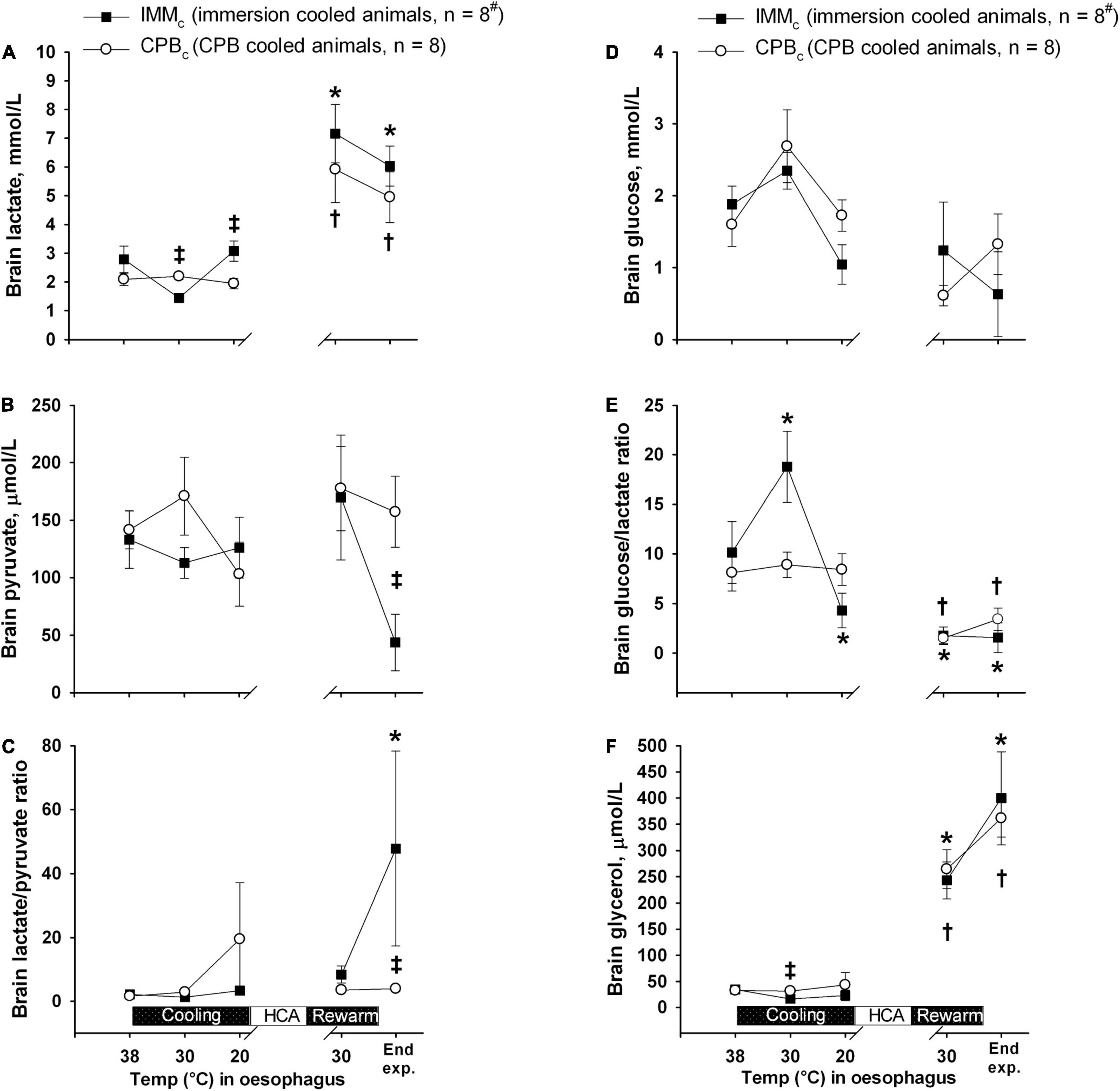
Figure 5. Brain microdialysate analyses. (A) Brain lactate. (B) Brain pyruvate. (C) Brain lactate/pyruvate ratio. (D) Brain glucose. (E) Brain glucose/lactate ratio. (F) Brain glycerol. Data presented as mean and SEM. *Statistically significant difference from baseline within IMMc group, and †within CPBc group (p < 0.05). ‡ Statistically significant difference between groups (p < 0.05). #n was reduced to 5 animals after termination of CPB (Post CPB) and to 4 animals at end of experiments (End experiment) in IMMc group.
Regional Cerebral Blood Flow
During cooling and rewarming, blood flow in forebrain, hippocampus, and cerebellum were higher in the CPBc group as compared to IMMc animals (Figure 6). Further, in the CPBc group, blood flow in forebrain and cerebellum was significantly reduced at 25°C during rewarming compared to baseline (38°C), whereas blood flow in hippocampus remained reduced after cooling to 25°C. In the IMMc group, blood flow in forebrain and cerebellum remained reduced compared to 38°C baseline values throughout the cooling and rewarming protocol, whereas blood flow in hippocampus was reduced only at 25°C rewarming.
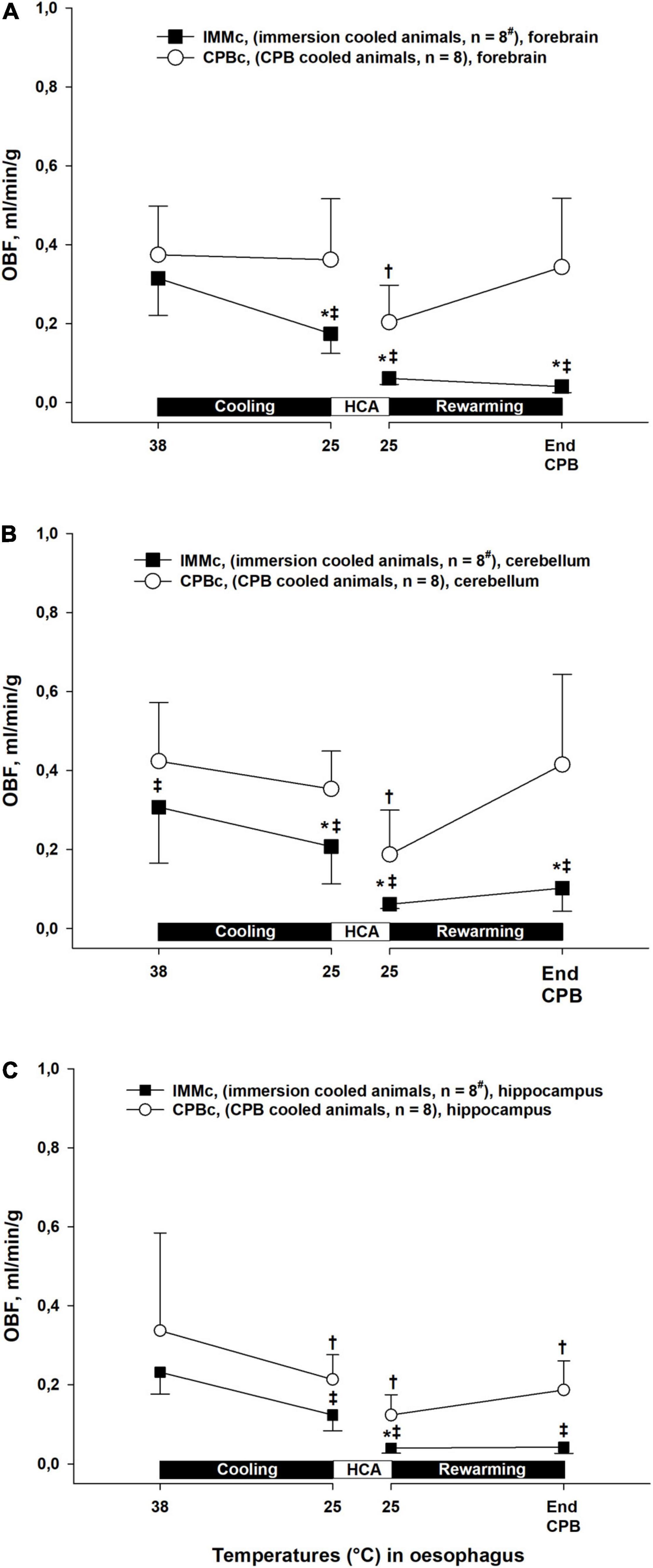
Figure 6. Cerebral blood flow. (A): forebrain, (B): cerebellum, (C): hippocampus. Data presented as mean and SEM. *Statistically significant difference from baseline within IMMc group, and †within CPBc group (p < 0.05). ‡ Statistically significant difference between groups (p < 0.05). #n was reduced to 5 animals after termination of CPB (Post CPB) and to 4 animals at end of experiments (End exp.) in IMMc group.
Biochemical Variables
Plasma albumin values decreased significantly in the CPBc group after initiating CPB during cooling, compared to both their own baseline values and IMMc animals (Figure 7). However, this difference in plasma albumin between groups was reversed during and after rewarming, with significantly higher albumin values in CPBc group by the end of experiment. A this time point, this was the only significant intergroup difference in biochemical variables.
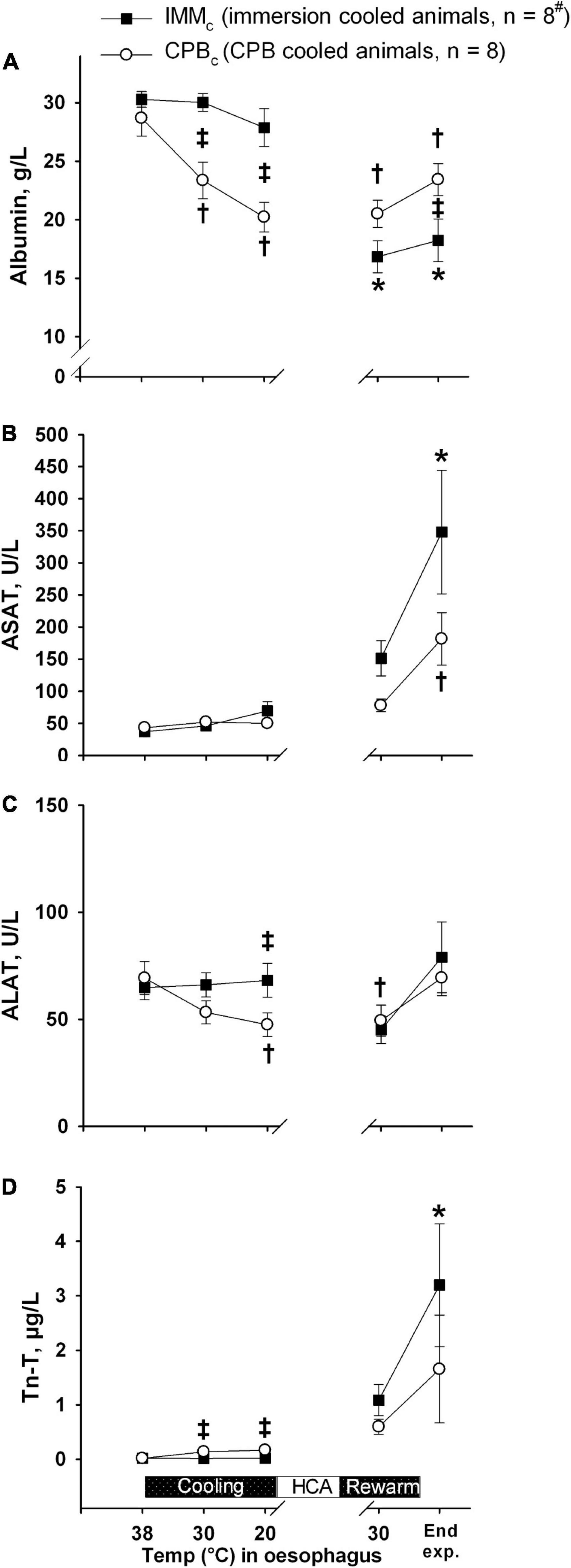
Figure 7. Biochemical analyses. (A): Albumin; (B): ASAT; (C): ALAT; (D): Troponin-T (Tn-T). Data presented as mean and SEM. *Statistically significant difference from baseline within IMMc group, and †within CPBc group (p < 0.05). ‡Statistically significant difference between groups (p < 0.05).
Fluid Administration Rates Weight Gains and Organ Weight Wet/Dry Ratios
The administration rate of fluid volume was significantly higher during cooling by CPB than by immersion cooling (Figure 8A). However, fluid administration rate in IMMc animals was significantly higher in the CPBc group during rewarming by CPB. However, no differences between groups in overall fluid administration rates and total body weight gain ratios were found. Post-mortem wet/dry organ weight ratios (Figure 8B) showed significantly increased water content in the heart and gut in the IMMc group compared to the CPBc group.
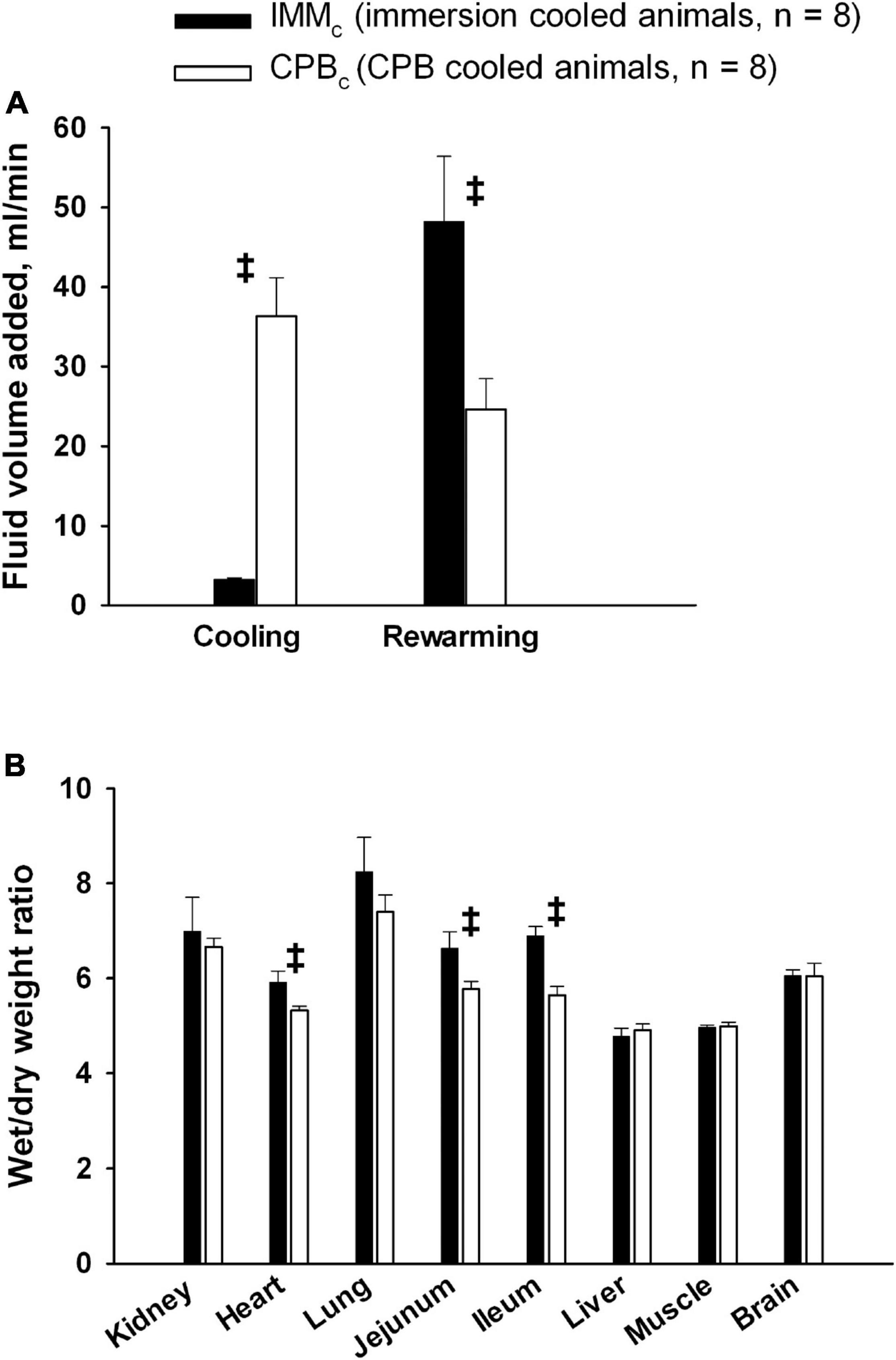
Figure 8. Fluid administration and organ edema. (A): Fluid volume administration rate. (B): Postmortem wet/dry organ weight ratio. Data presented as mean and SEM. ‡Statistically significant difference between groups (p < 0.05).
Cooling and Rewarming Times and Temperature Measurements
The total experiment times did not differ significantly between the groups (10 h, 28 ± 7 min in IMMc and 10 h, 11 ± 8 min in CPBc group (Figure 2). As expected, cooling was significantly slower in IMMc group (1 h 52 ± 10 min) compared to the CPBc group (52.8 ± 2.8 min, p < 0.001). But as considerable time was used for surgical preparation in the latter group, there was no significant time difference between groups in reaching cooling end-points, measured from baseline. Rewarming lasted significantly longer in the IMMc group (1 h, 19 ± 7 min) compared to in the CPBc group (56 ± 3 min, p = 0.007). Total CPB time was longer in the CPBc group (1 h 48 ± 5 min) when compared to rewarming time only in IMMc animals (p = 0.005). Figure 1A shows that temperatures in different organs (esophagus, urinary bladder, pulmonary artery, and brain) were relatively consistent in the IMMc group during cooling, while temperature in the urinary bladder lagged behind during the much more efficient extracorporeal cooling in the CPBc group (Figure 1B). Surviving IMMc animals did not manage to preserve body heat after weaning from CPB, resulting in significantly lower temperatures than in the CPBc group at end of experiments (Figures 2A,B).
Discussion
The results of the present study show that after rewarming, IMMc animals suffered from severe cardiovascular failure at weaning from CPB, leading to imminent or premature death in 4 out of 8 animals. In contrast, all animals in the CPBc group survived the 2-hour post CPB rewarming period. This finding underlines the well-recognized differences in success rate from CPB rewarming in accidental hypothermia compared to conventional CPB rewarming after cardiac surgery.
Animals in both groups were subjected to 75 minutes of global hypothermic ischemia, but post-hypothermic perturbation of cardiovascular function was far more severe in IMMc animals. The underlying pathophysiology may reflect hypothermic and/or ischemic damage during immersion cooling prior to HCA. In the pioneering work of Bigelow and colleagues, a significant reduction in CO was reported in dogs upon rewarming after cooling to HCA (Bigelow et al., 1950). These results were consistent with other studies showing cardiac dysfunction upon rewarming after cooling but without cardiac arrest (Prec et al., 1949; Ross, 1954; Blair et al., 1956; Fedor et al., 1958; Popovic and Kent, 1965; Steen et al., 1980; Tveita et al., 1994, 1996). The introduction of CPB and cardioplegic agents to achieve diastolic cardiac arrest before cardiac surgery (also applied during HCA in the actual CPBc group) seemed to alleviate post-hypothermic cardiac dysfunction (Taufic and Lewis, 1953). The pathophysiology of post-operative cardiac dysfunction is considered to be related to the preceding period of ischemia-reperfusion during surgery and not to the hypothermic exposure per se (Toller and Metzler, 2005). However, post-hypothermic cardiac dysfunction remains a clinical challenge related to rewarming from accidental hypothermia.
Inspired by these original research works (Prec et al., 1949; Bigelow et al., 1950, 1954; Fairley et al., 1957) our group has conducted experimental hypothermia/rewarming studies by use of intact animal models (Tveita et al., 1991a,b, 1993, 1994, 1996, 1998a,b, 1999) and in vitro heart muscle tissue (Han et al., 2010, 2018). Results from our studies are consistent with their findings of reduced myocardial function after rewarming (Filseth et al., 2010, 2012). Our results have consistently revealed that the hypothermia and rewarming cause direct effects on myocardial excitation-contraction coupling and actin-myosin interaction (Tveita et al., 1998b; Han et al., 2008; Filseth et al., 2010). These effects are most likely to explain the depression in left ventricular contractile function after rewarming, which underlies the reduction of cardiac output and systemic arterial pressure (Tveita, 2000). Impaired Ca2+ control is a key factor in the pathophysiology of heart failure in normothermia (Vassalle and Lin, 2004). Also, hypothermia/rewarming appears to disturb the mechanisms underlying excitation-contraction coupling in cardiac muscle force generation, and over time Ca2+ overload occurs (Tani and Neely, 1989; Vassalle and Lin, 2004), which results in mechanical dysfunction that may entail acute cardiac dysfunction. However, during rewarming in isolated cardiac myocytes the amplitude and duration of the evoked [Ca2+]cyt transient returns to normothermic levels (Schaible et al., 2016). Thus, it does not appear that the decrease in contractility of cardiac myocytes (heart failure) after rewarming can be solely attributed to dysregulation of [Ca2+]cyt release and reuptake. By the use of isolated, electrically stimulated myocytes cooled to 15°C, and rewarmed we have documented that the reduction in force generation, independent of [Ca2+]cyt levels, is the effect of an increased phosphorylation of cardiac troponin I (cTnI) leading to reduced Ca2+ sensitivity of the contractile response after rewarming (Han et al., 2010; Schaible et al., 2016, 2018). Further, with relevance to the group (CPBc) treated with a chemical cardioplegic solution during HCA in the present study; we observed both maintained cytosolic Ca2+ levels and myofilament Ca2+ sensitivity in myocytes, which were not electrically stimulated (cardioplegic) during cooling to 15°C and rewarming (Han et al., 2018).
The increase in O2 extraction in the IMMc versus the CPBc group compensated for the lower CO in this group and made global VO2 similar in the two groups. However, in IMMc animals below 25°C, the more than doubled SVR, combined with reduced MAP, may have contributed to a compromised regional tissue perfusion.
It was also noted that animals in the IMMc group that could not be weaned from CPB had a contracted, non-beating heart, similar to a “stone heart”. Before cardioplegic solutions and hypothermic bypass were introduced during the 1970’s, this clinical phenomenon, later linked to cytosolic Ca2+ accumulation (Jimenez et al., 1990), was a feared complication to ischemic cardiac arrest during heart surgery (Cooley et al., 1972). In the present study, the irreversible contracture developed in half of the IMMc animals during rewarming. If one should translate to the clinical situation, patients displaying a severe post-hypothermic cardiovascular failure should be supported by prolonged ECMO. Based on promising clinical reports (Ruttmann et al., 2007) the use of ECMO for rewarming from accidental hypothermia has been recommended as ECMO can also be continued after rewarming for cardio/respiratory support for days, if needed (Ruttmann et al., 2007; Morita et al., 2011). Recently, we have demonstrated that by the use of the present model that rewarming with ECMO restored blood flow to the heart and brain and created a “shockable” cardiac rhythm after 3 h of continuous CPR at 27°C (Nilsen et al., 2021).
It has been documented that a MAP as low as 40 mmHg during hypothermic CPB may lead to cerebral ischemia, indicated, among other variables, by increased levels of lactate and lactate/pyruvate ratio in brain microdialysate fluid (Haugen et al., 2006). Our finding of significantly increased brain lactate in IMMc animals compared to CPBc animals at the onset of HCA was preceded by significant drops in both CPP and SvjO2. Together with a significantly increased post-hypothermic brain lactate/pyruvate ratio in IMMc compared to CPBc animals, this may indicate that the brain suffered ischemic injury during spontaneous circulation below 25°C. The last conclusion finds support in the cerebral blood flow data in IMMc animals versus CBFc at 25°C showing significantly lower flow in IMMc in all three parts of the brain investigated: forebrain, hippocampus, and cerebellum. These differences in cerebral blood flow between groups remained throughout the intervention protocol, and possible mechanisms to explain these differences in blood flow may be found in other preclinical studies conducted during the early attempts to establish transplantation medicine. They demonstrated that the application of cold per se, by simply cooling an organ, is not sufficient to provide good preservation of donor organs. Follow up studies showed that hypothermia was associated with an increase in blood viscosity that contributes to red cell sludging in the microvasculature as well as detrimental coagulopathies (Dewall et al., 1962; Johansson and Huttunen, 1963; Suzuki and Penn, 1965; Michenfelder and Theye, 1970; Baumgartner et al., 1983). Other experiments showed increased survival during profound hypothermic CPB combined with exsanguination and substitution with acellular solutions, and successful rewarming with auto-transfusion after prolonged hypothermia (Taylor et al., 1995).
Further, extravasation of plasma fluid has been documented to take place during normothermic CPB, but this extravasation increases significantly during hypothermic CPB (Heltne et al., 2001; Farstad et al., 2003). Extravasation may also occur in surface cooling with maintained circulation, but obviously to a lesser extent (Hammersborg et al., 2005). Per protocol more fluid volume was added to the extracorporeal circuit in the IMMc group than in the CPBc group during rewarming (Figure 3A). Therefore, one could speculate that capillary leak was greater during CPB rewarming in IMMc animals.
Limitations
The combined hypothermic and ischemic injury inflicted to the animals in the IMMc group that resulted in immediate or delayed death in 4 out of 8 animals after rewarming and before the end of experiment, clearly affected power of post-hypothermic statistics. Furthermore, because only the fittest animals in IMMc group survived to the end, results in the post-hypothermic period may have been biased.
Conclusion
The translational value of this experiment is in order to create successful CPB-rewarming with favorable neurologic outcome in victims of accidental hypothermia, the CPB strategies need to differ from those applied in conventional cardiac surgery. Above all, the need for prolonged ECMO should be considered in all victims of HCA that cannot be weaned from CPB immediately after rewarming.
Data Availability Statement
The raw data supporting the conclusions of this article will be made available by the authors, without undue reservation.
Ethics Statement
The animal study was reviewed and approved by the Norwegian Animal Research Authority.
Author Contributions
OF conducted the experiments and, together with TT and GS, designed the protocol, discussed the data, and wrote the manuscript. SH took part in carrying out experiments and contributed to the writing process. TK took part in carrying out experiments and processing and interpretation of data. All authors contributed to the article and approved the submitted version.
Funding
This research project was supported by grants from the Norwegian Council on Cardiovascular Diseases, the Norwegian Society of Anesthesiology, the Norwegian Research Council (Petromax2), and the Norwegian Ministry of Foreign Affairs (Barents 2020).
Conflict of Interest
The authors declare that the research was conducted in the absence of any commercial or financial relationships that could be construed as a potential conflict of interest.
Publisher’s Note
All claims expressed in this article are solely those of the authors and do not necessarily represent those of their affiliated organizations, or those of the publisher, the editors and the reviewers. Any product that may be evaluated in this article, or claim that may be made by its manufacturer, is not guaranteed or endorsed by the publisher.
Acknowledgments
We highly appreciate the technical support from Knut Steinnes in sampling and analysis of hemodynamic data and the assistance of Hege Hagerup, Harry Jensen, Janne Andreassen, and Victoria Steinsund in carrying out experiments and collection and processing of blood samples.
References
Bakaeen, F. G., Chu, D., Huh, J., LeMaire, S. A., Cornwell, L., Sansgiry, S., et al. (2010). Outcomes of circulatory arrest procedures for the treatment of thoracic aortic disease at a veterans facility. Am. J. Surg. 200, 581–584. doi: 10.1016/j.amjsurg.2010.07.010
Baumgartner, W. A., Silverberg, G. D., Ream, A. K., Jamieson, S. W., Tarabek, J., and Reitz, B. A. (1983). Reappraisal of cardiopulmonary bypass with deep hypothermia and circulatory arrest for complex neurosurgical operations. Surgery 94, 242–249.
Bigelow, W. G., Lindsay, W. K., and Greenwood, W. F. (1950). Hypothermia. Its possible role in cardiac surgery: An investigation of factors governing survival in dogs at low body temperatures. Ann. Surg. 132, 849–864. doi: 10.1097/00000658-195011000-00001
Bigelow, W. G., Mustard, W. T., and Evans, J. G. (1954). Some physiologic consepts of hypothermia and their applications to cardiac surgery. J. thorac. Surg. 28, 463–480. doi: 10.1016/s0096-5588(20)30959-4
Blair, E., Montgomery, A. V., and Swan, H. (1956). Posthypothermic circulatory failure I. Physiologic observations on the circulation. Circulation 13, 909–915. doi: 10.1161/01.cir.13.6.909
Boyd, J., Brugger, H., and Shuster, M. (2010). Prognostic factors in avalanche resuscitation: a systematic review. Resuscitation 81, 645–652. doi: 10.1016/j.resuscitation.2010.01.037
Cooley, D. A., Reul, G. J., and Wukasch, D. C. (1972). Ischemic contracture of the heart: “stone heart”. Am. J. Cardiol. 29, 575–577. doi: 10.1016/0002-9149(72)90454-7
Dewall, R. A., Lillehei, R. C., and Sellers, R. D. (1962). Hemodilution perfusions for open-heart surgery. Use of five per cent dextrose in water for the priming volume. N. Engl. J. Med. 266, 1078–1084. doi: 10.1056/NEJM196205242662103
Fairley, H. B., Waddell, W. G., and Bigelow, W. G. (1957). Hypothermia for cardiovascular surgery: Acidosis in the rewarming period. Br. J. Anaesth. 29, 310–318. doi: 10.1093/bja/29.7.310
Farstad, M., Andersen, K. S., Koller, M. E., Grong, K., Segadal, L., and Husby, P. (2001). Rewarming from accidental hypothermia by extracorporeal circulation. A retrospective study. Eur. J. Cardiothorac. Surg. 20, 58–64. doi: 10.1016/s1010-7940(01)00713-8
Farstad, M., Heltne, J. K., Rynning, S. E., Lund, T., Mongstad, A., Eliassen, F., et al. (2003). Fluid extravasation during cardiopulmonary bypass in piglets–effects of hypothermia and different cooling protocols. Acta Anaesthesiol. Scand. 47, 397–406. doi: 10.1034/j.1399-6576.2003.00103.x
Fedor, E. J., Fisher, B., and Lee, S. H. (1958). Rewarming following hypothermia of two to twelve hours. Ann. Surg. 147, 515–530. doi: 10.1097/00000658-195804000-00007
Fehrenbacher, J. W., Siderys, H., Terry, C., Kuhn, J., and Corvera, J. S. (2010). Early and late results of descending thoracic and thoracoabdominal aortic aneurysm open repair with deep hypothermia and circulatory arrest. J. Thorac. Cardiovasc. Surg. 140, S154–S160. discussion S185-S190. doi: 10.1016/j.jtcvs.2010.08.054
Filseth, O. M., How, O. J., Kondratiev, T., Gamst, T. M., Sager, G., and Tveita, T. (2012). Changes in cardiovascular effects of dopamine in response to graded hypothermia in vivo. Crit. Care Med. 40, 178–186. doi: 10.1097/CCM.0b013e31822d78de
Filseth, O. M., How, O. J., Kondratiev, T., Gamst, T. M., and Tveita, T. (2010). Post-hypothermic cardiac left ventricular systolic dysfunction after rewarming in an intact pig model. Crit. Care 14:R211. doi: 10.1186/cc9334
Gilbert, M., Busund, R., Skagseth, A., Nilsen, P. A., and Solbo, J. P. (2000). Resuscitation from accidental hypothermia of 13.7 degrees C with circulatory arrest. Lancet 355, 375–376. doi: 10.1016/S0140-6736(00)01021-7
Hammersborg, S. M., Farstad, M., Haugen, O., Kvalheim, V., Onarheim, H., and Husby, P. (2005). Time course variations of haemodynamics, plasma volume and microvascular fluid exchange following surface cooling: an experimental approach to accidental hypothermia. Resuscitation 65, 211–219. doi: 10.1016/j.resuscitation.2004.11.020
Han, Y. S., Schaible, N., Tveita, T., and Sieck, G. (2018). Discontinued stimulation of cardiomyocytes provides protection against hypothermia-rewarming-induced disruption of excitation-contraction coupling. Exp. Physiol. 103, 819–826. doi: 10.1113/EP086774
Han, Y. S., Tveita, T., Kondratiev, T. V., Prakash, Y. S., and Sieck, G. C. (2008). Changes in cardiovascular beta-adrenoceptor responses during hypothermia. Cryobiology 57, 246–250. doi: 10.1016/j.cryobiol.2008.09.006
Han, Y. S., Tveita, T., Prakash, Y. S., and Sieck, G. C. (2010). Mechanisms underlying hypothermia-induced cardiac contractile dysfunction. Am. J. Physiol Heart Circ. Physiol. 298, H890–H897. doi: 10.1152/ajpheart.00805.2009
Haugen, O., Farstad, M., Lise, K. V., Rynning, S. E., Hammersborg, S., Mongstad, A., et al. (2006). Mean arterial pressure about 40 mmHg during CPB is associated with cerebral ischemia in piglets. Scand. Cardiovasc. J. 40, 54–61. doi: 10.1080/14017430500365185
Heltne, J. K., Koller, M. E., Lund, T., Farstad, M., Rynning, S. E., Bert, J. L., et al. (2001). Studies on fluid extravasation related to induced hypothermia during cardiopulmonary bypass in piglets. Acta Anaesthesiol. Scand. 45, 720–728. doi: 10.1034/j.1399-6576.2001.045006720.x
Jimenez, E., del Nido, P., Sarin, M., Nakamura, H., Feinberg, H., and Levitsky, S. (1990). Effects of low extracellular calcium on cytosolic calcium and ischemic contracture. J. Surg. Res. 49, 252–255. doi: 10.1016/0022-4804(90)90129-p
Johansson, B. W., and Huttunen, M. (1963). Influence of Dextran of Low and High Molecular Weight on the Electrocardiogram of Hypothermic Dogs. Am. J. Cardiol. 12, 792–794. doi: 10.1016/0002-9149(63)90282-0
Kondratiev, T. V., Wold, R. M., Aasum, E., and Tveita, T. (2008). Myocardial mechanical dysfunction and calcium overload following rewarming from experimental hypothermia in vivo. Cryobiology 56, 15–21. doi: 10.1016/j.cryobiol.2007.09.005
Kornberger, E., and Mair, P. (1996). Important aspects in the treatment of severe accidental hypothermia: the Innsbruck experience. J. Neurosurg. Anesthesiol. 8, 83–87. doi: 10.1097/00008506-199601000-00027
Locher, T., Walpoth, B., Pfluger, D., and Althaus, U. (1991). [Accidental hypothermia in Switzerland (1980-1987)–case reports and prognostic factors]. Schweiz Med. Wochenschr. 121, 1020–1028.
Michenfelder, J. D., and Theye, R. A. (1970). The effects of anesthesia and hypothermia on canine cerebral ATP and lactate during anoxia produced by decapitation. Anesthesiology 33, 430–439. doi: 10.1097/00000542-197010000-00013
Morita, S., Inokuchi, S., Yamagiwa, T., Iizuka, S., Yamamoto, R., Aoki, H., et al. (2011). Efficacy of portable and percutaneous cardiopulmonary bypass rewarming versus that of conventional internal rewarming for patients with accidental deep hypothermia. Crit. Care Med. 39, 1064–1068. doi: 10.1097/CCM.0b013e31820edd04
Nilsen, J. H., Schanche, T., Valkov, S., Mohyuddin, R., Haaheim, B., Kondratiev, T. V., et al. (2021). Effects of rewarming with extracorporeal membrane oxygenation to restore oxygen transport and organ blood flow after hypothermic cardiac arrest in a porcine model. Sci. Rep. 11:18918. doi: 10.1038/s41598-021-98044-2
Oberhammer, R., Beikircher, W., Hormann, C., Lorenz, I., Pycha, R., Adler-Kastner, L., et al. (2008). Full recovery of an avalanche victim with profound hypothermia and prolonged cardiac arrest treated by extracorporeal re-warming. Resuscitation 76, 474–480. doi: 10.1016/j.resuscitation.2007.09.004
Paal, P., Brugger, H., and Boyd, J. (2013). Accidental hypothermia. N. Engl. J. Med. 368:682. doi: 10.1056/NEJMc1215158
Paal, P., Gordon, L., Strapazzon, G., Brodmann, M. M., Putzer, G., Walpoth, B., et al. (2016). Accidental hypothermia-an update : The content of this review is endorsed by the International Commission for Mountain Emergency Medicine (ICAR MEDCOM). Scand. J. Trauma Resusc. Emerg. Med 24:111. doi: 10.1186/s13049-016-0303-7
Paal, P., Pasquier, M., Darocha, T., Lechner, R., Kosinski, S., Wallner, B., et al. (2022). Accidental Hypothermia: 2021 Update. Int. J. Environ. Res. Public Health 19:1. doi: 10.3390/ijerph19010501
Popovic, V., and Popovic, P. (1974). Hypothermia in biology and in medicine. New York: Grune & Stratton, Inc.
Popovic, V. P., and Kent, K. M. (1965). Cardiovascular responses in prolonged hypothermia. Am. J. Physiol. 209, 1069–1074. doi: 10.1152/ajplegacy.1965.209.6.1069
Prec, O. R., Rosenman, K., Rodbard, S., and Katz, L. N. (1949). The cardiovascular effects of acutely induced hypothermia. J. Clin Invest. 28, 293–297. doi: 10.1172/jci102071
Raab, S., Thein, E., Harris, A. G., and Messmer, K. (1999). A new sample-processing unit for the fluorescent microsphere method. Am. J. Physiol. 276, H1801–H1806. doi: 10.1152/ajpheart.1999.276.5.H1801
Ross, D. N. (1954). Hypothermia. 2. Physiological observations during hypothermia. Guys. Hosp. Rep. 103, 116–138.
Ruttmann, E., Weissenbacher, A., Ulmer, H., Muller, L., Hofer, D., Kilo, J., et al. (2007). Prolonged extracorporeal membrane oxygenation-assisted support provides improved survival in hypothermic patients with cardiocirculatory arrest. J. Thorac. Cardiovasc. Surg. 134, 594–600. doi: 10.1016/j.jtcvs.2007.03.049
Schaible, N., Han, Y. S., Hoang, T., Arteaga, G., Tveita, T., and Sieck, G. (2016). Hypothermia/rewarming disrupts excitation-contraction coupling in cardiomyocytes. Am. J. Physiol. Heart Circ. Physiol. 310, H1533–H1540. doi: 10.1152/ajpheart.00840.2015
Schaible, N., Han, Y. S., Tveita, T., and Sieck, G. C. (2018). Role of superoxide ion formation in hypothermia/rewarming induced contractile dysfunction in cardiomyocytes. Cryobiology 81, 57–64. doi: 10.1016/j.cryobiol.2018.02.010
Schultz, J. M., Karamlou, T., Swanson, J., Shen, I., and Ungerleider, R. M. (2006). Hypothermic low-flow cardiopulmonary bypass impairs pulmonary and right ventricular function more than circulatory arrest. Ann. Thorac. Surg. 81, 474–480. discussion 480. doi: 10.1016/j.athoracsur.2005.06.041
Steen, P. A., Milde, J. H., and Michenfelder, J. D. (1980). The detrimental effects of prolonged hypothermia and rewarming in the dog. Anesthesiology 52, 224–230. doi: 10.1097/00000542-198003000-00007
Suzuki, M., and Penn, I. (1965). A reappraisal of the microcirculation during general hypothermia. Surgery 58, 1049–1060.
Tani, M., and Neely, J. R. (1989). Role of intracellular Na+ in Ca2+ overload and depressed recovery of ventricular function of reperfused ischemic rat hearts. Possible involvement of H+-Na+ and Na+-Ca2+ exchange. Circ. Res. 65, 1045–1056. doi: 10.1161/01.res.65.4.1045
Tassani, P., Barankay, A., Haas, F., Paek, S. U., Heilmaier, M., Hess, J., et al. (2002). Cardiac surgery with deep hypothermic circulatory arrest produces less systemic inflammatory response than low-flow cardiopulmonary bypass in newborns. J. Thorac. Cardiovasc. Surg. 123, 648–654. doi: 10.1067/mtc.2002.121285
Taufic, M., and Lewis, F. J. (1953). A device for the experimental creation of ventricular septal defects; preliminary report. J. Thorac. Surg. 25, 413–416. doi: 10.1016/s0096-5588(20)30871-0
Taylor, M. J., Bailes, J. E., Elrifai, A. M., Shih, S. R., Teeple, E., Leavitt, M. L., et al. (1995). A new solution for life without blood. Asanguineous low-flow perfusion of a whole-body perfusate during 3 hours of cardiac arrest and profound hypothermia. Circulation 91, 431–444. doi: 10.1161/01.cir.91.2.431
Thoresen, M., Satas, S., Loberg, E. M., Whitelaw, A., Acolet, D., Lindgren, C., et al. (2001). Twenty-four hours of mild hypothermia in unsedated newborn pigs starting after a severe global hypoxic-ischemic insult is not neuroprotective. Pediatr. Res. 50, 405–411. doi: 10.1203/00006450-200109000-00017
Toller, W. G., and Metzler, H. (2005). Acute perioperative heart failure. Curr. Opin. Anaesthesiol. 18, 129–135. doi: 10.1097/01.aco.0000162830.59023.50
Tveita, T. (2000). Rewarming from hypothermia. Newer aspects on the pathophysiology of rewarming shock. Int. J. Circumpolar. Health 59, 260–266.
Tveita, T., Hevroy, O., Refsum, H., and Ytrehus, K. (1999). Coronary endothelium-derived vasodilation during cooling and rewarming of the in situ heart. Can. J Physiol. Pharmacol. 77, 56–63. doi: 10.1139/cjpp-77-1-56
Tveita, T., Mortensen, E., Hevrøy, O., Refsum, H., and Ytrehus, K. (1994). Experimental hypothermia: Effects of core cooling and rewarming on hemodynamics, coronary blood flow and myocardial metabolism in dogs. Anesth. Analg. 79, 212–218. doi: 10.1213/00000539-199408000-00002
Tveita, T., Mortensen, E., Hevroy, O., Ytrehus, K., and Refsum, H. (1991a). Hemodynamic and metabolic effects of hypothermia and rewarming. Arctic Med. Res. 50, 48–52.
Tveita, T., Ytrehus, K., Myklebust, R., and Refsum, H. (1991b). Changes in myocardial ultrastructure and mechanical performance after rewarming from prolonged hypothermia in rats. J. Mol. Cell. Cardiol. 23:103. doi: 10.1016/s0300-9572(03)00102-3
Tveita, T., Myklebust, R., and Ytrehus, K. (1998a). Changes in myocardial ultrastructure induced by cooling as well as rewarming. Res. Exp. Med. 197, 243–254. doi: 10.1007/s004330050073
Tveita, T., Ytrehus, K., Myhre, E. S., and Hevroy, O. (1998b). Left ventricular dysfunction following rewarming from experimental hypothermia. J. Appl. Physiol. 85, 2135–2139. doi: 10.1152/jappl.1998.85.6.2135
Tveita, T., Skandfer, M., Refsum, H., and Ytrehus, K. (1996). Experimental hypothermia and rewarming: changes in mechanical function and metabolism of rat hearts. J. Appl. Physiol. 80, 291–297. doi: 10.1152/jappl.1996.80.1.291
Tveita, T., Ytrehus, K., Skandfer, M., Øian, P., and Larsen, T. S. (1993). Organ blood flow and transcapillary fluid balance after rewarming from prolonged hypothermia in rat. FASEB J. 7:2552.
Vanden Hoek, T. L., Morrison, L. J., Shuster, M., Donnino, M., Sinz, E., Lavonas, E. J., et al. (2010). Part 12: cardiac arrest in special situations: 2010 American Heart Association Guidelines for Cardiopulmonary Resuscitation and Emergency Cardiovascular Care. Circulation 122, S829–S861. doi: 10.1161/CIRCULATIONAHA.110.971069
Vassal, T., Benoit-Gonin, B., Carrat, F., Guidet, B., Maury, E., and Offenstadt, G. (2001). Severe accidental hypothermia treated in an ICU: prognosis and outcome. Chest 120, 1998–2003. doi: 10.1378/chest.120.6.1998
Vassalle, M., and Lin, C. I. (2004). Calcium overload and cardiac function. J. Biomed. Sci. 11, 542–565. doi: 10.1007/BF02256119
Keywords: hypothermia induced, hypothermia accidental, cardiopulmonary bypass, immersion cooling, rewarming, cardiac index
Citation: Filseth OM, Hermansen SE, Kondratiev T, Sieck GC and Tveita T (2022) Cooling to Hypothermic Circulatory Arrest by Immersion vs. Cardiopulmonary Bypass (CPB): Worse Outcome After Rewarming in Immersion Cooled Pigs. Front. Physiol. 13:862729. doi: 10.3389/fphys.2022.862729
Received: 02 February 2022; Accepted: 25 February 2022;
Published: 31 March 2022.
Edited by:
Nephtali Marina, University College London, United KingdomReviewed by:
Wolfgang Weihs, Medical University of Vienna, AustriaPaul Philipp Heinisch, Congenital and Pediatric Heart Surgery, German Heart, Germany
Copyright © 2022 Filseth, Hermansen, Kondratiev, Sieck and Tveita. This is an open-access article distributed under the terms of the Creative Commons Attribution License (CC BY). The use, distribution or reproduction in other forums is permitted, provided the original author(s) and the copyright owner(s) are credited and that the original publication in this journal is cited, in accordance with accepted academic practice. No use, distribution or reproduction is permitted which does not comply with these terms.
*Correspondence: Torkjel Tveita, dG9ya2plbC50dmVpdGFAdWl0Lm5v