- 1Department of Orthodontics, School and Hospital of Stomatology, Cheeloo College of Medicine, Shandong University & Shandong Key Laboratory of Oral Tissue Regeneration & Shandong Engineering Laboratory for Dental Materials and Oral Tissue Regeneration, Jinan, China
- 2School of Stomatology, Heilongjiang Key Lab of Oral Biomedicine Materials and Clinical Application & Experimental Center for Stomatology Engineering, Jiamusi University, Jiamusi, China
- 3Department of General Surgery, The First Affiliated Hospital of Xi’an Medical University, Xi’an, China
- 4School of Basic Medicine, Heilongjiang Key Lab of Oral Biomedicine Materials and Clinical Application & Experimental Center for Stomatology Engineering, Jiamusi University, Jiamusi, China
- 5Department of Oral Implants, School of Stomatology, National Clinical Research Center for Oral Diseases & State Key Laboratory of Military Stomatology & Shaanxi Key Laboratory of Stomatology, The Fourth Military Medical University, Xi’an, China
- 6Key Laboratory of Shaanxi Province for Craniofacial Precision Medicine Research, College of Stomatology, Xi’an Jiaotong University, Xi’an, China
Temporomandibular joint osteoarthritis (TMJOA) is a common degenerative joint disease that can cause severe pain and dysfunction. It has a serious impact on the quality of lives of patients. Since mechanism underlying the pathogenesis of TMJOA is not fully understood, the development of effective tools for early diagnosis and disease-modifying therapies has been hindered. Animal models play a key role in understanding the pathological process of diseases and evaluating new therapeutic interventions. Although some similarities in disease processes between animals and humans are known, no one animal model is sufficient for studying all characteristics of TMJOA, as each model has different translatability to human clinical conditions. For the past 4 decades, TMJOA animal models have been studied by numerous researchers and can be broadly divided into induced, naturally occurring, and genetically modified models. The induced models can be divided into invasive models (intra-articular injection and surgical induction) or non-invasive models (mechanical loading, high-fat diet, and sleep deprivation). Different types of animal models simulate different pathological expressions of TMJOA and have their unique characteristics. Currently, mice, rats, and rabbits are commonly used in the study of TMJOA. This review sought to provide a general description of current experimental models of TMJOA and assist researchers in selecting the most appropriate models for different kinds of research.
Introduction
Osteoarthritis (OA) is a chronic degenerative condition that often affects the stress-bearing joints, such as the knee, spine, hips, and fingers (Kloppenburg and Berenbaum, 2020). The temporomandibular joint (TMJ), one of the most common and complex joints in the human body, can also be affected by OA. Temporomandibular joint osteoarthritis (TMJOA) is the most common form of arthritis occurring in TMJs due to its high clinical prevalence and consequences on TMJ (Wang et al., 2015). Patients with TMJOA usually have joint pain, swelling and stiffness that leads to activity limitations and even reduced quality of life. Therefore, numerous studies are needed to better understand the development and progression of TMJOA.
The etiology of TMJOA is complex and multifactorial, which is generally considered to be associated with mechanical overloading, abnormal occlusion, trauma, and psychological stress (Tanaka et al., 2008; de Souza et al., 2012). However, the causes of impaired cartilage and subchondral bone of TMJ remain unclear. Currently, the treatments of TMJOA mainly aim to reduce pain, restore TMJ function, and improve the quality of life of patients (Tanaka et al., 2008; Al-Moraissi et al., 2020). Although many clinical studies have investigated the effect of various treatments, no clinically approved therapeutics are currently available to restore the TMJ structure, given the limited understanding of its pathogenesis and the limited blood supply of the cartilage (Huey et al., 2012; Wang et al., 2015). Since obtaining clinical samples from patients with TMJOA is difficult and clinical symptoms often occur late in the disease process, animal models of TMJOA play a key role in understanding the pathogenesis of diseases and evaluating new therapeutic approaches (Vapniarsky et al., 2018; Liu et al., 2021). As various animal models of TMJOA have been developed over the past four decades, a major challenge lies in selecting the “best” model when designing a study. Animal models for TMD research and mouse genetic models for TMJ preclinical research have been reviewed elsewhere (Suzuki and Iwata, 2016; Ghassemi Nejad et al., 2017; Almarza et al., 2018; Bhatti et al., 2021; Xiang et al., 2021). This review serves to systematically summarize the usefulness, histopathological changes, and scope of application of each model and current animals used in TMJOA research. We hope to provide an evidence-based reference for researchers to deepen their understanding and to select appropriate TMJOA animal models.
Characteristics of Temporomandibular Joint/Temporomandibular Joint Osteoarthritis
Anatomy and Physiology of the Temporomandibular Joint
TMJ, a joint that connects the mandible to the skull and regulates mandibular movement, is composed of the mandibular condyle, articular disc, and the articular eminence and glenoid fossa. The cartilage layer on the mandibular condyle is from the superficial layer downward and composed of several layers: the fibrous, proliferative, hypertrophic and calcified cartilage layers (Thilander et al., 1976). Instead of being covered by the hyaline cartilage, the articular surface of the mandibular condyle is covered with a layer of mature fibrous tissue, consisting of a mass of collagen fibers (Toller, 1974). The hyaline cartilage is mainly composed of type II collagen, whereas the fibrocartilage is mainly composed of type I collagen (Vos et al., 2014). The orientation of the fibers on the condylar surface is a wavy interlacing of collagen fibers, which makes most fibers tangent to the surface (Toller and Wilcox, 1978). This property allows the TMJ to better withstand shear forces, whereas the hyaline cartilage is more resistant to compressive loading. Unlike the articular cartilage of the knee, the condylar cartilage has a different embryonic origin, which is derived from cranial neural crest cells (Shen and Darendeliler, 2005). Moreover, the most intriguing biological aspect of the condylar cartilage that differs from other cartilages lies in its ability to remodel in response to the changes in condylar repositioning, articular functioning, and mechanical loading (Nakano et al., 2003). Possibly, these essential structural differences in the TMJ significantly modifies the clinical expression of its pathological changes.
Radiographical Features of Temporomandibular Joint Osteoarthritis
Several imaging techniques are available for TMJ visualization, including panoramic radiography, plain radiography, computed tomography (CT), magnetic resonance imaging (MRI), and high-resolution ultrasonography. Currently, CT and MRI are the most used imaging techniques (Talmaceanu et al., 2018). The radiographic manifestations of TMJOA include flattening of the anterior surface of the condyle, erosions, and irregularities of the joint surfaces, flattening of the articular surface of the temporal eminence, generalized sclerosis, subchondral cysts, osteophytes, and idiopathic condyle resorption (Zhao et al., 2011; Nah, 2012). Several studies suggest that erosive lesions may indicate acute or active changes, whereas sclerosis and flattening osteophytes may indicate a later and relatively stable stage (Ahmad et al., 2009).
Histopathologic Features of Temporomandibular Joint Osteoarthritis
The main manifestations of TMJOA are articular cartilage damage and degeneration, as well as repair of periarticular tissues and hyperplastic changes of the synovial membrane. Cartilage damage is characterized by irregular thinning and fibrillation of the fibrous layer, and reduced proteoglycan content of the cartilage matrix. Chondrocytes are frequently arranged in small groups or clusters, and many degenerated and necrotic chondrocytes are observed (de Bont et al., 1985a; de Bont et al., 1985b). In the superficial layers, the density of the collagen fibrils is diminished. The collagen fibrils show a loose and disordered arrangement. The calcified cartilage layer shows an irregular border adjacent to the fibrous layer and subchondral bone. Exposure of subchondral bone, hyperplasia, sclerosis, osteophyte formation and osteoblast activity can be found in subchondral bone (Toller, 1977) (Figure 1).
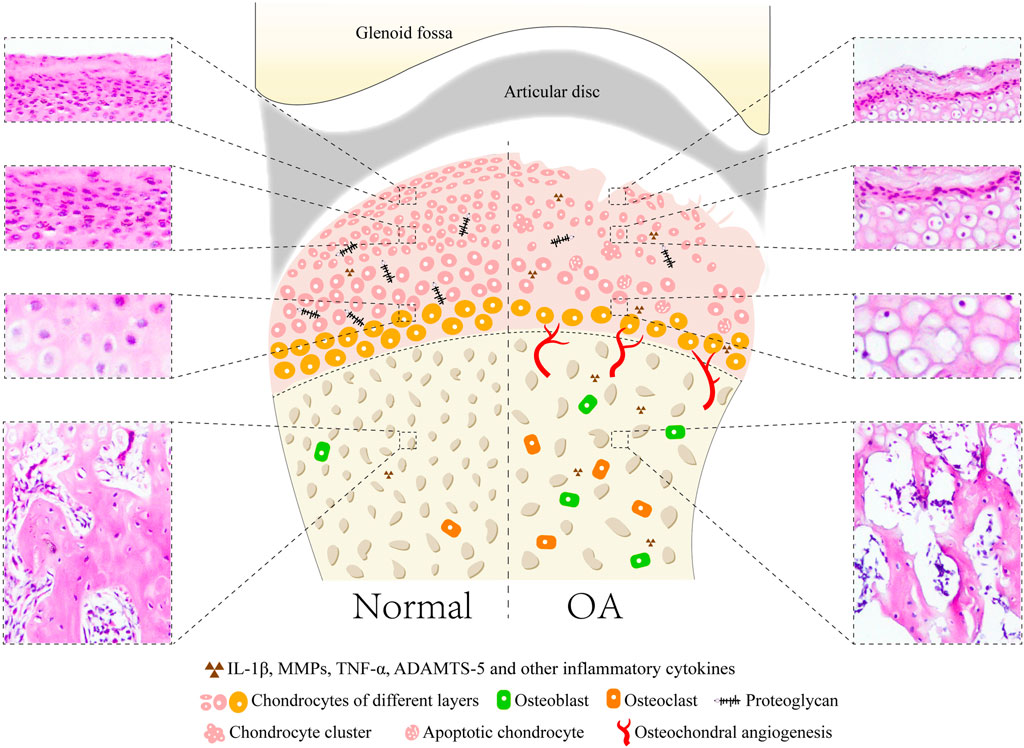
FIGURE 1. Common microstructural and histopathological alterations in cartilage and subchondral bone of TMJOA animal models with showing the normal joint and pathological joint. In normal joint, the surface of condylar cartilage is intact and smooth with four layers, including fibrous, proliferative, hypertrophic, and calcified cartilage layers. Lesions of cartilage include loss of cartilage surface integrity and proteoglycan, reduced and irregular arrangement of chondrocytes, presence of chondrocyte clusters and cell-free areas, decreased thickness of cartilage, apoptosis of chondrocytes and osteophyte formation. Subchondral bone appears as osteochondral angiogenesis, increased trabecular separation, large marrow cavities, decreased bone volume fraction, and activation of osteoblasts and osteoclasts.
The synovial membrane of the TMJ may initially undergo synovial intima hyperplasia and cell hypertrophy, and subsequently result in deposition of fibrous material in the intima matrix. Subintimal fibroblast activity increases, and subintima elastic fibers are present (Dijkgraaf et al., 1997). Neovascularization of the fossa cartilage and articular disc frequently occur. The joint capsule is usually thickened in the TMJ. Adhesions to the lateral TMJ structures, including the synovial membrane, articular disc, and articular eminence, are often found in the latter stages of TMJOA (Dijkgraaf et al., 1995).
In summary, TMJOA is a chronic disease characterized by degenerative changes in the cartilage, accompanied by repair of surrounding tissues. Notably, TMJOA is different from OA in other synovial joints. Numerous elastic fibers, giant collagen fibrils, prominent nuclear fibrous lamina, and mineral-containing matrix vesicles are found in the degenerated condylar cartilage, which are not found in knee joint OA. Moreover, the inflammatory infiltrate is less often present in the osteoarthritic synovial membrane of the TMJ than in other synovial joints (Roy and Meachim, 1968; Meachim and Sheffield, 1969; de Bont et al., 1985b). The etiology and treatment of TMJOA are different because of differences in the structure and origin of cells that give rise to TMJ structures. Therefore, special animal models are needed to study TMJOA.
Classification of Animal Models in Temporomandibular Joint Osteoarthritis
Although some similarities in the disease processes between animals and humans are known, no one animal model is sufficient for studying all features of TMJOA. The translatability of animal models mainly depends on how well they correspond to human conditions. Therefore, we systematically summarized the existing animal models of TMJOA (Figure 2). Animal models used to study TMJOA are broadly divided into induced, naturally occurring, and genetically modified models, depending on whether the animals are treated with or without intervention. The induced models can be divided into invasive models (intra-articular injection and surgical induction) or non-invasive models (mechanical loading, high-fat diet, and sleep deprivation). Different modeling approaches mimic different etiologies of TMJOA. Selection of an appropriate animal model in studying TMJOA may be challenging. Therefore, we summarized the advantages, disadvantages, and indications of TMJOA animal models (Table 1).
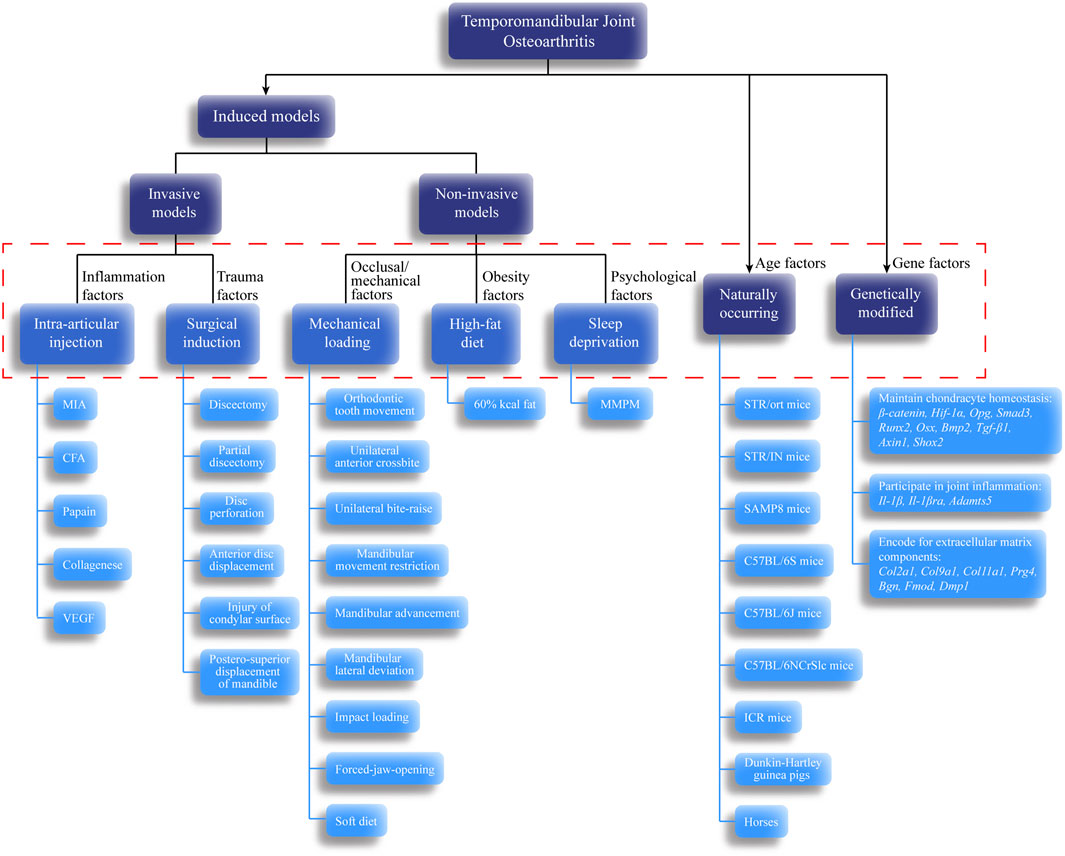
FIGURE 2. Classification of temporomandibular joint osteoarthritis (TMJOA) models. These models and their subdivisions share a relationship with TMJOA phenotypes. Black arrows indicate the classification based on whether the animals are treated with or without intervention. Dashed red box represents pathogenic factors simulated by each model. Blue lines indicate the specific animal species or model-building methods for each animal model.
Induced Models
Invasive Models
Invasive TMJOA models mainly work by producing joint destabilization, altered articular surface contact forces, and intra-articular inflammation in TMJs of animals. The procedures include injection and surgical approaches, which are related to high technique sensitivity. Therefore, improvement in the technical stability of researchers by long-term practice is the key to creating invasive models.
Intra-Articular Injection Models
Intra-articular injection is a well-characterized preclinical model of OA in the knee joint and TMJ. It causes disease by inducing intra-articular inflammation, cytotoxicity, or direct matrix damage in articular cartilage. Chondrocytes are the only cell type responsible for producing extracellular matrix and maintaining the homeostasis of cartilage (Dijkgraaf et al., 1995). Death of the chondrocytes, which results from necrosis or apoptosis, is a major feature of cartilage degeneration in OA (Aigner and Kim, 2002; Liu et al., 2021). Chemical drug injection can cause rapid death of many chondrocytes and destroy the homeostasis of chondrocytes, thereby creating joint damage and pain.
The commonly used drugs include monosodium iodoacetate (MIA) (Wang X. D. et al., 2012; Cledes et al., 2006; Guler et al., 2011), complete Freund’s adjuvant (CFA) (Rotpenpian et al., 2021; Xu et al., 2016; Xu et al., 2017), collagenase (Li W. et al., 2021; Li W. et al., 2014; Wu et al., 2015), papain (Molinet et al., 2020) and vascular endothelial growth factor (VEGF) (Shen et al., 2015) (Table 2). The first four drugs are common drugs in animal models of knee OA, which can cause different types of inflammation. The most frequently used drug among these drugs is MIA. However, a transcriptome study reported that ≤4% of total gene overlap occur between MIA-induced model and human OA (Barve et al., 2007). Despite this challenge, intra-articular injection still has the advantages of ease of induction and reproducibility. Additionally, the rate of disease progression and severity of joint lesions can be adjusted by changing drug concentration (Wang X. D. et al., 2012), which can provide acute disease model for researchers to design short-term studies.
Mice, rats, and rabbits are widely used in intra-articular injection models. The most common animal models are rats (Wang X. D. et al., 2012; Li W. et al., 2014; Xu et al., 2016; Xu et al., 2017), because rats are easily managed and require low maintenance costs. Using radio-opaque dye, Hutchins et al. have demonstrated that the superior joint space of rats can hold 50–70 µl of injectable fluid (Hutchins et al., 2000). Therefore, the current dose of drug injection is approximately 50 µl. Kameoka et al. (2010) have tested three puncture techniques which are commonly used in humans for TMJ cavity in rats, and have found that the puncture success rate for anterosuperior puncture technique (ASPT) was significantly higher than others. Currently, different puncture techniques are in use (Cledes et al., 2006; Wang X. D. et al., 2012; George et al., 2013). Further studies are still needed to determine a standard procedure.
Intra-articular injection models are mainly used to investigate the molecular mechanisms of osteoarthritic pain and screening of preclinical therapies (Kim et al., 2019; Sannajust et al., 2019; Yi et al., 2020; Rotpenpian et al., 2021). Xu et al. (2017) suggested that IHH signaling facilitates TMJOA in CFA-induced rats by driving formation of hypertrophic chondrocytes and expression of catabolic enzymes, such as type X collagen, MMP-13, and ADAMTS-5, which may lead to degenerative changes in the articular cartilage. As an angiogenic factor, NETRIN-1 has been found to be a possible regulator during bone degeneration and pain in the process of TMJOA in MIA-induced mice (Xiao et al., 2021). Moreover, intra-articular injection may be appropriate for screening symptom-modifying OA drugs but not for disease-modifying OA drugs, because its pathophysiology is distinct from that of naturally occurring OA (Thote et al., 2013). Even so, intra-articular injection models still have the virtue of detecting joint pain-related mechanisms due to the rapid injuries occurring in the cartilage.
Surgical Induction Models
Surgery is the most widely used approach for building OA models. It can cause structural damage and abnormal articular forces to induce OA-like lesions directly by using surgical and mechanical devices. The common surgical methods include discectomy (Hinton, 1992; Lan et al., 2017; Liu X. et al., 2020; Saito et al., 2021), partial discectomy (Man et al., 2009; Xu et al., 2009; Lei et al., 2022), disc perforation (Embree et al., 2015; Luo et al., 2020; Ruscitto et al., 2020), anterior disc displacement (Togni et al., 2018; Xu et al., 2018; Nguyen et al., 2020), injury of the condylar surface (Ishimaru and Goss, 1992; Wang F. et al., 2017), and postero-superior displacement of the mandible (Imai et al., 2001; Liu et al., 2006) (Table 3). These six methods well mimic advanced symptoms of joint injury in clinical patients, but they are not starting factors in general TMJOA. Hence, surgical induction models are not appropriate for the study of the mechanisms of TMJOA, except in the conditions of direct trauma to the mandible.
Each surgical approach has its unique effect on joint mechanics and causes different changes in the biomechanical environment in the TMJ cavity. Therefore, the OA-like lesions they formed have different disease progression rates. When selecting an appropriate surgical approach, the anatomy and biomechanics of the selected animal TMJ, expected progression of the disease, and severity of late-stage lesions of animal models should be understood. This understanding allows researchers gain control of the entire course of disease development.
Mice, rats, rabbits, pigs, and sheep are widely used in vivo preclinical studies as surgical induction models. Rabbits are the most used models (Imai et al., 2001; Man et al., 2009; Embree et al., 2015; Xu et al., 2018; Saito et al., 2021), because they are quite large and strong enough to fight off infection. Therefore, they tolerate surgeries; their joint tissues are large enough to undergo surgery and wear mechanical devices. In addition, rabbits are easier to operate and cheaper than large animals, such as pigs and sheep.
Surgical induction models are mainly used for tissue engineering, stem cell transplantation, and local growth factor treatment studies (Ying et al., 2013; Embree et al., 2016; Tarafder et al., 2016; Wang KH. et al., 2017). Embree et al. (2016) discovered that resident fibrocartilage stem cells (FCSCs) localized within the fibrous layer possess potent chondrogenic and osteogenic potential. Additionally, they suggested that regulation of canonical Wnt signals can sustain FCSC pool and maintain tissue homoeostasis, which provide new concepts on the development of potential therapies for TMJ regeneration. Before these regenerative strategies can be applied to humans, future studies using preclinical animal models are still required to include long-term cartilage and bone structure recovery, as well as biomechanical analyses, to verify that functional joint recovery is achieved. Therefore, surgical induction models are more suitable for osteochondral interface repair investigations, given the directly damaged TMJ structure.
One problem with many invasive models, however, is that they only operated on one side of the TMJ and use the other side as controls (Shinoda et al., 2005; Embree et al., 2015; Lemos et al., 2016). Unlike most other synovial joints, TMJ is bilaterally linked, and mastication, mouth opening, and other actions need to be completed together. Therefore, when using unilateral intervention methods, the influence on the other joint should be considered (Cohen et al., 2014). In recent years, various methods have been improved to create models while preserving as much tissue as possible in TMJ to prevent the effect of the surgical procedure on animals (Gu et al., 2006; Nguyen et al., 2020). In addition, different surgical approaches used in the same model will cause different pathological changes. Therefore, further studies on invasive models are needed in the future to achieve better model establishment.
Non-Invasive Models
Non-invasive models cause joint injury by applying external mechanical force, high fat diet, or mental stimulation without causing open trauma or articular capsule damage. In this way, the model-building process is completely sterile, and the effect of the invasive models on remaining joint tissues is eliminated. In addition, no surgical procedures are required on animals because such models mainly use mechanical devices to assist modeling.
Mechanical Loading Models
Appropriate stress stimulation can promote chondrocyte proliferation and extracellular matrix synthesis. However, when damage caused by mechanical loading exceeds the joint’s ability to repair itself, the affected joint would suffer damage, and even develops into OA (Tanaka et al., 2008; Liu et al., 2021). The functional movement and biomechanical loading of TMJ are closely related to occlusion. Abnormal dental occlusion is one of the potential causes of TMJOA, which includes severe malocclusion and skeletal jaw asymmetry. Thus, the TMJOA models can be built by disordered occlusion, which is the TMJ-specific model-building approach, including orthodontic tooth movement (Wang Q. Y. et al., 2012; Zhang et al., 2013), unilateral anterior crossbite (Wang Y. L. et al., 2014; Zhang et al., 2016; Liu J. et al., 2020), unilateral bite-raise (Long et al., 2019; Ou et al., 2021), mandibular movement restriction (Teramoto et al., 2003; Li et al., 2013), mandibular advancement (Yang et al., 2020; Li Y. et al., 2021), and mandibular lateral deviation (Zhao et al., 2010; Zou et al., 2022). Injury to the TMJ can be caused by indirect force to the mandible, which may lead to local pain, dislocation, or fracture, even TMJOA. Thus, impact loading can be used to establish the TMJOA model (Wang et al., 2008). Mastication provides a crucial mechanical stimulus for jawbone remodeling. Sufficient loading is important in maintaining the appropriate proliferation of chondrocytes and matrix production in the condyle. Thus, muscle overuse or underuse, such as forced-jaw-opening (Fujisawa et al., 2003; Nicoll et al., 2010; Khurel-Ochir et al., 2021) and soft diet (Ikeda et al., 2014; Robinson et al., 2019), can be used to establish TMJOA models by affecting the metabolism of the condyle. The above models simulated TMJOA caused by occlusal factors in clinical patients (Table 4).
The most common animals used for mechanical loading models are rats (Teramoto et al., 2003; Li et al., 2013; Long et al., 2019; Yang et al., 2020; Li Y. et al., 2021; Zou et al., 2022), because rats are common rodents and can tolerate the installation of mechanical devices. However, due to the significant differences in occlusal and TMJ structure between rats and human, OA-like lesions induced by the mechanical loading method are not completely equivalent to human TMJOA lesions (Wang et al., 2015). Given the high similarity between mechanical loading models and human TMJOA caused by occlusal factor, larger animals with a more similar structure to human TMJ, such as pigs and sheep, should be used in future studies on pathogenesis.
Currently, mechanical loading models are mainly applied to study the mechanism of pathological changes in TMJOA (Jiao et al., 2009; Jiao et al., 2011; Ma et al., 2020; Ou et al., 2021), probably because this kind of model directly mimics the disease process in TMJOA patients caused by disordered occlusion. The pathogenic mechanism of TMJOA in mechanical loading models has been widely discussed. Zhang et al. (2022) revealed that elevated expression of SEMA4D in early-stage TMJOA might decrease the bone formation activity of osteoblasts in the subchondral bone by binding to PLEXIN-B1 expressed by osteoblasts. HIF-1, which may repress OPG expression, was activated in mature chondrocytes in mechanical loading models, resulting in osteoclastogenesis and development of TMJOA (Shirakura et al., 2010). He et al. (2018) discovered several new genes that had never been reported to be associated with TMJOA by RNA sequencing. These genes may be used as potential therapeutic genes related to TMJOA. In the future, attention should be paid to the development of therapeutic strategies that take full advantage of this model.
High-Fat Diet Models
Obesity is found to be associated with OA. Overweight and obesity do not only significantly increase the risk of incident hip and knee OA, but also aggravate its radiographic changes (Johnson and Hunter, 2014). Studies in experimental animals have shown that obesity increases the incidence and severity of OA (Issa and Griffin, 2012). According to the cross-sectional studies, obesity is also associated with TMJ disease (Jordani et al., 2017; Karaman and Sadry, 2021). In addition, several chewing characteristics, such as chewing speed and duration, are associated with obesity in young adolescents, and they might affect the development of the TMJ. Therefore, further investigation is needed to reveal the relationship among jaw mastication, obesity, and TMJOA.
Griffin et al. (2010) first observed loss of proteoglycans in the TMJ of C57BL/6J mice with a high-fat diet (45% kcal fat) for 45 weeks. Du et al. (2020) studied the effect of high-fat diet (60% kcal fat) on TMJ of C57BL/6 mice. Less cartilage matrix, thinner condylar cartilage, and vertical clefts were observed in overweight mice after 12 weeks of high-fat feeding. Additionally, they found that the expression of IL-1β, MMP-3 and leptin were upregulated in condylar cartilage of high-fat-fed mice. Patients with knee OA have increased level of serum leptin and the abnormal leptin level in synovial fluid. Leptins are one of the increased proinflammatory factors in individuals with obesity (Conde et al., 2013). This research team also confirmed that statins had anti-inflammatory effects in TMJOA-like changes and a protective effect on the damaged TMJ cartilage.
Not only does diet induce obesity-increased OA joint pathology in mice but also induces anxiety and hyperalgesia, and reduces muscle function and locomotor activity (Griffin et al., 2010). Currently, studies only focus on the establishment of high-fat TMJOA model, and more investigations will be required in the future to reveal the mechanisms involved in obesity-induced TMJOA.
Sleep Deprivation Models
Osteoarthritis is one of the characteristics of premature aging in organisms. It is affected by circadian disturbances (Berenbaum and Meng, 2016). Many studies have shown that psychological factors, such as sleep disorders, mental stress, and depression, may be related to TMJ dysfunction (LeResche et al., 2007; Slade et al., 2007). Therefore, establishment of sleep deprivation models could be helpful for related studies. This model mainly applies the modified multiple platform method (MMPM) proposed by Suchecki and Tufik (2000). The principle of this technique is to take advantage of the rat’s fear of water and inability to sleep in water. A certain number of platforms with small diameters (≤6.5 cm in diameter) are placed in a tank filled with water. The rats can stand on the platform and jump between the platforms. When the rats are about to sleep, their muscles relax and their faces would touch the water, which could awake them to achieve the goal of sleep deprivation in rats.
Chen et al. (2013) first showed that the surface of fibrous layer was cracked and exfoliated in sleep-deprived rats, compared with control rats, suggesting that sleep deprivation may lead to histopathological changes in the TMJ of rats. Chen et al. (2020) then successfully built a TMJOA model by using a similar model and demonstrated that sleep deprivation could induce OA-like lesions in TMJ of rats, and the OA-like lesions may be reversible in the early stage. Additionally, they found that rhythmic gene expression dysregulation in sleep deprivation models, which further leads to MAPK/ERK signaling pathway activation and then aggravates TMJOA. Chen Y. et al. (2019) indicated that hypoxia played an important role in TMJOA and accelerated angiogenesis of condylar cartilage through the HIF-1-VEGF-Notch signaling pathway. These studies may provide new insights into the clock gene mechanism of endochondral homeostasis and the complex pathophysiological mechanism of TMJOA.
Currently, this model only applies to rats, and the therapeutic effect of low intensity pulsed ultrasound (LIPUS) on this model has been studied. Liang et al. have found that LIPUS had a good treatment effect on early TMJ injury by regulating the MMP-3/TIMP-1 and RANKL/OPG expression ratios in cartilage tissues, and have demonstrated that LIPUS treatment at an intensity of 45 mW/cm2 for at least 2 weeks is the optimal regimen for TMJOA in rats (Liang et al., 2019; Liang et al., 2020). Given the difficulty of using humans as participants to advance this study, the establishment of an experimental animal model of TMJOA is necessary to further study the pathogenesis of TMJOA under psychological stress, especially in studies for testing clinical treatment and exploring better medications.
Naturally Occurring Models
Some animals develop OA-like lesions with slow progression, which is very similar to the disease progression of primary OA in humans. Therefore, such models are often referred to as naturally occurring models. Studies have shown that STR/Ort (Kumagai et al., 2015; Yamashita-Futani et al., 2021), STR/IN (Dreessen and Halata, 1990), SAMP8 (Ishizuka et al., 2014), C57BL/6S (Fukuoka et al., 1993), C57BL/6J (Cui et al., 2020), C57BL/6NCrSlc (Ukita et al., 2020), ICR (Silbermann and Livne, 1979; Livne and Silbermann, 1986) mice, Dunkin-Hartley guinea pigs (Wu et al., 2016), and horses (Smyth et al., 2019) all manifest OA-like lesions with increasing age, among which multiple subtypes of SAM mice can develop OA-like lesions in TMJ (Chen et al., 1989) (Table 5). Yamashita-Futani et al. (2021) found in STR/Ort mice that the production of elastin-digested peptides was related to the upregulation of pro-inflammatory mediators, such as IL-6 and MMP-12. IL-6 induced the expression of ADAMTS-4 and ADAMTS-5 in chondrocytes, following cartilage degradation. OA lesions also appeared in articular cartilage of C57B/6S mice and were correlated to increased levels of collagen-like peptidase and prolyl endopeptidase in the serum, which indicated collagen degradation (Fukuoka et al., 1993). Ishizuka et al. revealed that a downregulation of IHH signaling accompanies the early onset TMJ degeneration changes in senescence-accelerated mice (Ishizuka et al., 2014). Naturally occurring models are ideal for studying cartilage degradation and bone remodeling in TMJOA and can provide evidence for the study of pathogenesis of TMJOA at different ages. The induced model-building methods can also be used to cause diseases in such animals, which can naturally result in TMJOA to study the effect of external stimulus during the disease course of primary TMJOA.
The naturally occurring models have slow disease progression. Like the spontaneous OA-like lesions in human TMJ, naturally occurring models do not require invasive procedures to generate the arthritis, thus eliminating many potential side effects. They are thought to be closely related to the natural progression of TMJOA in humans, which are inapplicable for simulating the development of post-traumatic TMJOA. Since obtaining the articular cartilage samples of TMJOA in humans is difficult, naturally occurring models have gradually served as important models for the study of pathogenesis of OA. The underlying mechanisms that drive the onset and progression of spontaneous TMJOA in these animals are not well defined and may reflect specific subtypes of idiopathic human TMJOA. Currently, few studies have been conducted on this type of model. Due to the extremely long study period and high cost, most of these studies focus on the etiological mechanism.
Genetically Modified Models
The use of genetically modified mice has greatly improved our understanding of the precise molecular pathophysiology and therapies of many human diseases (Little and Hunter, 2013). In the field of TMJOA, specific genetic modifications are made to the mice to reveal the role of different genes in TMJ development or disease processes. Unlike invasive animal models, genetically modified models can provide biological information for a population that is prone to developing TMJOA. Since genetic and environmental factors can be precisely controlled, this kind of model has the potential to reveal molecular pathways involved in the progressive degeneration of TMJ.
Preclinical studies of genetically modified mice have increased over the past two decades, making them the best candidate models for the study of the molecular pathway involved in TMJOA (Table 6). The genes involved in this review can be divided into three main categories. The first group mainly maintained chondrocyte homeostasis; they include transcription factors or signaling regulators (β-catenin, Hif-1α, Opg, Smad3, Runx2, Osx, Bmp2, Tgf-β1, Axin1, Shox2), enzymes [1α(OH)ase, Dnmt3b], and receptors (Fgfr3, Bmpr1α, Ddr1, Ddr2). Genes that participate in joint inflammation are the second group, including cytokine (Il-1β), receptor (Il-1βra) and enzyme (Adamts5). The third group includes genes encoding for extracellular matrix components (Col2a1, Col9a1, Col11a1, Prg4, Bgn, Fmod, Dmp1). The genes and gene products identified in genetically modified models as decreasing or increasing the severity of TMJOA-associated cartilage erosion can all be considered potential therapeutic targets. Appropriate inhibitors of these proteins and activators or recombinant versions may lead to the development of new therapies, which need to be further investigated.
Xu et al. found increased expression of DDR2 and increased level of proteoglycans in early TMJOA in both Col9a1−/− and Col11a1−/− mice (Lam et al., 2007). Over time, the chondrocytes synthesize and release matrix-degrading enzymes that degrade proteoglycans. One of the consequences of proteoglycan degradation is to enhance the exposure of chondrocytes to type II collagen fibrils, which may result in the activation of DDR2. The activation of DDR2 induces the expression of MMP-13, which then cleaves type II collagen. This eventually leads to the irreversible destruction of the articular cartilage. Therefore, chondrocyte clusters and increased proteoglycan production in the pericellular matrix have also been identified as early OA indicators.
Although the mouse models cannot simulate the biomechanical function of human joints, it is a major option for molecular studies. This is due to the advances in mouse genetics, and the easy availability of genetically modified mice, allowing the evaluation of time-dependent changes in TMJOA after specific genetic modifications. Moreover, genetically modified models have the virtue of eliminating other interferences to allow researchers directly observe how individual genes influence the process of TMJOA at the genetic level. Consequently, this kind of model facilitates the study and establishment of the molecular basis for TMJOA development. However, multiple genes are generally implicated in the pathogenesis of human TMJOA (Pitsillides and Beier, 2011), whereas genetically modified models mainly act on specific genes. Therefore, this model cannot comprehensively simulate the situation of TMJOA induced by multi-gene interaction. As with naturally occurring models, researchers need consider the lengthy experimental time and the cost of housing these animals.
Current Animals Used in Temporomandibular Joint Osteoarthritis Research
Animal models are the primary means of testing potential therapeutic agents to determine their potential efficacy in TMJOA. However, existing animal models are inadequate to simulate complex clinical conditions. On the one hand, most animal models of TMJOA are single-factor models, which only simulate one specific pathogenic factor of clinical patients. On the other hand, most animal models are characterized by histological phenotypes and a few key molecular markers of TMJOA. Many models mimic the phenotype, but the similarity to the underlying molecular components of human TMJOA is typically not known.
As mentioned earlier, rodents are the most frequently used animals for TMJOA modeling. The primary disadvantages of these models are related to differences in anatomical structure and joint mechanics between these species and humans. The mandibular condyles of rodents extend antero-posteriorly, whereas in humans, the direction is lateromedial (Bermejo et al., 1993). The anterior-posterior axial length of the condyle is about 5 mm in rats and about 10 mm in rabbits, both of which were much smaller than that in humans (Orset et al., 2014; Monteiro et al., 2021). Moreover, the condyle axis is sagittal in rodents for propulsion movement, whereas it is transversal in humans for tridimensional motions, including opening, deduction, and propulsion (Orset et al., 2014). Some differences in disease expression between animal and human remain inexplicable. Unlike in humans, the incidence and severity of TMJOA is higher in male mice than in female mice (Silberberg and Silberberg, 1963). Even so, the advantages of small animal models include relatively low cost, ease of handling, more rapid disease progression, and availability of housing. As a result, they have been particularly popular for evaluating new therapeutic interventions and investigating the pathological process of TMJOA.
The advantage of large animal models is that they are anatomically similar to humans, particularly in joint size and cartilage thickness. Pigs have been regarded as the most suitable experimental model for human TMJ due to their similar condyles, articular disc, and mechanical properties to those of humans (Herring et al., 2002; Sun et al., 2002). However, disadvantages of large animal models are primarily related to the high costs, long maturation periods, and slow disease progression. Due to the rapid progress of TMJOA in small animals, the TMJ in small animals can be used for screening of potential therapeutics. The efficacy of drugs in small animals may not accurately reflect the efficacy observed in human TMJOA. Therefore, the TMJs from large animals, such as pigs and sheep, are still needed for preclinical studies to evaluate the clinical processes and their treatment in TMJOA. Despite various problems, animal models are still irreplaceable at least in the study of the pathology and progression of TMJOA rather than the etiology of TMJOA.
Discussion
Animal models of TMJOA are important tools for studying the pathogenesis of TMJOA and evaluating potential therapeutic interventions. The value of these models mainly depends on how well they correspond with human disease. Various methods are used to build disease models of TMJOA, but each kind of model has its limitations. For each new study, considering the application of each model may help guide model selection. Different animal models could induce different TMJOA lesions. For example, chemical models can be used for the study of pain mechanisms; surgical models may be optimal for therapeutic study. Mechanical models may be appropriate for the study of pathogenesis; naturally occurring models would provide best models for studying aging phenotype; and genetically modified models are required for research of specific genes.
Animal models of OA can be classified into five categories: naturally occurring, genetically modified, surgically induced, chemically induced, and non-invasive animal models (Lampropoulou-Adamidou et al., 2014; McCoy, 2015). Some laboratory animals, such as certain strains of Syrian hamsters, dogs and cynomolgus macaques can develop OA spontaneously. These animals have not been used in studies of TMJOA yet. Since the anatomical structure and physiological composition of other synovial joints are different from those of the TMJ, most surgically induced models and non-invasive models cannot be applied to the modeling of TMJOA. Among them, impact loading has been applied to TMJ and successfully induced OA-like lesions in TMJ (Wang et al., 2008). In addition to the five drugs used for intra-articular injection models, quinolone and carrageenan are commonly used in other synovial joints to induce OA (McCoy, 2015). These drugs can be used to induce TMJOA in the future.
Age is one of the strongest risk factors for OA. The incidence and severity of TMJOA increase with age (Haskin et al., 1995). In contrast, most preclinical studies are conducted in young animals. For example, in the most widely used rat model of TMJOA, namely unilateral anterior crossbite (UAC), mechanical loading is usually performed on 6-8-week-old rats. However, comparison of 6-week-old and 28-week-old rats revealed that age affects the basal pattern of gene expression in joint tissues. When UAC is performed on 28-week-old rats, the ensuing TMJOA is more severe than in young rats (Zhang Y. et al., 2021). Since OA-like lesions occurr spontaneously with age in naturally occurring models, various induction methods can be used on these animals to study how other stimulus and aging synergistically affect TMJ.
Reports on the prevalence of TMJOA have shown significant gender differences (Zhao et al., 2011; Li et al., 2020). Its preponderance in women and early onset during reproductive years according to epidemiological research are totally different from the epidemiological characteristics of other joints, such as in knee OA, which primarily happens to postmenopausal women (Zhao et al., 2019; Dai et al., 2020). Severe TMJOA has been reported in young females whose blood oestrogen levels were medically low (Gunson et al., 2009), thus the relationship between TMJOA and oestrogen has attracted much attention. Studies have shown that oestrogen has an important effect on bone and cartilage metabolism. Oestrogen can regulate the secretion of cytokines and affect some key metabolic pathways to regulate bone and cartilage metabolism (Wang Q. P. et al., 2012). Several animal studies have confirmed that oestrogen deficiency leads to cartilage degeneration in the condyle, and results in more severe TMJOA-like lesions in the presence of mechanical stress stimulation (Nogami et al., 2020; Zhang J. et al., 2021). More studies are needed to further explore the role of oestrogen in the pathogenesis of TMJOA and related molecular mechanisms.
Notably, TMJOA involves not only cartilage but all the joint tissues; therefore, analysis of cartilage and periarticular tissues is recommended in vivo studies. Most of the studies evaluated cartilage degeneration and bone reconstruction by histology, histomorphometry, and immunohistochemistry. Only few studies analyzed the changes in articular disc, synovial membrane, and temporal surface. Therefore, the pathological changes in the whole joint should be studied to understand the etiological mechanism of TMJOA from a comprehensive perspective.
Over the past 40 years, TMJOA animal models have undoubtedly improved our understanding of the pathophysiology of the disease and contributed to the development of disease-modifying therapies. This review presents an overview of animal models used to study TMJOA, as well as the usefulness, histopathological changes, and scope of application of each model and current animals used in TMJOA research. Although many methods are used to build disease models, no single ideal animal model has been established for the comprehensive study TMJOA. This is because current models are mostly single-factor models, which cannot fully reflect the etiology and progression of TMJOA. In the future, the modeling approach should be improved, and more multi-factor models should be established to provide more suitable animal models for further study of TMJOA.
Author Contributions
YZ, YA and LZ contributed equally to the content of the work. All authors contributed to the article and approved the submitted version.
Funding
This work was supported by National Natural Science Foundation of China (61701520, 61871393, 81602651) and Taishan Scholar Foundation of Shandong Province (tsqn201812137).
Conflict of Interest
The authors declare that the research was conducted in the absence of any commercial or financial relationships that could be construed as a potential conflict of interest.
Publisher’s Note
All claims expressed in this article are solely those of the authors and do not necessarily represent those of their affiliated organizations, or those of the publisher, the editors and the reviewers. Any product that may be evaluated in this article, or claim that may be made by its manufacturer, is not guaranteed or endorsed by the publisher.
References
Ahmad M., Hollender L., Anderson Q., Kartha K., Ohrbach R., Truelove E. L., et al. (2009). Research Diagnostic Criteria for Temporomandibular Disorders (Rdc/Tmd): Development of Image Analysis Criteria and Examiner Reliability for Image Analysis. Oral Surg. Oral Med. Oral Pathol. Oral Radiol. Endodontology 107, 844–860. doi:10.1016/j.tripleo.2009.02.023
Aigner T., Kim H. A. (2002). Apoptosis and Cellular Vitality: Issues in Osteoarthritic Cartilage Degeneration. Arthritis Rheum. 46, 1986–1996. doi:10.1002/art.10554
Al-Moraissi E. A., Wolford L. M., Ellis E., Neff A. (2020). The Hierarchy of Different Treatments for Arthrogenous Temporomandibular Disorders: A Network Meta-Analysis of Randomized Clinical Trials. J. Craniomaxillofac. Surg. 48, 9–23. doi:10.1016/j.jcms.2019.10.004
Almarza A. J., Brown B. N., Arzi B., Ângelo D. F., Chung W., Badylak S. F., et al. (2018). Preclinical Animal Models for Temporomandibular Joint Tissue Engineering. Tissue Eng. B: Rev. 24, 171–178. doi:10.1089/ten.teb.2017.0341
Barve R. A., Minnerly J. C., Weiss D. J., Meyer D. M., Aguiar D. J., Sullivan P. M., et al. (2007). Transcriptional Profiling and Pathway Analysis of Monosodium Iodoacetate-Induced Experimental Osteoarthritis in Rats: Relevance to Human Disease. Osteoarthritis and Cartilage 15, 1190–1198. doi:10.1016/j.joca.2007.03.014
Berenbaum F., Meng Q.-J. (2016). The Brain-Joint Axis in Osteoarthritis: Nerves, Circadian Clocks and beyond. Nat. Rev. Rheumatol. 12, 508–516. doi:10.1038/nrrheum.2016.93
Bermejo A., González O., González J. M. (1993). The Pig as an Animal Model for Experimentation on the Temporomandibular Articular Complex. Oral Surg. Oral Med. Oral Pathol. 75, 18–23. doi:10.1016/0030-4220(93)90399-o
Bhatti F.-U. -R., Karydis A., Lee B. S., Deguchi T., Kim D.-G., Cho H. (2021). Understanding Early-Stage Posttraumatic Osteoarthritis for Future Prospects of Diagnosis: From Knee to Temporomandibular Joint. Curr. Osteoporos. Rep. 19, 166–174. doi:10.1007/s11914-021-00661-3
Chen D., Liu Y., Liu Z., Wang P. (2019a). Opg Is Required for the Postnatal Maintenance of Condylar Cartilage. Calcif Tissue Int. 104, 461–474. doi:10.1007/s00223-018-00510-z
Chen G., Zhao H., Ma S., Chen L., Wu G., Zhu Y., et al. (2020). Circadian Rhythm Protein Bmal1 Modulates Cartilage Gene Expression in Temporomandibular Joint Osteoarthritis via the Mapk/Erk Pathway. Front. Pharmacol. 11, 527744. doi:10.3389/fphar.2020.527744
Chen J., Wu G., Zhu G., Wang P., Chen H., Zhao H. (2013). Influence of Sleep Deprivation on Expression of Mkk4 and C-Fos in the Mandibular Condylar Cartilage of Rats. Br. J. Oral Maxillofac. Surg. 51, E250–E255. doi:10.1016/j.bjoms.2013.06.010
Chen W. H., Hosokawa M., Tsuboyama T., Ono T., Iizuka T., Takeda T. (1989). Age-Related Changes in the Temporomandibular Joint of the Senescence Accelerated Mouse. Sam-P/3 as A New Murine Model of Degenerative Joint Disease. Am. J. Pathol. 135, 379–385.
Chen Y., Zhao B., Zhu Y., Zhao H., Ma C. (2019b). Hif-1-Vegf-Notch Mediates Angiogenesis in Temporomandibular Joint Osteoarthritis. Am. J. Transl Res. 11, 2969–2982.
Cledes G., Felizardo R., Foucart J.-M., Carpentier P. (2006). Validation of A Chemical Osteoarthritis Model in Rabbit Temporomandibular Joint: A Compliment to Biomechanical Models. Int. J. Oral Maxill. Surg. 35, 1026–1033. doi:10.1016/j.ijom.2006.05.003
Cohen W. A., Servais J. M., Polur I., Li Y., Xu L. (2014). Articular Cartilage Degeneration in the Contralateral Non-surgical Temporomandibular Joint in Mice with A Unilateral Partial Discectomy. J. Oral Pathol. Med. 43, 162–165. doi:10.1111/jop.12113
Conde J., Scotece M., López V., Gómez R., Lago F., Pino J., et al. (2013). Adipokines: Novel Players in Rheumatic Diseases. Discov. Med. 15, 73–83.
Cui C., Zheng L., Fan Y., Zhang J., Xu R., Xie J., et al. (2020). Parathyroid Hormone Ameliorates Temporomandibular Joint Osteoarthritic-like Changes Related to Age. Cell Prolif 53, E12755. doi:10.1111/cpr.12755
Dai X., Ying P., Ding W., Li H., Xu P., Huang Z., et al. (2020). Genetic Estrogen Receptor Alpha Gene Pvuii Polymorphism in Susceptibility to Knee Osteoarthritis in A Chinese Han Population: A Southern Jiangsu Study. The Knee 27, 803–808. doi:10.1016/j.knee.2020.02.010
de Bont L. G. M., Boering G., Liem R. S. B., Havinga P. (1985a). Osteoarthritis of the Temporomandibular Joint: A Light Microscopic and Scanning Electron Microscopic Study of the Articular Cartilage of the Mandibular Condyle. J. Oral Maxill. Surg. 43, 481–488. doi:10.1016/s0278-2391(85)80024-0
de Souza R. F., Lovato Da Silva C. H., Nasser M., Fedorowicz Z., Al-Muharraqi M. A. (2012). Interventions for the Management of Temporomandibular Joint Osteoarthritis. Cochrane Database Syst Rev 4, 1465–1858. doi:10.1002/14651858.CD007261.pub2
Dijkgraaf L. C., De Bont L. G. M., Boering G., Liem R. S. B. (1995). The Structure, Biochemistry, and Metabolism of Osteoarthritic Cartilage: A Review of the Literature. J. Oral Maxill. Surg. 53, 1182–1192. doi:10.1016/0278-2391(95)90632-0
Dijkgraaf L. C., Liem R. S. B., De Bont L. G. M. (1997). Synovial Membrane Involvement in Osteoarthritic Temporomandibular Joints. Oral Surg. Oral Med. Oral Pathol. Oral Radiol. Endodontology 83, 373–386. doi:10.1016/s1079-2104(97)90246-8
Dreessen D., Halata Z. (1990). Age-Related Osteo-Arthrotic Degeneration of the Temporomandibular Joint in the Mouse. Cells Tissues Organs 139, 91–96. doi:10.1159/000146984
Du J., Jiang Q., Mei L., Yang R., Wen J., Lin S., et al. (2020). Effect of High Fat Diet and Excessive Compressive Mechanical Force on Pathologic Changes of Temporomandibular Joint. Sci. Rep. 10, 17457. doi:10.1038/s41598-020-74326-z
Embree M. C., Chen M., Pylawka S., Kong D., Iwaoka G. M., Kalajzic I., et al. (2016). Exploiting Endogenous Fibrocartilage Stem Cells to Regenerate Cartilage and Repair Joint Injury. Nat. Commun. 7, 13073. doi:10.1038/ncomms13073
Embree M. C., Iwaoka G. M., Kong D., Martin B. N., Patel R. K., Lee A. H., et al. (2015). Soft Tissue Ossification and Condylar Cartilage Degeneration Following Tmj Disc Perforation in A Rabbit Pilot Study. Osteoarthritis and Cartilage 23, 629–639. doi:10.1016/j.joca.2014.12.015
Embree M. C., Kilts T. M., Ono M., Inkson C. A., Syed-Picard F., Karsdal M. A., et al. (2010). Biglycan and Fibromodulin Have Essential Roles in Regulating Chondrogenesis and Extracellular Matrix Turnover in Temporomandibular Joint Osteoarthritis. Am. J. Pathol. 176, 812–826. doi:10.2353/ajpath.2010.090450
Fujisawa T., Kuboki T., Kasai T., Sonoyama W., Kojima S., Uehara J., et al. (2003). A Repetitive, Steady Mouth Opening Induced an Osteoarthritis-like Lesion in the Rabbit Temporomandibular Joint. J. Dent Res. 82, 731–735. doi:10.1177/154405910308200914
Fukuoka Y., Hagihara M., Nagatsu T., Kaneda T. (1993). The Relationship between Collagen Metabolism and Temporomandibular Joint Osteoarthrosis in Mice. J. Oral Maxill. Surg. 51, 288–291. doi:10.1016/s0278-2391(10)80177-6
Ge C., Mohamed F., Binrayes A., Kapila S., Franceschi R. T. (2018). Selective Role of Discoidin Domain Receptor 2 in Murine Temporomandibular Joint Development and Aging. J. Dent Res. 97, 321–328. doi:10.1177/0022034517738190
George M. D., Owen C. M., Reinhardt A. L., Giannini P. J., Marx D. B., Reinhardt R. A. (2013). Effect of Simvastatin Injections on Temporomandibular Joint Inflammation in Growing Rats. J. Oral Maxill. Surg. 71, 846–853. doi:10.1016/j.joms.2012.12.020
Ghassemi Nejad S., Kobezda T., Tar I., Szekanecz Z. (2017). Development of Temporomandibular Joint Arthritis: The Use of Animal Models. Jt. Bone Spine 84, 145–151. doi:10.1016/j.jbspin.2016.05.016
G.M. de Bont L., S.B. Liem R., Boering G. (1985b). Ultrastructure of the Articular Cartilage of the Mandibular Condyle: Aging and Degeneration. Oral Surg. Oral Med. Oral Pathol. 60, 631–641. doi:10.1016/0030-4220(85)90367-6
Griffin T. M., Fermor B., Huebner J. L., Kraus V. B., Rodriguiz R. M., Wetsel W. C., et al. (2010). Diet-Induced Obesity Differentially Regulates Behavioral, Biomechanical, and Molecular Risk Factors for Osteoarthritis in Mice. Arthritis Res. Ther. 12, R130. doi:10.1186/ar3068
Gu Z., Zhou Y., Zhang Y., Zhao S., Liu J., Hu J. (2006). An Animal Model for Inducing Anterior Disc Displacement of the Temporomandibular Joint. J. Orofac Pain 20, 166–173.
Güler N., Kürkçü M., Duygu G., Çam B. (2011). Sodium Iodoacetate Induced Osteoarthrosis Model in Rabbit Temporomandibular Joint: Ct and Histological Study (Part I). Int. J. Oral Maxill. Surg. 40, 1289–1295. doi:10.1016/j.ijom.2011.07.908
Gunson M. J., Arnett G. W., Formby B., Falzone C., Mathur R., Alexander C. (2009). Oral Contraceptive Pill Use and Abnormal Menstrual Cycles in Women with Severe Condylar Resorption: A Case for Low Serum 17β-Estradiol as a Major Factor in Progressive Condylar Resorption. Am. J. Orthod. Dentofacial Orthopedics 136, 772–779. doi:10.1016/j.ajodo.2009.07.011
Haskin C. L., Milam S. B., Cameron I. L. (1995). Pathogenesis of Degenerative Joint Disease in the Human Temporomandibular Joint. Crit. Rev. Oral Biol. Med. 6, 248–277. doi:10.1177/10454411950060030601
He D., An Y., Li Y., Wang J., Wu G., Chen L., et al. (2018). Rna Sequencing Reveals Target Genes of Temporomandibular Joint Osteoarthritis in Rats after the Treatment of Low-Intensity Pulsed Ultrasound. Gene 672, 126–136. doi:10.1016/j.gene.2018.06.002
Herring S. W., Decker J. D., Liu Z.-J., Ma T. (2002). Temporomandibular Joint in Miniature Pigs: Anatomy, Cell Replication, and Relation to Loading. Anat. Rec. 266, 152–166. doi:10.1002/ar.10049
Hill A., Duran J., Purcell P. (2014). Lubricin Protects the Temporomandibular Joint Surfaces from Degeneration. Plos One 9, E106497. doi:10.1371/journal.pone.0106497
Hinton R. J. (1992). Alterations in Rat Condylar Cartilage Following Discectomy. J. Dent Res. 71, 1292–1297. doi:10.1177/00220345920710060501
Huang H., Shank G., Ma L., Tallents R., Kyrkanides S. (2013). Nerve Growth Factor Induced after Temporomandibular Joint Inflammation Decelerates Chondrocyte Differentiation. Oral Dis. 19, 604–610. doi:10.1111/odi.12045
Huey D. J., Hu J. C., Athanasiou K. A. (2012). Unlike Bone, Cartilage Regeneration Remains Elusive. Science 338, 917–921. doi:10.1126/science.1222454
Hui T., Zhou Y., Wang T., Li J., Zhang S., Liao L., et al. (2018). Activation of β-catenin Signaling in Aggrecan-Expressing Cells in Temporomandibular Joint Causes Osteoarthritis-like Defects. Int. J. Oral Sci. 10, 13. doi:10.1038/s41368-018-0016-z
Hutchins B., Spears R., Hinton R. J., Harper R. P. (2000). Calcitonin Gene-Related Peptide and Substance P Immunoreactivity in Rat Trigeminal Ganglia and Brainstem Following Adjuvant-Induced Inflammation of the Temporomandibular Joint. Arch. Oral Biol. 45, 335–345. doi:10.1016/s0003-9969(99)00129-6
Ikeda Y., Yonemitsu I., Takei M., Shibata S., Ono T. (2014). Mechanical Loading Leads to Osteoarthritis-like Changes in the Hypofunctional Temporomandibular Joint in Rats. Arch. Oral Biol. 59, 1368–1376. doi:10.1016/j.archoralbio.2014.08.010
Imai H., Sakamoto I., Yoda T., Yamashita Y. (2001). A Model for Internal Derangement and Osteoarthritis of the Temporomandibular Joint with Experimental Traction of the Mandibular Ramus in Rabbit. Oral Dis. 7, 185–191. doi:10.1034/j.1601-0825.2001.70308.x
Ishimaru J.-I., Goss A. N. (1992). A Model for Osteoarthritis of the Temporomandibular Joint. J. Oral Maxill. Surg. 50, 1191–1195. doi:10.1016/0278-2391(92)90153-q
Ishizuka Y., Shibukawa Y., Nagayama M., Decker R., Kinumatsu T., Saito A., et al. (2014). Tmj Degeneration in Samp8 Mice Is Accompanied by Deranged Ihh Signaling. J. Dent Res. 93, 281–287. doi:10.1177/0022034513519649
Issa R. I., Griffin T. M. (2012). Pathobiology of Obesity and Osteoarthritis: Integrating Biomechanics and Inflammation. Pathobiol Aging Age Relat. Dis. 2, 1. doi:10.3402/pba.v2i0.17470
Jiao K., Niu L.-N., Wang M.-Q., Dai J., Yu S.-B., Liu X.-D., et al. (2011). Subchondral Bone Loss Following Orthodontically Induced Cartilage Degradation in the Mandibular Condyles of Rats. Bone 48, 362–371. doi:10.1016/j.bone.2010.09.010
Jiao K., Wang M.-Q., Niu L.-N., Dai J., Yu S.-B., Liu X.-D., et al. (2009). Death and Proliferation of Chondrocytes in the Degraded Mandibular Condylar Cartilage of Rats Induced by Experimentally Created Disordered Occlusion. Apoptosis 14, 22–30. doi:10.1007/s10495-008-0279-5
Jiao K., Zhang M., Niu L., Yu S., Zhen G., Xian L., et al. (2014). Overexpressed TGF-β in Subchondral Bone Leads to Mandibular Condyle Degradation. J. Dent Res. 93, 140–147. doi:10.1177/0022034513513034
Jing J., Hinton R. J., Mishina Y., Liu Y., Zhou X., Feng J. Q. (2014b). Critical Role of Bmpr1a in Mandibular Condyle Growth. Connect. Tissue Res. 55 (Suppl. 1), 73–78. doi:10.3109/03008207.2014.923858
Jing J., Hinton R. J., Jing Y., Liu Y., Zhou X., Feng J. Q. (2014a). Osterix Couples Chondrogenesis and Osteogenesis in Post-Natal Condylar Growth. J. Dent Res. 93, 1014–1021. doi:10.1177/0022034514549379
Johnson V. L., Hunter D. J. (2014). The Epidemiology of Osteoarthritis. Best Pract. Res. Clin. Rheumatol. 28, 5–15. doi:10.1016/j.berh.2014.01.004
Jordani P. C., Campi L. B., Circeli G. Z., Visscher C. M., Bigal M. E., Gonçalves D. A. G. (2017). Obesity as A Risk Factor for Temporomandibular Disorders. J. Oral Rehabil. 44, 1–8. doi:10.1111/joor.12453
Kameoka S., Matsumoto K., Kai Y., Yonehara Y., Arai Y., Honda K. (2010). Establishment of Temporomandibular Joint Puncture Technique in Rats Usingin Vivomicro-Computed Tomography (R_mCT). Dentomaxillofacial Radiol. 39, 441–445. doi:10.1259/dmfr/37174063
Karaman A., Sadry S. (2021). Evaluation of Temporomandibular Disorders and Oral Health-Related Quality of Life with Obese Patients. Cranio 39, 510–517. doi:10.1080/08869634.2019.1694777
Khurel-Ochir T., Izawa T., Iwasa A., Kano F., Yamamoto A., Tanaka E. (2021). The Immunoregulatory Role of P21 in the Development of the Temporomandibular Joint-Osteoarthritis. Clin. Exp. Dent Res. 7, 313–322. doi:10.1002/cre2.404
Kim Y.-H., Bang J.-I., Son H.-J., Kim Y., Kim J. H., Bae H., et al. (2019). Protective Effects of Extracorporeal Shockwave on Rat Chondrocytes and Temporomandibular Joint Osteoarthritis; Preclinical Evaluation with In Vivo 99mTc-HDP SPECT and Ex Vivo Micro-CT. Osteoarthritis and Cartilage 27, 1692–1701. doi:10.1016/j.joca.2019.07.008
Kloppenburg M., Berenbaum F. (2020). Osteoarthritis Year in Review 2019: Epidemiology and Therapy. Osteoarthritis and Cartilage 28, 242–248. doi:10.1016/j.joca.2020.01.002
Koyama E., Saunders C., Salhab I., Decker R. S., Chen I., Um H., et al. (2014). Lubricin Is Required for the Structural Integrity and Post-Natal Maintenance of Tmj. J. Dent Res. 93, 663–670. doi:10.1177/0022034514535807
Kumagai K., Suzuki S., Kanri Y., Matsubara R., Fujii K., Wake M., et al. (2015). Spontaneously Developed Osteoarthritis in the Temporomandibular Joint in Str/Ort Mice. Biomed. Rep. 3, 453–456. doi:10.3892/br.2015.467
Lai Y.-C., Shaftel S. S., Miller J.-n. H., Tallents R. H., Chang Y., Pinkert C. A., et al. (2006). Intraarticular Induction of Interleukin-1β Expression in the Adult Mouse, with Resultant Temporomandibular Joint Pathologic Changes, Dysfunction, and Pain. Arthritis Rheum. 54, 1184–1197. doi:10.1002/art.21771
Lam N. P., Li Y., Waldman A. B., Brussiau J., Lee P. L., Olsen B. R., et al. (2007). Age-Dependent Increase of Discoidin Domain Receptor 2 and Matrix Metalloproteinase 13 Expression in Temporomandibular Joint Cartilage of Type Ix and Type Xi Collagen-Deficient Mice. Arch. Oral Biol. 52, 579–584. doi:10.1016/j.archoralbio.2006.10.014
Lampropoulou-Adamidou K., Lelovas P., Karadimas E. V., Liakou C., Triantafillopoulos I. K., Dontas I., et al. (2014). Useful Animal Models for the Research of Osteoarthritis. Eur. J. Orthop. Surg. Traumatol. 24, 263–271. doi:10.1007/s00590-013-1205-2
Lan L., Jiang Y., Zhang W., Li T., Ying B., Zhu S. (2017). Expression of Notch Signaling Pathway during Osteoarthritis in the Temporomandibular Joint. J. Craniomaxillofac. Surg. 45, 1338–1348. doi:10.1016/j.jcms.2017.05.029
Lei J., Chen S., Jing J., Guo T., Feng J., Ho T. V., et al. (2022). Inhibiting Hh Signaling in Gli1+ Osteogenic Progenitors Alleviates TMJOA. J. Dent Res. 2022, 220345211059079. doi:10.1177/00220345211059079
Lemos G. A., Rissi R., De Souza Pires I. L., De Oliveira L. P., De Aro A. A., Pimentel E. R., et al. (2016). Low-Level Laser Therapy Stimulates Tissue Repair and Reduces the Extracellular Matrix Degradation in Rats with Induced Arthritis in the Temporomandibular Joint. Lasers Med. Sci. 31, 1051–1059. doi:10.1007/s10103-016-1946-3
LeResche L., Mancl L. A., Drangsholt M. T., Huang G., Von Korff M. (2007). Predictors of Onset of Facial Pain and Temporomandibular Disorders in Early Adolescence. Pain 129, 269–278. doi:10.1016/j.pain.2006.10.012
Li D., Li S., Chen Q., Xie X. (2020). The Prevalence of Symptomatic Knee Osteoarthritis in Relation to Age, Sex, Area, Region, and Body Mass Index in China: A Systematic Review and Meta-Analysis. Front. Med. 7, 304. doi:10.3389/fmed.2020.00304
Li H., Zhang X.-Y., Wu T.-J., Cheng W., Liu X., Jiang T.-T., et al. (2013). Endoplasmic Reticulum Stress Regulates Rat Mandibular Cartilage Thinning under Compressive Mechanical Stress. J. Biol. Chem. 288, 18172–18183. doi:10.1074/jbc.m112.407296
Li W., Hu S., Chen X., Shi J. (2021a). The Antioxidant Resveratrol Protects against Chondrocyte Apoptosis by Regulating the COX-2/nf-Κb Pathway in Created Temporomandibular Osteoarthritis. Biomed. Res. Int. 2021, 9978651. doi:10.1155/2021/9978651
Li W., Wu M., Jiang S., Ding W., Luo Q., Shi J. (2014a). Expression of Adamts-5 and Timp-3 in the Condylar Cartilage of Rats Induced by Experimentally Created Osteoarthritis. Arch. Oral Biol. 59, 524–529. doi:10.1016/j.archoralbio.2014.02.016
Li X., Liang W., Ye H., Weng X., Liu F., Liu X. (2014b). Overexpression of Shox2 Leads to Congenital Dysplasia of the Temporomandibular Joint in Mice. Ijms 15, 13135–13150. doi:10.3390/ijms150813135
Li X., Liu H., Gu S., Liu C., Sun C., Zheng Y., et al. (2014c). Replacing Shox2 with Human Shox Leads to Congenital Disc Degeneration of the Temporomandibular Joint in Mice. Cell Tissue Res 355, 345–354. doi:10.1007/s00441-013-1743-2
Li Y., Yang J., Liu Y., Yan X., Zhang Q., Chen J., et al. (2021b). Inhibition of Mtorc1 in the Rat Condyle Subchondral Bone Aggravates Osteoarthritis Induced by the Overly Forward Extension of the Mandible. Am. J. Transl Res. 13, 270–285.
Liang C., Yang T., Wu G., Li J., Geng W. (2020). The Optimal Regimen for the Treatment of Temporomandibular Joint Injury Using Low-Intensity Pulsed Ultrasound in Rats with Chronic Sleep Deprivation. Biomed. Res. Int. 2020, 5468173. doi:10.1155/2020/5468173
Liang C., Yang T., Wu G., Li J., Geng W. (2019). Therapeutic Effect of Low-Intensity Pulsed Ultrasound on Temporomandibular Joint Injury Induced by Chronic Sleep Deprivation in Rats. Am. J. Transl Res. 11, 3328–3340.
Liang W., Li X., Chen H., Shao X., Lin X., Shen J., et al. (2016). Expressing Human SHOX in Shox2SHOX KI/KI Mice Leads to Congenital Osteoarthritis-like Disease of the Temporomandibular Joint in Postnatal Mice. Mol. Med. Rep. 14, 3676–3682. doi:10.3892/mmr.2016.5715
Liao L., Zhang S., Zhou G. Q., Ye L., Huang J., Zhao L., et al. (2019). Deletion of Runx2 in Condylar Chondrocytes Disrupts Tmj Tissue Homeostasis. J. Cel Physiol 234, 3436–3444. doi:10.1002/jcp.26761
Little C. B., Hunter D. J. (2013). Post-traumatic Osteoarthritis: From Mouse Models to Clinical Trials. Nat. Rev. Rheumatol. 9, 485–497. doi:10.1038/nrrheum.2013.72
Liu J., Yang H., Zhang H., Liu Q., Zhou P., He F., et al. (2020a). Biomechanically Reduced Expression of Derlin-3 Is Linked to the Apoptosis of Chondrocytes in the Mandibular Condylar Cartilage via the Endoplasmic Reticulum Stress Pathway. Arch. Oral Biol. 118, 104843. doi:10.1016/j.archoralbio.2020.104843
Liu L. K., Hong Y. J., Tao D. T., Li X. Q., Yi X. Z. (2006). Evaluation of A Model of Temporomandibular Disorders Established by Transzygomatic Arch Traction of the Mandibular Ramus in Rabbits. Zhonghua Kou Qiang Yi Xue Za Zhi 41, 755–756.
Liu Q., Yang H., Zhang M., Zhang J., Lu L., Yu S., et al. (2021). Initiation and Progression of Dental-Stimulated Temporomandibular Joints Osteoarthritis. Osteoarthritis and Cartilage 29, 633–642. doi:10.1016/j.joca.2020.12.016
Liu X., Cai H. X., Cao P. Y., Feng Y., Jiang H. H., Liu L., et al. (2020b). TLR4 Contributes to the Damage of Cartilage and Subchondral Bone in Discectomy-Induced TMJOA Mice. J. Cel. Mol. Med. 24, 11489–11499. doi:10.1111/jcmm.15763
Livne E., Silbermann M. (1986). Further Characterization of Spontaneous Arthritic Changes in Murine Squamo-Mandibular Joint: Histopathological Aspects. Histol. Histopathol 1, 161–170.
Long E., Motwani R., Reece D., Pettit N., Hepworth J., Wong P., et al. (2016). The Role of Tgf-Ss1 in Osteoarthritis of the Temporomandibular Joint in Two Genetic Mouse Models. Arch. Oral Biol. 67, 68–73. doi:10.1016/j.archoralbio.2016.03.004
Long H.-q., Tian P.-f., Guan Y.-x., Liu L.-x., Wu X.-p., Li B. (2019). Expression of Ihh Signaling Pathway in Condylar Cartilage after Bite-Raising in Adult Rats. J. Mol. Hist. 50, 459–470. doi:10.1007/s10735-019-09840-0
Luo P., Peng S., Yan Y., Ji P., Xu J. (2020). Il-37 Inhibits M1-like Macrophage Activation to Ameliorate Temporomandibular Joint Inflammation through the Nlrp3 Pathway. Rheumatology (Oxford) 59, 3070–3080. doi:10.1093/rheumatology/keaa192
Ma S., Zhang A., Li X., Zhang S., Liu S., Zhao H., et al. (2020). Mir-21-5p Regulates Extracellular Matrix Degradation and Angiogenesis in Tmjoa by Targeting Spry1. Arthritis Res. Ther. 22, 99. doi:10.1186/s13075-020-2145-y
Man C., Zhu S., Zhang B., Hu J. (2009). Protection of Articular Cartilage from Degeneration by Injection of Transforming Growth Factor-Beta in Temporomandibular Joint Osteoarthritis. Oral Surg. Oral Med. Oral Pathol. Oral Radiol. Endodontology 108, 335–340. doi:10.1016/j.tripleo.2009.05.001
McCoy A. M. (2015). Animal Models of Osteoarthritis. Vet. Pathol. 52, 803–818. doi:10.1177/0300985815588611
Meachim G., Sheffield S. R. (1969). Surface Ultrastructure of Mature Adult Human Articular Cartilage. J. Bone Jt. Surg Br 51, 529–539.
Mino-Oka A., Izawa T., Shinohara T., Mori H., Yasue A., Tomita S., et al. (2017). Roles of Hypoxia Inducible Factor-1α in the Temporomandibular Joint. Arch. Oral Biol. 73, 274–281. doi:10.1016/j.archoralbio.2016.10.028
Molinet M., Alves N., Vasconcelos A., Deana N. F. (2020). Comparative Study of Osteoarthritis Induced by Monoiodoacetate and Papain in Rabbit Temporomandibular Joints: Macroscopic and Microscopic Analysis. Folia Morphol. 79, 516–527. doi:10.5603/fm.a2019.0104
Monteiro J., Takusagawa T., Vasconcelos B. C. E., Pai S. I., Mccain J. P., Guastaldi F. P. S. (2021). A Rabbit Model to Investigate Temporomandibular Joint Osteochondral Regeneration. Oral Surg. Oral Med. Oral Pathol. Oral Radiol. [Online ahead of print]. doi:10.1016/j.oooo.2021.12.004
Mori H., Izawa T., Tanaka E. (2015). Smad3 Deficiency Leads to Mandibular Condyle Degradation via the Sphingosine 1-Phosphate (S1p)/S1p3 Signaling Axis. Am. J. Pathol. 185, 2742–2756. doi:10.1016/j.ajpath.2015.06.015
Nah K.-S. (2012). Condylar Bony Changes in Patients with Temporomandibular Disorders: A Cbct Study. Imaging Sci. Dent 42, 249–253. doi:10.5624/isd.2012.42.4.249
Nakano H., Watahiki J., Kubota M., Maki K., Shibasaki Y., Hatcher D., et al. (2003). Micro X-Ray Computed Tomography Analysis for the Evaluation of Asymmetrical Condylar Growth in the Rat. Orthod. Craniofac. Res. 6 (Suppl. 1), 168–172. doi:10.1034/j.1600-0544.2003.252.x
Nguyen N. G. K., Nishiyama A., Shimada M. (2020). A Rat Model for Inducing Temporomandibular Anterior Disc Displacement Experimentally. J. Oral Sci. 62, 70–74. doi:10.2334/josnusd.19-0093
Nicoll S. B., Hee C. K., Davis M. B., Winkelstein B. A. (2010). A Rat Model of Temporomandibular Joint Pain with Histopathologic Modifications. J. Orofac Pain 24, 298–304.
Nogami S., Yamauchi K., Odashima K., Ito K., Iikubo M., Kumasaka A., et al. (2020). Influence of Oestrogen Deficiency and Excessive Mechanical Stress on Condylar Head of Mandible. Oral Dis. 26, 1718–1726. doi:10.1111/odi.13452
O'Brien M. H., Dutra E. H., Mehta S., Chen P. J., Yadav S. (2021). Bmp2 Is Required for Postnatal Maintenance of Osteochondral Tissues of the Temporomandibular Joint. Cartilage 13, 734s–743s. doi:10.1177/1947603520980158
Orset E., Chaffanjon P., Bettega G. (2014). Temporomandibular Joint Model: Anatomic and Radiologic Comparison between Rat and Human. Surg. Radiol. Anat. 36, 163–166. doi:10.1007/s00276-013-1159-4
Ou F., Huang Y., Sun J., Su K., He Y., Zeng R., et al. (2021). Yohimbine Ameliorates Temporomandibular Joint Chondrocyte Inflammation with Suppression of NF-Κb Pathway. Inflammation 44, 80–90. doi:10.1007/s10753-020-01310-0
Pitsillides A. A., Beier F. (2011). Cartilage Biology in Osteoarthritis-Lessons from Developmental Biology. Nat. Rev. Rheumatol. 7, 654–663. doi:10.1038/nrrheum.2011.129
Polur I., Lee P. L., Servais J. M., Xu L., Li Y. (2010). Role of Htra1, A Serine Protease, in the Progression of Articular Cartilage Degeneration. Histol. Histopathol 25, 599–608. doi:10.14670/HH-25.599
Ricks M. L., Farrell J. T., Falk D. J., Holt D. W., Rees M., Carr J., et al. (2013). Osteoarthritis in Temporomandibular Joint of Col2a1 Mutant Mice. Arch. Oral Biol. 58, 1092–1099. doi:10.1016/j.archoralbio.2013.02.008
Rintala M., Metsäranta M., Säämänen A. M., Vuorio E., Rönning O. (1997). Abnormal Craniofacial Growth and Early Mandibular Osteoarthritis in Mice Harbouring A Mutant Type Ii Collagen Transgene. J. Anat. 190 (Pt 2), 201–208. doi:10.1046/j.1469-7580.1997.19020201.x
Robinson J., Soria P., Lu H. H., Chen J., Wadhwa S. (2019). Structure-Function Relationships of the Temporomandibular Joint in Response to Altered Loading. J. Oral Facial Pain Headache 39, 451–458. doi:10.11607/ofph.2094
Rogers A. W., Cisewski S. E., Kern C. B. (2018). The Zonal Architecture of the Mandibular Condyle Requires Adamts5. J. Dent Res. 97, 1383–1390. doi:10.1177/0022034518777751
Rogers-DeCotes A. W., Porto S. C., Dupuis L. E., Kern C. B. (2021). Adamts5 Is Required for Normal Trabeculated Bone Development in the Mandibular Condyle. Osteoarthritis and Cartilage 29, 547–557. doi:10.1016/j.joca.2021.01.005
Rotpenpian N., Tapechum S., Vattarakorn A., Chindasri W., Care C., Pakaprot N., et al. (2021). Evolution of Mirror-Image Pain in Temporomandibular Joint Osteoarthritis Mouse Model. J. Appl. Oral Sci. 29, E20200575. doi:10.1590/1678-7757-2020-0575
Roy S., Meachim G. (1968). Chondrocyte Ultrastructure in Adult Human Articular Cartilage. Ann. Rheum. Dis. 27, 544–558. doi:10.1136/ard.27.6.544
Ruscitto A., Morel M. M., Shawber C. J., Reeve G., Lecholop M. K., Bonthius D., et al. (2020). Evidence of Vasculature and Chondrocyte to Osteoblast Transdifferentiation in Craniofacial Synovial Joints: Implications for Osteoarthritis Diagnosis and Therapy. FASEB j. 34, 4445–4461. doi:10.1096/fj.201902287r
Saito Y., Tsutsui T., Takayama A., Moroi A., Yoshizawa K., Ueki K. (2021). Effect of Low-Intensity Pulsed Ultrasound on Injured Temporomandibular Joints with or without Articular Disc Removal in A Rabbit Model. J. Dental Sci. 16, 287–295. doi:10.1016/j.jds.2020.04.002
Sannajust S., Imbert I., Eaton V., Henderson T., Liaw L., May M., et al. (2019). Females Have Greater Susceptibility to Develop Ongoing Pain and Central Sensitization in A Rat Model of Temporomandibular Joint Pain. Pain 160, 2036–2049. doi:10.1097/j.pain.0000000000001598
Schminke B., Muhammad H., Bode C., Sadowski B., Gerter R., Gersdorff N., et al. (2014). A Discoidin Domain Receptor 1 Knock-Out Mouse as A Novel Model for Osteoarthritis of the Temporomandibular Joint. Cell. Mol. Life Sci. 71, 1081–1096. doi:10.1007/s00018-013-1436-8
Shen G., Darendeliler M. A. (2005). The Adaptive Remodeling of Condylar Cartilage- A Transition from Chondrogenesis to Osteogenesis. J. Dent Res. 84, 691–699. doi:10.1177/154405910508400802
Shen M., Luo Y., Niu Y., Chen L., Yuan X., Goltzman D., et al. (2013). 1,25(Oh)2d Deficiency Induces Temporomandibular Joint Osteoarthritis via Secretion of Senescence-Associated Inflammatory Cytokines. Bone 55, 400–409. doi:10.1016/j.bone.2013.04.015
Shen P., Jiao Z., Zheng J. S., Xu W. F., Zhang S. Y., Qin A., et al. (2015). Injecting Vascular Endothelial Growth Factor into the Temporomandibular Joint Induces Osteoarthritis in Mice. Sci. Rep. 5, 16244. doi:10.1038/srep16244
Shinoda M., Ozaki N., Asai H., Nagamine K., Sugiura Y. (2005). Changes in P2x3 Receptor Expression in the Trigeminal Ganglion Following Monoarthritis of the Temporomandibular Joint in Rats. Pain 116, 42–51. doi:10.1016/j.pain.2005.03.042
Shirakura M., Tanimoto K., Eguchi H., Miyauchi M., Nakamura H., Hiyama K., et al. (2010). Activation of the Hypoxia-Inducible Factor-1 in Overloaded Temporomandibular Joint, and Induction of Osteoclastogenesis. Biochem. Biophysical Res. Commun. 393, 800–805. doi:10.1016/j.bbrc.2010.02.086
Silberberg M., Silberberg R. (1963). Role of Sex Hormone in the Pathogenesis of Osteoarthrosis of Mice. Lab. Invest. 12, 285–289.
Silbermann M., Livne E. (1979). Age-Related Degenerative Changes in the Mouse Mandibular Joint. J. Anat. 129, 507–520.
Slade G. D., Diatchenko L., Bhalang K., Sigurdsson A., Fillingim R. B., Belfer I., et al. (2007). Influence of Psychological Factors on Risk of Temporomandibular Disorders. J. Dent Res. 86, 1120–1125. doi:10.1177/154405910708601119
Smyth T. T., Allen A. L., Carmalt J. L. (2019). Histologic Assessment of Age-Related Changes in the Temporomandibular Joints of Horses. Am. J. Vet. Res. 80, 1107–1113. doi:10.2460/ajvr.80.12.1107
Suchecki D., Tufik S. (2000). Social Stability Attenuates the Stress in the Modified Multiple Platform Method for Paradoxical Sleep Deprivation in the Rat. Physiol. Behav. 68, 309–316. doi:10.1016/s0031-9384(99)00181-x
Sun Z., Liu Z.-J., Herring S. W. (2002). Movement of Temporomandibular Joint Tissues during Mastication and Passive Manipulation in Miniature Pigs. Arch. Oral Biol. 47, 293–305. doi:10.1016/s0003-9969(02)00004-3
Suzuki A., Iwata J. (2016). Mouse Genetic Models for Temporomandibular Joint Development and Disorders. Oral Dis. 22, 33–38. doi:10.1111/odi.12353
Tabeian H., Betti B. F., Dos Santos Cirqueira C., De Vries T. J., Lobbezoo F., Ter Linde A. V., et al. (2019). IL-1β Damages Fibrocartilage and Upregulates MMP-13 Expression in Fibrochondrocytes in the Condyle of the Temporomandibular Joint. Int. J. Mol. Sci. 20. doi:10.3390/ijms20092260
Talmaceanu D., Lenghel L. M., Bolog N., Hedesiu M., Buduru S., Rotar H., et al. (2018). Imaging Modalities for Temporomandibular Joint Disorders: An Update. Med. Pharm. Rep. 91, 280–287. doi:10.15386/cjmed-970
Tanaka E., Detamore M. S., Mercuri L. G. (2008). Degenerative Disorders of the Temporomandibular Joint: Etiology, Diagnosis, and Treatment. J. Dent Res. 87, 296–307. doi:10.1177/154405910808700406
Tang Y., Hong C., Cai Y., Zhu J., Hu X., Tian Y., et al. (2020). HIF-1α Mediates Osteoclast-Induced Mandibular Condyle Growth via AMPK Signaling. J. Dent Res. 99, 1377–1386. doi:10.1177/0022034520935788
Tarafder S., Koch A., Jun Y., Chou C., Awadallah M. R., Lee C. H. (2016). Micro-Precise Spatiotemporal Delivery System Embedded in 3d Printing for Complex Tissue Regeneration. Biofabrication 8, 025003. doi:10.1088/1758-5090/8/2/025003
Teramoto M., Kaneko S., Shibata S., Yanagishita M., Soma K. (2003). Effect of Compressive Forces on Extracellular Matrix in Rat Mandibular Condylar Cartilage. J. Bone Mineral Metab. 21, 276–286. doi:10.1007/s00774-003-0421-y
Thilander B., Carlsson G. E., Ingervall B. (1976). Postnatal Development of the Human Temporomandibular Joint I. A Histological Study. Acta Odontologica Scand. 34, 117–126. doi:10.3109/00016357609026564
Thote T., Lin A. S. P., Raji Y., Moran S., Stevens H. Y., Hart M., et al. (2013). Localized 3d Analysis of Cartilage Composition and Morphology in Small Animal Models of Joint Degeneration. Osteoarthritis and Cartilage 21, 1132–1141. doi:10.1016/j.joca.2013.05.018
Togni L., De Abreu M. C., Augustin A. H., Da Silva R. B. M., Campos M. M. (2018). Characterization of A Rat Model with Temporomandibular Joint Osteoarthritis Following A Surgical Anterior Disc Displacement. Am. J. Transl Res. 10, 3806–3817.
Toller P. A. (1974). Temporomandibular Arthropathy. Proc. R. Soc. Med. 67, 153–159. doi:10.1177/003591577406700228
Toller P. A. (1977). Ultrastructure of the Condylar Articular Surface in Severe Mandibular Pain-Dysfunction Syndrome. Int. J. Oral Surg. 6, 297–312. doi:10.1016/s0300-9785(77)80023-9
Toller P. A., Wilcox J. H. (1978). Ultrastructure of the Articular Surface of the Condyle in Temporomandibular Arthropathy. Oral Surg. Oral Med. Oral Pathol. 45, 232–245. doi:10.1016/0030-4220(78)90090-7
Ukita M., Matsushita K., Tamura M., Yamaguchi T. (2020). Histone H3k9 Methylation Is Involved in Temporomandibular Joint Osteoarthritis. Int. J. Mol. Med. 45, 607–614. doi:10.3892/ijmm.2019.4446
Vapniarsky N., Huwe L. W., Arzi B., Houghton M. K., Wong M. E., Wilson J. W., et al. (2018). Tissue Engineering toward Temporomandibular Joint Disc Regeneration. Sci. Transl Med. 10. doi:10.1126/scitranslmed.aaq1802
Vos L. M., Kuijer R., Huddleston Slater J. J. R., Bulstra S. K., Stegenga B. (2014). Inflammation Is More Distinct in Temporomandibular Joint Osteoarthritis Compared to the Knee Joint. J. Oral Maxill. Surg. 72, 35–40. doi:10.1016/j.joms.2013.08.022
Wadhwa S., Embree M., Ameye L., Young M. F. (2005). Mice Deficient in Biglycan and Fibromodulin as A Model for Temporomandibular Joint Osteoarthritis. Cells Tissues Organs 181, 136–143. doi:10.1159/000091375
Wang F., Sun Y., He D., Wang L. (2017a). Effect of Concentrated Growth Factors on the Repair of the Goat Temporomandibular Joint. J. Oral Maxill. Surg. 75, 498–507. doi:10.1016/j.joms.2016.09.006
Wang K. H., Chan W. P., Chiu L. H., Tsai Y. H., Fang C. L., Yang C. B., et al. (2017b). Histological and Immunohistochemical Analyses of Repair of the Disc in the Rabbit Temporomandibular Joint Using A Collagen Template. Materials (Basel) 10, 924. doi:10.3390/ma10080924
Wang M., Li S., Li S., Xie W., Shen J., Im H.-J., et al. (2014a). Activation of Beta-Catenin Signalling Leads to Temporomandibular Joint Defects. Eur. Cel Mater 28, 223–235. doi:10.22203/ecm.v028a15
Wang Q. P., Yang L., Li X. P., Xie H., Liao E. Y., Wang M., et al. (2012a). Effects of 17beta-Estradiol on Adiponectin Regulation of the Expression of Osteoprotegerin and Receptor Activator of Nuclear Factor-Kappab Ligand. Bone 51, 515–523. doi:10.1016/j.bone.2012.05.011
Wang Q. Y., Dai J., Kuang B., Zhang J., Yu S. B., Duan Y. Z., et al. (2012b). Osteochondral Angiogenesis in Rat Mandibular Condyles with Osteoarthritis-like Changes. Arch. Oral Biol. 57, 620–629. doi:10.1016/j.archoralbio.2011.12.006
Wang X. D., Kou X. X., He D. Q., Zeng M. M., Meng Z., Bi R. Y., et al. (2012c). Progression of Cartilage Degradation, Bone Resorption and Pain in Rat Temporomandibular Joint Osteoarthritis Induced by Injection of Iodoacetate. Plos One 7, E45036. doi:10.1371/journal.pone.0045036
Wang X. D., Zhang J. N., Gan Y. H., Zhou Y. H. (2015). Current Understanding of Pathogenesis and Treatment of Tmj Osteoarthritis. J. Dent Res. 94, 666–673. doi:10.1177/0022034515574770
Wang Y. L., Li X. J., Qin R. F., Lei D. L., Liu Y. P., Wu G. Y., et al. (2008). Matrix Metalloproteinase and its Inhibitor in Temporomandibular Joint Osteoarthrosis after Indirect Trauma in Young Goats. Br. J. Oral Maxillofac. Surg. 46, 192–197. doi:10.1016/j.bjoms.2007.10.007
Wang Y. L., Zhang J., Zhang M., Lu L., Wang X., Guo M., et al. (2014b). Cartilage Degradation in Temporomandibular Joint Induced by Unilateral Anterior Crossbite Prosthesis. Oral Dis. 20, 301–306. doi:10.1111/odi.12112
Weng Y., Liu Y., Du H., Li L., Jing B., Zhang Q., et al. (2017). Glycosylation of Dmp1 Is Essential for Chondrogenesis of Condylar Cartilage. J. Dent Res. 96, 1535–1545. doi:10.1177/0022034517717485
Wu G., Zhu S., Sun X., Hu J. (2015). Subchondral Bone Changes and Chondrogenic Capacity of Progenitor Cells from Subchondral Bone in the Collagenase-Induced Temporomandibular Joints Osteoarthritis Rabbit Model. Int. J. Clin. Exp. Pathol. 8, 9782–9789.
Wu M., Lu H., Yu F., Zhou Y. (2016). Trend of Cadherin-11 Expression and its Impact on Cartilage Degradation in the Temporomandibular Joints of Guinea Pigs with Spontaneous Osteoarthritis. J. Oral Pathol. Med. 45, 534–538. doi:10.1111/jop.12403
Xiang T., Tao Z. Y., Liao L. F., Wang S., Cao D. Y. (2021). Animal Models of Temporomandibular Disorder. J. Pain Res. 14, 1415–1430. doi:10.2147/jpr.s303536
Xiao M., Hu Z., Jiang H., Li C., Guo H., Fang W., et al. (2021). The Expression of Netrin-1 in the Mia-Induced Osteoarthritic Temporomandibular Joint in Mice. Sci. Rep. 11, 15695. doi:10.1038/s41598-021-95251-9
Xu L., Guo H., Li C., Xu J., Fang W., Long X. (2016). A Time-dependent Degeneration Manner of Condyle in Rat Cfa-Induced Inflamed Tmj. Am. J. Transl Res. 8, 556–567.
Xu L., Polur I., Lim C., Servais J. M., Dobeck J., Li Y., et al. (2009). Early-Onset Osteoarthritis of Mouse Temporomandibular Joint Induced by Partial Discectomy. Osteoarthritis Cartilage 17, 917–922. doi:10.1016/j.joca.2009.01.002
Xu T., Gu Z., Wu H., Yao H., Wang G. (2018). Expression of Endoplasmic Reticulum Stress Protein in Rabbit Condyle Cartilage Following Anterior Disk Displacement. J. Oral Pathol. Med. 47, 606–612. doi:10.1111/jop.12715
Xu T., Xu G., Gu Z., Wu H. (2017). Hedgehog Signal Expression in Articular Cartilage of Rat Temporomandibular Joint and Association with Adjuvant-Induced Osteoarthritis. J. Oral Pathol. Med. 46, 284–291. doi:10.1111/jop.12497
Yamashita-Futani Y., Jokaji R., Ooi K., Kobayashi K., Kanakis I., Liu K., et al. (2021). Metalloelastase-12 Is Involved in the Temporomandibular Joint Inflammatory Response as Well as Cartilage Degradation by Aggrecanases in Str/Ort Mice. Biomed. Rep. 14, 51. doi:10.3892/br.2021.1427
Yang J., Li Y., Liu Y., Zhang Q., Zhang Q., Chen J., et al. (2020). Role of the Sdf-1/Cxcr4 Signaling Pathway in Cartilage and Subchondral Bone in Temporomandibular Joint Osteoarthritis Induced by Overloaded Functional Orthopedics in Rats. J. Orthop. Surg. Res. 15, 330. doi:10.1186/s13018-020-01860-x
Yasuda T., Nah H. D., Laurita J., Kinumatsu T., Shibukawa Y., Shibutani T., et al. (2012). Muenke Syndrome Mutation, Fgfr3p(2)(4)(4)R, Causes Tmj Defects. J. Dent Res. 91, 683–689. doi:10.1177/0022034512449170
Yi X., Wu L., Liu J., Qin Y. X., Li B., Zhou Q. (2020). Low-Intensity Pulsed Ultrasound Protects Subchondral Bone in Rabbit Temporomandibular Joint Osteoarthritis by Suppressing Tgf-Beta1/Smad3 Pathway. J. Orthop. Res. 38, 2505–2512. doi:10.1002/jor.24628
Ying B., Chen K., Hu J., Man C., Feng G., Zhang B., et al. (2013). Effect of Different Doses of Transforming Growth Factor-Beta(1) on Cartilage and Subchondral Bone in Osteoarthritic Temporomandibular Joints. Br. J. Oral Maxillofac. Surg. 51, 241–246. doi:10.1016/j.bjoms.2012.05.014
Zhang J., Zhang S., Qi W. J., Xu C. L., Zhou J., Wang J. H., et al. (2021a). Mechanism and Potential Contributing Factors to Temporomandibular Joint Osteoarthritis. Oral Dis 00, 1–10. doi:10.1111/odi.14061
Zhang M., Wang H., Zhang J., Zhang H., Yang H., Wan X., et al. (2016). Unilateral Anterior Crossbite Induces Aberrant Mineral Deposition in Degenerative Temporomandibular Cartilage in Rats. Osteoarthritis Cartilage 24, 921–931. doi:10.1016/j.joca.2015.12.009
Zhang M., Zhang J., Lu L., Qiu Z. Y., Zhang X., Yu S. B., et al. (2013). Enhancement of Chondrocyte Autophagy Is an Early Response in the Degenerative Cartilage of the Temporomandibular Joint to Biomechanical Dental Stimulation. Apoptosis 18, 423–434. doi:10.1007/s10495-013-0811-0
Zhang Y., Xu X., Zhou P., Liu Q., Zhang M., Yang H., et al. (2021b). Elder Mice Exhibit More Severe Degeneration and Milder Regeneration in Temporomandibular Joints Subjected to Bilateral Anterior Crossbite. Front. Physiol. 12, 750468. doi:10.3389/fphys.2021.750468
Zhang Z., Lu L., Ye T., Yu S., Zhang J., Zhang M., et al. (2022). Inhibition of Semaphorin 4d/Plexin-B1 Signaling Inhibits the Subchondral Bone Loss in Early-Stage Osteoarthritis of the Temporomandibular Joint. Arch. Oral Biol. 135, 105365. doi:10.1016/j.archoralbio.2022.105365
Zhao C., Kurita H., Kurashina K., Hosoya A., Arai Y., Nakamura H. (2010). Temporomandibular Joint Response to Mandibular Deviation in Rabbits Detected by 3d Micro-ct Imaging. Arch. Oral Biol. 55, 929–937. doi:10.1016/j.archoralbio.2010.07.019
Zhao H., Liu S., Ma C., Ma S., Chen G., Yuan L., et al. (2019). Estrogen-Related Receptor Gamma Induces Angiogenesis and Extracellular Matrix Degradation of Temporomandibular Joint Osteoarthritis in Rats. Front. Pharmacol. 10, 1290. doi:10.3389/fphar.2019.01290
Zhao Y. P., Zhang Z. Y., Wu Y. T., Zhang W. L., Ma X. C. (2011). Investigation of the Clinical and Radiographic Features of Osteoarthrosis of the Temporomandibular Joints in Adolescents and Young Adults. Oral Surg. Oral Med. Oral Pathol. Oral Radiol. Endod. 111, E27–E34. doi:10.1016/j.tripleo.2010.09.076
Zhou S., Xie Y., Li W., Huang J., Wang Z., Tang J., et al. (2016). Conditional Deletion of Fgfr3 in Chondrocytes Leads to Osteoarthritis-like Defects in Temporomandibular Joint of Adult Mice. Sci. Rep. 6, 24039. doi:10.1038/srep24039
Zhou Y., Chen M., O'keefe R. J., Shen J., Li Z., Zhou J., et al. (2019a). Epigenetic and Therapeutic Implications of Dnmt3b in Temporomandibular Joint Osteoarthritis. Am. J. Transl Res. 11, 1736–1747.
Zhou Y., Shu B., Xie R., Huang J., Zheng L., Zhou X., et al. (2019b). Deletion of Axin1 in Condylar Chondrocytes Leads to Osteoarthritis-like Phenotype in Temporomandibular Joint via Activation of Beta-Catenin and Fgf Signaling. J. Cel Physiol 234, 1720–1729. doi:10.1002/jcp.27043
Keywords: temporomandibular joint, osteoarthritis, animal models, induced models, naturally occurring models, genetically modified models
Citation: Zhao Y, An Y, Zhou L, Wu F, Wu G, Wang J and Chen L (2022) Animal Models of Temporomandibular Joint Osteoarthritis: Classification and Selection. Front. Physiol. 13:859517. doi: 10.3389/fphys.2022.859517
Received: 24 January 2022; Accepted: 04 April 2022;
Published: 28 April 2022.
Edited by:
Ali Mobasheri, University of Oulu, FinlandReviewed by:
Shouan Zhu, Ohio University, United StatesAkiko Suzuki, University of Missouri–Kansas City, United States
Copyright © 2022 Zhao, An, Zhou, Wu, Wu, Wang and Chen. This is an open-access article distributed under the terms of the Creative Commons Attribution License (CC BY). The use, distribution or reproduction in other forums is permitted, provided the original author(s) and the copyright owner(s) are credited and that the original publication in this journal is cited, in accordance with accepted academic practice. No use, distribution or reproduction is permitted which does not comply with these terms.
*Correspondence: Jing Wang, MTUwMTkxMTQ1QHFxLmNvbQ==; Lei Chen, c2RkeGNoZW5sZWlAMTYzLmNvbQ==
†These authors have contributed equally to this work and share first authorship