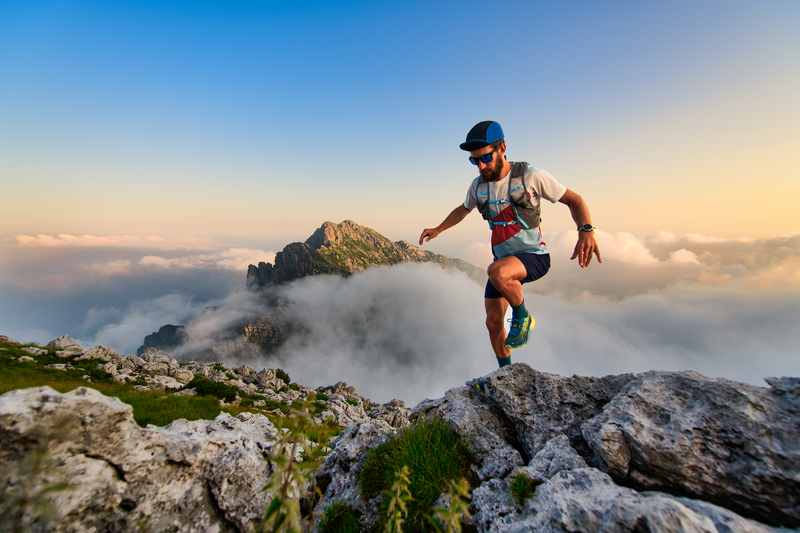
95% of researchers rate our articles as excellent or good
Learn more about the work of our research integrity team to safeguard the quality of each article we publish.
Find out more
ORIGINAL RESEARCH article
Front. Physiol. , 05 April 2022
Sec. Environmental, Aviation and Space Physiology
Volume 13 - 2022 | https://doi.org/10.3389/fphys.2022.853995
This article is part of the Research Topic Adaptive response of Living Beings to Extreme Environments: Integrative Approaches from Cellular and Molecular Biology, Biotechnology, Microbiology to Physiology View all 8 articles
Pigs are susceptible to low temperature conditions, and cold stress causes metabolic changes in the body to increase heat production as an adaption to adverse environments. To characterize and validate different metabolites in piglet livers at different cold exposure times, sixteen 30-day-old male weaned piglets with similar weights were randomly divided into four groups: the normal temperature group (24 ± 2°C, NT) and cold exposure (4 ± 2°C) 2-h group (CS2), 6-h group (CS6), and 12-h group (CS12). At the end of the experiment, the liver samples were analyzed using systemic non-targeted metabolomics. Eight known differentially abundant metabolites (farnesyl pyrophosphate, isocitrate, triethanolamine, phenylethylamine, deoxynosine, citric acid, maltotriose, and epinephrine) were observed between the CS groups and the control group in positive and negative ion modes. The eight main differentially abundant metabolites involved in seven metabolite classifications. Metabolic pathways and enrichment analyses revealed that the pathways involved three KEGG pathway classifications. Most of the pathways were related to amino acid or energy metabolism. Moreover, the metabolic pathways were not identical under different cold exposure times, with those following 2 and 6 h of cold exposure more related to carbohydrates and energy production and those following 12 h of cold exposure more related to the metabolism connected with epinephrine. Thus, under different cold exposure times, the metabolite profiles and metabolic pathways differed.
Low and high ambient temperature conditions are very common in livestock production and induce different levels of stress (Young, 1981). Pigs are sensitive to temperature changes, especially to low temperature, and cold stress is an important contributing factor to piglet morbidity and mortality (Muns et al., 2016).
Under low ambient temperature, the energy requirements increased dramatically (Chmielowiec-Korzeniowska et al., 2012). Non-shivering thermogenesis is important in cold-stressed pigs due to a lack of brown adipose tissue (White et al., 2015; Zhang et al., 2017). Herpin et al. (2005) noted that the muscles of pigs have high oxidative potential and oxygen consumption to increase heat production for body thermal regulation. Cold stress causes metabolic changes in the body and decreases the gain rate and feed efficiency of pigs as metabolic responses to cold conditions for heat production (Birkelo et al., 1991). Depending on the intensity of the exposure, cold stress can trigger different stress responses in cells. Sonna et al. (2002) indicated that more than 17 genes (apoptosis specific protein, IL-8, HSP25, ATPase, etc.) upregulated or downregulated under cold stress, and most were downregulated to adapt to the metabolic demands of the body. A study on puffer fish under cold conditions observed the upregulation of bile salt export pumps involved in the transport of oligosaccharides (D-maltose and maltotriose) which are beneficial for energy requirement (Wen et al., 2019). The muscle gene expression profile of pigs suffering cold stress showed that the differently expressed genes were mainly distributed in the cytoplasm, mitochondrion, organelle envelope, and ribosomal subunit and enriched pathways primarily in ribosomes, fatty acid metabolism, RNA transport, and metabolic pathways (Zhang et al., 2017). Under cold conditions, the activity of the sympathetic system and hormones regulates the physiology and metabolism of pigs (Young, 1981). Gebregeziabhear et al. (2015) reported that pigs in a negative condition had higher cortisol concentrations and lower growth rates. Nie et al. (2021) pointed out that stress hormones (epinephrine, norepinephrine, and glucocorticoids) are important for the metabolism of brown adipose tissue to maintain thermogenesis. The secretions of adrenal corticosteroids and some hormones stimulated by cold stress also affect the body immune function based on the differentiation, proliferation, or gene expression of immune cells (Zhang et al., 2017). Moreover, cold conditions change the hair coat, surface tissues, appetite, and digestive function (Young, 1981). Many cellular processes are regulated at the level of metabolites, such as cell signaling, energy transfer, and intercellular communication. Metabolites reflect the environment of cells and are closely related to the nutritional status of cells, the role of drugs and environmental pollutants, and other external factors.
Metabonomics is an important technique for systems biology and involved the measurement of metabolites that are the end products of biological processes. High-throughput detection and data processing have detected the low molecular weight metabolites of a certain organism or cell in a specific physiological period, and some of these data have been indexed in databases. A metabonomic study on pig cecal content revealed that short-term cold stress changed the concentration of certain metabolite concentrations, and among the identified metabolites, creatinine and oxypurinol mainly occur in the blood and are related to physiological activities such as muscle contraction (Yang et al., 2021). In a study of rats suffering from cold stress, metabolomic profiling was performed, and important biochemical responses (e.g., tricarboxylic acid cycle, gut microbiota) to acute and chronic cold stress were identified (Wang et al., 2009).
The main objectives of this study were to characterize the metabolic profiles of the piglet liver under different cold exposure times and determine the effects of cold exposure times on the metabolome and metabolic pathways. The identification of different metabolites and pathways, especially at different times, is helpful for better understanding the adaptation process under different cold exposure conditions, and such data can be used for improving climate adaptability in pig production.
Sixteen 30-day-old male weaned piglets with similar weights (22.9 ± 0.2 kg) were randomly divided into four groups: a normal temperature group (24 ± 2°C, NT) and a cold exposure (4 ± 2°C) 2-h group (CS2), 6-h group (CS6), and 12-h group (CS12). The piglets in all groups were reared in artificial intelligence climate chambers for 7 days with a 12/12-h light/dark cycle (light from 8:00 a.m. to 8:00 p.m.). The temperature was maintained at 24 ± 2°C, and the relative humidity was 40–50%. Then, the piglets in the cold exposure groups were exposed to 4 ± 2°C conditions for 2, 6, or 12 h in artificial intelligence climate chambers, while the normal temperature control group was kept at 24°C. All piglets were fed the same commercial pellet feed and water, which were provided ad libitum. At the end of the experiment, all piglets were anesthetized and immediately euthanized. Liver samples (3–5 g) were then frozen with liquid nitrogen and stored in a -80 °C freezer until analysis by LC–MS metabonomics.
Liver samples (50 mg) were added to 1,000 μl of the extraction solution (methanol: acetonitrile: water = 2: 2: 1 (V/V), including 2 μg/ml of the internal standard L-2-chlorophenylalanine), vortexed, homogenized, and centrifuged at 13,800 ×g for 10 min at 4°C. Then, 400 μl of the supernatant was placed in an EP tube for vacuum drying, 200 μL of 50% acetonitrile was added for further dissolution, and then, the samples were vortexed and centrifuged at 16,200 ×g for 15 min at 4°C. Then, 75 μL of the supernatant was added to the sampler bottle (Dunn et al., 2011).
The supernatant in the sampler bottle was analyzed using a 1,290 Infinity series UHPLC System equipped with an UPLC BEH Amide column (2.1 × 100 mm, 1.7 μm, Waters) (Agilent Technologies) with a mobile phase comprising 25 mmol L−1 ammonium acetate and 25 mmol L−1 ammonia hydroxide in water (pH = 9.75) (A) and acetonitrile (B). The analysis was carried out with an elution gradient as follows: 0 ∼ 0.5 min, 95% B; 0.5 ∼ 7.0 min, 95 ∼ 65% B; 7.0 ∼ 8.0 min, 65 ∼ 40% B; 8.0 ∼ 9.0 min, 40% B; 9.0 ∼ 1 min, 40 ∼ 95% B; and 9.1 ∼ 12.0 min, 95% B. The column temperature was 25°C, and the injection volume was 2 μL (positive) or 2 μL (negative).
A triple TOF 6600 mass spectrometer (AB Sciex) was used to acquire MS/MS spectra on an information-dependent basis (IDA). The acquisition software (Analyst TF1.7, AB Sciex) continuously evaluates the full-scan survey MS data as it collects and triggers the acquisition of MS/MS spectra depending on preselected criteria. The ESI source conditions were as follows: Gas 1 was 60 psi, Gas 2 was 60 psi, curtain gas was 35 psi, source temperature was 600°C, declustering potential was 60 V, and ion spray voltage floating (ISVF) was 5000 V or -4000 V in positive or negative modes, respectively.
MS raw data files were converted to the mzXML format by ProteoWizard and processed by the R package XCMS (version 3.2). The process includes peak deconvolution, alignment, and integration. MinFrac and cut off were set as 0.5 and 0.3, respectively. The in-house MS2 database (based on HMDB, PubChem, KEGG, and METLIN) was applied for metabolite identification.
Non-targeted metabolomics data from the piglet liver were collected by a UHPLC-QTOF-MS analysis in positive and negative modes. In total, 993 metabolite ion features were detected in the liver samples in positive and negative ion modes.
To obtain an overview of the chemical changes in the piglet liver during different cold exposure durations, the data were analyzed by unsupervised PCA. The PCA scores are shown in Figure 1. The data showed one principal component (PC) (PC1), two PCs (PC1 and PC2), and three PCs (PC1, PC2, and PC3), which accounted for 37.0, 59.2,, and 70.3% of the variation, respectively.
FIGURE 1. PCA score plots of different cold exposure duration groups. Note: NT=normal temperature group (24±2°C), CS2=cold exposure (4±2°C) 2-hour group, CS6=cold exposure 6-hour group, and CS12=cold exposure 12-hour group; NT1 ∼ 4 in the figure corresponded to the four samples of the NT group (24±2°C), similar to the CS2, CS6, and CS12 groups.
To further verify the significant difference between the control and cold exposure groups, we used the supervised OPLS-DA multivariate method for each of the two comparisons. The OPLS-DA score plots indicate the good fitness and high predictability of the model, with high statistical values of R2Y and Q2. The parameters of R2Y and Q2Y for CS2-NT, CS6-NT, and CS12-NT were 0.991 and 0.27, 0.997 and 0.379, and 0.999 and 0.482, respectively (Figures 2A,C,E). The permutation test results showed that all Q2 points from left to right were lower than the original red Q2 points on the right side, indicating that the model was robust and reliable without overfitting (Figures 2B,D,F). The control and cold exposure groups could be clearly differentiated, suggesting that the metabolic profiles changed significantly and could be exploited in the subsequent analysis.
FIGURE 2. OPLS-DA score plot diagrams (A), NT vs CS2; (C), NT vs CS6; (E), NT vs CS12 and permutation test results (B), NT vs CS2; (D), NT vs CS6; (F), NT vs CS12.
According to the supervised manipulations, potential variables were chosen as differentially abundant metabolites based on VIP >1, fold change >2, and p < 0.05 ((Thévenot et al., 2015)). There were 10 differentially abundant metabolites (6 upregulated and 4 downregulated) in the CS2 group, 4 upregulated differentially abundant metabolites in the CS6 group, and 10 differentially abundant metabolites (6 upregulated and 4 downregulated) in the CS12 group compared with the control group (NT) (Table 1 and Figure 3).
FIGURE 3. Volcano plots of the NT and CS groups based on spectral data from the ion modes (A), NT vs CS2; (B), NT vs CS6; (C), NT vs CS12. Note: one point in the graph corresponds to a metabolite, and the size of the points represents the VIP value of the OPLS-DA model. Red indicates upregulated metabolites, blue indicates downregulated metabolites, black indicates metabolites that were not significantly different between the CS and NT groups.
Among the NT and cold exposure groups, there were 8 known differentially abundant metabolites, which were primarily important intermediate metabolites for body metabolism.
Isocitrate is important as an electron donor for NADP+-dependent isocitrate dehydrogenase to reduce NADP+ to NADPH (Minich et al., 2003). In mitochondria, NADPH is critical for antioxidant defense to reduce the byproducts of respiration (Lin et al., 2011). Cold stress may elevate the metabolic rate and induce high reactive oxygen species levels (Şahin and Gümüşlü, 2004). In our study, the isocitrate level was downregulated in the 2-h cold exposure group compared with the NT group; however, its level did not show a change in the 6- and 12-h cold exposure groups compared with the NT group. The different isocitrate levels showed that 2 h of cold exposure may result in oxidative damage to the cells, although the injured state could be relieved with additional time for metabolic adjustments of the body. A cold stress study on rats showed similar changes, with decreases observed in the urinary excretion levels of isocitrate in Sprague–Dawley rats exposed to -10 °C for 2 h (Wang et al., 2007). However, an alternative 4°C treatment and a normal temperature treatment of Wistar rats for 2 weeks did not result in changes in plasma isocitrate (Yang et al., 2015). The different effects are probably due to the different cold stress times or conditions.
FPP is endogenously synthesized and represents a key intermediate for the biosynthesis of steroids, carotenoids, and polyisoprenoids. G protein-coupled receptors (GPRs) are cell surface receptors that play varied roles in pathophysiological processes by transmitting cell signals (Oh et al., 2008). In GPR92-expressing cells, FPP directly interacts with GPR92 and induces immediate responses, such as Ca2+ mobilization, inositol phosphate and cAMP accumulation, and phospho-ERK elevation (Oh et al., 2008). In our study, FPP was upregulated in the 2-h cold exposure group compared with the NT group, which was probably related to the ability of FPP to accelerate body metabolism at the first stage of cold stress. Moreover, FPP is positively related to the biosynthesis of some non-steroidal isoprenes that are important for intracellular antioxidants (Qi et al., 2010).
PEA has weak sympathomimetic traits, stimulates motor activity, and is a potentially common mediator of amphetamine and stress (Durden et al., 1973). In humans and rats, stress increases the impaired excretion of PEA (Snoddy et al., 1985). At the beginning of cold stress, it was speculated that depression led to the impaired excretion of PEA, which caused the downregulation of PEA in the 2-h cold exposure group compared with the NT group. Along with the improvement of depression, the pigs showed tolerance toward cold stress. Therefore, there was no difference in PEA between the 6- or 12-h cold exposure groups and the NT group.
Citric acid is a component of the Krebs cycle, and it is affected by many factors, such as stressors and hormone and dietary protein levels (Hill et al., 1961). Hill et al. (1961) noted that the citric acid levels in the blood increased when chickens were subjected to heat stress, cold stress, starvation stress, or ACTH administration; however, the citric acid concentration did not show these changes if the dietary protein level was lower than 10%, which probably reflects that a protein or some amino acids are required for synthetases related to citric acid (Hill and Baker, 1961). Studies on rabbits showed that administering citrate was beneficial to improving the antioxidant function of the liver and reduced the effects of cold and heat stress, although changes in acupuncture stress were not observed (Liao et al., 1980). In the present study, the level of citric acid was upregulated only in the 6-h cold exposure group compared with the NT group, which may be associated with the effects of different cold conditions. The pigs suffered longer cold stress times (24, 48 h, and 5 days), and variations in the citric acid level were not detected (Zhang et al., 2017; Zhang et al., 2020). Marai and Habeeb (2010) noted that the citric acid contents also decreased in the milk of Friesian cows and semen of buffalos under high-temperature conditions.
Maltotriose is a product of starch digestion and can enhance energy metabolism. Maltose and maltotriose are the first carbohydrates accumulated under cold conditions, followed by the others (hexose phosphates, fructose-6-phosphate, glucose-6-phosphate, galactose-6-phosphate, and mannose-6-phosphate) (Purdy et al., 2011). A cold stress response study on juvenile pufferfish showed that the liver maltotriose level was upregulated at 12°C for 24 h compared with that in the fish at 26°C (Wen et al., 2019). However, juvenile yellow drums did not show the same change under cold stress, and the liver maltotriose level of fish in 8°C water for 2 weeks was not upregulated or downregulated compared with that of fish in 16°C water (Jiao et al., 2020). Moreover, the liver maltotriose level of starved yellow drums was downregulated compared with that of yellow drums fed under normal temperature conditions (Jiao et al., 2020). In our study, the liver maltotriose of the pigs did not show differences between the 2- or 6-h groups and the NT group; however, the liver maltotriose level of pigs in the 12-h group was downregulated. The reason for these findings may be the non-acute cold condition. However, the body starch level decreased with the cold exposure duration, which caused a decrease in maltotriose, and this change may affect energy metabolism.
Epinephrine is secreted by the adrenal gland, and it is necessary for appropriate responses to various stressors and is beneficial for metabolic regulation in the body. Heat stress increases the body maintenance requirements in rodents, poultry, sheep, and cattle and elevates the level of epinephrine (Baumgard and Rhoads, 2013). Pigs at 18 weeks in the heat stress group (37°C for 9 h and 23°C for 15 h) had higher epinephrine concentrations than those in the control group (23°C for 24 h) (Lan and Kim, 2018). However, the plasma epinephrine level of large white pigs (6 h of age) at 25°C (cold) was not significantly different from that of newborn pigs at 34°C (thermoneutral) for 48 h (Berthon et al., 1996). The plasma epinephrine level of large white pigs aged 6–10 weeks was not affected by cold stress. The change in epinephrine levels is likely linked to severe stress (Barrand et al., 1981; Young, 1981). Ambient temperatures of less than 20°C have a significant effect on the sympathetic adrenal system of humans. Under severe stress (<15°C), functional alterations were observed in the adrenal medulla (epinephrine). Acute cold stress increased plasma epinephrine concentrations of shorn sheep by two- and five-fold and increased the urinary excretion of epinephrine (Young, 1981). In our study, the liver epinephrine level did not show a difference between the 2/6-h group and the NT group; however, the epinephrine level in the 12-h group was upregulated. These findings may not have been caused by the acute cold conditions but rather by the decreased body energy metabolism under increased cold exposure time.
Except for the differential metabolites between the cold exposure groups and the control, many of the identified metabolites did not show differences; however, some differential metabolites have been observed under different cold stress conditions in previous studies. For example, deoxyuridine, indoleacetic acid, histidine, phenylacetylglycine, enterostatin, methoxyacetic acid, dihydroxyfumarate, creatinine, and oxypurinol were identified as differential metabolites in the serum or cecal mucosa samples under long-term cold stress conditions (Zhang et al., 2020; Yang et al., 2021). Researchers have pointed out that metabolites are mainly associated with the physiological activities of energy metabolism and muscle contraction (Zhang et al., 2020; Yang et al., 2021).
The metabolic pathways of differentially abundant metabolites were explored using the KEGG database. The pathway enrichment analysis of metabolites was performed via the R package clusterProfiler. The different metabolic pathways between the NT and CS groups are presented in Figure 4, and network maps of metabolites and metabolic pathways between the NT and CS groups are shown in Figure 5.
FIGURE 4. KEGG pathway-enriched dot plots of differentially abundant metabolites. (A), NT vs CS2; (B), NT vs CS6; and (C), NT vs CS12.
FIGURE 5. KEGG pathway-enriched cnetplots of differentially abundant metabolites. (A), NT vs CS2; (B), NT vs CS6; and (C), NT vs CS12. (meta_15: farnesy1 pyrophosphate, meta_264: sphingosine-1-phosphate, and meta_505: epinephrine,).
Metabolic pathway analysis is very important for metabonomics and useful for identifying the metabolic signals and pathways of metabolites and exploring the related metabolites and genes (Li et al., 2019). There were eight main differentially abundant metabolites involved in three KEGG pathway classifications (metabolism, organismal systems, and environmental information processing) between the NT group and the cold exposure groups. Compared with the NT group, the differentially abundant metabolites of the CS2 group participated in 20 metabolic or biosynthetic pathways in the body; those of the CS6 group participated in 17 metabolic or biosynthetic pathways, and those of the CS12 group participated in 11 metabolic or biosynthetic pathways.
Metabolic pathways and enrichment analyses revealed that most of the altered pathways were related to body metabolism, such as 2-oxocarboxylic acid metabolism, alanine, aspartate and glutamate metabolism, biosynthesis of amino acids, citrate cycle (TCA cycle), glycerophospholipid metabolism, glyoxylate and dicarboxylate metabolism, phenylalanine metabolism, purine metabolism, steroid biosynthesis, terpenoid backbone biosynthesis, and tyrosine metabolism. Cold stress affects the body’s ability to adjust its metabolic functions to adapt to abnormal environmental conditions. Changes in body metabolism were also observed in chickens, pigs, fish, and other animals and plants under cold stress conditions (Lu, et al., 2018; Pan et al., 2018; Nie, et al., 2018; Song et al., 2019; Wang, et al., 2018; Zhou, et al., 2018).
In the present study, compared with the NT group, the most affected metabolic pathway among the three cold exposure groups was associated with amino acid metabolism, which indicated greater body amino acid mobilization and requirements. A study on tilapia also showed that cold stress significantly changed the amino acid levels and classes connected with the metabolism (Li et al., 2020). Zhou et al. (2011) reported that the concentration of five free amino acids in the hepatopancreas increased directly with decreasing temperature. Amino acid metabolism is closely related to a variety of proteins in the body, and it is conducive to maintaining the protein integrity and function and enhancing the adaptation of the body to cold environments. An early study on protein–concentration and environment–temperature interactions reported that pigs showed an increased feed intake at environmental temperatures below 16.5°C and a slight reduction in weight gain (Seymour et al., 1964). Henry (1985) reported that exposure to a cold environment increases the energy requirement of pigs for thermoregulation by stimulating the food intake, which also increases the daily amino acid and protein intake and improves the body amino acid and protein balance during cold stress.
In the current study, the 2-h and 6-h cold exposure groups shared 11 similar pathways (except two pathways), which revealed that these pathways are strongly correlated with short-term cold stress and that the pigs under 2-h and 6-h cold conditions had similar metabolic processes. Most of the pathways were connected with isocitrate (2-h exposure; downregulation) and citric acid (6-h exposure; upregulation), which are important for body energy metabolism. The downregulation of isocitrate and the altered pathways probably contributed to energy consumption under sudden and 2-h cold stimulation. With the extension of cold adaptation and the upregulation of citric acid, the body adapts to meet the needs of energy metabolism to some extent. The altered metabolic pathways of the 12-h cold exposure group were almost different from those of the 2-h and 6-h groups, and most of the pathways were related to epinephrine (tyrosine metabolism, adrenergic signaling in cardiomyocytes, regulation of lipolysis in adipocytes, renin secretion, cAMP signaling pathway, and neuroactive ligand–receptor interaction). Epinephrine is a hormone secreted mainly by the adrenal medulla. Its main function is to increase the cardiac output and blood glucose levels. Studies have shown that cold stress promotes its secretion to adjust the body metabolism (Nachankar et al., 2005). Epinephrine is also related to increased heat production. The upregulation of epinephrine during 12 h of cold exposure altered the relevant pathway and contributed to the adjustment of energy metabolism. Moreover, high-quality and adequate diets are beneficial for improving body energy and protein metabolism and minimizing the risk of diseases under cold exposure, especially for long-term cold exposure (da Silva et al., 2019).
Cold stress can change the body metabolism and impair the immune system (LaVoy et al., 2011). Under cold conditions, more effective heat conservation mechanisms can be developed in response to cold stimuli by adaptive training compared to those under acute or initial cold exposure (Castellani and Young, 2016).
The results revealed that the metabolite profiles of piglet livers differed under different cold exposure times, and the metabolic pathways were also different. Eight known differentially abundant metabolites were observed between the CS groups and the control group. The KEGG analysis showed that the metabolic pathways of related metabolites were more related to carbohydrates under 2-h and 6-h cold exposure and more related to epinephrine under 12 h of cold exposure.
The original contributions presented in the study are included in the article/Supplementary Material; further inquiries can be directed to the corresponding author.
The animal study was reviewed and approved by the Animal Care and Use Committee on Animal Ethics of Heilongjiang Bayi Agricultural University.
Conceptualization, YoC and LZ; methodology, LZ; software, HJ and WL; validation, HJ and YaC; formal analysis, YoC; investigation, JG and WL; resources, SW; data curation, YoC and YaC; writing—original draft preparation, YoC; writing—review and editing, JG; visualization, YaC and WL; supervision, HJ, SW, and LZ; project administration, LZ; funding acquisition, SW, LZ, and YoC. All authors have read and agreed to the submitted version of the manuscript.
This study was supported by the Heilongjiang Bayi Agricultural University Startup Fund Project (XDB201805), the Jiangxi Key Research and Development Program (20201BBF61005), the Heilongjiang Bayi Agricultural University Support Program for San Heng San Zong (ZRCPY201906), the Key R&D plan of Hunan Science and technology innovation plan project (2022NK 2041), and the Natural Science Foundation of Changsha City (kq2014173).
The authors declare that the research was conducted in the absence of any commercial or financial relationships that could be construed as a potential conflict of interest.
All claims expressed in this article are solely those of the authors and do not necessarily represent those of their affiliated organizations, or those of the publisher, the editors, and the reviewers. Any product that may be evaluated in this article, or claim that may be made by its manufacturer, is not guaranteed or endorsed by the publisher.
Barrand M. A., Dauncey M. J., Ingram D. L. (1981). Changes in Plasma Noradrenaline and Adrenaline Associated with central and Peripheral thermal Stimuli in the Pig. J. Physiol. 316, 139–152. doi:10.1113/jphysiol.1981.sp013778
Baumgard L. H., Rhoads R. P. (2013). Effects of Heat Stress on Postabsorptive Metabolism and Energetics. Annu. Rev. Anim. Biosci. 1, 311–337. doi:10.1146/annurev-animal-031412-103644
Berthon D., Herpin P., Bertin R., De Marco F., Le Dividich J. (1996). Metabolic Changes Associated with Sustained 48-hr Shivering Thermogenesis in the Newborn Pig. Comp. Biochem. Physiol. B: Biochem. Mol. Biol. 114 (4), 327–335. doi:10.1016/0305-0491(96)00044-2
Castellani J. W., Young A. J. (2016). Human Physiological Responses to Cold Exposure: Acute Responses and Acclimatization to Prolonged Exposure. Auton. Neurosci. 196, 63–74. doi:10.1016/j.autneu.2016.02.009
Chmielowiec-Korzeniowska A., Tymczyna L., Babicz M. (2012). Assessment of Selected Parameters of Biochemistry, Hematology, Immunology and Production of Pigs Fattened in Different Seasons. Arch. Anim. Breed. 55, 469–479. doi:10.1271/bbb.64.172910.5194/aab-55-469-2012
Dong-jie Z., Di L., Liang W., Wen-tao W., Xin-miao H., Guo-wei Y. (2017). Gene Expression Profile Analysis of Pig Muscle in Response to Cold Stress. J. Appl. Anim. Res. 45, 195–198. doi:10.1080/09712119.2015.1129338
Dunn W. B., Broadhurst D., Broadhurst D., Begley P., Zelena E., Francis-McIntyre S., et al. (2011). Procedures for Large-Scale Metabolic Profiling of Serum and Plasma Using Gas Chromatography and Liquid Chromatography Coupled to Mass Spectrometry. Nat. Protoc. 6 (7), 1060–1083. doi:10.1038/nprot.2011.335
Durden D. A., Philips S. R., Boulton A. A. (1973). Identification and Distribution of β-Phenylethylamine in the Rat. Can. J. Biochem. 51, 995–1002. doi:10.1139/o73-129
Gebregeziabhear E., Ameha N., Zeit D., Dawa D. (2015). The Effect of Stress on Productivity of Animals: a Review. J. Biol. Agric. Healthc. 5 (3), 165–172.
Henry Y. (1985). Dietary Factors Involved in Feed Intake Regulation in Growing Pigs: a Review. Livestock Prod. Sci. 12, 339–354. doi:10.1016/0301-6226(85)90133-2
Herpin P., Louveau I., Damon M., Le Dividich J. (2005). Chapter 14 Environmental and Hormonal Regulation of Energy Metabolism in Early Development of the Pig. Biol.Grow. Anim. 3, 351–374. doi:10.1016/S1877-1823(09)70021-9
Hill C. H., Baker V. C. (1961). Dietary Protein Levels as They Affect Blood Citric Acid Levels of Chicks Subjected to Certain Stresses. Poult. Sci. 40, 762–765. doi:10.3382/ps.0400762
Hill C. H., Warren M. K., Garren H. W., Baker V. C. (1961). Blood Citric Acid Concentration as Affected by Heat and Cold Stress and Adrenocorticotrophic Hormone. Poult. Sci. 40, 422–424. doi:10.3382/ps.0400422
Jiao S., Nie M., Song H., Xu D., You F. (2020). Physiological Responses to Cold and Starvation Stresses in the Liver of Yellow Drum (Nibea Albiflora) Revealed by LC-MS Metabolomics. Sci. Total Environ. 715, 136940. doi:10.1016/j.scitotenv.2020.136940
Lan R., Kim I. (2018). Effects of Feeding Diets Containing Essential Oils and Betaine to Heat-Stressed Growing-Finishing Pigs. Arch. Anim. Nutr. 72, 368–378. doi:10.1080/1745039X.2018.1492806
LaVoy E. C. P., McFarlin B. K., Simpson R. J. (2011). Immune Responses to Exercising in a Cold Environment. Wilderness Environ. Med. 22, 343–351. doi:10.1016/j.wem.2011.08.005
Leite da Silva A., dos Santos S. G. C. G., Saraiva E. P., Fonsêca V. d. F. C., Givisiez P. E. N., Pascoal L. A. F., et al. (2019). Supplementation of Diets with Glutamine and Glutamic Acid Attenuated the Effects of Cold Stress on Intestinal Mucosa and Performance of Weaned Piglets. Anim. Prod. Sci. 59 (10), 1880–1885. doi:10.1071/AN17630
Li B. J., Zhu Z. X., Qin H., Meng Z. N., Lin H. R., Xia J. H. (2020). Genome-wide Characterization of Alternative Splicing Events and Their Responses to Cold Stress in tilapia. Front. Genet. 11, 244. doi:10.3389/fgene.2020.00244
Li G., Gao W., Xu Y., Xie M., Tang S., Yin P., et al. (2019). Serum Metabonomics Study of Pregnant Women with Gestational Diabetes Mellitus Based on LC-MS. Saudi J. Biol. Sci. 26, 2057–2063. doi:10.1016/j.sjbs.2019.09.016
Liao Y.-Y., Seto K., Saito H., Kawakami M. (1980). Effects of Acupuncture on the Citrate and Glucose Metabolism in the Liver under Various Types of Stress. Am. J. Chin. Med. 08 (04), 354–366. doi:10.1142/S0192415X80000335
Lin J. F., Wu S., Huang S. S., Lu B. Y., Lin S. M., Tsai S. K. (2012). Resveratrol Protects Left Ventricle by Increasing Adenylate Kinase 1 and Isocitrate Dehydrogenase Activities in Rats with Myocardial Infarction. Chin. J. Physiol. 54, 406–412. doi:10.4077/CJP.2011.AMM097
Lu Z., He X., Ma B., Zhang L., Li J., Jiang Y., et al. (2018). Serum Metabolomics Study of Nutrient Metabolic Variations in Chronic Heat-Stressed Broilers. Br. J. Nutr. 119, 771–781. doi:10.1017/S0007114518000247
Marai I. F., Haeeb A. A. M. (2010). Buffaloes' Reproductive and Productive Traits as Affected by Heat Stress. Trop. Subtrop. Agroecos. 12, 193–217.
Minich T., Yokota S., Dringen R. (2003). Cytosolic and Mitochondrial Isoforms of NADP+-dependent Isocitrate Dehydrogenases Are Expressed in Cultured Rat Neurons, Astrocytes, Oligodendrocytes and Microglial Cells. J. Neurochem. 86, 605–614. doi:10.1046/j.1471-4159.2003.01871.x
Muns R., Nuntapaitoon M., Tummaruk P. (2016). Non-infectious Causes of Pre-weaning Mortality in Piglets. Livestock Sci. 184, 46–57. doi:10.1016/j.livsci.2015.11.025
Nachankar R. S., Juvekar A. R., Sonawane A. (2005). Prevention of Cold Stress Induced Adrenal Hypertrophy by Spirulina Platensis. Acta Hortic. 680, 101–107. doi:10.17660/ActaHortic.2005.680.14
Nie X., Lei J., Chen S., Zhang Y., Zhang C., Hong W. (2018). Physiological, Proteomic, and Gene Expression Analysis of Turbot (Scophthalmus maximus) in Response to Cold Acclimation. Aquaculture 495, 281–287. doi:10.1016/j.aquaculture.2018.05.054
Oh D. Y., Yoon J. M., Moon M. J., Hwang J.-I., Choe H., Lee J. Y., et al. (2008). Identification of Farnesyl Pyrophosphate and N-Arachidonylglycine as Endogenous Ligands for GPR92. J. Biol. Chem. 283, 21054–21064. doi:10.1074/jbc.M708908200
Pan L., Meng C., Wang J., Ma X., Fan X., Yang Z., et al. (2018). Integrated Omics Data of Two Annual Ryegrass (Lolium Multiflorum L.) Genotypes Reveals Core Metabolic Processes under Drought Stress. BMC Plant Biol. 18, 1–18. doi:10.1186/s12870-018-1239-z
Purdy S. J., Bussell J. D., Nelson D. C., Villadsen D., Smith S. M. (2011). A Nuclear-Localized Protein, KOLD SENSITIV-1, Affects the Expression of Cold-Responsive Genes during Prolonged Chilling in Arabidopsis. J. Plant Physiol. 168, 263–269. doi:10.1016/j.jplph.2010.07.001
Qi X.-F., Kim D.-H., Yoon Y.-S., Kim S.-K., Cai D.-Q., Teng Y.-C., et al. (2010). Involvement of Oxidative Stress in Simvastatin-Induced Apoptosis of Murine Ct26 colon Carcinoma Cells. Toxicol. Lett. 199, 277–287. doi:10.1016/j.toxlet.2010.09.010
Sahin E., Gümüslü S. (2004). Cold-stress-induced Modulation of Antioxidant Defence: Role of Stressed Conditions in Tissue Injury Followed by Protein Oxidation and Lipid Peroxidation. Int. J. Biometeorology 48 (4), 165–171. doi:10.1007/s00484-004-0205-7
Seymour E. W., Speer V. C., Hays V. W., Mangold D. W., Hazen T. E. (1964). Effects of Dietary Protein Level and Environmental Temperature on Performance and Carcass Quality of Growing-Finishing Swine. J. Anim. Sci. 23, 375–379. doi:10.2527/jas1964.232375x
Snoddy A. M., Heckathorn D., Tessel R. E. (1985). Cold-restraint Stress and Urinary Endogenous β-phenylethylamine Excretion in Rats. Pharmacol. Biochem. Behav. 22, 497–500. doi:10.1016/0091-3057(85)90054-1
Song M., Zhao J., Wen H.-S., Li Y., Li J.-F., Li L.-M., et al. (2019). The Impact of Acute thermal Stress on the Metabolome of the Black Rockfish (Sebastes Schlegelii). PloS one 14 (5), e0217133. doi:10.1371/journal.pone.0217133
Sonna L. A., Fujita J., Gaffin S. L., Lilly C. M. (2002). Invited Review: Effects of Heat and Cold Stress on Mammalian Gene Expression. J. Appl. Physiol. 92, 1725–1742. doi:10.1152/japplphysiol.01143.2001
Thévenot E. A., Roux A., Xu Y., Ezan E., Junot C. (2015). Analysis of the Human Adult Urinary Metabolome Variations with Age, Body Mass index, and Gender by Implementing a Comprehensive Workflow for Univariate and OPLS Statistical Analyses. J. Proteome Res. 14, 3322–3335. doi:10.1021/acs.jproteome.5b00354
Wang Q., Liu Y., Zheng Z., Deng Y., Jiao Y., Du X. (2018). Adaptive Response of Pearl Oyster Pinctada Fucata Martensii to Low Water Temperature Stress. Fish Shellfish Immunol. 78, 310–315. doi:10.1016/j.fsi.2018.04.049
Wang X., Su M., Qiu Y., Ni Y., Zhao T., Zhou M., et al. (2007). Metabolic Regulatory Network Alterations in Response to Acute Cold Stress and Ginsenoside Intervention. J. Proteome Res. 6, 3449–3455. doi:10.1021/pr070051w
Wang X., Zhao T., Qiu Y., Su M., Jiang T., Zhou M., et al. (2009). Metabonomics Approach to Understanding Acute and Chronic Stress in Rat Models. J. Proteome Res. 8 (5), 2511–2518. doi:10.1021/pr801086k
Wen X., Hu Y., Zhang X., Wei X., Wang T., Yin S. (2019). Integrated Application of Multi-Omics Provides Insights into Cold Stress Responses in Pufferfish Takifugu Fasciatus. BMC genomics 20, 1–15. doi:10.1186/s12864-019-5915-7
White R. R., Miller P. S., Hanigan M. D. (2015). Evaluating Equations Estimating Change in Swine Feed Intake during Heat and Cold Stress1. J. Anim. Sci. 93, 5395–5410. doi:10.2527/jas.2015-9220
Yang Y., Chen N., Sun L., Zhang Y., Wu Y., Wang Y., et al. (2021). Short-term Cold Stress Can Reduce the Abundance of Antibiotic Resistance Genes in the Cecum and Feces in a Pig Model. J. Hazard. Mater. 416, 125868. doi:10.1016/j.jhazmat.2021.125868
Yang Y., Wang Y., Zhang J., Han Z., Chen A., Pan S., et al. (2015). System Responses to Chronic Cold Stress Probed via1H NMR Spectroscopy in Plasma and Urine Matrices. Mol. Biosyst. 11, 1425–1433. doi:10.1039/C5MB00033E
Young B. A. (1981). Cold Stress as it Affects Animal Production. J. Anim. Sci. 52, 154–163. doi:10.2527/jas1981.521154x
Zhang S., Gao H., Yuan X., Wang J., Zang J. (2020). Integrative Analysis of Energy Partition Patterns and Plasma Metabolomics Profiles of Modern Growing Pigs Raised at Different Ambient Temperatures. Animals 10 (11), 1953. doi:10.3390/ani10111953
Zhou M., Wang A.-L., Xian J.-A. (2011). Variation of Free Amino Acid and Carbohydrate Concentrations in white Shrimp, Litopenaeus Vannamei: Effects of Continuous Cold Stress. Aquaculture 317 (1-4), 182–186. doi:10.1016/j.aquaculture.2011.04.033
Keywords: piglets, non-targeted metabolomics, cold exposure, LC–MS, liver
Citation: Chen Y, Ji H, Guo J, Chen Y, Li W, Wang S and Zhen L (2022) Non-targeted Metabolomics Analysis Based on LC–MS to Assess the Effects of Different Cold Exposure Times on Piglets. Front. Physiol. 13:853995. doi: 10.3389/fphys.2022.853995
Received: 13 January 2022; Accepted: 07 March 2022;
Published: 05 April 2022.
Edited by:
Gregoire P Millet, University of Lausanne, SwitzerlandReviewed by:
Xiaoshang Ru, Key Laboratory of Marine Ecology and Environmental Sciences (CAS), ChinaCopyright © 2022 Chen, Ji, Guo, Chen, Li, Wang and Zhen. This is an open-access article distributed under the terms of the Creative Commons Attribution License (CC BY). The use, distribution or reproduction in other forums is permitted, provided the original author(s) and the copyright owner(s) are credited and that the original publication in this journal is cited, in accordance with accepted academic practice. No use, distribution or reproduction is permitted which does not comply with these terms.
*Correspondence: Shengping Wang, NDQyNjI5MjY1QHFxLmNvbQ==; Li Zhen, Y3Jvc3MtbW9vbkAxNjMuY29t
Disclaimer: All claims expressed in this article are solely those of the authors and do not necessarily represent those of their affiliated organizations, or those of the publisher, the editors and the reviewers. Any product that may be evaluated in this article or claim that may be made by its manufacturer is not guaranteed or endorsed by the publisher.
Research integrity at Frontiers
Learn more about the work of our research integrity team to safeguard the quality of each article we publish.