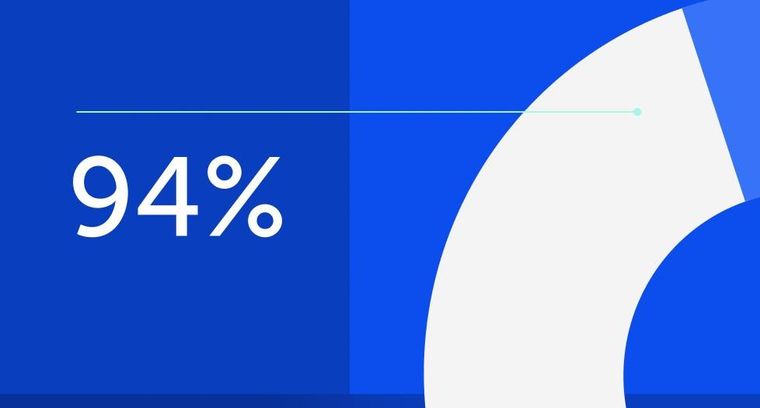
94% of researchers rate our articles as excellent or good
Learn more about the work of our research integrity team to safeguard the quality of each article we publish.
Find out more
REVIEW article
Front. Physiol., 03 March 2022
Sec. Redox Physiology
Volume 13 - 2022 | https://doi.org/10.3389/fphys.2022.840293
This article is part of the Research TopicAdvances in Metabolic Mechanisms of Aging and Its Related Diseases, Volume IIView all 5 articles
Osteoporosis is a common bone imbalance disease that threatens the health of postmenopausal women. Estrogen deficiency accelerates the aging of women. Oxidative stress damage is regarded as the main pathogenesis of postmenopausal osteoporosis. The accumulation of reactive oxygen species in the bone microenvironment plays a role in osteoblast and osteoclast apoptosis. Improving the oxidative state is essential for the prevention and treatment of postmenopausal osteoporosis. There are three classes of antioxidant defense systems in the body to eliminate free radicals and peroxides including antioxidant substances, antioxidant enzymes, and repair enzymes. In our review, we demonstrated the mechanism of antioxidants and their effect on bone metabolism in detail. We concluded that glutathione/oxidized glutathione (GSH/GSSG) conversion involved the PI3K/Akt-Nrf2/HO-1 signaling pathway and that the antioxidant enzyme-mediated mitochondrial apoptosis pathway of osteoblasts was necessary for the development of postmenopausal osteoporosis. Since the current therapeutic effects of targeting bone cells are not significant, improving the systemic peroxidation state and then regulating bone homeostasis will be a new method for the treatment of postmenopausal osteoporosis.
Osteoporosis is a metabolic bone disease characterized by a decrease in bone mass per unit volume. Elderly and postmenopausal women are at high risk of osteoporosis (Srivastava and Deal, 2002). The thin cortical bone and sparse cancellous bone increase the risk of fractures in patients with osteoporosis, which seriously threaten public health and cause a huge social burden (Black and Rosen, 2016). At present, more studies have focused on osteoblasts and osteoclasts. Most of the drugs used to treat osteoporosis directly act on the process of bone formation and absorption, mainly inhibiting osteoclasts. Osteogenesis drugs are parathyroid hormone (PTH), prostaglandin E2 (PGE2), calcium, and vitamin D (Kabasawa et al., 2003). Drugs that inhibit osteoclasts include estrogen replacement treatment and bisphosphonates (Qaseem et al., 2017). Improving lifestyle and eating habits also helped prevent osteoporosis. These treatments are not fully satisfying due to limitations in bone microenvironmental regulation. They ignore the complex changes in the body caused by estrogen deficiency. Estrogen or selective estrogen receptor modulators have a better effect, although there are some limitations in indications (Mandelli et al., 2021). Therefore, to propose a more effective and widely applicable treatment method, fully analyzing the pathogenesis of postmenopausal osteoporosis and the pathological changes of the body is a necessary means.
Recent studies have shown that the pathogenesis of postmenopausal osteoporosis is mainly due to aging (Pignolo et al., 2021). Aging is believed to be caused by the accumulation of reactive oxygen species (ROS) (Davalli et al., 2016). ROS are generated by various organelles, especially mitochondria, through enzymatic and non-enzymatic reactions in cell metabolism (Zorov et al., 2014). ROS can cause DNA damage and protein denaturation, thereby causing gene mutations and affecting normal biological functions (Zhang et al., 2020a). Additionally, some ROS, such as oxygen-containing free radicals, are also regarded as inflammatory mediators that affect the microenvironment of the organism, leading to the occurrence of diseases (Forrester et al., 2018). Under physiological conditions, there are three classes of antioxidant defense systems in the human body to remove excess ROS and avoid oxidative damage, including antioxidant substances, antioxidant enzymes, and repair enzymes. In the detection of blood oxidative stress indicators in postmenopausal women, it was found that antioxidant indicators, including glutathione peroxidase (GSH-Px), folate, and superoxide dismutase, decreased significantly (Zhou et al., 2016). Estrogen could protect mitochondrial membrane potential through estrogen receptor beta (ERβ) (Simpkins et al., 2008). The antioxidant effect depended on decreasing the activity of NADPH oxidase via the angiotensin II (Ang II) pathway and reducing inducible nitric oxide synthase (iNOS) by enhancing NO activity (Miyazaki-Akita et al., 2007; Yung et al., 2011; Figure 1). Dysfunction of the antioxidant defense systems causes redox imbalance and leads to the body being in a state of peroxidation, which makes it difficult to remove ROS.
As mentioned above, the weakening of the body’s antioxidant capacity, leading to the accumulation of free radicals and inducing bone aging, is a necessary cause of postmenopausal osteoporosis. Improving antioxidant capacity and removing excess ROS will be an effective method for the systemic treatment of osteoporosis. Therefore, we reviewed the relationship between the three classes of antioxidant systems and the development of postmenopausal osteoporosis.
The essence of postmenopausal osteoporosis is weakened osteogenesis and increased osteoclastogenesis caused by the lack of estrogen. However, the pathogenesis remains unclear. With continuous exploration, researchers have paid more attention to aging accelerated by estrogen deficiency and have shown that oxidative stress damage is the pathogenesis of postmenopausal osteoporosis (Mohamad et al., 2020; Tu et al., 2020; Shahriarpour et al., 2021). The accumulation of ROS is regarded as an important factor in destroying bone homeostasis without estrogen protection (Mohamad et al., 2020). On the one hand, estrogen activates endothelium-derived hyperpolarizing factor (EDHF) to release NO and modulates NADPH oxidase involved in the Ang II process to inhibit ROS production in skeletal vascular endothelium (Silva, 2021; Youn et al., 2021). On the other hand, estrogen upregulates MnSOD activity and inhibits cellular ROS production (Oh et al., 2019). The main effect of ROS on osteoblasts is to induce the cell mitochondrial apoptosis pathway (Luo et al., 2021). ROS change the permeability of mitochondrial membranes and release internal apoptotic factors including cytochrome c (Cytc) and apoptosis-inducing factor (AIF) (Zhao et al., 2020a; Seminotti et al., 2021). These factors combine with apoptotic protease activating factor-1 (Apaf-1) and activate caspase-9 and caspase-3 in the cytoplasm, causing cell apoptosis (Wang et al., 2019). The positive effect of ROS on osteoclasts is to promote differentiation. ROS can activate three essential pathways involved in osteoclast differentiation including the MAPK, PI3K, and nuclear factor kappa-B (NF-κB) pathways (Thummuri et al., 2017; Zhou et al., 2020; Xiao et al., 2021). The activation of these pathways contributes to the expression of the osteoclast maturation genes CTSK, MMP9, and NFATC1 (Tao et al., 2020). In conclusion, there is an axis of estrogen deficiency/ROS accumulation/osteoblast apoptosis and osteoclast differentiation in postmenopausal osteoporosis (Figure 2).
The biosynthesis of glutathione (GSH) mainly reduces oxidized glutathione (GSSG) with glutathione reductase, assisted by NADPH produced by the pentose phosphate pathway (Fan et al., 2014). The sulfhydryl group (-SH) in GSH provides reducing hydrogen to give free radicals a pair of electrons, so that the free radicals lose their strong oxidizing and aggressive properties (Bánhegyi et al., 2003). Previous studies have shown that serum GSH levels were significantly reduced in osteoporotic rats (Yalin et al., 2012; Ameen et al., 2020). Increasing the GSH/GSSG ratio can attenuate the oxidative damage of ROS on osteoblasts via the PI3K/Akt-Nrf2 signaling pathways (Casati et al., 2020). Nrf2 is a nuclear factor that regulates gene encoding proteins involved in the response to injury and inflammation, including the production of free radicals (Süntar et al., 2021). When the PI3K/AKT pathway located on the cell membrane is activated, the signal is transmitted to the cytoplasm to release Nrf2 anchored by Kelch-like ECH-associated protein 1 (Keap1) (Chen et al., 2021a). After Nrf2 enters the nucleus, it forms a coactivator complex with the small Maf protein. This heterodimer binds to the promoter region of the antioxidant response element (ARE) to activate the expression of antioxidant genes (Shan et al., 2021). It has also been demonstrated that the activation of Nrf2 promotes osteogenic differentiation by increasing heme oxygenase-1 (HO-1) expression (Liu et al., 2018; Chen et al., 2020b). However, it is worth noting that the overexpression of Nrf2 might inhibit the differentiation of osteoblasts (Chen et al., 2021b). GSH inhibits the osteoclast differentiation-mediated NF-κB signaling pathway induced by ROS (Han et al., 2020). Nrf2 is considered to be a key factor in alleviating the formation of osteoclasts in inflammatory bone loss (Hong et al., 2021). Nrf2 also modulates NFATc1, the main transcription factor secreted by osteoclasts, to inhibit osteoclast differentiation (Sun et al., 2015). Therefore, the GSH/Nrf2-mediated antioxidant pathway is essential for the balance of osteogenesis and osteoclastogenesis.
Vitamin C is an antioxidant that can protect -SH and keep the -SH of sulfhydrylase in a reduced state. It reduces GSSG to GSH to remove lipid oxides from cell membranes with the assistance of glutathione reductase (Xu et al., 2020). Vitamin C, also known as ascorbic acid, is an important bone-promoting substance (Mizerska-Kowalska et al., 2019). It can be combined with β-glycerophosphate sodium and dexamethasone for the differentiation induction of osteoblasts (Dey et al., 2020). On the one hand, vitamin C can promote the expression of osteogenic genes including bone morphogenetic protein-2 (BMP2) and runt-related transcription factor 2 (Runx2) (Choi et al., 2019). On the other hand, it assists proline hydroxylase in promoting the maturation of collagen and the production of osteocalcin (OCN) in the bone matrix (Chojkier et al., 1983; Nielsen-Marsh et al., 2005).
The physiological function is mainly to resist free radicals produced by lipid peroxidation on biological membranes. However, the mechanism of vitamin E is to capture lipid peroxide free radicals and then to reduce them by glutathione or vitamin C, instead of directly acting as a reducing substance. For the study of vitamin E in the skeletal system, Vakili et al. (2021) found that vitamin E can improve bone mass in ovariectomized rats by inhibiting bone cell apoptosis and autophagy. However, compared with osteoblasts, vitamin E has a stronger inhibitory effect on osteoclasts. Receptor activator of NF-κB (RANK), receptor activator of NF-κB ligand (RANKL), osteoprotegerin (OPG), and Wnt/β-catenin signaling were involved in the process of inhibiting osteoclastogenesis (Wong et al., 2019). In addition to its direct effects on the skeletal system, vitamin E is also involved in inflammatory and immune responses and intervenes in bone metabolism by regulating bone-resorbing cytokines including interleukin-1 (IL-1) and IL-6 (Nazrun et al., 2012; Nazrun Shuid et al., 2019).
In a clinical trial, researchers administered vitamin C and E alone or in combination with patients with osteoporosis and found that the bone mass of the patients was significantly improved, and the serum antioxidant level was significantly increased (Chavan et al., 2007), which indicated that vitamins C and E are effective in the treatment of osteoporosis.
Melatonin is an endogenous antioxidant hormone secreted by the pineal gland. Melatonin can directly combine with reactive oxygen free radicals and reactive nitrogen-free radicals (Zhao et al., 2008). The combined product is chemically stable, and free radicals combined with melatonin cannot be regenerated. Our previous study revealed that intragastric melatonin could significantly improve bone mass in postmenopausal mice (Da et al., 2020). Melatonin could enhance osteogenic effects by increasing SIRT1 and SIRT3, the essential factors regulating antioxidant enzyme formation in mitochondria (Qiu et al., 2019; Zhou et al., 2019; Chen et al., 2020b). Melatonin also directly prevents ROS damage by eliminating lipid peroxide and lipopolysaccharide (LPS) (Yu and Tan, 2019; Hossain et al., 2020). In addition to alleviating oxidative stress damage, melatonin affected bone homeostasis by regulating the rhythm of the biological clock (Song et al., 2018). OCN and type I collagen (collagen I) showed a strong correlation with the melatonin rhythm, leading to bone remodeling destruction when circadian disturbances occurred, which was demonstrated in postmenopausal women (Munmun and Witt-Enderby, 2021). The direct relationship between melatonin and bone metabolism is closely related. Melatonin could induce osteogenic differentiation via the BMP/Wnt signaling pathway (Park et al., 2011). BMP proteins regulate the recruitment and activation of Smad family transcription factors (Zhao et al., 2020b). Then, the Wnt/β-catenin signaling pathway could be activated by upstream signal (Wang et al., 2020). β-catenin could promote the expression of osteogenic factors including Runx2, osterix, and type I collagen (Oh et al., 2021). At present, there are many clinical studies using melatonin as an auxiliary drug for the treatment of osteoporosis.
Fe2+ is very active and can react with oxygen to produce hydroxyl radicals and peroxide radicals and become Fe3+ with strong oxidizing properties (Obraztsov et al., 1975). Ferritin combines with Fe3+ to store iron and to relieve the oxidative damage of iron ions (Zhao et al., 2006). Ferritin is closely related to the development of postmenopausal osteoporosis. A serum test of 4,000 women found that serum ferritin is more closely related to bone density than iron intake and serum iron, which indicates that ferritin is a more reliable variable linking iron and osteoporosis (Lu et al., 2020). M. Spanner demonstrated that ferritin was widely expressed in osteoblastic lineage cells to maintain the intracellular metal balance through the uptake and storage of iron (Spanner et al., 1995). The positive effect of ferritin on osteoporosis is mainly to inhibit iron ions. Iron and iron-induced ROS accumulation was indicated to mediate osteoblast apoptosis and osteoclast differentiation via the NF-κB signaling pathway (Wang et al., 2018; Liu et al., 2021). Increasing the combination of ferritin and iron ions is an effective means to relieve iron damage and ferritin to treat osteoporosis.
Ceruloplasmin (CER), also called copper oxidase, is an important antioxidant in the body. Its antioxidant effect mainly inhibits the production of ROS induced by Fe2 + 73. CER can reduce free radicals produced in xanthine metabolism by inhibiting xanthine oxidase (Krsek-Staples and Webster, 1993). Similar to ferritin, the effect of inducing lipid peroxidation by copper ions has also been weakened when combined with CER (Burkitt, 2001). Current research indicates that CER relieves osteoporosis by inhibiting iron overload (Zarjou et al., 2010). However, the direct effect of CER on osteoporosis is unclear.
Superoxide oxidoreductase (SOD), an endogenous antioxidant metalloenzyme, is the first defense system of antioxidant enzymes that can catalyze the dismutation reaction of superoxide radical anion () to transform into hydrogen peroxide (H2O2) (Lushchak, 2014). A previous study indicated that the total SOD level decreased in menopausal mice, as measured by ELISA (Cao et al., 2020). Hidetoshi Nojiri found that bone mass decreased significantly in SOD-deficient mice (Nojiri et al., 2011). SOD activity is regulated by Smad family transcription factors and is directly involved in the OPG/RANKL/RANK axis to maintain bone homeostasis by increasing osteoblast and inhibiting osteoclast differentiation (Lee et al., 2010; Araújo et al., 2017; Jiang et al., 2020; Qi et al., 2021). Increasing mitochondrial SOD activity prevented osteoblast apoptosis induced by ROS (Yang et al., 2021a). Moreover, SOD has a significant regulatory effect on the differentiation trend of bone marrow mesenchymal stem cells (BM-MSCs). SOD participates in the osteogenesis-adipogenesis balance of BM-MSCs by inhibiting ROS impairment via the p38/MARK pathway (Jurczuk et al., 2004; Wang et al., 2017).
Catalase (CAT) is the marker enzyme of peroxisomes and is widely present in various tissues of the body (Shin et al., 2018). As the second defense system of antioxidant enzymes, the antioxidant mechanism of CAT mainly acts on the dismutation reaction on H2O2 produced in SOD-mediated processes (Ray and Husain, 2002). A large number of studies have reported that H2O2 increased lipid peroxidation following the decrease of CAT in a postmenopausal osteoporosis model (Ozgocmen et al., 2007; Sendur et al., 2009; Zhou et al., 2016). The main effect of CAT is promoting osteogenic differentiation via the Nrf2/HO-1 pathway and preventing mitochondrial apoptosis of osteoblasts as mentioned above (Mada et al., 2017; Sant et al., 2018). In addition, CAT can have a positive effect on bone mass by inhibiting H2O2-induced osteoclastic resorption (Fraser et al., 1996). However, the physiological role of CAT is mainly dependent on the regulation of forkhead box O1 (FOXO1) (Venkatesan et al., 2007). FOXO1 increased the expression of SIRT1 participating in the mitochondrial biosynthesis to maintain the level of CAT (Alcendor et al., 2007). Estrogen deficiency decreases the expression of FOXO1 protein, leading to the inhibition of BM-MSCs into osteoblasts (Liao et al., 2016). Therefore, the FOXO1/CAT pathway has a potential effect on osteoporosis treatment.
Glutathione peroxidase (GSH-Px) is an important peroxidase enzyme characterized by each subunit containing a selenium (Se) atom in the form of selenocysteine (Liu et al., 1999). The antioxidant effect of GSH-Px is determined by the Se cysteine in its active center (Zachara, 1992). The Se of the GSH-Px enzyme system catalyzes GSH to GSSG and reduces toxic peroxides to non-toxic hydroxyl compounds. GSH-Px can intervene in the development of osteoporosis through the abovementioned GSH-dependent pathways and endoplasmic reticulum-mediated osteogenic differentiation of BM-MSCs via the mTOR pathway (Wiswedel et al., 2010; Hu et al., 2021). Additionally, GSH-Px can relieve inflammation-induced osteolytic bone destruction by breaking down LPS (Islam et al., 2007; Li et al., 2020). Upregulating GSH-PX activity can inhibit pro-inflammatory factors associated with osteoclast maturation genes, such as iNOS, IL-1β, and tumor necrosis factor-alpha (TNF-α) (Kruger et al., 2010; He et al., 2020; Li et al., 2020; Umar et al., 2021). GSH-Px may be the key link in the oxidative stress-inflammation reaction in postmenopausal osteoporosis with great potential research value. However, the content of peroxidase in the fracture site was increased to compensate for fracture-induced stress damage when fractures occurred in patients with osteoporosis (Föger-Samwald et al., 2016).
The metabolism of free purine bases after DNA damage aggravates oxidative stress damage. This process continues to convert O2 into , H2O2, and •OH, enhancing oxidative damage. In contrast, a growing number of studies have shown that high uric acid levels can lead to decreased bone density and osteoporosis (Sharaf El Din et al., 2017). The ROS produced by the oxidation of purine inhibits osteoblast differentiation from BM-MSCs and bone mineralization through the ERK and NF-KB pathways (Chang et al., 2009). ROS can stimulate the proliferation and differentiation of osteoclast progenitor cells through the RANKL pathway (Garrett et al., 1990). In addition to direct effects, purine metabolism also regulates bone homeostasis through the indirect activation of inflammatory cytokines (Martinon, 2010). At high uric acid levels, mononuclear cell-derived inflammasomes phagocytose monosodium urate (MSU) and release IL-1, TNF-α, IL-6, and IL-8 (Chhana et al., 2018). They further activate RANK and macrophage colony-stimulating factor (M-CSF), resulting in a large number of osteoclasts (Ritchlin et al., 2003). Therefore, the damaged DNA must be repaired to ensure genomic integrity (Radak and Boldogh, 2010). DNA repair enzymes reduce the production of purine bases to prevent further damage to the skeletal system, as well as the occurrence of osteoporosis (Yao et al., 2020).
Lipid peroxide, a peroxidation product of unsaturated fatty acids with ROS, is the core of lipofuscin (Adibhatla and Hatcher, 2008; Zadlo et al., 2017). The accumulation of lipid peroxide will disrupt the body’s acid balance and vitamin utilization, leading to faster cell division and aging (Zhang et al., 2020b). Previous studies indicated that a large amount of lipid peroxide is deposited in the bone tissue of ovariectomized mice (Al et al., 2018; Abdallah et al., 2020). It triggers oxidative damage and inflammation in the bone microenvironment, destroying bone homeostasis (Wu et al., 2016; Li et al., 2018). Oxidized lipids cannot be repaired and need to be broken down into non-toxic products by specific enzymes. Phospholipase A2 (PLA2) and acyltransferase (AT) are the important metabolic enzymes of lipid peroxide. AT directly participates in lipid mobilization and β-oxidation (Wang et al., 2021). PLA2 catalyzes the hydrolysis of the ester bond formed by the C2 hydroxyl group on the glycerol backbone in the phospholipid (Prunonosa Cervera et al., 2021). Compared with AT, the effect of PLA2 on the skeletal system is not limited to accelerate the metabolism of lipid peroxide. PLA2 can increase the expression of PGE2 to promote osteogenesis through the cyclooxygenase 2 (COX2) pathway (Yoshida et al., 2007; Piazzolla et al., 2015; Li et al., 2021; Figure 3).
At present, osteoporosis affects approximately one-third of postmenopausal women worldwide (Gosset et al., 2021). Nearly 50% of these women will develop osteoporosis-related fractures (Porter and Varacallo, 2021). In the past, scientific researchers and clinicians focused on the skeletal system, especially the inhibition of osteoclasts, to treat postmenopausal osteoporosis (Ukon et al., 2019; Hsiao et al., 2020). Although the deterioration has been improved to a certain extent, the pathogenesis of the disease has not been clarified, and effective control has not been achieved. Oxidative stress damage, as a mediator linking estrogen, aging, and bone, is regarded as a breakthrough in exploring the development of postmenopausal osteoporosis. Overloaded ROS break the balance between osteogenesis and osteoclastogenesis, leading to bone mass loss and bone quality decline (Lee et al., 2021).
The ROS accumulation is due to excessive production and inefficient removal. The DNA damage caused by ROS and the metabolism of DNA purine bases form a closed loop, which continuously increases the production of free radicals (Calkins et al., 2016; Taras-Goslinska et al., 2019). DNA repair enzymes are essential to break this vicious cycle (Figure 4). For the elimination of ROS shown in Figure 5, antioxidant enzymes and GSH/GSSH conversion play an essential role in mitochondria (Zhang et al., 2021). The endogenous hormone melatonin maintains these antioxidant processes by protecting mitochondrial function (Yang et al., 2021b). Other antioxidant substances, such as protein and vitamin antioxidants, also assist the conversion of GSH/GSSG to provide electrons to free radicals to alleviate oxidative damage. The protective effect of the GSH/Nrf2 pathway and SOD-CAT on mitochondrial apoptosis contributes to osteogenesis. The ROS activation of the NF-κB pathway involved in osteoclast maturation is also inhibited by antioxidant systems.
Inflammation is also an important connection between oxidative stress and postmenopausal osteoporosis. Oxidative stress causes biomolecular damage and releases cytokines and chemokines to recruit and activate inflammatory cells, resulting in chronic inflammation in the body (Sindhu et al., 2020). ROS can induce the hyperactivation of NF-κB by modulating the activity of AP-1 (Arcambal et al., 2019). Activated NF-κB also induces the expression of inflammatory factors, such as IL-1β, IL-6, and TNF-α to exacerbate inflammation (Ma et al., 2020). These inflammatory factors also stimulate ROS production to exacerbate oxidative damage (Zhu et al., 2022). A vicious cycle exists between oxidative stress and inflammation. Osteoporosis is also regarded as a chronic inflammatory disease (Montalcini et al., 2013). Estrogen deficiency could induce an inflammatory storm and decrease antioxidant capacity (Mohamad et al., 2020). The secretion of inflammatory factors activates osteoclasts to worsen osteoporosis (Wu et al., 2020; Pan et al., 2021). Therefore, anti-inflammatory drugs have been applied to treat osteoporosis and have the ability to improve bone mass (Tao et al., 2021; Feng et al., 2022).
Due to the unclear pathogenesis of postmenopausal osteoporosis, past studies have obvious limitations. Our review clarified the nature of postmenopausal osteoporosis from the perspective of oxidative stress damage induced by aging and described the potential ability of antioxidants to treat it in detail. Antioxidants not only systematically improve the oxidation state of the body, but also locally regulate the imbalance of the skeletal system. At present, antioxidant substances have been verified to improve bone mass in animal models, such as vitamin C, vitamin E, and GSH (Deng et al., 2014; Lindsey et al., 2019; Han et al., 2020). However, there is no special drug designed based on the antioxidant ability that is being applied for osteoporosis treatment. Three classes of antioxidant systems are very important for the prevention and treatment of postmenopausal osteoporosis. Our review contributes to antioxidant drug designs for postmenopausal osteoporosis.
KY contributed to data curation, formal analysis, data curation, methodology, and writing – original draft. FC contributed to investigation, methodology, software, and writing – original draft. YX contributed to software and validation. LT contributed to conceptualization, software, validation, writing, review, and editing. YZ contributed to funding acquisition, project administration, resources, writing, reviewing, and editing. All authors read and approved the manuscript.
This study was supported by the Shenyang Young and Middle-aged Innovative Talent Project (RC210171).
The authors declare that the research was conducted in the absence of any commercial or financial relationships that could be construed as a potential conflict of interest.
All claims expressed in this article are solely those of the authors and do not necessarily represent those of their affiliated organizations, or those of the publisher, the editors and the reviewers. Any product that may be evaluated in this article, or claim that may be made by its manufacturer, is not guaranteed or endorsed by the publisher.
Abdallah, H. M., Farag, M. A., Algandaby, M. M., Nasrullah, M. Z., Abdel-Naim, A. B., Eid, B. G., et al. (2020). Osteoprotective Activity and Metabolite Fingerprint via UPLC/MS and GC/MS of Lepidium sativum in Ovariectomized Rats. Nutrients 12:2075. doi: 10.3390/nu12072075
Adibhatla, R. M., and Hatcher, J. F. (2008). Phospholipase A(2), reactive oxygen species, and lipid peroxidation in CNS pathologies. BMB Rep. 41, 560–567. doi: 10.5483/bmbrep.2008.41.8.5602/18/2022
Al, E. A. A., Parsian, H., Fathi, M., Faghihzadeh, S., Hosseini, S. R., Nooreddini, H. G., et al. (2018). ALOX12 gene polymorphisms and serum selenium status in elderly osteoporotic patients. Adv. Clin. Exp. Med. 27, 1717–1722. doi: 10.17219/acem/75689
Alcendor, R. R., Gao, S., Zhai, P., Zablocki, D., Holle, E., Yu, X., et al. (2007). Sadoshima, Sirt1 regulates aging and resistance to oxidative stress in the heart. Circ. Res. 100, 1512–1521.
Ameen, O., Yassien, R. I., and Naguib, Y. M. (2020). Activation of FoxO1/SIRT1/RANKL/OPG pathway may underlie the therapeutic effects of resveratrol on aging-dependent male osteoporosis. BMC Musculoskelet. Disord. 21:375. doi: 10.1186/s12891-020-03389-w
Araújo, A. A., Pereira, A., Medeiros, C., Brito, G. A. C., Leitão, R. F. C., Araújo, L. S., et al. (2017). Effects of metformin on inflammation, oxidative stress, and bone loss in a rat model of periodontitis. PLoS One 12:e0183506. doi: 10.1371/journal.pone.0183506
Arcambal, A., Taïlé, J., Rondeau, P., Viranaïcken, W., Meilhac, O., and Gonthier, M. P. (2019). Hyperglycemia modulates redox, inflammatory and vasoactive markers through specific signaling pathways in cerebral endothelial cells: insights on insulin protective action. Free Radical Biol. Med. 130, 59–70. doi: 10.1016/j.freeradbiomed.2018.10.430
Bánhegyi, G., Csala, M., Szarka, A., Varsányi, M., Benedetti, A., and Mandl, J. (2003). Role of ascorbate in oxidative protein folding. Biofactors 17, 37–46. doi: 10.1002/biof.5520170105
Black, D. M., and Rosen, C. J. (2016). Clinical Practice. Postmenopausal Osteoporosis. N. Engl. J. Med. 374, 254–262.
Burkitt, M. J. (2001). A critical overview of the chemistry of copper-dependent low density lipoprotein oxidation: roles of lipid hydroperoxides, alpha-tocopherol, thiols, and ceruloplasmin. Arch. Biochem. Biophys. 394, 117–135. doi: 10.1006/abbi.2001.2509
Calkins, M. J., Vartanian, V., Owen, N., Kirkali, G., Jaruga, P., Dizdaroglu, M., et al. (2016). Enhanced sensitivity of Neil1(-/-) mice to chronic UVB exposure. DNA Repair 48, 43–50. doi: 10.1016/j.dnarep.2016.10.010
Cao, X., Luo, D., Li, T., Huang, Z., Zou, W., Wang, L., et al. (2020). MnTBAP inhibits bone loss in ovariectomized rats by reducing mitochondrial oxidative stress in osteoblasts. J. Bone Mineral Metabol. 38, 27–37. doi: 10.1007/s00774-019-01038-4
Casati, L., Pagani, F., Limonta, P., Vanetti, C., Stancari, G., and Sibilia, V. (2020). Beneficial effects of δ-tocotrienol against oxidative stress in osteoblastic cells: studies on the mechanisms of action. Eur. J. Nutr. 59, 1975–1987. doi: 10.1007/s00394-019-02047-9
Chang, J., Wang, Z., Tang, E., Fan, Z., McCauley, L., Franceschi, R., et al. (2009). Inhibition of osteoblastic bone formation by nuclear factor-kappaB. Nat. Med. 15, 682–689. doi: 10.1038/nm.1954
Chavan, S. N., More, U., Mulgund, S., Saxena, V., and Sontakke, A. N. (2007). Effect of supplementation of vitamin C and E on oxidative stress in osteoporosis. Indian J. Clin. Biochem. 22, 101–105. doi: 10.1007/BF02913324
Chen, L., Hu, S. L., Xie, J., Yan, D. Y., Weng, S. J., Tang, J. H., et al. (2020a). Proanthocyanidins-Mediated Nrf2 Activation Ameliorates Glucocorticoid-Induced Oxidative Stress and Mitochondrial Dysfunction in Osteoblasts. Oxid. Med. Cell Longev. 2020:9102012. doi: 10.1155/2020/9102012
Chen, L., Jin, X. H., Luo, J., Duan, J. L., Cai, M. Y., Chen, J. W., et al. (2021a). ITLN1 inhibits tumor neovascularization and myeloid derived suppressor cells accumulation in colorectal carcinoma. Oncogene 40, 5925–5937. doi: 10.1038/s41388-021-01965-5
Chen, W., Chen, X., Chen, A. C., Shi, Q., Pan, G., Pei, M., et al. (2020b). Melatonin restores the osteoporosis-impaired osteogenic potential of bone marrow mesenchymal stem cells by preserving SIRT1-mediated intracellular antioxidant properties. Free Radic. Biol. Med. 146, 92–106. doi: 10.1016/j.freeradbiomed.2019.10.412
Chen, X., Ma, F., Zhai, N., Gao, F., and Cao, G. (2021b). Long non-coding RNA XIST inhibits osteoblast differentiation and promotes osteoporosis via Nrf2 hyperactivation by targeting CUL3. Int. J. Mol. Med. 48:137. doi: 10.3892/ijmm.2021.4970
Chhana, A., Pool, B., Callon, K. E., Tay, M. L., Musson, D., Naot, D., et al. (2018). Monosodium urate crystals reduce osteocyte viability and indirectly promote a shift in osteocyte function towards a proinflammatory and proresorptive state. Arthritis Res. Ther. 20, 208. doi: 10.1186/s13075-018-1704-y
Choi, H. K., Kim, G. J., Yoo, H. S., Song, D. H., Chung, K. H., Lee, K. J., et al. (2019). Vitamin C Activates Osteoblastogenesis and Inhibits Osteoclastogenesis via Wnt/β-Catenin/ATF4 Signaling Pathways. Nutrients 11:506. doi: 10.3390/nu11030506
Chojkier, M., Spanheimer, R., and Peterkofsky, B. (1983). Specifically decreased collagen biosynthesis in scurvy dissociated from an effect on proline hydroxylation and correlated with body weight loss. In vitro studies in guinea pig calvarial bones. J. Clin. Invest. 72, 826–835. doi: 10.1172/JCI111053
Da, W., Tao, L., Wen, K., Tao, Z., Wang, S., and Zhu, Y. (2020). Protective Role of Melatonin Against Postmenopausal Bone Loss via Enhancement of Citrate Secretion From Osteoblasts. Front. Pharmacol. 11:667. doi: 10.3389/fphar.2020.00667
Davalli, P., Mitic, T., Caporali, A., Lauriola, A., and D’Arca, D. (2016). ROS, Cell Senescence, and Novel Molecular Mechanisms in Aging and Age-Related Diseases. Oxid. Med. Cell Longev. 2016:3565127. doi: 10.1155/2016/3565127
Deng, L., Ding, Y., Peng, Y., Wu, Y., Fan, J., Li, W., et al. (2014). γ-Tocotrienol protects against ovariectomy-induced bone loss via mevalonate pathway as HMG-CoA reductase inhibitor. Bone 67, 200–207. doi: 10.1016/j.bone.2014.07.006
Dey, D., Jingar, P., Agrawal, S., Shrivastava, V., Bhattacharya, A., Manhas, J., et al. (2020). Symphytum officinale augments osteogenesis in human bone marrow-derived mesenchymal stem cells in vitro as they differentiate into osteoblasts. J. Ethnopharmacol. 248:112329. doi: 10.1016/j.jep.2019.112329
Fan, J., Ye, J., Kamphorst, J. J., Shlomi, T., Thompson, C. B., and Rabinowitz, J. D. (2014). Quantitative flux analysis reveals folate-dependent NADPH production. Nature 510, 298–302. doi: 10.1038/nature13236
Feng, P., Shu, S., and Zhao, F. (2022). Anti-osteoporosis Effect of Fisetin against Ovariectomy Induced Osteoporosis in Rats: in silico, in vitro and in vivo Activity. J. Oleo. Sci. 71, 105–118. doi: 10.5650/jos.ess21252
Föger-Samwald, U., Vekszler, G., Hörz-Schuch, E., Salem, S., Wipperich, M., Ritschl, P., et al. (2016). Molecular mechanisms of osteoporotic hip fractures in elderly women. Exp. Gerontol. 73, 49–58. doi: 10.1016/j.exger.2015.11.012
Forrester, S. J., Kikuchi, D. S., Hernandes, M. S., Xu, Q., and Griendling, K. K. (2018). Reactive Oxygen Species in Metabolic and Inflammatory Signaling. Circ. Res. 122, 877–902. doi: 10.1161/CIRCRESAHA.117.311401
Fraser, J. H., Helfrich, M. H., Wallace, H. M., and Ralston, S. H. (1996). Hydrogen peroxide, but not superoxide, stimulates bone resorption in mouse calvariae. Bone 19, 223–226. doi: 10.1016/8756-3282(96)00177-9
Garrett, I. R., Boyce, B. F., Oreffo, R. O., Bonewald, L., Poser, J., and Mundy, G. R. (1990). Oxygen-derived free radicals stimulate osteoclastic bone resorption in rodent bone in vitro and in vivo. J. Clin. Invest. 85, 632–639. doi: 10.1172/JCI114485
Gosset, A., Pouillès, J. M., and Trémollieres, F. (2021). Menopausal hormone therapy for the management of osteoporosis. Best Pract. Res. Clin. Endocrinol. Metab. 35:101551.
Han, B., Geng, H., Liu, L., Wu, Z., and Wang, Y. (2020). GSH attenuates RANKL-induced osteoclast formation in vitro and LPS-induced bone loss in vivo. Biomed. Pharmacother. 128, 110305. doi: 10.1016/j.biopha.2020.110305
He, Z., Sun, Y., Wu, J., Xiong, Z., Zhang, S., Liu, J., et al. (2020). Evaluation of genetic variants in IL-1B and its interaction with the predisposition of osteoporosis in the northwestern Chinese Han population. J. Gene Med. 22:e3214. doi: 10.1002/jgm.3214
Hong, J., Shi, Z., Li, C., Ji, X., Li, S., Chen, Y., et al. (2021). Virtual screening identified natural Keap1-Nrf2 PPI inhibitor alleviates inflammatory osteoporosis through Nrf2-mir214-Traf3 axis. Free Radical Biol. Med. 171, 365–378. doi: 10.1016/j.freeradbiomed.2021.05.020
Hossain, M. S., Li, J., Sikdar, A., Hasanuzzaman, M., Uzizerimana, F., Muhammad, I., et al. (2020). Exogenous Melatonin Modulates the Physiological and Biochemical Mechanisms of Drought Tolerance in Tartary Buckwheat (Fagopyrum tataricum (L.) Gaertn). Molecules 25, 2828. doi: 10.3390/molecules25122828
Hsiao, C. Y., Chen, T. H., Chu, T. H., Ting, Y. N., Tsai, P. J., and Shyu, J. F. (2020). Calcitonin Induces Bone Formation by Increasing Expression of Wnt10b in Osteoclasts in Ovariectomy-Induced Osteoporotic Rats. Front. Endocrinol. 11:613. doi: 10.3389/fendo.2020.00613
Hu, X., Li, B., Wu, F., Liu, X., Liu, M., Wang, C., et al. (2021). GPX7 Facilitates BMSCs Osteoblastogenesis via ER Stress and mTOR Pathway. J. Cell. Mol. Med. 25, 10454–10465. doi: 10.1111/jcmm.16974
Islam, S., Hassan, F., Tumurkhuu, G., Dagvadorj, J., Koide, N., Naiki, Y., et al. (2007). Bacterial lipopolysaccharide induces osteoclast formation in RAW 264.7 macrophage cells. Biochem. Biophys. Res. Commun. 360, 346–351. doi: 10.1016/j.bbrc.2007.06.023
Jiang, Y., Luo, W., Wang, B., Wang, X., Gong, P., and Xiong, Y. (2020). Resveratrol promotes osteogenesis via activating SIRT1/FoxO1 pathway in osteoporosis mice. Life Sci. 246:117422. doi: 10.1016/j.lfs.2020.117422
Jurczuk, M., Brzóska, M. M., Moniuszko-Jakoniuk, J., Gałazyn-Sidorczuk, M., and Kulikowska-Karpińska, E. (2004). Antioxidant enzymes activity and lipid peroxidation in liver and kidney of rats exposed to cadmium and ethanol. Food Chem. Toxicol. 42, 429–438. doi: 10.1016/j.fct.2003.10.005
Kabasawa, Y., Asahina, I., Gunji, A., and Omura, K. (2003). Administration of parathyroid hormone, prostaglandin E2, or 1-alpha,25-dihydroxyvitamin D3 restores the bone inductive activity of rhBMP-2 in aged rats. DNA Cell Biol. 22, 541–546. doi: 10.1089/104454903322405428
Krsek-Staples, J. A., and Webster, R. O. (1993). Ceruloplasmin inhibits carbonyl formation in endogenous cell proteins. Free Radic. Biol. Med. 14, 115–125. doi: 10.1016/0891-5849(93)90002-c
Kruger, M. C., Coetzee, M., Haag, M., and Weiler, H. (2010). Long-chain polyunsaturated fatty acids: selected mechanisms of action on bone. Prog. Lipid Res. 49, 438–449. doi: 10.1016/j.plipres.2010.06.002
Lee, C. W., Lin, H. C., Wang, B. Y., Wang, A. Y., Shin, R. L., Cheung, S. Y. L., et al. (2021). Ginkgolide B monotherapy reverses osteoporosis by regulating oxidative stress-mediated bone homeostasis. Free Radic. Biol. Med. 168, 234–246. doi: 10.1016/j.freeradbiomed.2021.03.008
Lee, Y. H., Lee, N. H., Bhattarai, G., Oh, Y. T., Yu, M. K. I, Yoo, D., et al. (2010). Enhancement of osteoblast biocompatibility on titanium surface with Terrein treatment. Cell Biochem. Funct. 28, 678–685. doi: 10.1002/cbf.1708
Li, H., Huang, C., Zhu, J., Gao, K., Fang, J., and Li, H. (2018). Lutein Suppresses Oxidative Stress and Inflammation by Nrf2 Activation in an Osteoporosis Rat Model. Med. Sci. Monit. 24, 5071–5075. doi: 10.12659/MSM.908699
Li, L., Park, Y. R., Shrestha, S. K., Cho, H. K., and Soh, Y. (2020). Suppression of Inflammation, Osteoclastogenesis and Bone Loss by PZRAS Extract. J. Microbiol. Biotechnol. 30, 1543–1551. doi: 10.4014/jmb.2004.04016
Li, Y. H., Zhu, D., Yang, T., Cheng, L., Sun, J., and Tan, L. (2021). Crosstalk between the COX2-PGE2-EP4 signaling pathway and primary cilia in osteoblasts after mechanical stimulation. J. Cell Physiol. 236, 4764–4777. doi: 10.1002/jcp.30198
Liao, L., Su, X., Yang, X., Hu, C., Li, B., Lv, Y., et al. (2016). TNF-alpha Inhibits FoxO1 by Upregulating miR-705 to Aggravate Oxidative Damage in Bone Marrow-Derived Mesenchymal Stem Cells during Osteoporosis. Stem Cells 34, 1054–1067. doi: 10.1002/stem.2274
Lindsey, R. C., Cheng, S., and Mohan, S. (2019). Vitamin C effects on 5-hydroxymethylcytosine and gene expression in osteoblasts and chondrocytes: potential involvement of PHD2. PLoS One 14:e0220653. doi: 10.1371/journal.pone.0220653
Liu, H., Wang, Y. W., Chen, W. D., Dong, H. H., and Xu, Y. J. (2021). Iron accumulation regulates osteoblast apoptosis through lncRNA XIST/miR-758-3p/caspase 3 axis leading to osteoporosis. IUBMB Life 73, 432–443. doi: 10.1002/iub.2440
Liu, Q., Lauridsen, E., and Clausen, J. (1999). The major selenium-containing protein in human peripheral granulocytes. Biol. Trace Elem. Res. 68, 193–207. doi: 10.1007/BF02783903
Liu, S., Fang, T., Yang, L., Chen, Z., Mu, S., and Fu, Q. (2018). Gastrodin protects MC3T3-E1 osteoblasts from dexamethasone-induced cellular dysfunction and promotes bone formation via induction of the NRF2 signaling pathway. Int. J. Mol. Med. 41, 2059–2069. doi: 10.3892/ijmm.2018.3414
Lu, M., Liu, Y., Shao, M., Tesfaye, G. C., and Yang, S. (2020). Associations of Iron Intake, Serum Iron and Serum Ferritin with Bone Mineral Density in Women: the National Health and Nutrition Examination Survey, 2005-2010. Calcif. Tissue Int. 106, 232–238. doi: 10.1007/s00223-019-00627-9
Luo, J., Bai, L., Tao, J., Wen, Y., Li, M., Zhu, Y., et al. (2021). Autophagy induced by H. pylori VacA regulated the survival mechanism of the SGC7901 human gastric cancer cell line. Genes Genom. 43, 1223–1230. doi: 10.1007/s13258-021-01151-7
Lushchak, V. I. (2014). Free radicals, reactive oxygen species, oxidative stress and its classification. Chem. Biol. Interact. 224, 164–175. doi: 10.1016/j.cbi.2014.10.016
Ma, L., Zhang, Q., Hao, J., Wang, J., and Wang, C. (2020). LncRNA PVT1 exacerbates the inflammation and cell-barrier injury during asthma by regulating miR-149. J. Biochem. Mol. Toxicol. 34:e22563. doi: 10.1002/jbt.22563
Mada, S. B., Reddi, S., Kumar, N., Kapila, S., and Kapila, R. (2017). Protective effects of casein-derived peptide VLPVPQK against hydrogen peroxide-induced dysfunction and cellular oxidative damage in rat osteoblastic cells. Hum. Exp. Toxicol. 36, 967–980. doi: 10.1177/0960327116678293
Mandelli, A., Tacconi, E., Levinger, I., Duque, G., and Hayes, A. (2021). The role of estrogens in osteosarcopenia: from biology to potential dual therapeutic effects. Climacteric 25, 81–87. doi: 10.1080/13697137.2021.1965118
Martinon, F. (2010). Update on biology: uric acid and the activation of immune and inflammatory cells. Curr. Rheumatol. Rep. 12, 135–141. doi: 10.1007/s11926-010-0092-3
Miyazaki-Akita, A., Hayashi, T., Ding, Q. F., Shiraishi, H., Nomura, T., Hattori, Y., et al. (2007). 17beta-estradiol antagonizes the down-regulation of endothelial nitric-oxide synthase and GTP cyclohydrolase I by high glucose: relevance to postmenopausal diabetic cardiovascular disease. J. Pharmacol. Exp. Ther. 320, 591–598. doi: 10.1124/jpet.106.111641
Mizerska-Kowalska, M., Sławińska-Brych, A., Kaławaj, K., Żurek, A., Pawińska, B., Rzeski, W., et al. (2019). Betulin Promotes Differentiation of Human Osteoblasts In Vitro and Exerts an Osteoinductive Effect on the hFOB 1.19 Cell Line Through Activation of JNK, ERK1/2, and mTOR Kinases. Molecules 24:2637. doi: 10.3390/molecules24142637
Mohamad, N. V., Ima-Nirwana, S., and Chin, K. Y. (2020). Are Oxidative Stress and Inflammation Mediators of Bone Loss Due to Estrogen Deficiency? A Review of Current Evidence. Endocr. Metab. Immune. Disord. Drug Targets 20, 1478–1487. doi: 10.2174/1871530320666200604160614
Montalcini, T., Romeo, S., Ferro, Y., Migliaccio, V., Gazzaruso, C., and Pujia, A. (2013). Osteoporosis in chronic inflammatory disease: the role of malnutrition. Endocrine 43, 59–64. doi: 10.1007/s12020-012-9813-x
Munmun, F., and Witt-Enderby, P. A. (2021). Melatonin effects on bone: implications for use as a therapy for managing bone loss. Journal of pineal research 71, e12749. doi: 10.1111/jpi.12749
Nazrun, A. S., Norazlina, M., Norliza, M., and Nirwana, S. I. (2012). The anti-inflammatory role of vitamin e in prevention of osteoporosis. Adv. Pharmacol. Sci. 2012:142702. doi: 10.1155/2012/142702
Nazrun Shuid, A., Das, S., and Mohamed, I. N. (2019). Therapeutic effect of Vitamin E in preventing bone loss: an evidence-based review. Int. J. Vitam. Nutr. Res. 89, 357–370. doi: 10.1024/0300-9831/a000566
Nielsen-Marsh, C. M., Richards, M. P., Hauschka, P. V., Thomas-Oates, J. E., Trinkaus, E., Pettitt, P. B., et al. (2005). Osteocalcin protein sequences of Neanderthals and modern primates. Proc. Natl. Acad. Sci. U S A 102, 4409–4413. doi: 10.1073/pnas.0500450102
Nojiri, H., Saita, Y., Morikawa, D., Kobayashi, K., Tsuda, C., Miyazaki, T., et al. (2011). Cytoplasmic superoxide causes bone fragility owing to low-turnover osteoporosis and impaired collagen cross-linking. J. Bone Mineral Res. Am. Soc. Bone Mineral Res. 26, 2682–2694. doi: 10.1002/jbmr.489
Obraztsov, V. V., Glushchenko, N. N., Orlov, S. N., and Danilov, V. S. (1975). [Free radical oxidation of biological membrane lipids. IV. iron salts in peroxidation catalysis of linoleic acid]. Biofizika 20, 93–97.
Oh, J. Y., Choi, G. E., Lee, H. J., Jung, Y. H., Chae, C. W., Kim, J. S., et al. (2019). 17β-Estradiol protects mesenchymal stem cells against high glucose-induced mitochondrial oxidants production via Nrf2/Sirt3/MnSOD signaling. Free Radl Biol. Med. 130, 328–342. doi: 10.1016/j.freeradbiomed.2018.11.003
Oh, Y., Ahn, C. B., Marasinghe, M., and Je, J. Y. (2021). Insertion of gallic acid onto chitosan promotes the differentiation of osteoblasts from murine bone marrow-derived mesenchymal stem cells. Int. J. Biol. Macromol. 183, 1410–1418. doi: 10.1016/j.ijbiomac.2021.05.122
Ozgocmen, S., Kaya, H., Fadillioglu, E., and Yilmaz, Z. (2007). Effects of calcitonin, risedronate, and raloxifene on erythrocyte antioxidant enzyme activity, lipid peroxidation, and nitric oxide in postmenopausal osteoporosis. Arch. Med. Res. 38, 196–205. doi: 10.1016/j.arcmed.2006.09.010
Pan, B., Zheng, L., Fang, J., Lin, Y., Lai, H., Gao, J., et al. (2021). Azilsartan Suppresses Osteoclastogenesis and Ameliorates Ovariectomy-Induced Osteoporosis by Inhibiting Reactive Oxygen Species Production and Activating Nrf2 Signaling. Front. Pharmacol. 12:774709. doi: 10.3389/fphar.2021.774709
Park, K. H., Kang, J. W., Lee, E. M., Kim, J. S., Rhee, Y. H., Kim, M., et al. (2011). Melatonin promotes osteoblastic differentiation through the BMP/ERK/Wnt signaling pathways. J. Pineal Res. 51, 187–194. doi: 10.1111/j.1600-079X.2011.00875.x
Piazzolla, A., Solarino, G., Bizzoca, D., Garofalo, N., Dicuonzo, F., Setti, S., et al. (2015). CAPACITIVE COUPLING ELECTRIC FIELDS IN THE TREATMENT OF VERTEBRAL COMPRESSION FRACTURES. J. Biol. Regul. Homeost. Agents 29, 637–646.
Pignolo, R. J., Law, S. F., and Chandra, A. (2021). Bone Aging. Cell. Senescen. Osteo.. JBMR Plus 5:e10488.
Prunonosa Cervera, I., Gabriel, B. M., Aldiss, P., and Morton, N. M. (2021). The phospholipase A2 family’s role in metabolic diseases: focus on skeletal muscle. Physiol. Rep. 9:e14662. doi: 10.14814/phy2.14662
Qaseem, A., Forciea, M. A., McLean, R. M., Denberg, T. D., Barry, M. J., Cooke, M., et al. (2017). Treatment of Low Bone Density or Osteoporosis to Prevent Fractures in Men and Women: a Clinical Practice Guideline Update From the American College of Physicians. Ann. Intern. Med. 166, 818–839. doi: 10.7326/M15-1361
Qi, Y., Wang, H., Chen, X., and Zhu, Y. (2021). The role of TGF-β1/Smad3 signaling pathway and oxidative stress in the inhibition of osteoblast mineralization by copper chloride. Environ. Toxicol. Pharmacol. 84, 103613. doi: 10.1016/j.etap.2021.103613
Qiu, X., Wang, X., Qiu, J., Zhu, Y., Liang, T., Gao, B., et al. (2019). Melatonin Rescued Reactive Oxygen Species-Impaired Osteogenesis of Human Bone Marrow Mesenchymal Stem Cells in the Presence of Tumor Necrosis Factor-Alpha. Stem Cells Int 2019:6403967. doi: 10.1155/2019/6403967
Radak, Z., and Boldogh, I. (2010). 8-Oxo-7,8-dihydroguanine: links to gene expression, aging, and defense against oxidative stress. Free Radical Biol. Med. 49, 587–596. doi: 10.1016/j.freeradbiomed.2010.05.008
Ray, G., and Husain, S. A. (2002). Oxidants, antioxidants and carcinogenesis. Ind. J. Exp. Biol. 40, 1213–1232.
Ritchlin, C. T., Haas-Smith, S. A., Li, P., Hicks, D. G., and Schwarz, E. M. (2003). Mechanisms of TNF-alpha- and RANKL-mediated osteoclastogenesis and bone resorption in psoriatic arthritis. J. Clin. Invest. 111, 821–831. doi: 10.1172/JCI16069
Sant, K. E., Sinno, P. P., Jacobs, H. M., and Timme-Laragy, A. R. (2018). Nrf2a modulates the embryonic antioxidant response to perfluorooctanesulfonic acid (PFOS) in the zebrafish. Danio rerio. Aquat. Toxicol. 198, 92–102. doi: 10.1016/j.aquatox.2018.02.010
Seminotti, B., Roginski, A. C., Zanatta, Â, Amaral, A. U., Fernandes, T., and Spannenberger, K. P. (2021). S-adenosylmethionine induces mitochondrial dysfunction, permeability transition pore opening and redox imbalance in subcellular preparations of rat liver. J. Bioenerg. Biomembr. 53, 525–539. doi: 10.1007/s10863-021-09914-3
Sendur, O. F., Turan, Y., Tastaban, E., and Serter, M. (2009). Antioxidant status in patients with osteoporosis: a controlled study. Joint Bone Spine 76, 514–518. doi: 10.1016/j.jbspin.2009.02.005
Shahriarpour, Z., Nasrabadi, B., Hejri-Zarifi, S., Shariati-Bafghi, S. E., Yousefian-Sanny, M., Karamati, M., et al. (2021). Oxidative balance score and risk of osteoporosis among postmenopausal Iranian women. Arch. Osteoporos 16:43. doi: 10.1007/s11657-021-00886-w
Shan, H. J., Zhu, L. Q., Yao, C., Zhang, Z. Q., Liu, Y. Y., Jiang, Q., et al. (2021). MAFG-driven osteosarcoma cell progression is inhibited by a novel miRNA miR-4660. Mol. Ther. Nucleic Acids 24, 385–402. doi: 10.1016/j.omtn.2021.03.006
Sharaf El Din, U. A. A., Salem, M. M., and Abdulazim, D. O. (2017). Uric acid in the pathogenesis of metabolic, renal, and cardiovascular diseases: a review. J. Adv. Res. 8, 537–548. doi: 10.1016/j.jare.2016.11.004
Shin, S. K., Cho, H. W., Song, S. E., and Song, D. K. (2018). Catalase and nonalcoholic fatty liver disease. Pflugers Arch. 470, 1721–1737.
Silva, H. (2021). The Vascular Effects of Isolated Isoflavones-A Focus on the Determinants of Blood Pressure Regulation. Biology 10:49. doi: 10.3390/biology10010049
Simpkins, J. W., Yang, S. H., Sarkar, S. N., and Pearce, V. (2008). Estrogen actions on mitochondria–physiological and pathological implications. Mol. Cell Endocrinol. 290, 51–59. doi: 10.1016/j.mce.2008.04.013
Sindhu, S., Akhter, N., Wilson, A., Thomas, R., Arefanian, H., Al Madhoun, A., et al. (2020). 1α Expression Induced by Co-Stimulation of Human Monocytic Cells with Palmitate and TNF-α Involves the TLR4-IRF3 Pathway and Is Amplified by Oxidative Stress. Cells 9, 1799. doi: 10.3390/cells9081799
Song, C., Wang, J., Kim, B., Lu, C., Zhang, Z., Liu, H., et al. (2018). Insights into the Role of Circadian Rhythms in Bone Metabolism: a Promising Intervention Target? BioMed Res. Int. 2018:9156478. doi: 10.1155/2018/9156478
Spanner, M., Weber, K., Lanske, B., Ihbe, A., Siggelkow, H., Schütze, H., et al. (1995). The iron-binding protein ferritin is expressed in cells of the osteoblastic lineage in vitro and in vivo. Bone 17, 161–165. doi: 10.1016/s8756-3282(95)00176-x
Srivastava, M., and Deal, C. (2002). Osteoporosis in elderly: prevention and treatment. Clin. Geriatr. Med. 18, 529–555.
Sun, Y. X., Xu, A. H., Yang, Y., and Li, J. (2015). Role of Nrf2 in bone metabolism. J. Biomed. Sci. 22:101. doi: 10.1186/s12929-015-0212-5
Süntar, I., Çetinkaya, S., Panieri, E., Saha, S., Buttari, B., Profumo, E., et al. (2021). Regulatory Role of Nrf2 Signaling Pathway in Wound Healing Process. Molecules 26:2424. doi: 10.3390/molecules26092424
Tao, H., Ge, G., Liang, X., Zhang, W., Sun, H., Li, M., et al. (2020). ROS signaling cascades: dual regulations for osteoclast and osteoblast. Acta Biochim. Biophys. Sin. 52, 1055–1062. doi: 10.1093/abbs/gmaa098
Tao, H., Li, W., Zhang, W., Yang, C., Zhang, C., Liang, X., et al. (2021). A suppresses RANKL-induced osteoclastogenesis and postmenopausal osteoporosis by, suppresses inflammation and downstream NF-κB activated pyroptosis pathways. Pharmacol. Res. 174:105967. doi: 10.1016/j.phrs.2021.105967
Taras-Goslinska, K., Vetica, F., Barata-Vallejo, S., Triantakostanti, V., Marciniak, B., and Chatgilialoglu, C. (2019). Converging Fate of the Oxidation and Reduction of 8-Thioguanosine. Molecules 24:3143. doi: 10.3390/molecules24173143
Thummuri, D., Naidu, V. G. M., and Chaudhari, P. (2017). Carnosic acid attenuates RANKL-induced oxidative stress and osteoclastogenesis via induction of Nrf2 and suppression of NF-κB and MAPK signalling. J. Mol. Med. 95, 1065–1076. doi: 10.1007/s00109-017-1553-1
Tian, S., Jones, S. M., and Solomon, E. I. (2020). Role of a Tyrosine Radical in Human Ceruloplasmin Catalysis. ACS Cent. Sci. 6, 1835–1843. doi: 10.1021/acscentsci.0c00953
Tu, C., Wu, D. Z., Huang, Y. S., Zhuang, J. S., Zeng, J. H., Xu, P., et al. (2020). Oxidative Stress Contributes to Hyperalgesia in Osteoporotic Mice. J. Pain Res. 13, 131–142. doi: 10.2147/JPR.S234334
Ukon, Y., Makino, T., Kodama, J., Tsukazaki, H., Tateiwa, D., Yoshikawa, H., et al. (2019). Molecular-Based Treatment Strategies for Osteoporosis: a Literature Review. Int. J. Mol. Sci. 20, 2557. doi: 10.3390/ijms20102557
Umar, S., Palasiewicz, K., Van Raemdonck, K., Volin, M. V., Romay, B., Ahmad, I., et al. (2021). CCL25 and CCR9 is a unique pathway that potentiates pannus formation by remodeling RA macrophages into mature osteoclasts. Eur. J. Immunol. 51, 903–914. doi: 10.1002/eji.202048681
Vakili, S., Zal, F., Mostafavi-Pour, Z., Savardashtaki, A., and Koohpeyma, F. (2021). Quercetin and vitamin E alleviate ovariectomy-induced osteoporosis by modulating autophagy and apoptosis in rat bone cells. J. Cell. Physiol. 236, 3495–3509. doi: 10.1002/jcp.30087
Venkatesan, B., Mahimainathan, L., Das, F., Ghosh-Choudhury, N., and Ghosh Choudhury, G. (2007). Downregulation of catalase by reactive oxygen species via PI 3 kinase/Akt signaling in mesangial cells. J. Cell Physiol. 211, 457–467. doi: 10.1002/jcp.20953
Wang, D., Sun, X., Maziade, M., Mao, W., Zhang, C., Wang, J., et al. (2021). Characterising phospholipids and free fatty acids in patients with schizophrenia: a case-control study. World J. Biol. Psychiatr. 22, 161–174. doi: 10.1080/15622975.2020.1769188
Wang, J., Wu, D., Zhang, J., Liu, H., Wu, J., and Dong, W. (2019). α-Hederin Induces Apoptosis of Esophageal Squamous Cell Carcinoma via an Oxidative and Mitochondrial-Dependent Pathway. Dig. Dis. Sci. 64, 3528–3538. doi: 10.1007/s10620-019-05689-1
Wang, X., Chen, B., Sun, J., Jiang, Y., Zhang, H., Zhang, P., et al. (2018). Iron-induced oxidative stress stimulates osteoclast differentiation via NF-κB signaling pathway in mouse model. Metabolism 83, 167–176. doi: 10.1016/j.metabol.2018.01.005
Wang, Y., Wu, H., Shen, M., Ding, S., Miao, J., and Chen, N. (2017). Role of human amnion-derived mesenchymal stem cells in promoting osteogenic differentiation by influencing p38 MAPK signaling in lipopolysaccharide -induced human bone marrow mesenchymal stem cells. Exp. Cell Res. 350, 41–49. doi: 10.1016/j.yexcr.2016.11.003
Wang, Y. Q., Wang, N. X., Luo, Y., Yu, C. Y., and Xiao, J. H. (2020). Ganoderal A effectively induces osteogenic differentiation of human amniotic mesenchymal stem cells via cross-talk between Wnt/β-catenin and BMP/SMAD signaling pathways. Biomed. Pharmacother. 123:109807. doi: 10.1016/j.biopha.2019.109807
Wiswedel, I., Gardemann, A., Storch, A., Peter, D., and Schild, L. (2010). Degradation of phospholipids by oxidative stress–exceptional significance of cardiolipin. Free Radic. Res. 44, 135–145. doi: 10.3109/10715760903352841
Wong, S. K., Mohamad, N. V., Ibrahim, N., Chin, K. Y., Shuid, A. N., and Ima-Nirwana, S. (2019). The Molecular Mechanism of Vitamin E as a Bone-Protecting Agent: a Review on Current Evidence. Int. J. Mol. Sci. 20:1453. doi: 10.3390/ijms20061453
Wu, L. F., Wang, W. Y., Zhu, D. C., He, P., Zhu, K., Gui, G. P., et al. (2020). Protein array test detected three osteoporosis related plasma inflammatory cytokines in Chinese postmenopausal women. Cytokine 133:155166. doi: 10.1016/j.cyto.2020.155166
Wu, Y. X., Wu, T. Y., Xu, B. B., Xu, X. Y., Chen, H. G., Li, X. Y., et al. (2016). Protocatechuic acid inhibits osteoclast differentiation and stimulates apoptosis in mature osteoclasts. Biomed. Pharmacother. 82, 399–405. doi: 10.1016/j.biopha.2016.05.008
Xiao, Y., Xian, Y., Hu, X., and Qi, Z. (2021). D(-)-salicin inhibits RANKL-induced osteoclast differentiation and function in vitro. Fitoterapia 157:104981. doi: 10.1016/j.fitote.2021.104981
Xu, L. L., Zhao, B., Sun, S. L., Yu, S. F., Wang, Y. M., Ji, R., et al. (2020). High-dose vitamin C alleviates pancreatic injury via the NRF2/NQO1/HO-1 pathway in a rat model of severe acute pancreatitis. Ann. Transl. Med 8:852. doi: 10.21037/atm-19-4552
Yalin, S., Sagír, O., Comelekoglu, U., Berköz, M., and Eroglu, P. (2012). Strontium ranelate treatment improves oxidative damage in osteoporotic rat model. Pharmacol. Rep. 64, 396–402. doi: 10.1016/s1734-1140(12)70780-6
Yang, K., Pei, L., Zhou, S., Tao, L., and Zhu, Y. (2021a). Metformin attenuates H(2)O(2)-induced osteoblast apoptosis by regulating SIRT3 via the PI3K/AKT pathway. Exp. Ther. Med. 22:1316. doi: 10.3892/etm.2021.10751
Yang, S. G., Joe, S. Y., Bae, J. W., Heo, G. D., Park, H. J., and Koo, D. B. (2021b). Melatonin Protects Against Mdivi-1-Induced Abnormal Spindle Assembly and Mitochondrial Superoxide Production During Porcine Oocyte Maturation. Front. Cell Dev. Biol. 9:693969. doi: 10.3389/fcell.2021.693969
Yao, X., Chen, L., Xu, H., and Zhu, Z. (2020). The Association between Serum Uric Acid and Bone Mineral Density in Older Adults. Int. J. Endocrinol. 2020:3082318. doi: 10.1155/2020/3082318
Yoshida, K., Shinohara, H., Haneji, T., and Nagata, T. (2007). Arachidonic acid inhibits osteoblast differentiation through cytosolic phospholipase A2-dependent pathway. Oral Dis. 13, 32–39. doi: 10.1111/j.1601-0825.2006.01239.x
Youn, J. Y., Zhang, Y., Wu, Y., Cannesson, M., and Cai, H. (2021). Therapeutic application of estrogen for COVID-19: attenuation of SARS-CoV-2 spike protein and IL-6 stimulated, ACE2-dependent NOX2 activation, ROS production and MCP-1 upregulation in endothelial cells. Redox Biol. 46:102099. doi: 10.1016/j.redox.2021.102099
Yu, G. M., and Tan, W. (2019). Melatonin Inhibits Lipopolysaccharide-Induced Inflammation and Oxidative Stress in Cultured Mouse Mammary Tissue. Media. Inflamm. 2019:8597159. doi: 10.1155/2019/8597159
Yung, L. M., Wong, W. T., Tian, X. Y., Leung, F. P., Yung, L. H., Chen, Z. Y., et al. (2011). Inhibition of renin-angiotensin system reverses endothelial dysfunction and oxidative stress in estrogen deficient rats. PLoS One 6:e17437. doi: 10.1371/journal.pone.0017437
Zadlo, A., Pilat, A., Sarna, M., Pawlak, A., and Sarna, T. (2017). Redox Active Transition Metal ions Make Melanin Susceptible to Chemical Degradation Induced by Organic Peroxide. Cell Biochem. Biophys. 75, 319–333. doi: 10.1007/s12013-017-0793-6
Zarjou, A., Jeney, V., Arosio, P., Poli, M., Zavaczki, E., Balla, G., et al. (2010). Ferritin ferroxidase activity: a potent inhibitor of osteogenesis. J. Bone Miner. Res. 25, 164–172. doi: 10.1359/jbmr.091002
Zhang, S., He, Y., Sen, B., and Wang, G. (2020a). Reactive oxygen species and their applications toward enhanced lipid accumulation in oleaginous microorganisms. Bioresour. Technol. 307:123234. doi: 10.1016/j.biortech.2020.123234
Zhang, Y., Roh, Y. J., Han, S. J., Park, I., Lee, H. M., Ok, Y. S., et al. (2020b). Role of Selenoproteins in Redox Regulation of Signaling and the Antioxidant System: a Review. Antioxidants 9, 383. doi: 10.3390/antiox9050383
Zhang, Z. D., Yang, Y. J., Liu, X. W., Qin, Z., Li, S. H., and Li, J. Y. (2021). Aspirin eugenol ester ameliorates paraquat-induced oxidative damage through ROS/p38-MAPK-mediated mitochondrial apoptosis pathway. Toxicology 453:152721. doi: 10.1016/j.tox.2021.152721
Zhao, F., Liu, Z. Q., and Wu, D. (2008). Antioxidative effect of melatonin on DNA and erythrocytes against free-radical-induced oxidation. Chem. Phys. Lipids 151, 77–84. doi: 10.1016/j.chemphyslip.2007.10.002
Zhao, G., Arosio, P., and Chasteen, N. D. (2006). Iron(II) and hydrogen peroxide detoxification by human H-chain ferritin. An EPR Spin-Trapping Study. Biochem. 45, 3429–3436. doi: 10.1021/bi052443r
Zhao, R., Tao, L., Qiu, S., Shen, L., Tian, Y., Gong, Z., et al. (2020a). Melatonin rescues glucocorticoid-induced inhibition of osteoblast differentiation in MC3T3-E1 cells via the PI3K/AKT and BMP/Smad signalling pathways. Life Sci. 257:118044. doi: 10.1016/j.lfs.2020.118044
Zhao, Y., Guo, R., Li, L., Li, S., Fan, G., Zhao, X., et al. (2020b). Tongmai formula improves cardiac function via regulating mitochondrial quality control in the myocardium with ischemia/reperfusion injury. Biomed. Pharmacother. 132:110897. doi: 10.1016/j.biopha.2020.110897
Zhou, L., Song, H., Zhang, Y., Ren, Z., Li, M., and Fu, Q. (2020). Polyphyllin VII attenuated RANKL-induced osteoclast differentiation via inhibiting of TRAF6/c-Src/PI3K pathway and ROS production. BMC Musculoskelet. Disord. 21:112. doi: 10.1186/s12891-020-3077-z
Zhou, Q., Zhu, L., Zhang, D., Li, N., Li, Q., Dai, P., et al. (2016). Oxidative Stress-Related Biomarkers in Postmenopausal Osteoporosis: a Systematic Review and Meta-Analyses. Dis. Markers 2016:7067984. doi: 10.1155/2016/7067984
Zhou, W., Liu, Y., Shen, J., Yu, B., Bai, J., Lin, J., et al. (2019). Melatonin Increases Bone Mass around the Prostheses of OVX Rats by Ameliorating Mitochondrial Oxidative Stress via the SIRT3/SOD2 Signaling Pathway. Oxid. Med. Cell Longev. 2019:4019619. doi: 10.1155/2019/4019619
Zhu, F., Duan, W., Zhong, C., Ji, B., and Liu, X. (2022). The protective effects of dezocine on interleukin-1β-induced inflammation, oxidative stress and apoptosis of human nucleus pulposus cells and the possible mechanisms. Bioengineered 13, 1399–1410. doi: 10.1080/21655979.2021.2017700
Keywords: postmenopausal osteoporosis, oxidative stress, antioxidant system, PI3K/AKT/Nrf2/HO-1, GSH/GSSG
Citation: Yang K, Cao F, Xue Y, Tao L and Zhu Y (2022) Three Classes of Antioxidant Defense Systems and the Development of Postmenopausal Osteoporosis. Front. Physiol. 13:840293. doi: 10.3389/fphys.2022.840293
Received: 21 December 2021; Accepted: 25 January 2022;
Published: 03 March 2022.
Edited by:
Daniele Lettieri Barbato, Tor Vergata University of Rome, ItalyReviewed by:
Zhipeng Fan, Capital Medical University, ChinaCopyright © 2022 Yang, Cao, Xue, Tao and Zhu. This is an open-access article distributed under the terms of the Creative Commons Attribution License (CC BY). The use, distribution or reproduction in other forums is permitted, provided the original author(s) and the copyright owner(s) are credited and that the original publication in this journal is cited, in accordance with accepted academic practice. No use, distribution or reproduction is permitted which does not comply with these terms.
*Correspondence: Lin Tao, dGFvbGluZHJAMTYzLmNvbQ==; Yue Zhu, emh1eXVlZHJAMTYzLmNvbQ==
†These authors have contributed equally to this work
Disclaimer: All claims expressed in this article are solely those of the authors and do not necessarily represent those of their affiliated organizations, or those of the publisher, the editors and the reviewers. Any product that may be evaluated in this article or claim that may be made by its manufacturer is not guaranteed or endorsed by the publisher.
Research integrity at Frontiers
Learn more about the work of our research integrity team to safeguard the quality of each article we publish.