- 1Henan International Joint Laboratory of Green Pest Control, College of Plant Protection, Henan Agricultural University, Zhengzhou, China
- 2State Key Laboratory for Biology of Plant Diseases and Insect Pests, Institute of Plant Protection, Chinese Academy of Agricultural Sciences, Beijing, China
Large numbers of chemosensory genes have been identified in the peripheral sensory organs of the pest Mythimna separata (Walker) to increase our understanding of chemoreception-related molecular mechanisms and to identify molecular targets for pest control. Chemosensory-related genes are expressed in various tissues, including non-sensory organs, and they play diverse roles. To better understand the functions of chemosensory-related genes in non-sensory organs, transcriptomic analyses of M. separata brains were performed. In total, 29 odorant-binding proteins (OBPs) and 16 chemosensory proteins (CSPs) putative genes were identified in the transcriptomic data set. The further examination of sex- and tissue-specific expression using RT-PCR suggested that eight OBPs (OBP5, -7, -11, -13, -16, -18, -21, and -24) and eight CSPs (CSP2–4, -8, CSP10–12, and -15) genes were expressed in the brain. Furthermore, bands representing most OBPs and CSPs could be detected in antennae, except for a few that underwent sex-biased expression in abdomens, legs, or wings. An RT-qPCR analysis of the expression profiles of six OBPs (OBP3–5, -9, -10, and -16) and two CSPs (CSP3 and CSP4) in different tissues and sexes indicated that OBP16 was highly expressed in male brain, and CSP3 and CSP4 were female-biased and highly expressed in brain. The expression levels of OBP5 and OBP10 in brain were not significantly different between the sexes. The findings expand our current understanding of the expression patterns of OBPs and CSPs in M. separata sensory and non-sensory tissues. These results provide valuable reference data for exploring novel functions of OBPs and CSPs in M. separata and may help in developing effective biological control strategies for managing this pest by exploring novel molecular targets.
Introduction
The oriental armyworm Mythimna separata (Walker) is a migratory and polyphagous pest species in China and other parts of Asia and Oceania (Jiang et al., 2011, 2014; Liu et al., 2016). The larvae of M. separata feed on more than 300 kinds of crops, including wheat, rice, corn, and cotton, resulting in serious yield losses. As with many other moth species, the M. separata adults rely heavily on olfaction to find host plants for food and mates for reproduction. To find the optimal chemical attractants for the control of the pest, the olfactory mechanisms of M. separata have been explored in many studies at the behavior, electrophysiology, and molecular levels (Mitsuno et al., 2008; Jiang et al., 2019, 2020; Wang et al., 2021). Thanks to advances in transcriptome sequencing techniques, a large number of chemosensory genes of M. separata, including genes for olfactory receptors (ORs), ionotropic receptors (IRs), sensory neuron membrane proteins, odorant-binding proteins (OBPs), and chemosensory proteins (CSPs), have been identified (Bian et al., 2017; Chang X. Q. et al., 2017; He et al., 2017; Liu et al., 2017; Du et al., 2018). The functions of some chemosensory-related proteins in M. separata, such as ORs, IRs, and CSPs, have also been well examined, and they are involved in sex pheromone, host volatiles and acid sensing (Mitsuno et al., 2008; Younas et al., 2018a,b, 2021; Zhang et al., 2019; Jiang et al., 2020; Tang et al., 2020; Wang et al., 2021). In addition to these proteins having known functional specificities, there is a large number of proteins of unknown specificity still awaiting experimental testing.
In general, chemosensory genes are expressed in the chemosensory organs of insects (Liu et al., 2012, 2015; Gu et al., 2013; Xiao et al., 2017; Li et al., 2020). However, chemosensory-related proteins are also present in various tissues and play diverse roles (Pelosi et al., 2017). Some insect chemosensory receptors have been identified in non-sensory organs, and their new physiological functions have been further clarified. For example, several gustatory receptors are expressed in the brains of Drosophila and Bombyx mori, and they are involved in the sensing of internal sugar and fructose nutrient cues, proprioception, hygroreception, and other sensory modalities (Thorne and Amrein, 2010; Miyamoto et al., 2012, Miyamoto and Amrein, 2014; Mang et al., 2016a,b). A subset of ORs are expressed in the testes of the malaria-causing mosquito, and their functions may be associated with sperm activation (Pitts et al., 2014). The OBPs and CSPs are small water-soluble proteins containing a hydrophobic pocket for ligand binding, and they mainly mediate the first step of olfactory signal transmission, which has been widely proven (Pelosi et al., 2017). In addition, the OBPs and CSPs have been detected in various tissues other than olfactory organs. For example, CSPs have been identified in the pheromone glands of Mamestra brassicae and B. mori (Jacquin-Joly et al., 2001; Dani et al., 2011). The OBP10 of Helicoverpa armigera was found on the egg surface (Sun et al., 2012), and OBPs and CSPs have been detected in the seminal fluids of Drosophila melanogaster, Aedes aegypti and Apis mellifera (Li et al., 2008; Takemori and Yamamoto, 2009; Baer et al., 2012). They have also been identified in venom glands of the parasitic wasps Leptopilina heterotoma and Pteromalus puparum (Heavner et al., 2013; Wang et al., 2015), in the eye of H. armigera (Zhu et al., 2016), and in the ovaries and eggshells of A. aegypti (Costa-da-Silva et al., 2013; Marinotti et al., 2014). These proteins may be involved in carrying semiochemicals that have various roles, such as in reproduction, regeneration, development, nutrition, anti-inflammatory action, and vision (Pelosi et al., 2017).
The chemosensory genes of M. separata identified from the transcriptomes of a head, antenna, palp, and proboscis also revealed that they have multiple points of origination (Bian et al., 2017; Chang X. Q. et al., 2017; He et al., 2017; Liu et al., 2017; Du et al., 2018). The antennal transcriptomes of M. separata revealed 37 OBPs and 14 CSPs in one study, and 32 OBPs and 16 CSPs in another (Chang X. Q. et al., 2017; He et al., 2017). Two studies of M. separata head transcriptomes revealed 50 OBPs and 22 CSPs, and 38 OBPs and 18 CSPs, respectively (Bian et al., 2017; Liu et al., 2017). More chemosensory genes in head compared with antennal transcriptomes may indicate that some of the genes are expressed in the brain. Previously, some chemosensory proteins, such as OBPs, CSPs, ORs, and gustatory receptors, were identified in insect brain tissues, and it was hypothesized that these proteins performed important unknown physiological functions as well as the specific known physiological functions (Miyamoto et al., 2012, Miyamoto and Amrein, 2014; Mang et al., 2016a,b; Walker et al., 2019; Wang et al., 2020).
In the present study, RNA-Seq was applied to mine OBPs and CSPs genes from the brain transcriptome of M. separata, and then semi-quantitative RT-PCR and RT-qPCR were used to confirm the expression patterns of OBPs and CSPs in different sexes and tissues. The findings serve as a foundation for exploring novel functions of chemosensory genes in insect brains and provide new pest control targets.
Materials and Methods
Insects Rearing and Tissue Collection
Larvae of M. separata were collected in Xinxiang, Henan Province, China. The colony was reared on an artificial diet in the laboratory and maintained under the conditions of 27 ± 1°C, 75 ± 5% relative humidity, and a 14-h/10-h light/dark cycle. Pupae of different sexes were separated in glass Petri dishes before eclosion. Adult moths were provided with sucrose solution 10% (v/v). Brains, antennae, wings, legs and abdomens of unmated moths were collected 2–4 days after eclosion, immediately frozen in liquid nitrogen, and stored at −70°C for RNA extraction.
cDNA Library Construction and Transcriptome Sequencing
Total RNA extracted from brains of approximately 600 adult males and females independently were used to construct separately three female and three male cDNA libraries. The libraries were sequenced using the PE100 strategy on the Illumina HiSeq™ 2000 platform (Illumina, San Diego, CA, United States) at Novogene Bioinformatics Technology Co. Ltd. (Beijing, China). Briefly, mRNA was purified from total RNA using magnetic beads with Oligo (dT), and then, it was fragmented into short fragments after adding fragmentation buffer. First-strand cDNA was synthesized using random hexamer primer and M-MuLV reverse transcriptase (RNase H). Subsequently, the second-strand cDNA was synthesized using DNA polymerase I and RNase H. The double-stranded cDNA was purified using the AMPure XP system (Beckman Coulter, Beverly, MA, United States). NEBNext Adaptors having a hairpin loop structure were ligated to prepare for hybridization after the adenylation of the DNA fragments’ 3′ ends. Library fragments were purified using the AMPure XP system for selecting preferentially cDNA fragments of 150–200 bp. Then, the selected fragments were used as templates for PCR amplification. PCR products were also purified using the AMPure XP system, and library quality was assessed on an Agilent 2100 Bioanalyzer and with a Q-PCR system.
Brain Transcriptome Assembly and Functional Annotation
A de novo transcriptome was assembled using the paired-reads mode with default parameters using the short-read program Trinity (Grabherr et al., 2011). Trinity outputs were clustered using TGICL (Pertea et al., 2003). The consensus cluster sequences and singletons made up the final unigene dataset. The generation of unigenes was performed using BLASTx and BLASTn programs against the public databases, with an E-value threshold of 10–5. GO terms were extracted from the best hits obtained from BLASTx against the NR database using the Blast2GO program (Conesa et al., 2005). A GO functional classification of all the unigenes was performed using WEGO software.1 KOG and KEGG annotations were performed using Blastall software against the KOG2 and KEGG3 databases, respectively.
Identification of Putative OBPs and CSPs Genes
Candidate unigenes encoding putative OBPs and CSPs were selected on the basis of the NR annotation results in the remote server. All the candidate chemosensory genes were further manually checked using the BLASTx program. The open reading frame (ORF) of each candidate unigene was predicted using the ORF finder tool.4 The putative signal peptides of OBP and CSP protein sequences were predicted using SignalP 4.1.5 In addition, all the candidate genes were compared with previously reported sequences using the BLASTn program (with an E-value threshold of 10–5) to identify novel OBPs and CSPs genes (Du et al., 2018). These genes were named in accordance with gene naming rules by adding a suffix with a number to indicate the descending order of their coding region lengths.
Phylogenetic Analyses of Odorant-Binding Protein and Chemosensory Protein Family Proteins
Multiple alignments of amino acid sequences were performed using the online prediction website MAFFT.6 The phylogenetic trees were constructed using the maximum-likelihood method with a bootstrap analysis of 1,000 replicates and the JTT with Freqs. (+F) Substitution Model using MEGA5.2 (Tamura et al., 2011). The phylogenetic trees were visualized using FigTree v1.4.3.7 OBPs data sets contained 29 candidate OBPs from M. separata, and 150 from other Lepidopteran moths, including B. mori (Gong et al., 2009), H. armigera (Liu et al., 2012), Helicoverpa assulta (Chang H. et al., 2017), Spodoptera exigua (Liu et al., 2015), Heliothis virescens (Vogel et al., 2010), and Spodoptera litura (Gu et al., 2015). CSPs data sets contained 16 putative CSPs from M. separata, and 72 from other Lepidopteran moths, including B. mori (Gong et al., 2009), H. assulta (Chang H. et al., 2017), H. armigera (Zhang J. et al., 2015), H. virescens (Picimbon et al., 2001), Agrotis ipsion (Gu et al., 2014), S. litura (Zhang Y. N. et al., 2015), and S. exigua (Liu et al., 2015). The amino acid sequences used in the phylogenetic analyses are listed in Supplementary Materials 1, 2.
Tissue- and Sex-Specific Expression Analyses of OBPs and CSPs
To confirm the expression profiles of the identified OBPs and CSPs genes, semi-quantitative PCR (RT-PCR) was performed. Total RNA was isolated from brains, antennae, wings, legs, and abdomens of 50–60 adults and extracted using TRIzol reagent (Invitrogen, Carlsbad, CA, United States) following the manufacturer’s instructions. Single-stranded cDNA templates were synthesized from 1 μg of total RNA from various tissue samples using the FastKing gDNA Dispelling RT SuperMix (TianGen, Beijing, China). Specific primers of predicted OBPs and CSPs genes were designed using Premier 5.0 (Supplementary Material 3 and Supplementary Table 1). PCR reactions were carried out using equal amounts of cDNA (200 ng) template. The β-actin (GenBank Acc. GQ856238.1) of M. separata was selected as the reference gene to test the integrity of the cDNA templates and also the expression quantification of the target genes. The PCR was performed in a Mastercycler® (Eppendorf, Hamburg, Germany) under the following conditions: 94°C for 5 min, 25–33 cycles (depending on the expression level of each gene) of 94°C for 30 s, 56°C for 30 s, and 72°C for 30 s, followed by a final extension at 72°C for 10 min. PCR products were analyzed on 1.0% agarose gels and visualized after staining with ethidium bromide.
The RT-qPCR analysis was conducted using an ABI QuantStudio3 (Applied Biosystems, Carlsbad, CA, United States). The specific RT-qPCR primers were designed using Beacon Designer 8.13 (PREMIER Biosoft International, CA, United States) (Supplementary Material 3 and Supplementary Table 2). Two reference genes, β-actin (GenBank Acc. GQ856238.1) and glyceraldehyde-3-phosphate dehydrogenase (gapdh) (GenBank Acc. HM055756.1) were used to normalize target gene expression. The amplification efficiencies of the target and reference gene primers were evaluated using a four-fold serial dilution of cDNA templates from adult antennae. Reactions for each sample (20 μl) consisted of 10 μl of SuperReal PreMix Plus (TianGen, Beijing, China), 0.5 μl of each primer (10 μM), 0.4 μl of Rox reference dye, 1 μl of sample cDNA, and 7.6 μl of sterilized ultrapure water. Amplification conditions were an initial denaturation at 95°C for 3 min, followed by 40 cycles of 95°C for 15 s, and a single step for annealing and extension was performed at 60°C for 30 s. The PCR products were heated to 95°C for 15 s, cooled to 60°C for 1 min, heated to 95°C for 30 s, and cooled to 60°C for 15 s to determine the dissociation curves. The RT-qPCR reaction of each sample was performed in three technical replicates and three biological replicates. Then, we used the relative quantitation method (2–ΔΔCT) (Livak and Schmittgen, 2001) to evaluate quantitative variation. Transcript amounts were standardized to 1 using the sample from adult male brain. Data were analyzed using Data Processing System software version 9.5 (Tang and Zhang, 2013). A one-way analysis of variance with Tukey’s multiple comparison test was performed to analyze differences in gene expression levels among multiple samples, and p < 0.05 was considered to be significant.
Results
An Overview of Brain Transcriptomes
Six adult brain cDNA libraries, three for females and three for males of M. separata, were constructed and sequenced using the Illumina HiSeq™ 2000 platform. As a result, 61,283,994, 59,876,048, and 68,196,054 raw reads were produced from the three separate female brain samples; and 46,814,292, 60,931,890, and 43,177,818 raw reads were produced from the three separate male brain samples. After trimming the adaptor sequences, contaminating sequences, and low quality sequences, 56,689,466, 54,841,746, and 62,601,386 clean reads of the three separate female brain samples, and 43,297,968, 56,087,138, and 40,004,380 clean reads of the three separate male brain samples, remained for the following assembly (Supplementary Material 3 and Supplementary Table 3). Subsequently, all the clean reads were assembled together and generated 132,516 unigenes with lengths ranging from 201 to 28,894 bp, with a mean length of 579 bp.
Homology Searches and Functional Annotation of Mythimna separata Brain Unigenes
Homology searches querying the 132,516 unigenes against other insect species were performed using the BLASTx and BLASTn programs, with the E-value cut-off of 1.0E–5. In total, 27,594 unigenes (20.82%) had BLASTx hits in the NR database, and 12,499 unigenes (9.43%) had BLASTn hits in the NT database. Among the annotated unigenes, 4445 (3.35%) were annotated in all of the databases [NR, NT, KO (KEGG ontology), SwissProt, protein family (PFAM), GO, and KOG], whereas 35,484 (26.77%) were annotated in at least one database (Supplementary Material 3 and Supplementary Table 4). The analysis showed that most M. separata protein sequences were orthologs of proteins in B. mori (33.5%), Danaus plexippus (15.5%), and Plutella xylostella (14.1%) (Figure 1).
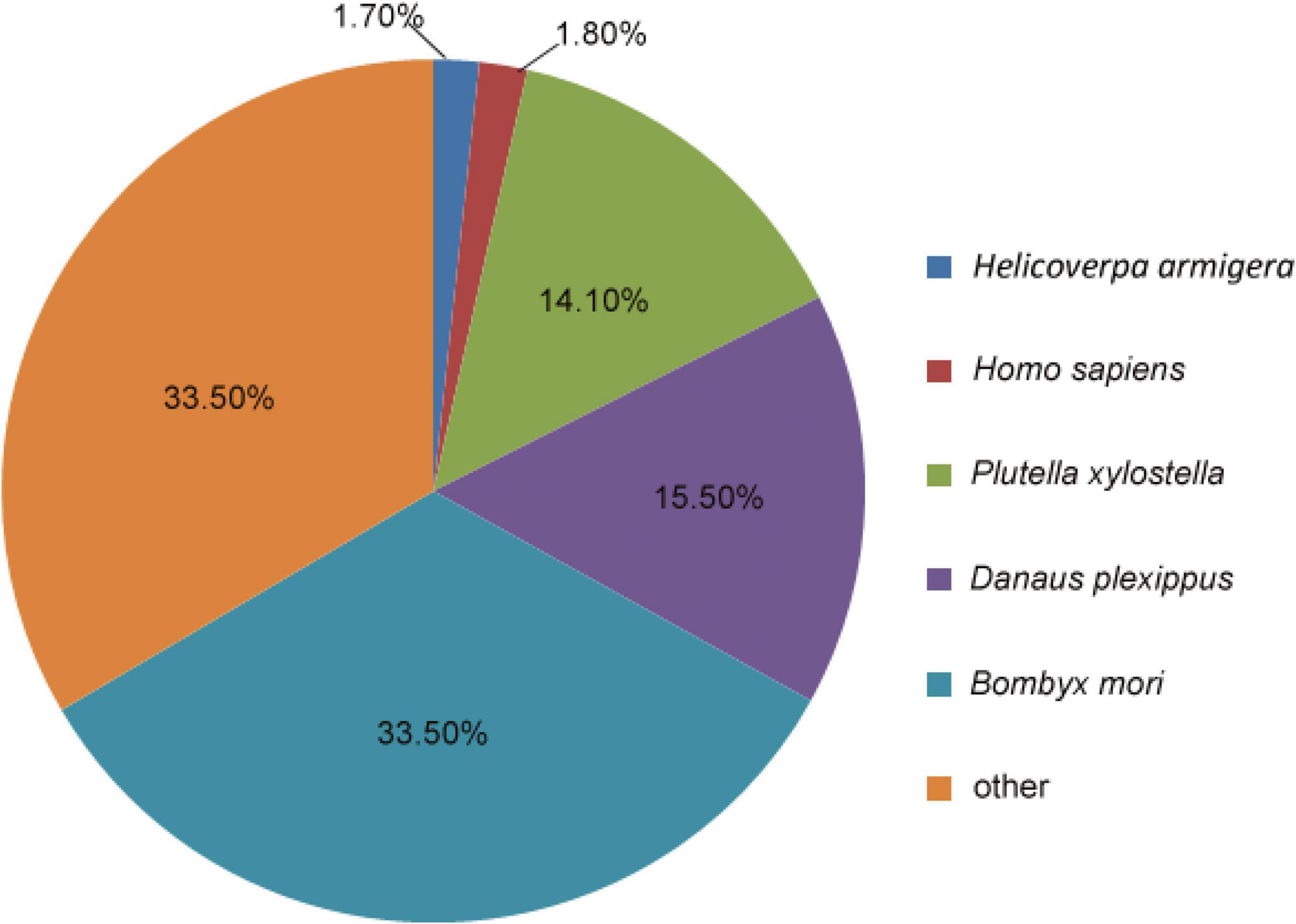
Figure 1. Proportional homology distribution among other insect species based on the best BLAST hits against the NR database for the assembled unigenes from the Mythimna separata brain transcriptomes.
According to the GO category analysis, only 21,188 (15.99%) assembled unigenes corresponded to different functional groups. Because one unigene can align to multiple GO categories, 54,623 (41.22%) unigenes were assigned to biological process, 33,526 (25.30%) to cellular component, and 23,545 (17.77%) to molecular function. In the molecular function category, the terms of binding and catalytic activity were the most represented. In the cellular component terms, cell and cell part were the most abundant. In the biological process category, cellular process, metabolic process, and single-organism process were most abundant (Figure 2A).
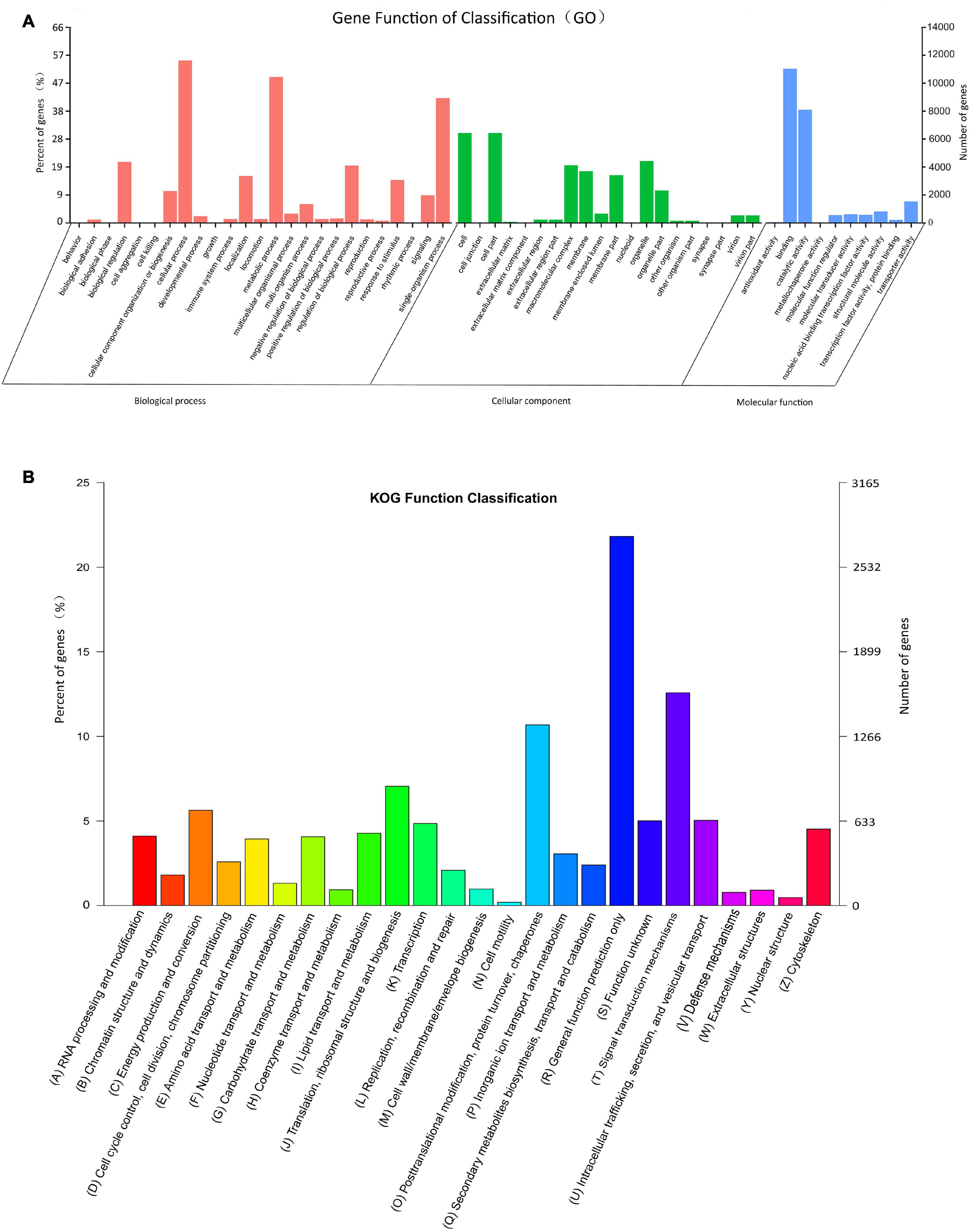
Figure 2. Histograms of gene ontology (GO) classifications (A) and clusters of orthologous groups of proteins (KOG) (B). (A) The GO classifications are summarized into three main categories: biological processes, cellular component, and molecular function. The right y-axis indicates the number of genes in a category, and the left y-axis indicates the percentage of genes in a specific term in that main category. (B) The x-axis indicates 26 categories. The left y-axis indicates the percentage of a specific gene classification in that main category, and the right y-axis indicates the number of genes in a category.
In the obtained KOG functional annotation, 12,672 unigenes were categorized into 26 functional groups (Figure 2B). “General function prediction only” was the largest group (2764, 21.81%), followed by the “Signal transduction mechanism (1594, 12.58%) and Posttranslational modification, protein turnover, chaperones” (1353, 10.68%) groups, and “Cell motility” (25, 0.20%) was the smallest group.
Identification of Putative OBPs and CSPs Genes
In the M. separata brain transcriptomes, 29 OBPs were annotated on the basis of the TBLASTN results. Among them, 16 OBPs contained intact ORFs, with lengths ranging from 133 to 334 amino acids (Table 1). Based on the numbers and locations of the conserved cysteines, the OBPs were classified into three categories, Classic, Pluc-C, and Minus-C OBPs families. Seven full-length OBPs (GOBP2, OBP6, OBP9, OBP11–13, and OBP15) had the typical six conserved cysteines and spacing, forming the Classic OBPs family. Three full-length OBPs (OBP4, -5, and -10) belonged to the Pluc-C OBPs family, having additional two, three, and six cysteines located downstream of the conserved C6. The remaining six full-length OBPs (OBP1–3, -8, -14, and -16) belonged to the Minus-C OBPs family. OBP1 lacked conserved cysteine C1; OBP2 had none of the typical six conserved cysteines; OBP3 only had conserved cysteines C1 and C6; and OBP8, -14, and -16 lacked the conserved cysteines C2 and C5 (Figure 3). Compared with our earlier identified OBPs in M. separata (Du et al., 2018), many of them shared high sequence identity levels, ranging from 80 to 100%. However, OBP2, -3, -17, and -23 shared a no more than 36% sequence identity (Supplementary Material 3 and Supplementary Table 5).
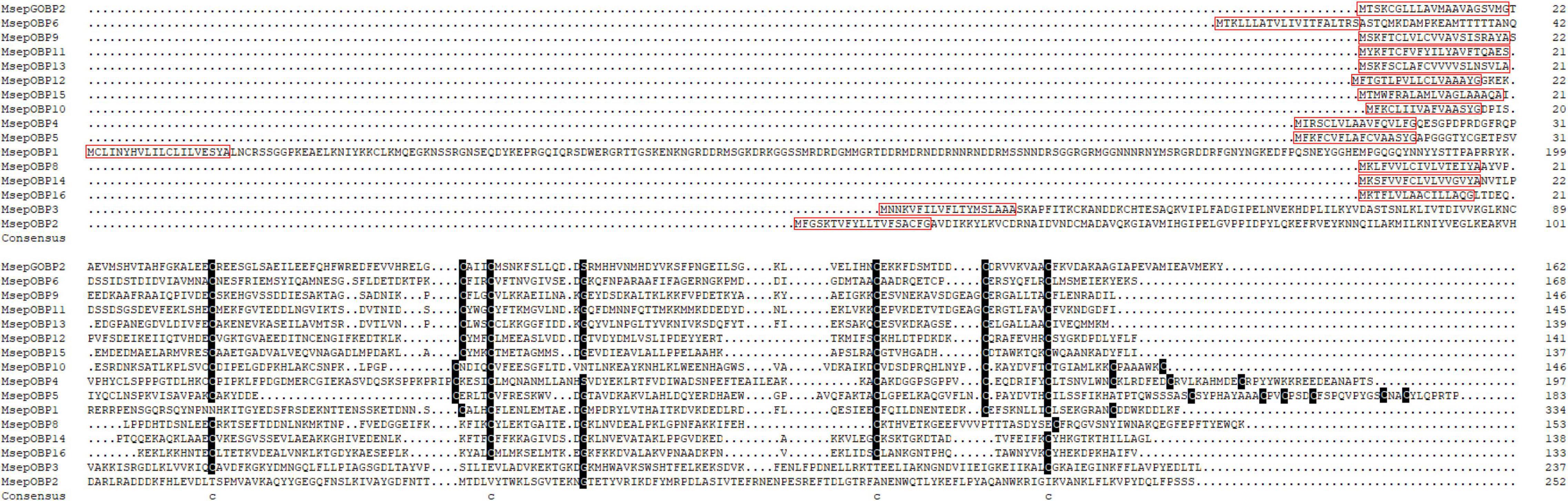
Figure 3. Multiple alignment of amino acid sequences of OBPs from M. separata. In the sequence alignments, only the proteins with full-length ORFs were selected. Conserved cysteine residues are highlighted, and signal peptides are boxed in red.
Sixteen transcripts encoding candidate CSPs were identified. Among them, 14 CSPs contained intact ORFs, with lengths ranging from 106 to 290 amino acids (Table 2). These identified full-length CSPs proteins included a signal peptide and four highly conserved cysteine profiles (C1-X6-C2-X18-C3-X2-C4, where X represents any amino acid) (Figure 4). The CSP15 and CSP16 amino acid sequences were incomplete due to the lack of a 3′ or 5′ terminus. Compared with previously identified CSPs (Du et al., 2018), most of them shared high sequence identity levels, ranging from 74 to 100%. However, there were two CSPs, CSP9 and CSP12, that shared less than a 41% sequence identity (Supplementary Material 3 and Supplementary Table 5).
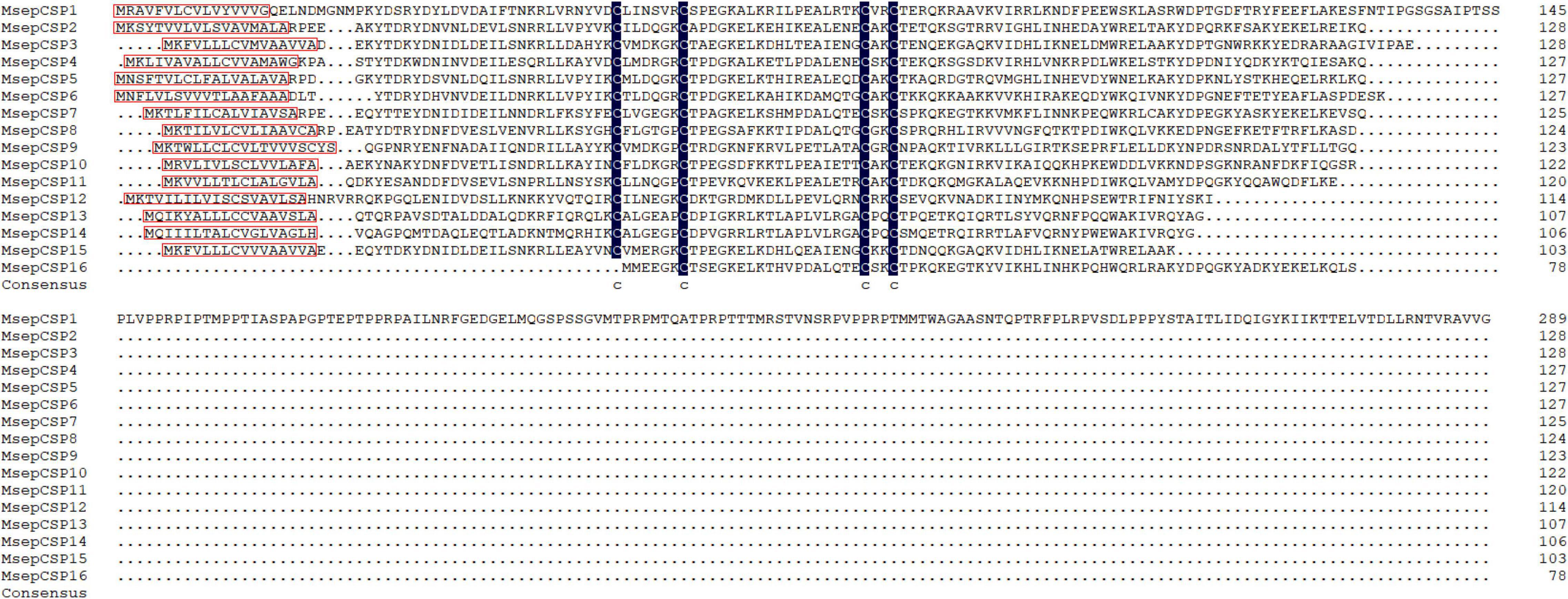
Figure 4. Multiple alignment of amino acid sequences of CSPs from M. separata. In the sequence alignments, the C-terminus of CSP15 and N-terminus of CSP16 are truncated. Four conserved residues are highlighted, and signal peptides are boxed in red.
Phylogenetic Analysis of Odorant-Binding Proteins and Chemosensory Proteins
All the putative OBPs clustered with at least one lepidopteran ortholog in the phylogenetic tree (Figure 5). The identified GOBP and PBP protein sequences in brain clustered into the GOBP and PBP clades in the phylogenetic tree, respectively. OBP5 and OBP7 shared a 68.28% sequences identity and clustered together. OBP11 and OBP18 only shared a 29.66% similarity, but they also clustered together. With OBP13, they formed a clade containing SlitOBP25. All the candidate CSPs proteins clustered with at least one lepidopteran ortholog in the phylogenetic tree (Figure 6). CSP3 and CSP15 shared a 67.97% sequences identity, and formed a clade with AipsCSP7. CSP7 and CSP16 shared a 45.60% sequences identity and clustered together.
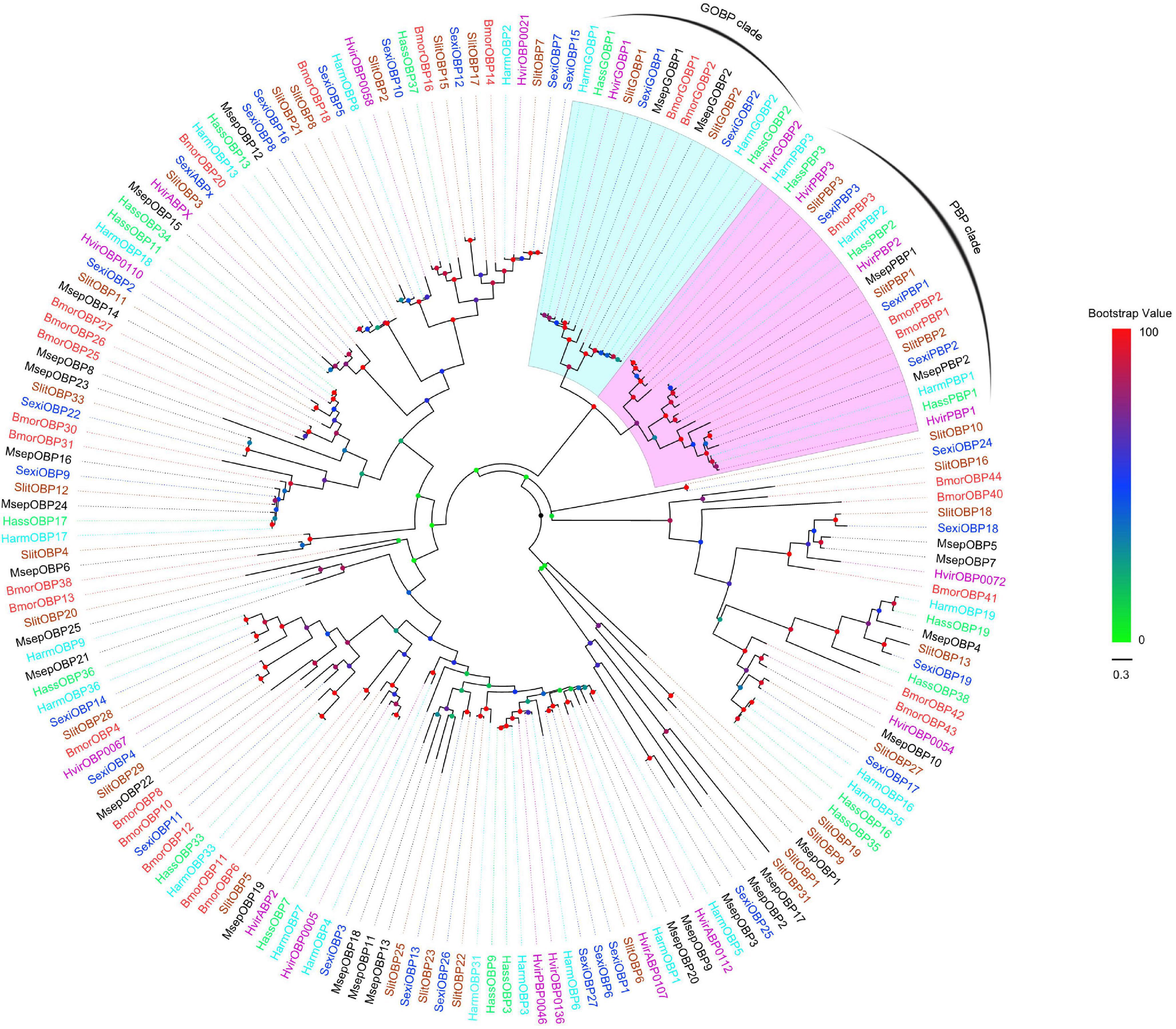
Figure 5. Phylogenetic tree of putative OBPs from lepidopteran species. This tree was constructed using MEGA5.2 based on alignment results of MAFFT. Msep: Mythimna separata (black); Harm: Helicoverpa armigera (cyan); Hass: Helicoverpa assulta (green); Bmor: Bombyx mori (red); Hvir: Heliothis virescens (dark violet); Sexi: Spodoptera exigua (blue) Slit: Spodoptera litura (sandy brown). The clades in violet and light cyan represent general odorant-binding proteins and pheromone-binding proteins, respectively.
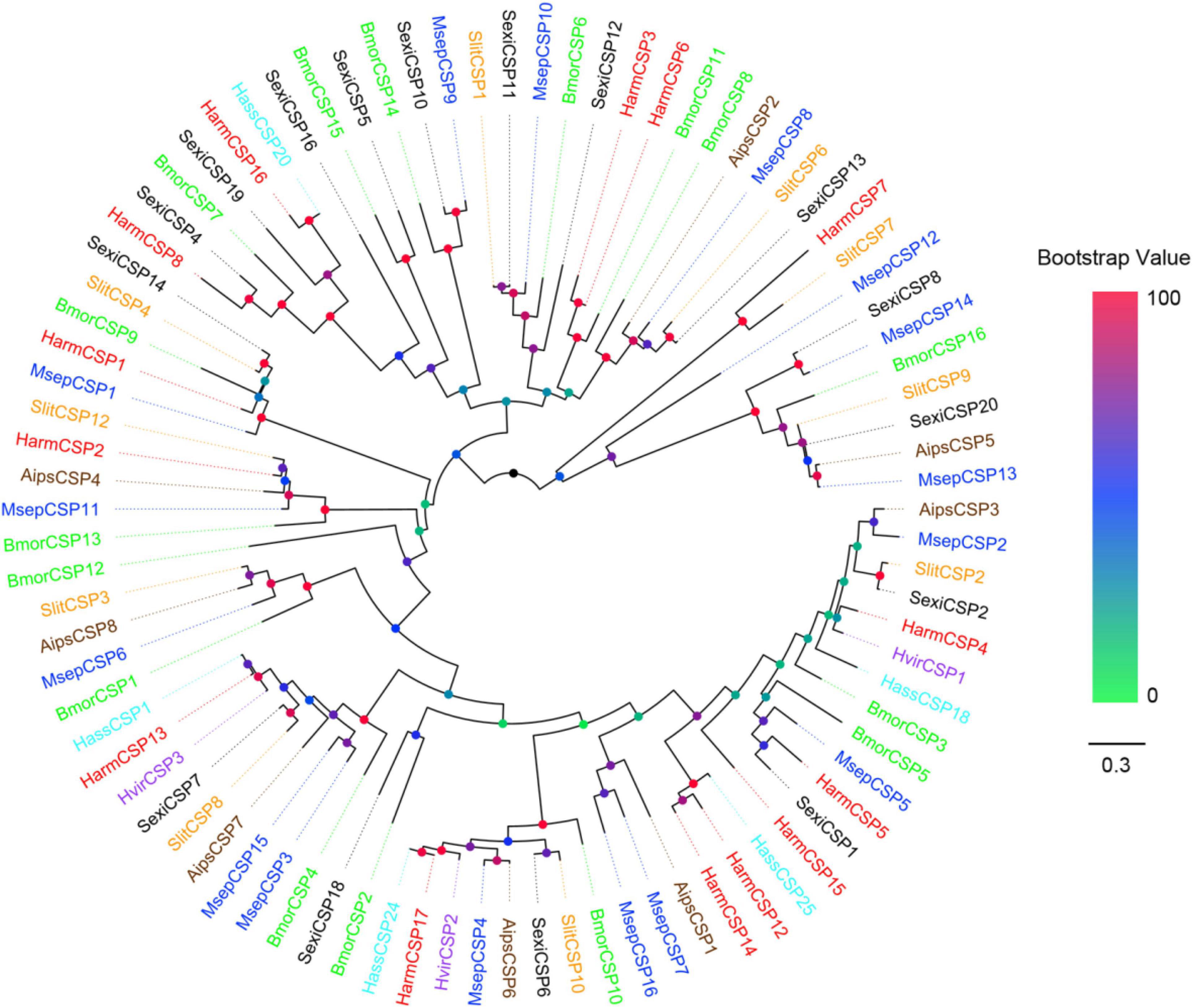
Figure 6. Phylogenetic tree of putative CSPs from lepidopteran species. This tree was constructed using MEGA5.2 based on alignment results of MAFFT. Msep: Mythimna separata (blue); Aips: Agrotis ipsilon (brown); Harm: Helicoverpa armigera (red); Hvir: Heliothis virescens (dark orchid); Hass: Helicoverpa assulta (cyan); Bmor: Bombyx mori (green); Slit: Spodoptera litura (orange); Sexi: Spodoptera exigua (black).
Expression Profiles of Putative OBPs and CSPs Genes
The RT-PCR expression profiles indicated that the majority of OBPs genes were expressed in the antennae. OBP5, -7, -11, -13, -16, -18, -21, and -24 were detected in the brain. Among them, OBP5, -7, -11, and -13 showed male brain-biased expression, whereas OBP21 showed female brain-biased expression. OBP16, -18, and -24 were expressed in both female and male brains. OBP5, -7, and -11 were also detected in the antennae and abdomens. OBP13 could be detected in the abdomens. OBP16 and OBP24 were detected in the antennae, abdomens, and legs. OBP18 could be detected in all the tissues, and OBP21 was detected in the legs and wings (Figure 7). The RT-PCR expression profiles indicated that most CSPs genes were expressed in all the examined tissues (Figure 7). Three CSP genes, CSP2–4, were expressed in female brain. CSP8 was detected in male brain, and CSP10–12, and CSP15 were detected in both female and male brains (original gel images of RT-PCR can be found in Supplementary Material 4).
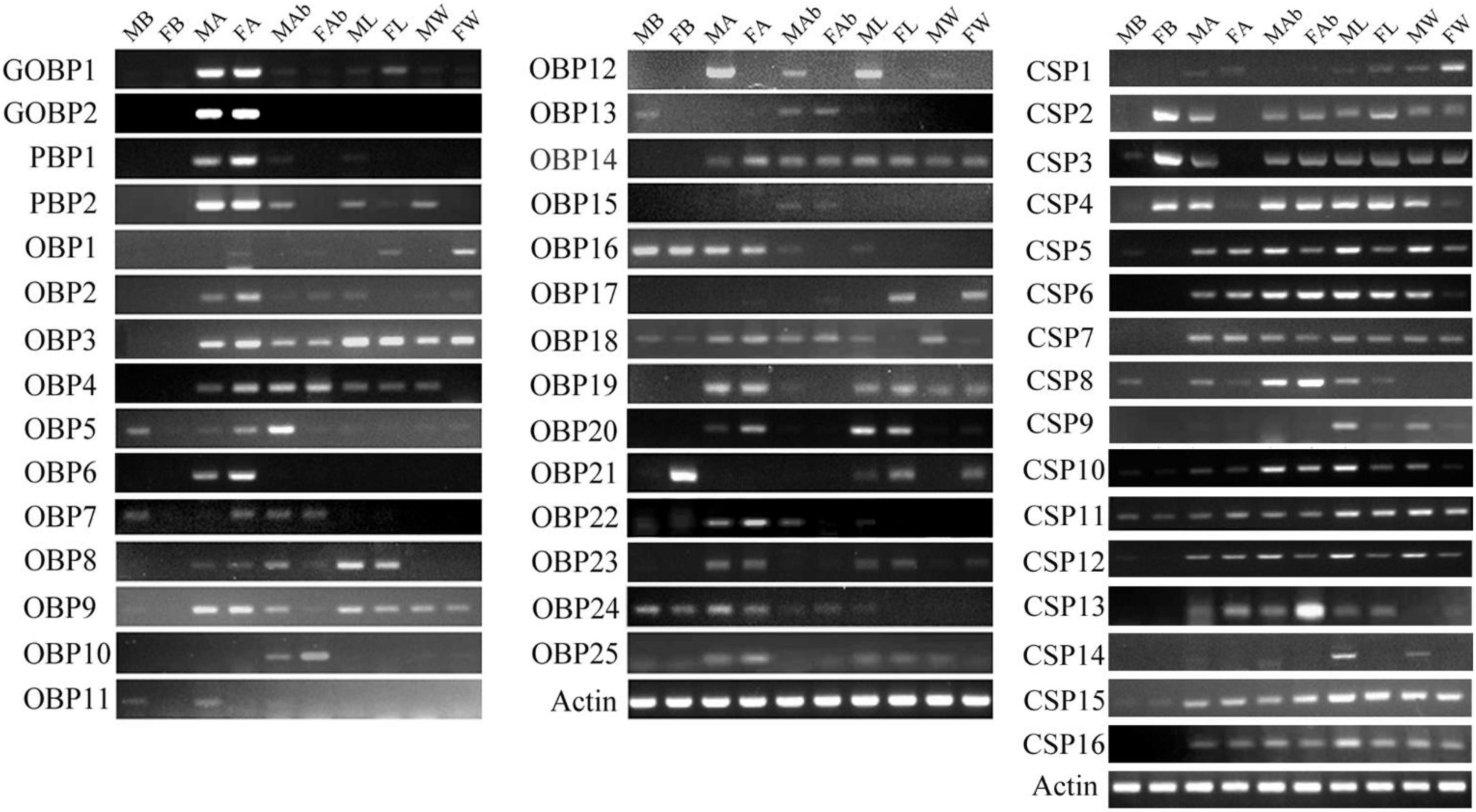
Figure 7. Mythimna separata OBPs and CSPs transcript levels in different tissues of male and female adults as evaluated by RT-PCR. MB: male brains; FB: female brains; MA: male antennae; FA: female antennae; MAb: male abdomens; FAb: female abdomens; ML: male legs; FL: female legs; MW: male wings; FW: female wings.
To confirm the RT-PCR results, RT-qPCR was performed to measure quantitatively the expression levels of six OBPs (OBP3–5, -9, -10, and -16) and 2 CSPs (CSP3 and CSP4) genes in the various tissues (Figure 8). The RT-qPCR results were mostly consistent with the RT-PCR results. They confirmed that OBP5, OBP16, CSP3, and CSP4 were expressed in brain, and further revealed that OBP10, which was not detected by RT-PCR, was also expressed in brain. The expression levels of OBP5 and OBP10 in brain were not significantly different between the sexes (p > 0.05), whereas that of OBP16 was three times higher in male brain than in female brain (p < 0.05). The expression levels of CSP3 and CSP4 were 40 and 24 times higher in female brain than in the male, respectively (p < 0.05).
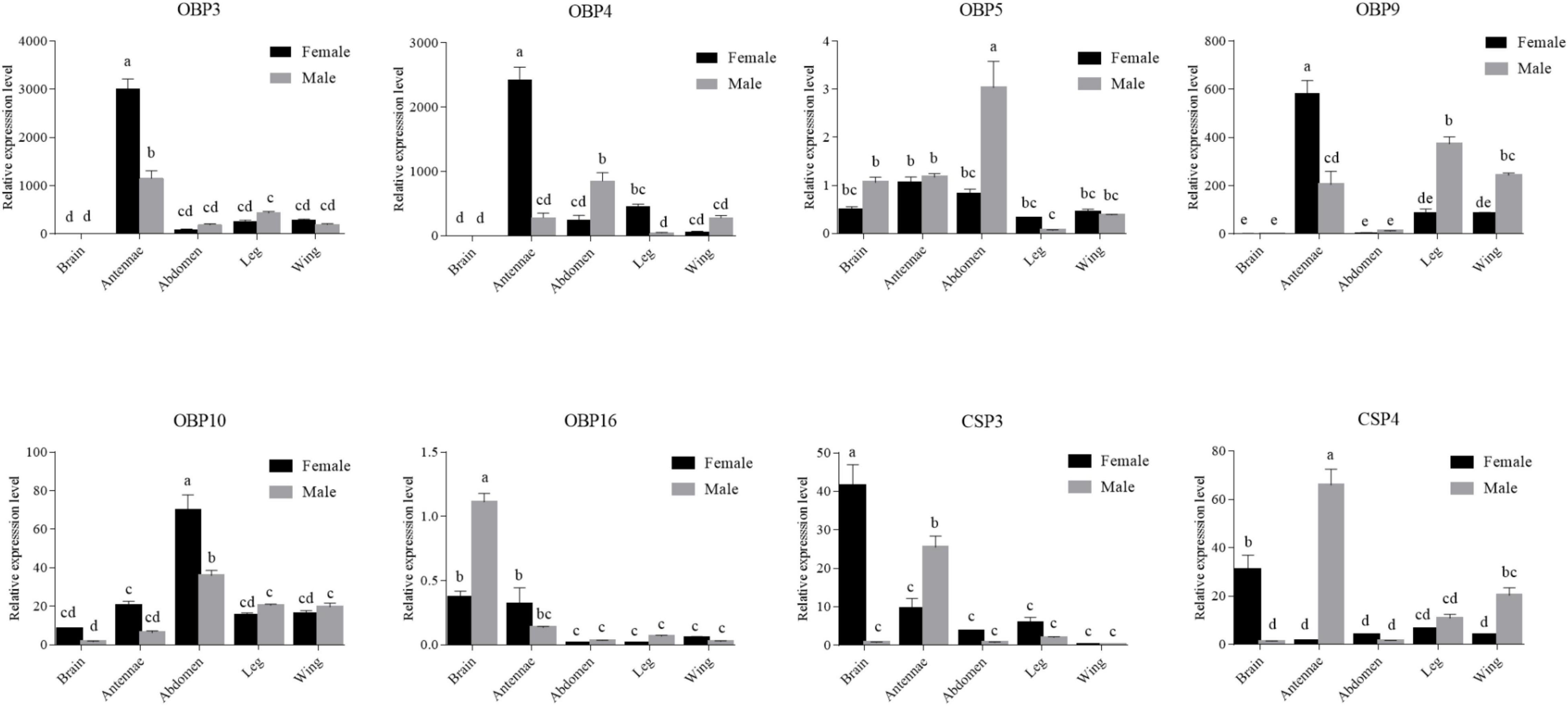
Figure 8. Six OBPs and two CSPs transcript levels in different tissues of both sexes as evaluated by RT-qPCR. The internal controls β-actin and gapdh were used to normalize transcript levels in each sample. The standard error is represented by the error bar, and the different letters above each bar denote significant differences (p < 0.05).
Discussion
In the present study, we first sequenced and analyzed the transcriptomes of adult male and female M. seperata brains. Among the 132,516 unigenes identified, only 15.98% were annotated to one or more GO term, and only 20.82% had homologous matches to entries in the NCBI NR protein database. This was similar to other lepidopteran species (Liu et al., 2012; Zhang Y. N. et al., 2015), indicating that a large number of M. seperata genes are either non-coding or homologs of genes that have not been annotated to GO terms. Importantly, we identified 29 OBPs and 16 CSPs putative genes in the data set, providing valuable reference data for exploring novel functions of chemosensory genes in M. separata.
The number of OBPs obtained in this study was less than the number identified from the antennal (37 and 32) and head (50 and 38) transcriptomes of M. separata (Bian et al., 2017; Chang X. Q. et al., 2017; He et al., 2017; Liu et al., 2017). Here, we identified two novel OBPs (OBP2 and OBP3) and two OBPs (OBP17 and OBP23) that share a no more than 36% sequence identity compared with our earlier identified 38 OBPs from the antennae, labial palps, and proboscises transcriptomes of M. separata (Du et al., 2018). The other OBPs shared high sequences identity levels, ranging from 80% to 100% (Supplementary Material 3 and Supplementary Table 5). The small number of OBPs identified in brain may be because OBPs genes are mainly expressed in antennae, mouth organs, and other chemosensory structures. We identified 16 CSPs in the adult brain transcriptome, which is comparable with the numbers identified from earlier reported antennal transcriptomes, 14 and 16 CSPs reported by Chang X. Q. et al. (2017) and He et al. (2017), respectively, and head transcriptomes of M. separate, 18 CSPs reported by Liu et al. (2017), but fewer than the numbers identified in other head transcriptomes (22 CSPs) by Bian et al. (2017) and our earlier analyzed antennae, labial palps, and proboscises transcriptomes (38 CSPs) (Du et al., 2018). However, we also found that two CSPs (CSP9 and CSP12) shared less than a 41% sequence identity compared with our earlier identified CSPs. Most other CSPs shared high sequence identity levels, ranging from 74 to 100% (Supplementary Material 3 and Supplementary Table 5). The novel and relatively low sequence homology levels of OBPs and CSPs identified in the present study may indicate that they are specifically expressed and function in the brain, and their ligand-binding functions need to be investigated in the future.
The further examination of sex- and tissue-specific expression using RT-PCR confirmed that eight OBPs (OBP5, -7, -11, -13, -16, -18, -21, and -24) and eight CSPs (CSP2–4, -8, CSP10–12, and -15) were expressed in brain. The RT-qPCR results indicated that OBP5, OBP10, OBP16, CSP3, and CSP4 have relatively abundant and sex-biased expression levels in adult brain. These findings in M. separata brain are consistent with previous research on other insect species. For example, at least two OBPs and three CSPs were identified in the brain of Spodoptera littoralis. The genes with relatively abundant expression levels in the brain were SlitOBP4, SlitPBP2, SlitCSP1, SlitCSP2, and SlitCSP8 (Walker et al., 2019). Four OBPs were identified in the brain transcriptome of Vespa velutina (Wang et al., 2020). In the brain of Adelphocoris lineolatus, AlinOBP14 was identified (Tian et al., 2021). In A. mellifera, there are six CSPs and most have been detected in the brain (Liu et al., 2020). Indeed, several studies have reported their putative physiological functions as carriers for endogenous compounds in brain. In situ hybridization with mRNA of AlinOBP14 showed that the gene was expressed in the antennal lobe of the brain and fluorescence-based competitive-binding assays showed that juvenile hormone and the precursors of the hormone bound to the AlinOBP14 protein (Sun et al., 2019; Tian et al., 2021). Therefore, AlinOBP14 in the antennal lobes of A. lineolatus might function as a carrier of endogenous compounds, including juvenile hormone and hormone precursors. Juvenile hormone affects the responsiveness of olfactory interneurons in the antennal lobe and is likely involved in the plasticity of the insect brain (Anton and Gadenne, 1999). The possible roles of CSPs in the nervous system has also been demonstrated using gene knockout assays. A CSP AmelGB10389 knockout in honey bee resulted in abnormal brain development, which suggests the CSPs may play roles in neuronal plasticity (Maleszka et al., 2007; Liu et al., 2020). The CSPs may function as carriers of lipids and juvenile hormone to modulate olfactory responses, olfactory learning, and memorization in the antennal lobe and mushroom body neuropiles (Liu et al., 2020). The expression of CSPs in the neural systems of insects may function, by controlling diacylglycerol and protein phosphorylation, in neuroplasticity, neurogenesis, synaptogenesis, the formation of new synapses, and the generation of new neuron connections (Liu et al., 2020).
OBPs and CSPs are typically expressed in chemosensory structures, such as antennae and mouth organs, and their proteins function as carriers of odorants in insect chemoreception (Pelosi et al., 2017). However, OBPs and CSPs proteins in other insect species are endowed with multiple functions in the non-sensory organs of the insect body, such as pheromone delivery, solubilization of nutrients, development, and insecticide resistance (Pelosi et al., 2017). The varying expression patterns of OBPs and CSPs genes across tissues of M. separata may also suggest that their proteins play broader physiological roles in addition to carrying odorants. In particular, in the present study, the expression of several OBPs and CSPs in M. separata brain may expand our current understanding of the expression patterns of chemosensory genes in insect non-sensory tissues, and the results establish a foundation for further studies on the novel functions of chemosensory genes in non-sensory tissues of M. separata.
Data Availability Statement
The datasets presented in this study can be found in online repositories. The names of the repository/repositories and accession number(s) can be found below: https://www.ncbi.nlm.nih.gov/sra/PRJNA793072.
Author Contributions
W-BC and X-CZ conceived and designed the experiments, wrote the manuscript. W-BC, L-XD, and X-CZ performed the experiments. X-YG, L-LS, L-LC, G-YX, and S-HA analyzed the data. All authors have read and approved the manuscript for publication.
Funding
This study was funded by grants from the National Natural Science Foundation of China (Nos. 32130089 and 32001912), Department of Science and Technology of Henan Province Key Research and Development and Promotion Special Projects (No. 202102110226), and the Support Program for Young Talents in Henan Province (No. 2020HYTP043).
Conflict of Interest
The authors declare that the research was conducted in the absence of any commercial or financial relationships that could be construed as a potential conflict of interest.
Publisher’s Note
All claims expressed in this article are solely those of the authors and do not necessarily represent those of their affiliated organizations, or those of the publisher, the editors and the reviewers. Any product that may be evaluated in this article, or claim that may be made by its manufacturer, is not guaranteed or endorsed by the publisher.
Supplementary Material
The Supplementary Material for this article can be found online at: https://www.frontiersin.org/articles/10.3389/fphys.2022.839559/full#supplementary-material
Footnotes
- ^ http://wego.genomics.org.cn/cgi-bin/wego/index.pl
- ^ http://www.ncbi.nlm.nih.gov/COG/
- ^ http://www.genome.jp/kegg/
- ^ http://www.ncbi.nlm.nih.gov/gorf/gorf.html
- ^ http://www.cbs.dtu.dk/services/SignalP/
- ^ https://www.ebi.ac.uk/Tools/msa/mafft/
- ^ http://tree.bio.ed.ac.uk/software/figtree/
References
Anton, S., and Gadenne, C. (1999). Effect of juvenile hormone on the central nervous processing of sex pheromone in an insect. Proc. Natl. Acad. Sci. U.S.A. 96, 5764–5767. doi: 10.1073/pnas.96.10.5764
Baer, B., Zareie, R., Paynter, E., Poland, V., and Millar, A. H. (2012). Seminal fluid proteins differ in abundance between genetic lineages of honeybees. J. Proteomics 75, 5646–5653. doi: 10.1016/j.jprot.2012.08.002
Bian, H. X., Ma, H. F., Zheng, X. X., Peng, M. H., Li, Y. P., Su, J. F., et al. (2017). Characterization of the adult head transcriptome and identification of migration and olfaction genes in the oriental armyworm mythimna separata. Sci. Rep. 7:2324. doi: 10.1038/s41598-017-02513-6
Chang, H., Dong, A., Zhang, J., Dong, S., Liu, Y., and Wang, G. (2017). Candidate odorant binding proteins and chemosensory proteins in the larval chemosensory tissues of two closely related noctuidae moths, Helicoverpa armigera and H. assulta. PLoS One 12:e0179243. doi: 10.1371/journal.pone.0179243
Chang, X. Q., Nie, X. P., Zhang, Z., Zeng, F. F., Lv, L., Zhang, S., et al. (2017). De novo, analysis of the oriental armyworm, Mythimna separata, antennal transcriptome and expression patterns of odorant-binding proteins. Comput. Biochem. Phys. 22, 120–130. doi: 10.1016/j.cbd.2017.03.001
Conesa, A., Götz, S., García-Gómez, J. M., Terol, J., Talón, M., and Robles, M. (2005). Blast2GO: a universal tool for annotation, visualization and analysis in functional genomics research. Bioinformatics 21, 3674–3676. doi: 10.1093/bioinformatics/bti610
Costa-da-Silva, A. L., Kojin, B. B., Marinotti, O., James, A. A., and Capurro, M. L. (2013). Expression and accumulation of the two-domain odorant-binding protein AaegOBP45 in the ovaries of blood-fed Aedes aegypti. Parasite Vector 6:364. doi: 10.1186/1756-3305-6-364
Dani, F. R., Michelucci, E., Francese, S., Mastrobuoni, G., Cappellozza, S., La Marca, G., et al. (2011). Odorant-binding proteins and chemosensory proteins in pheromone detection and release in the silkmoth bombyx mori. Chem. Senses 36, 335–344. doi: 10.1093/chemse/bjq137
Du, L., Zhao, X., Liang, X., Gao, X., Liu, Y., and Wang, G. (2018). Identification of candidate chemosensory genes in Mythimna separata by transcriptomic analysis. BMC Genom. 19:518. doi: 10.1186/s12864-018-4898-0
Gong, D. P., Zhang, H. J., Zhao, P., Xia, Q. Y., and Xiang, Z. H. (2009). The odorant binding protein gene family from the genome of silkworm, bombyx mori. BMC Genom. 10:332. doi: 10.1186/1471-2164-10-332
Grabherr, M. G., Haas, B. J., Yassour, M., Levin, J. Z., Thompson, D. A., Amit, I., et al. (2011). Full length transcriptome assembly from RNA-Seq data without a reference genome. Nat. Biotechnol. 29:644. doi: 10.1038/nbt.1883
Gu, S. H., Sun, L., Yang, R. N., Wu, K. M., Guo, Y. Y., Li, X. C., et al. (2014). Molecular characterization and differential expression of olfactory genes in the antennae of the black cutworm moth Agrotis ipsilon. PLoS. One 9:e103420. doi: 10.1371/journal.pone.0103420
Gu, S. H., Wu, K. M., Gu, Y. Y., Pickett, J. A., Field, L. M., Zhou, J. J., et al. (2013). Identification of genes expressed in the sex pheromone gland of the black cutworm Agrotis ipsilon with putative roles in sex pheromone biosynthesis and transport. BMC Genom. 14:636. doi: 10.1186/1471-2164-14-636
Gu, S. H., Zhou, J. J., Gao, S., Wang, D. H., Li, X. C., Guo, Y. Y., et al. (2015). Identification and comparative expression analysis of odorant binding protein genes in the tobacco cutworm Spodoptera litura. Sci. Rep. 5:13800. doi: 10.1038/srep13800
He, Y. Q., Feng, B., Guo, Q. S., and Du, Y. (2017). Age influences the olfactory profiles of the migratory oriental armyworm Mythimna separata at the molecular level. BMC Genom. 18:32. doi: 10.1186/s12864-016-3427-2
Heavner, M. E., Gueguen, G., Rajwani, R., Pagan, P. E., Small, C., and Govind, S. (2013). Partial venom gland transcriptome of a drosophila parasitoid wasp, Leptopilina heterotoma, reveals novel and shared bioactive profiles with stinging hymenoptera. Gene 526, 195–204. doi: 10.1016/j.gene.2013.04.080
Jacquin-Joly, E., Vogt, R. G., FranÇois, M. C., and Nagnan-Le Meillour, P. (2001). Functional and expression pattern analysis of chemosensory proteins expressed in antennae and pheromonal gland of mamestra brassicae. Chem. Senses 26, 833–844. doi: 10.1093/chemse/26.7.833
Jiang, N. J., Tang, R., Guo, H., Ning, C., Li, J. C., Wu, H., et al. (2020). Olfactory coding of intra- and interspecific pheromonal messages by the male mythimna separata in north China. Insect Biochem. Mol. Biol. 125:103439. doi: 10.1016/j.ibmb.2020.103439
Jiang, N. J., Tang, R., Wu, H., Xu, M., Ning, C., Huang, L. Q., et al. (2019). Dissecting sex pheromone communication of mythimna separata (walker) in north China from receptor molecules and antennal lobes to behavior. Insect Biochem. Mol. Biol. 111:103176. doi: 10.1016/j.ibmb.2019.103176
Jiang, X., Luo, L., Zhang, L., Sappington, T. W., and Hu, Y. (2011). Regulation of migration in mythimna separata (walker) in China: a review integrating environmental, physiological, hormonal, genetic, and molecular factors. Environ. Entomol. 40, 516–533. doi: 10.1603/EN10199
Jiang, X., Zhang, L., Cheng, Y., and Luo, L. (2014). Current status and trends in research on the oriental armyworm, Mythimna separata (walker) in China. Chin. J. Appl. Entomol. 51, 881–889.
Li, S., Picimbon, J. F., Ji, S. D., Kan, Y. C., Qiao, C. L., Zhou, J. J., et al. (2008). Multiple functions of an odorant-binding protein in the mosquito Aedes aegypti. Biochem. Bioph. Res. 372, 464–468. doi: 10.1016/j.bbrc.2008.05.064
Li, Z., Zhang, Y., An, X., Wang, Q., Khashaveh, A., Gu, S., et al. (2020). Identication of leg chemosensory genes and sensilla in the Apolygus lucorum. Front. Physiol. 11:276.
Liu, G., Xuan, N., Rajashekar, B., Arnaud, P., Offmann, B., and Picimbon, J.-F. (2020). Comprehensive history of CSP genes: evolution, phylogenetic distribution and functions. Genes 11:413. doi: 10.3390/genes11040413
Liu, N. Y., Zhang, T., Ye, Z. F., Li, F., and Dong, S. L. (2015). Identification and characterization of candidate chemosensory gene families from spodoptera exigua developmental transcriptomes. Int. J. Biol. Sci. 11, 1036–1048. doi: 10.7150/ijbs.12020
Liu, Y., Gu, S., Zhang, Y., Guo, Y., and Wang, G. (2012). Candidate olfaction genes identified within the Helicoverpa armigera antennal transcriptome. PLoS One 7:e48260. doi: 10.1371/journal.pone.0048260
Liu, Y., Qi, M., Chi, Y., and Wuriyanghan, H. (2016). De novo assembly of the transcriptome for oriental armyworm Mythimna separata (lepidoptera: noctuidae) and analysis on insecticide resistance-related genes. J. Insect Sci. 16:92. doi: 10.1093/jisesa/iew079
Liu, Z., Wang, X., Lei, C., and Zhu, F. (2017). Sensory genes identification with head transcriptome of the migratory armyworm, Mythimna separata. Sci. Rep. 7:46033. doi: 10.1038/srep46033
Livak, K. J., and Schmittgen, T. D. (2001). Analysis of relative gene expression data using real-time quantitative PCR and the 2-ΔΔCT method. Methods 25, 402–408. doi: 10.1006/meth.2001.1262
Maleszka, J., Forêt, S., Saint, R., and Maleszka, R. (2007). RNAi-induced phenotypes suggest a novel role for a chemosensory protein CSP5 in the development of embryonic integument in the honeybee Apis mellifera. Dev. Genes Evol. 217, 189–196. doi: 10.1007/s00427-006-0127-y
Mang, D., Shu, M., Endo, H., Yoshizawa, Y., Nagata, S., Kikuta, S., et al. (2016a). Expression of a sugar clade gustatory receptor, BmGr6, in the oral sensory organs, midgut, and central nervous system of larvae of the silkworm bombyx mori. Insect Biochem. Mol. Biol. 70, 85–98. doi: 10.1016/j.ibmb.2015.12.008
Mang, D., Shu, M., Tanaka, S., Nagata, S., Takada, T., Endo, H., et al. (2016b). Expression of the fructose receptor BmGr9 and its involvement in the promotion of feeding, suggested by its co-expression with neuropeptide F1 in Bombyx mori. Insect Biochem. Mol. Biol. 75, 58–69. doi: 10.1016/j.ibmb.2016.06.001
Marinotti, O., Ngo, T., Kojin, B. B., Chou, S. P., Nguyen, B., Juhn, J., et al. (2014). Integrated proteomic and transcriptomic analysis of the Aedes aegypti eggshell. BMC Dev. Biol. 14:15. doi: 10.1186/1471-213X-14-15
Mitsuno, H., Sakurai, T., Murai, M., Yasuda, T., Kugimiya, S., Ozawa, R., et al. (2008). Identification of receptors of main sex-pheromone components of three Lepidopteran species. Eur. J. Neurosci. 28, 893–902. doi: 10.1111/j.1460-9568.2008.06429.x
Miyamoto, T., and Amrein, H. (2014). Diverse roles for the drosophila fructose sensor Gr43a. Fly 8, 19–25. doi: 10.4161/fly.27241
Miyamoto, T., Slone, J., Song, X., and Amrein, H. (2012). A fructose receptor functions as a nutrient sensor in the drosophila brain. Cell 151, 1113–1125. doi: 10.1016/j.cell.2012.10.024
Pelosi, P., Iovinella, I., Zhu, J., Wang, G., and Dani, F. R. (2017). Beyond chemoreception: diverse tasks of soluble olfactory proteins in insects. Biol. Rev. 93, 184–200. doi: 10.1111/brv.12339
Pertea, G., Huang, X., Liang, F., Antonescu, V., Sultana, R., Karamycheva, S., et al. (2003). TIGR gene indices clustering tools (TGICL): a software system for fast clustering of large EST datasets. Bioinformatics 19, 651–652. doi: 10.1093/bioinformatics/btg034
Picimbon, J. F., Dietrich, K., Krieger, J., and Breer, H. (2001). Identity and expression pattern of chemosensory proteins in Heliothis virescens (lepidoptera, noctuidae). Insect Biochem. Mol. Biol. 31, 1173–1181. doi: 10.1016/s0965-1748(01)00063-7
Pitts, R. J., Liu, C., Zhou, X., Malpartida, J. C., and Zwiebel, L. J. (2014). Odorant receptor mediated sperm activation in disease vector mosquitoes. Proc. Natl. Acad. Sci. U.S.A. 111, 2566–2571. doi: 10.1073/pnas.1322923111
Sun, L., Li, Y., Zhang, Z., Guo, H., Xiao, Q., Wang, Q., et al. (2019). Expression patterns and ligand binding characterization of plus-C odorant-binding protein 14 from Adelphocoris lineolatus (goeze). Comput. Biochem. Physiol. 227, 75–82. doi: 10.1016/j.cbpb.2018.10.001
Sun, Y. L., Huang, L. Q., Pelosi, P., and Wang, C. Z. (2012). Expression in antennae and reproductive organs suggests a dual role of an odorant-binding protein in two sibling Helicoverpa species. PLoS One 7:e30040. doi: 10.1371/journal.pone.0030040
Takemori, N., and Yamamoto, M. T. (2009). Proteome mapping of the drosophila melanogaster male reproductive system. Proteomics 9, 2484–2493. doi: 10.1002/pmic.200800795
Tamura, K., Peterson, D., Peterson, N., Stecher, G., Nei, M., and Kumar, S. (2011). MEGA5: molecular evolutionary genetics analysis using maximum likelihood, evolutionary distance, and maximum parsimony methods. Mol. Biol. Evol. 28, 2731–2739. doi: 10.1093/molbev/msr121
Tang, Q. Y., and Zhang, C. X. (2013). Data processing system (DPS) software with experimental design, statistical analysis and data mining developed for use in entomological research. Insect Sci. 20, 254–260. doi: 10.1111/j.1744-7917.2012.01519.x
Tang, R., Jiang, N. J., Ning, C., Li, G. C., Huang, L. Q., and Wang, C. Z. (2020). The olfactory reception of acetic acid and ionotropic receptors in the Oriental armyworm, Mythimna separata walker. Insect Biochem. Mol. Biol. 118:103312. doi: 10.1016/j.ibmb.2019.103312
Thorne, N., and Amrein, H. (2010). Atypical expression of drosophila gustatory receptor genes in sensory and central neurons. J. Comput. Neurol. 506, 548–568. doi: 10.1002/cne.21547
Tian, W., Zhang, T., Gu, S., Guo, Y., Gao, X., and Zhang, Y. (2021). OBP14 (odorant-binding-protein) sensing in Adelphocoris lineolatus based on peptide nucleic acid and grapheme oxide. Insects 12:422. doi: 10.3390/insects12050422
Vogel, H., Heidel, A. J., Heckel, D. G., and Groot, A. T. (2010). Transcriptome analysis of the sex pheromone gland of the noctuid moth Heliothis virescens. BMC Genom. 11:29. doi: 10.1186/1471-2164-11-29
Walker, W. B., Roy, A., Anderson, P., Schlyter, F., and Larsson, M. C. (2019). Transcriptome analysis of gene families involved in chemosensory function in Spodoptera littoralis (lepidoptera: noctuidae). BMC Genom. 20:428. doi: 10.1186/s12864-019-5815-x
Wang, C., Wang, B., and Wang, G. (2021). Functional characterization of sex pheromone neurons and receptors in the armyworm, Mythimna separata (walker). Front. Neuroanat. 15:673420. doi: 10.3389/fnana.2021.673420
Wang, L., Zhu, J. Y., Qian, C., Fang, Q., and Ye, G. Y. (2015). Venom of the parasitoid wasp pteromalus puparum contains an odorant binding protein. Arch. Insect Biochem. 88, 101–110. doi: 10.1002/arch.21206
Wang, M., Li, H., Zheng, H., Zhao, L., Xue, X., and Wu, L. (2020). De novo transcriptomic resources in the brain of vespa velutina for invasion control. Insects 11:101. doi: 10.3390/insects11020101
Xiao, Y., Sun, L., Ma, X. Y., Dong, K., Liu, H. W., Wang, Q., et al. (2017). Identification and characterization of the distinct expression profiles of candidate chemosensory membrane proteins in the antennal transcriptome of Adelphocoris lineolatus (goeze). Insect Mol. Biol. 26, 74–91. doi: 10.1111/imb.12272
Younas, A., Waris, M. I., Chang, X. Q., Shaaban, M., Abdelnabby, H., Tahir ul Qamar, M., et al. (2018a). A chemosensory protein MsepCSP5 involved in chemoreception of oriental armyworm Mythimna separata. Int. J. Biol. Sci. 14, 1935–1949. doi: 10.7150/ijbs.27315
Younas, A., Waris, M. I., Tahir ul Qamar, M., Shaaban, M., Prager, S. M., and Wang, M. Q. (2018b). Functional analysis of the chemosensory protein MsepCSP8 from the oriental armyworm Mythimna separata. Front. Physiol. 9:872. doi: 10.3389/fphys.2018.00872
Younas, A., Waris, M. I., Shaaban, M., Tahir ul Qamar, M., and Wang, M. Q. (2021). Appraisal of Msep CSP14 for chemosensory functions in Mythimna separata. Insect Sci. 1–15. doi: 10.1111/1744-7917.12909
Zhang, J., Wang, B., Dong, S., Cao, D., Dong, J., Walker, W. B., et al. (2015). Antennal transcriptome analysis and comparison of chemosensory gene families in two closely related noctuidae moths, Helicoverpa armigera and H. assulta. PLoS One 10:e0117054. doi: 10.1371/journal.pone.0117054
Zhang, K., Feng, Y., Du, L., Gao, S., Yan, H., Li, K., et al. (2019). Functional analysis of MsepOR13 in the oriental armyworm Mythimna separata (walker). Front. Physiol. 10:367. doi: 10.3389/fphys.2019.00367
Zhang, Y. N., Zhu, X. Y., Fang, L. P., He, P., Wang, Z. Q., Chen, G., et al. (2015). Identification and expression profiles of sex pheromone biosynthesis and transport related genes in Spodoptera litura. PLoS One 10:e0140019. doi: 10.1371/journal.pone.0140019
Keywords: Mythimna separata, brain transcriptome, chemosensory genes, chemosensory protein, non-sensory organ, odorant binding protein
Citation: Chen W-B, Du L-X, Gao X-Y, Sun L-L, Chen L-L, Xie G-Y, An S-H and Zhao X-C (2022) Identification of Odorant-Binding and Chemosensory Protein Genes in Mythimna separata Adult Brains Using Transcriptome Analyses. Front. Physiol. 13:839559. doi: 10.3389/fphys.2022.839559
Received: 20 December 2021; Accepted: 24 January 2022;
Published: 28 February 2022.
Edited by:
Ya-Nan Zhang, Huaibei Normal University, ChinaReviewed by:
Dingze Mang, Tokyo University of Agriculture and Technology, JapanPeng-Fei Lu, Beijing Forestry University, China
Copyright © 2022 Chen, Du, Gao, Sun, Chen, Xie, An and Zhao. This is an open-access article distributed under the terms of the Creative Commons Attribution License (CC BY). The use, distribution or reproduction in other forums is permitted, provided the original author(s) and the copyright owner(s) are credited and that the original publication in this journal is cited, in accordance with accepted academic practice. No use, distribution or reproduction is permitted which does not comply with these terms.
*Correspondence: Xin-Cheng Zhao, eGluY2hlbmdAaGVuYXUuZWR1LmNu
†These authors have contributed equally to this work