- Department of Molecular Biology, Cell Biology and Biochemistry, Brown University, Providence, RI, United States
Diapause is a physiological adaptation to conditions that are unfavorable for growth or reproduction. During diapause, animals become long-lived, stress-resistant, developmentally static, and non-reproductive, in the case of diapausing adults. Diapause has been observed at all developmental stages in both vertebrates and invertebrates. In adults, diapause traits weaken into adaptations such as hibernation, estivation, dormancy, or torpor, which represent evolutionarily diverse versions of the traditional diapause traits. These traits are regulated through modifications of the endocrine program guiding development. In insects, this typically includes changes in molting hormones, as well as metabolic signals that limit growth while skewing the organism’s energetic demands toward conservation. While much work has been done to characterize these modifications, the interactions between hormones and their downstream consequences are incompletely understood. The current state of diapause endocrinology is reviewed here to highlight the relevance of diapause beyond its use as a model to study seasonality and development. Specifically, insect diapause is an emerging model to study mechanisms that determine lifespan. The induction of diapause represents a dramatic change in the normal progression of age. Hormones such as juvenile hormone, 20-hydroxyecdysone, and prothoracicotropic hormone are well-known to modulate this plasticity. The induction of diapause—and by extension, the cessation of normal aging—is coordinated by interactions between these pathways. However, research directly connecting diapause endocrinology to the biology of aging is lacking. This review explores connections between diapause and aging through the perspective of endocrine signaling. The current state of research in both fields suggests appreciable overlap that will greatly contribute to our understanding of diapause and lifespan determination.
Introduction
Diapause is a physiological adaptation to adverse conditions meant to increase the odds of an organism’s survival into reproductive age (Karp, 2021). Traits of diapause include extended lifespan, slowed development, altered metabolism and body composition, delayed reproduction, and increased hardiness against environmental threats. Some insects exhibit a programmed period of diapause during development (obligatory diapause), while others use environmental cues such as nutrient availability, temperature, humidity and photoperiod, to determine whether it is appropriate to delay development until favorable conditions appear, or whether the current environment will support the energy-intensive process of normal development. The latter mode is named “facultative diapause.” In cases where diapause phenotypes are milder, fewer, easily reversed, or induced quickly as part of a generalized stress response, other terms such as “quiescence,” “dormancy” or “torpor” are sometimes used (Zonato et al., 2017; Karp, 2021). While functionally distinct, variations in diapause or diapause-like physiology are worthwhile to study in the context of longevity for their evolutionary and mechanistic similarities.
The transmission of information about the environment occurs through a complex network of competing endocrine signals. Though the hormones involved are largely homologous across species, the precise endocrine program of diapause has remained elusive due to its branching nature; no individual hormone is regulated apart from any other, and there is extensive overlap in the downstream molecular targets of each (Figure 1). It is therefore difficult to attribute any given effect of diapause to one hormone by itself. However, it is equally difficult to consider the complete breadth of diapause endocrinology in any one study. This review seeks to assist by drawing connections between results from recent literature. Adding to the intricacy of this task is the fact that diapause consists of several phases. Preparation, initiation, maintenance, and termination are mediated by separate endocrine states. Recent advances in diapause research show that each phase consists of transcriptionally distinct sub-phases (Kubrak et al., 2014; Li et al., 2015), as well. Much work in the field of insect diapause has focused on mechanisms of diapause initiation. Specifically, much attention has been granted to mechanisms responsible for the induction of early diapauses between larval or pupal transitions. Reproductive dormancy, characterized by developmental regression of the gonads, has also been well-studied as a proxy for the complete diapause phenotype in adults.
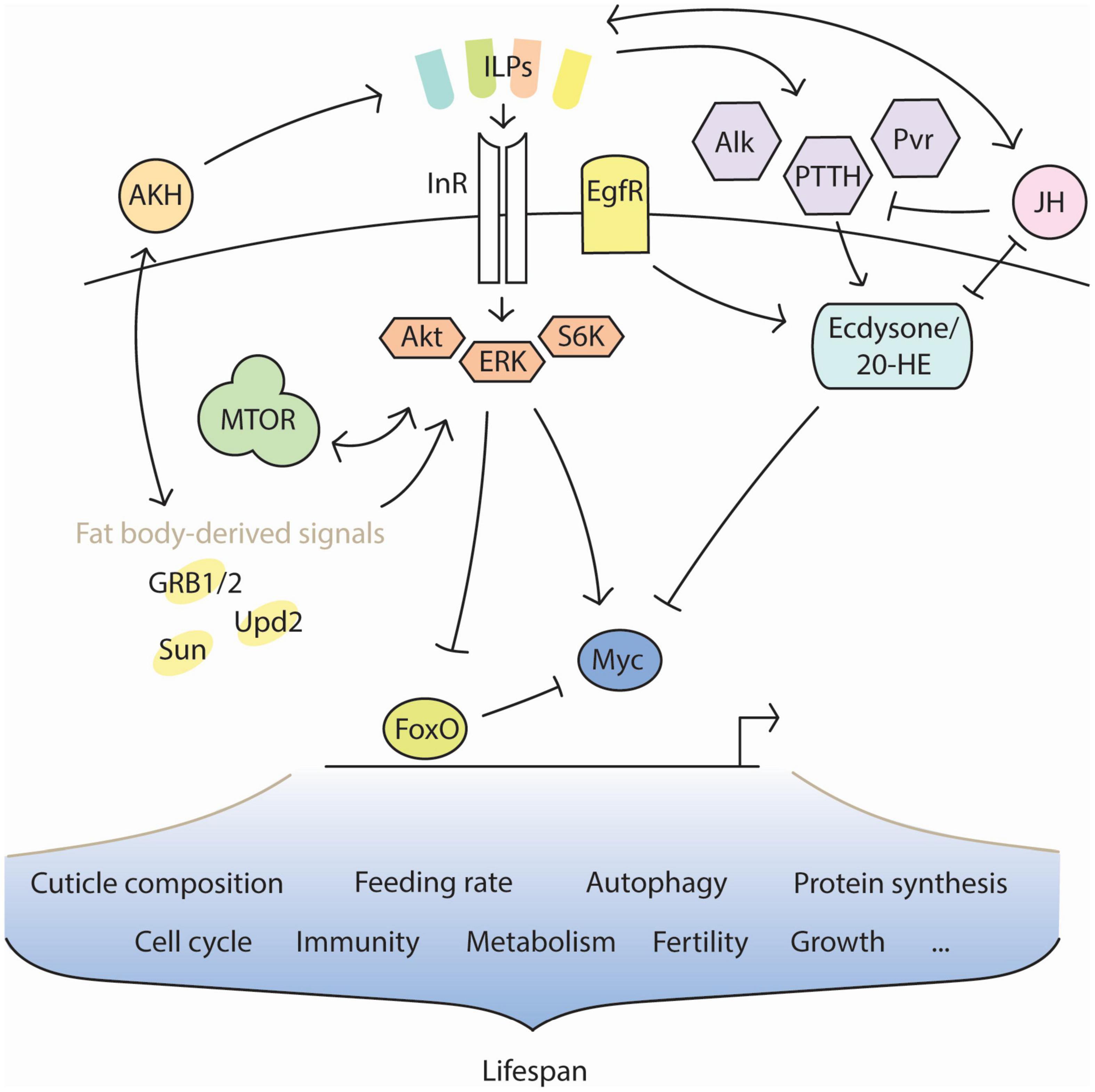
Figure 1. Interaction of key factors in diapause. Insulin signaling profiles change in diapause, caused by the activity of different insulin-like peptides (ILPs). The ILP profile must remain dynamic in diapause to support the diapause-associated metabolism over time and changing conditions. Other factors, such as the nutrient-sensitive adipokinetic hormone (AKH) and the pro-reproductive juvenile hormone (JH), influence insulin signaling and are themselves influenced in turn. JH opposes the metamorphic action of ecdysone and its upstream regulators. FoxO is typically inhibited by insulin signaling, but is disinhibited during diapause. Conversely, the pro-growth transcription factor Myc is typically promoted by insulin signaling, but becomes inhibited during diapause. Many features of diapause physiology are regulated through interactions between insulin signaling, JH, ecdysone, and nutritive feedback signals from the fat body. The extended lifespan of insects in diapause is likely a product of these features’ combination.
Studies in diapause often involve perturbation of known elements of diapause physiology—pathways such as insulin/insulin growth factor signaling (IIS) or ecdysone biosynthesis—or modification of tissues responsible for these hormones’ secretion. Laboratory disruptions in the precisely timed endocrine program coordinating normal development can result in partial or complete induction of diapause. The degree to which partial diapause phenotypes are induced without exposure to diapause-inducing environmental stimuli, or the degree to which these phenotypes are ameliorated upon environmental exposure, is usually taken to indicate something about the importance of the hormone in question. Nearly all cases extend lifespan. Lifespan extension is robustly conserved among insect diapauses across developmental phases, as it enables the organism to persist through unfavorable seasons that typically last much longer than the organism’s normal lifespan. This trait is slowly emerging as a promising model system to study mechanisms of lifespan plasticity outside the immediate context of insect diapause. Diapause represents a fascinating adaptation wherein the median lifespan of a species dramatically changes in a reversible manner. The conservation of this trait across taxa, experimental mode of diapause induction, and developmental stage suggests that lifespan is not a static trait programmed per species, but is determined by integrating external information with processes that are fundamental to most organisms. Understanding what controls the diapause-associated change in lifespan will help answer the question of why we age at the rate that we do.
Many findings in the aging biology field underpin longevity with changes in pathways that mimic the way those pathways are naturally modified during diapause. For example, it has long been observed that manipulations of IIS extend lifespan across species (Sim and Denlinger, 2013). IIS-dependent lifespan extension is usually accompanied by other diapause-like phenotypes, such as resistance to infection and loss in reproductive capacity. Although increasing evidence finds ways to uncouple these phenotypes, it is unlikely that they are co-induced by coincidence. Rather, it may be the case that activation of one facet of diapause signals a systemic “diapause response” to perceived stress, which in turn activates supporting pathways. Other modes of lifespan extension (manipulations in neuronal signaling, amino acid metabolism, dietary restriction, and more) also invoke diapause physiology (Suo et al., 2009; Katewa et al., 2012; Itskov and Ribeiro, 2013; Kapahi et al., 2017; Ling and Raikhel, 2018; Parkhitko et al., 2020). These results from aging biology can be simultaneously united and explained by considering them in the context of diapause. Doing so will benefit both fields by expanding the relevance of conclusions from either. Therefore, this review is written from the perspective that diapause represents the evolutionary context for laboratory manipulations of lifespan.
Endocrine in Diapause
As a mechanism that evolved to modify development, the onset of diapause demands changes to the endocrine program that defines normal progression through molts to reach reproductive adulthood. Historically, the regulation of endocrine has been characterized in the context of development. Less is known about how the entrance or maintenance of diapause imposes upon developmental hormones, but especially in the case of adult diapause, this topic is beneficial for understanding the role of early development in lifespan plasticity. Examination of the work done in development is informative for speculating about how endocrine functions and changes course in diapause and longevity.
Ecdysone
The steroid hormone ecdysone is well-known to catalyze metamorphosis between developmental stages and antagonize growth. In holometabolous insects, growth and metamorphosis are distinct processes. Growth is defined by the accumulation of mass, cell division, and increase in body size, whereas metamorphosis refers specifically to the differentiation of prime tissues into developmentally terminal ones. The large upregulation of ecdysone at the end of each molt signals the organism’s pausing of growth and the initiation of metamorphosis into the next stage. This period is called “critical weight,” which means the molt has accumulated enough mass to signal entrance into the next stage of development. In pre-adult molts such as larvae or nymphs, ecdysone is synthesized and secreted from the prothoracic gland (PG). The PG is part of the larger complex of endocrine signaling glands adjacent to the brain called the ring gland. Ecdysone is released into the hemolymph, which distributes the hormone to peripheral tissues where it is imported via the ecdysone importer (EcI) (Okamoto et al., 2018). Once imported, ecdysone can differentially regulate transcription depending on whether it is converted to 20-hydroxyecdysone (20HE); both forms have been shown to have active effects, though fewer studies have investigated the biological relevance of ecdysone signaling before its conversion (Beckstead et al., 2007; Tanaka, 2013; Yamanaka et al., 2013; Ono, 2014). Conversion to 20HE occurs through the activity of Shade, one enzyme derived from the ‘Halloween’ group of genes responsible for the complete ecdysone biosynthetic pathway (Petryk et al., 2003). Briefly, precursor ecdysone is derived from nutritionally acquired sterols and is modified through a series of early oxidation steps terminating in a chain of cytochrome P450 monooxygenases. For a thorough examination of the ecdysteroid biosynthetic process, see Niwa and Niwa (2014).
The binding of 20HE to the nuclear ecdysone receptor (EcR) induces the receptor’s entrance into the nucleus and subsequent heterodimerization to Ultraspiracle (Usp) (Yao et al., 1992, 1993). The complex then binds DNA to mediate the transcription of ecdysone response genes that catalyze metamorphosis, such as the Broad-Complex, E93, and EcR itself (Karim et al., 1993; Lee et al., 2000; Ghbeish et al., 2001). It has been shown that the set of genes regulated by nuclear EcR differs according to developmental stage, though it remains unclear how the organism measures developmental progress to modify the transcriptional targets of the same receptor complex across stages (Yamanaka et al., 2013; Uyehara and McKay, 2019). Apart from binding to ecdysone response elements, EcR also affects transcription through changes in chromatin structure (Ashburner, 1973). Chromosome puffing—sites of decompressed chromatin that facilitate transcription—has been observed in a variety of insects in the presence of ecdysone (Stocker and Pavan, 1977; Ashburner, 1990; Russell and Ashburner, 1996; Gariou-Papalexiou et al., 2001). As the genes regulated by EcR depend on developmental timing, so do the genomic loci of puffs. This decompaction is traceable at least in part to the EcR cofactor SMRTER, whose activation leads to repression of the histone deacetylase Rpd3 (Tsai et al., 1999). Other transcription factors, such as the EcR-Usp complex itself, may induce similar structural changes through the recruitment of RNA polymerase-associated chromatin binding proteins.
Our understanding of the effectors of ecdysone signaling are still incomplete, however. Recent work has suggested involvement of Thor, a 4E-binding translation initiation factor. Thor is an effector of insulin/insulin-like signaling (IIS) and the mechanistic Target of Rapamycin (mTOR) pathway that each regulate the intersection of lifespan and nutrition (Kapahi et al., 2004; Herboso et al., 2015). Wing disks from third instar, ecdysone-deficient larvae have increased expression of Thor, suggesting that ecdysone plays a repressive role against transcription of the Thor gene product. Indeed, while insulin-like peptide (ILP) secretion is unaffected in these larvae, many tissues show impaired growth. This result complicates the long-held idea that the growth-inhibitory effects of ecdysone are inversely correlated with pro-growth IIS and mTOR signaling (Rusten et al., 2004; Colombani et al., 2005).
The picture becomes cloudier yet from the perspective of age and adulthood. As the PG no longer exists in adult insects, ecdysone synthesis originates from other tissues. In Drosophila, evidence suggests adult ecdysone synthesis takes place in the gut, muscle, Malpighian tubules, and male accessory glands, at least (Hentze et al., 2013; Zheng et al., 2018). This variety of locations may indicate an adult capacity to respond to environmental stress in a tissue-specific manner. Increasing 20HE titers no longer initiates metamorphosis in adults; instead, 20HE responds to stresses such as nutritional deficiency, water loss, or heat (Nghiem et al., 2000; Rauschenbach et al., 2000; Gibbs and Markow, 2001; Terashima et al., 2005; Zheng et al., 2018). These effects are both more common and more damaging in old age, and also represent stressors that the physiological adaptations of diapause have evolved to protect against. Accordingly, it has been shown that ecdysone increases with age and induces expression of peptidoglycan recognition protein-LC (PRP-LC), a critical component of immune signaling needed to respond against infections that are expected under suboptimal environmental conditions (Rus et al., 2013; Zheng et al., 2018). Increasing ecdysone in older adults also causes fibrosis in a manner dependent on epidermal growth factor receptor (EgfR) and dopamine-EcR, a receptor that binds ecdysone before its conversion to 20HE. Results from this work suggest ecdysone continues its metamorphic role in adults, but has lost the tight regulation required for development (Zheng et al., 2020). It follows that heterozygous mutation for EcR extends adult lifespan (Simon et al., 2003). Presumably, these flies evade some of the fibrosis expected with age and do not aberrantly induce age-associated inflammation through PRP-LC (Garschall and Flatt, 2018). This occurs in a manner independent of reproductive capacity.
Extrapolating from its role in development, perhaps ecdysone responds to stress in adults by inducing more subtle changes to the organism that benefit survival and longevity. One such modification may be alteration of the cuticle composition. The cuticle serves several functions to protect against stress: just after eclosion, cuticle hardening helps resist mechanical forces such as punctures or tears, which could lead to infection (Noh et al., 2016). The cuticle also undergoes a tyrosine-dependent tanning process through the deposition of various pigments such as melanins or tetrapyrroles (Shamim et al., 2014; Solano, 2014; Noh et al., 2016). Pigmentation is an important step in the final stage of development to resist damage from UV radiation, to which the insect is especially vulnerable during winter or drought when shade is sparse from failing vegetation. Increased sunlight exposure also causes water loss, leading to dehydration and inflammation, which are exacerbated with age (Franceschi et al., 2018; Zheng et al., 2018). The function of water retention is controlled by lipid barriers deposited throughout the cuticle (Lockey, 1988). As the organism ages, these barriers deteriorate and change composition (Gibbs et al., 1991; Nghiem et al., 2000; Kuo et al., 2012). Therefore, the age-associated increase in 20HE titers may act as a compensatory mechanism intended to remodel the cuticle to defend against the damaging effects of water loss caused by deterioration of cuticle lipid barriers.
The expression patterns of ecdysone throughout the different stages of diapause are largely unknown across species. However, there is evidence from flesh flies and moths to suggest entrance into diapause involves an initial increase in ecdysone, perhaps as a stress response similar to that seen in aging, where ecdysone is elevated in anticipation of the drought conditions associated with diapause season(s) (Denlinger et al., 1997; Rinehart et al., 2001). An initial burst of ecdysone may serve to drive the generation of alternative, diapause-specific structures in young, diapause-destined adults, such as the further tanning of the cuticle and restructuring of its lipid composition that have already been observed (Denlinger, 2002; Li and Denlinger, 2009). Other insect species, however, initiate diapause with a drop in ecdysone titers (Denlinger et al., 2012; Guo et al., 2021). While these observations do not preclude the suggestion that ecdysone induces diapause-specific structures in later developmental stages, it is worth noting that the functions of ecdysone—and endocrine more broadly—are poorly conserved.
In conclusion, it may be the case that ecdysone has significant effects on lifespan depending on its time of expression: during metamorphosis and diapause, elevated titers of ecdysone affect the insulin signaling profile to downregulate pro-aging factors like Myc and activate anti-aging factors like the Forkhead box-class O transcription factor (FoxO). Additionally, ecdysone could function in diapause to catalyze the metamorphosis of structures that elongate lifespan through resistance against stresses that exacerbate aging. However, there is currently no direct evidence as to whether these structures in diapause contribute to longevity, or whether their generation depends on ecdysone. It is possible that their effects are limited to survival; without them, the organism might be incapable of withstanding the harsh conditions expected during diapause.
Insulin
Diapause-associated modifications to IIS cause physiological changes that often mirror those reported in studies examining the relation between IIS and aging (Garsin et al., 2003; Kramer et al., 2003; Broughton et al., 2005; Sim and Denlinger, 2008; Kubrak et al., 2014; Altintas et al., 2016; Cambron et al., 2021; Karp, 2021). Indeed, these changes are also derived from similar molecular events, such as the tissue-specific activation of the growth-inhibitory factor FoxO, upregulation of Toll-dependent innate immunity, and increased expression of adipokinetic hormone (AKH), the functional homolog to mammalian glucagon (Kubrak et al., 2014; Suzawa et al., 2019). Correspondingly, insofar as ecdysone signaling mediates IIS as well, many of these characteristics are also observed in the context of metamorphosis (Davidson and Swalla, 2002; Hughson et al., 2021). In both metamorphosis and diapause, modification of IIS correlates with a reduction or cessation of feeding, concurrent with the antagonism of growth and aging. These similarities warrant exploration of the idea that laboratory manipulations of IIS do not extend lifespan without evolutionary precedent, but recapitulate elements of the metabolic and reproductive programs of diapause and metamorphosis, which present as slow aging, low fecundity, and stress resistance.
Insulin and Ecdysone
The deceleration of growth coupled to metamorphosis is achieved through ecdysone-dependent inhibition of the mitogenic transcription factor Myc (Delanoue et al., 2010; Rajan and Perrimon, 2010). Myc is repressed by FoxO, which is in turn inhibited by IIS. Little is known about the exact mechanism by which ecdysone inhibits IIS to activate FoxO. In Helicoverpa armigera, recent work provides evidence that 20HE acts upstream of IIS to maintain InR in a dephosphorylated state (Li et al., 2021). Because ecdysone has strong effects in the fat body, it is also possible that ecdysone downregulates IIS during metamorphosis by inhibiting one or more of the fat body-derived growth signals. These signals will be described later in this review for their interaction with IIS under variable nutrient conditions that contribute to diapause (Colombani et al., 2005). In sum, more research is needed to understand this pathway in its entirety, but it is clear that ecdysone is one among many regulators of IIS (Ahmad et al., 2020).
In turn, IIS controls the production of ecdysone. Increasing or decreasing IIS during development alters the time to critical weight and the consequent timing of expression of ecdysone synthesis genes (Rulifson et al., 2002; Shingleton et al., 2005; Walkiewicz and Stern, 2009). Perturbations in nutritional sensing via mTOR or IIS limits the growth of the PG, causing increased larval body size (an extension of the growth period), and delayed ecdysis (Gu et al., 2009; Kemirembe et al., 2012). Downstream of IIS, extracellular signal-regulated kinase (ERK) can also induce activity of the transcription factor Pointed, which modifies the EGF receptor signaling pathway (O’Neill et al., 1994; Traverse et al., 1994; Morimoto et al., 1996). Among other functions, EGFR signaling regulates the production of ecdysone in the PG (Cruz et al., 2020; Pan and O’Connor, 2021).
Insulin and Nutrition
During growth phases, insulin peptide synthesis and secretion are regulated in response to nutritional cues acquired through dietary amino acids, sugars and carbohydrates (Kim and Neufeld, 2015). Insulin producing cells (IPCs) of insects are most prominently studied in the brain and fat body, but IPCs exist in many other tissues throughout the organism, such as the digestive and reproductive systems (Nässel et al., 2013). IIS regulates lifespan and tissue growth by sensing the availability of metabolites both directly and indirectly (Colombani et al., 2003; Fridell et al., 2009; Kréneisz et al., 2010; Rajan and Perrimon, 2012; Park et al., 2014). In the brain, exogenous glucose is sufficient to stimulate fluctuation in intracellular IPC Ca2+, causing secretion of insulin peptides (Kréneisz et al., 2010). Similarly, amino acids can pass through the gut lumen to signal directly to tissues via hemolymph circulation (Holtof et al., 2019). Indirectly, the import of leucine via the IPC-bound transmembrane proteins Juvenile Hormone-Inducible-21 (JhI-21) or Minidiscs (Mnd) is also sufficient to cause insulin secretion (Manière et al., 2016; Ziegler et al., 2018). In the gut and fat body, amino acids are imported via Mnd and Slimfast (Slif) to initiate multiple modes of insulin regulation that are largely dependent on secreted factors. The amino acid sensing folliculin/folliculin-interacting protein complex activates mTOR in the fat body through a series of RAG GTPases (Tsun et al., 2013; Shimobayashi and Hall, 2016). Once translocated to the lysosome, active mTOR modifies IIS by acting on downstream components like S6 kinase (S6K), Akt and FoxO (Zhang et al., 2011; Sangüesa et al., 2019). Evidence also exists for mTOR regulation of IPCs upstream of the insulin signaling cascade, though these studies are mainly focused in mammalian models (Gu et al., 2011; Alejandro et al., 2017; Sangüesa et al., 2019). Another mode of indirect insulin regulation in the fat body comes from the ligand Stunted (Sun). The receptor for Sun, Methuselah (Mth) (Delanoue et al., 2016), is expressed in the IPCs. Amino acids stimulate the release of Sun from the fat body and allow it to circulate to the brain via hemolymph, where Sun binds Mth to induce the release of IPC-derived ILPs; it follows that mutation of Mth extends lifespan via downregulation of IIS (Lin et al., 1998). Fat body-derived growth blocking peptides (GRB1, GRB2) are also capable of regulating IIS. Secreted GRB1 binds an epidermal growth factor (EGF) receptor on inhibitory neurons innervating the IPCs (Koyama and Mirth, 2016; Meschi et al., 2019). Although originally defined as growth inhibitors, GBPs encourage growth by repressing the activity of inhibitory neurons, thereby promoting the secretion of ILPs from the innervated IPCs (Hayakawa, 1991). Furthermore, the presence of sugars or carbohydrates in the fat body stimulates the release of CCHamide-2 (CCHa2) and Unpaired 2 (Upd2) that each modify IIS in the brain (Rajan and Perrimon, 2012; Li et al., 2013; Ren et al., 2015). There are numerous non-redundant mechanisms by which insulin is regulated before its secretion, and there are likely even more that extend beyond what we currently understand (Teleman, 2009; Post and Tatar, 2016; Ahmad et al., 2020; Manière et al., 2020; Chowański et al., 2021; Ling and Raikhel, 2021). Non-nutritional cues and endogenous insulin decoys can modify IIS after secretion to generate more specialized physiological and behavioral responses (Sloth Andersen et al., 2000; Siddle, 2012; Stefanatos et al., 2012; Okamoto et al., 2013; Barber et al., 2016). In species with multiple ILPs, each peptide signals in a distinct manner whose targets are not yet fully known (Post et al., 2018; Yamamoto et al., 2021). Downstream effects of IIS are additionally modified by tissue-specific levels of intracellular IIS targets, as well as competing signals from other pathways that intersect with IIS effectors (Siddle, 2012; Ruzzi et al., 2020). For these reasons, IIS is best construed as a highly flexible pathway. The phenotypes observed via disruptions in individual components likely represent real world scenarios in which the pathway is modified to produce a programmed response to some environmental cue.
After secretion into the hemolymph, ILPs bind the insulin receptor (InR) in peripheral tissues and cause the receptor to autophosphorylate (Zhang et al., 2011; Sim and Denlinger, 2013). Recruitment and phosphorylation of the insulin receptor substrates Lnk and Chico then initiates a phosphorylation cascade dependent on the kinases phosphatidylinositide 3-kinase (PI3K), Akt, and ERK (Yenush et al., 1996; Werz et al., 2009; Zhang et al., 2011; Almudi et al., 2013). Activation of Akt leads to the inhibition of FoxO, which then promotes cell proliferation through the disinhibition of Myc and the inhibition of Thor and several negative regulators of mTOR signaling (Koyama et al., 2013). Akt also promotes energy storage through the activation of glycogen synthase kinase-3 (GSK-3), which opposes the tissue-autonomous, energy mobilizing action of glycogen phosphorylase (GlyP) (Yamada et al., 2018). The downstream effects of ERK signaling are diverse and highly dependent on crosstalk with other pathways, encapsulating functions such as cell division, differentiation and apoptosis (Traverse et al., 1994; Shaul and Seger, 2007; Zhang et al., 2011).
Insulin Peptides
Most insects have multiple ILPs with varying degrees of structural and functional redundancy. Accordingly, ILPs are regulated in manners both concerted and individual, depending on stimulus. Much work has been done to establish the diapause-like phenotypes of IPC-ablated flies (Rulifson et al., 2002; Broughton et al., 2005; Haselton et al., 2010; Ojima et al., 2018). The functions of ILPs are best studied in Drosophila and will be summarized here for their roles in aging and diapause, keeping in mind that while individual ILPs may not be conserved across taxa, the regulatory framework is broadly applicable: environmental stimuli induce a subset of ILPs with specialized functions appropriate for the given conditions.
Insulin-Like Peptide 2
ILP2 is the most abundant and extensively characterized of the Drosophila ILPs and is most similar to mammalian insulin in that its sequence is most homologous and its activity is primarily (but not exclusively) glucose-dependent (Brogiolo et al., 2001; Buch et al., 2008; Géminard et al., 2009; Kim and Neufeld, 2015; Post and Tatar, 2016). ILP2 chiefly regulates the production of glycogen by maintaining the phosphorylation state of glycogen phosphorylase (GlyP) (Post et al., 2018). Buch et al. (2008) revealed that the lifespan extension of IPC-ablated flies is partially rescued through expression of ilp2 alone, supporting a central role of ILP2 in mediating IIS-dependent longevity. However, conflicting results challenge the assertion that ILP2 is sufficient to control lifespan. RNAi knockdown of ilp2 resulted in flies with lifespan similar to that of wildtype flies of the same genetic background, whereas in a separate study from the same group, null mutation of ilp2 resulted in modest extension with statistical significance (p < 0.001) (Broughton et al., 2008; Grönke et al., 2010). These conflicting results are possibly attributable to the many interacting pathways of ILP signaling that affect physiology in ways we do not yet understand. Changes in the level of a single ILP causes change in the levels of other ILPs, as well as changes in how the organism responds to temperature, diet, and even commensal bacteria (Broughton et al., 2005; Grönke et al., 2010; Bai et al., 2012; Chowański et al., 2021; Ling and Raikhel, 2021; Semaniuk et al., 2021). Correspondingly, though mRNA levels of ilp2 and ilp5 correlate with the inducibility of reproductive diapause, these findings were temperature-dependent, suggesting ILP levels alone do not control diapause physiology, but are likely an important facet of the larger diapause program (Schiesari et al., 2016).
Insulin-Like Peptide 6
The IGF-like ILP6 was first characterized in the fat body, but sites of production also include the gut, salivary glands and surface glia (Brogiolo et al., 2001; Okamoto et al., 2009; Chell and Brand, 2010; Okamoto and Nishimura, 2015). ILP6 is known to promote growth during periods of starvation, including both the natural non-feeding larval-pupal transition, as well as imposed starvation conditions outside this stage, such as diapause (Slaidina et al., 2009; Kubrak et al., 2014). Interestingly, expression of ilp6 alone is sufficient to induce phenotypes associated with diapause, such as decreased fecundity, increased concentration of energy storage molecules and extended lifespan. ilp6 is also positively correlated with ilp5, an ILP that responds to amino acids via mTOR in surface glia (Okamoto and Nishimura, 2015). Both are required for sustained growth under limited nutrient availability. The lifespan extension of ilp6 overexpression mutants may be attributable to the mutants’ downregulation of ilp2; loss of ilp2 has been shown to increase lifespan in a ilp1-dependent manner (Grönke et al., 2010; Post et al., 2019). However, ilp1 overexpression in an otherwise wildtype background does not extend lifespan, leaving the question open as to how ILPs like ILP1 and ILP6 function in a paradoxically anti-aging mechanism.
Insulin-Like Peptide 1
ilp1 is typically expressed during the pupal stage and remains repressed for the duration of adulthood (Slaidina et al., 2009). During the onset of diapause, however, ilp1 becomes highly expressed and remains so for several weeks (Liu et al., 2016). Little is known about the downstream mechanism of ILP1. Still, the convergence of its roles in diapause and lifespan extension support the idea that diapause represents the natural context for longevity achieved through manipulations of IIS. Indeed, in a study examining the relationship between taste perception and longevity, Ostojic et al. (2014) found that taste-impaired female flies exhibit longer lifespan and elevated transcripts for ilp1, ilp3, and ilp6 in a FoxO-dependent manner. Taste is a sensory modality similar to olfaction used to determine the availability of nutrition. With this understanding, these results could imply that ILP1 responds to starvation, as one environmental cue signaling the onset of winter, to induce the metabolic program of diapause that supports longevity.
The metabolism of diapause typically relies on an abundance of lipid storage and circulating trehalose, but less is known about the molecular changes that bring about this state. As a diapause-specific ILP, ILP1 is an attractive candidate to regulate the induction of the diapause metabolism upstream, and will be expanded upon here. In the absence of ilp2, ilp1 induces AKH (Post et al., 2019). AKH has been reported to extend lifespan (Waterson et al., 2014). However, ilp1 overexpression does not extend lifespan. This discrepancy suggests a missing mediator between AKH and its effects on longevity. The usual context of AKH signaling is during periods of starvation or water loss. Perhaps the pro-longevity effect of ilp1 does not result from its expression alone, nor its regulation of AKH, but may be mediated through conditions closer to those that signal diapause. Under this model, the pro-longevity metabolism of diapause may depend on unknown factors that mediate between AKH and the perception of nutrition. One molecule that could play this role is trehalose, as a nutritive sugar that is upregulated in response to the cold conditions expected in Drosophila diapause. Indeed, though AKH is traditionally considered a starvation-responsive hormone, Kim and Neufeld (2015) demonstrate that AKH responds to sugars, as well (Doğan et al., 2021). This suggestion is further supported by the fact that AKH stimulates trehalose production, generating a putative feedback loop in diapause to support both longevity and cryo-protection (Isabel et al., 2005). Other candidate mediators include fat body-derived signals, whose effects are incompletely understood. Particularly, Upd2 is known to both regulate AKH secretion and respond to high sugar levels (Post and Tatar, 2016; Zhao and Karpac, 2017). Upd2 is also a known regulator of ILP secretion in the IPCs, where ILP1 is produced (Rajan and Perrimon, 2012). However, in a time course study examining Drosophila diapause, Upd2 expression was found to trail after ilp1 expression (Kubrak et al., 2014). Upd2 is also known to oppose FoxO and promote of the release of ILP2, discrediting the idea that Upd2 supports diapause longevity during the induction phase (Rajan and Perrimon, 2012; Zhao and Karpac, 2017; Lourido et al., 2021). It is possible that another starvation associated, fat body-derived signal supplies the missing mediator for AKH, but more research is needed to address this problem directly.
Insulin-Like Peptide 3
Mobilization of glycogen is a key process during diapause and starvation. Glycogen is primarily stored in the muscle, rather than the fat body, presumably to facilitate energy transport to tissues responsible for flight and evasion (Veenstra et al., 2008). Glycogen is broken down to glucose and trehalose in response to perceived starvation through AKH, but can also occur in the fat body via ilp3 in an AKH-independent manner (Yamada et al., 2018). Perception of dietary sugars stimulates ILP3 secretion to activate fat body mTOR (Kim and Neufeld, 2015; Yamada et al., 2018). Work from Varghese et al. (2010), also implicates ILP3 in lipid homeostasis. ilp3 overexpression is sufficient to rescue hyperlipidemia in miR-14 mutant flies, though this function has not been further researched (Varghese et al., 2010). Interestingly, ilp3 expression decreases with age, whereas most ILPs are known to increase (Tanabe et al., 2017). According to one study, ilp3 is modestly increased in diapause (Kučerová et al., 2016). However, separate work demonstrates that mutants lacking ilp2, ilp3, and ilp5 are more prone to diapause induction (Kubrak et al., 2014; Schiesari et al., 2016). Clearly, the function of ILP3 is dependent on competing signals in ways we do not yet understand. The age-associated changes in ILP3 will be of particular interest to the biology of aging, warranting further inspection into the opposing expression trajectories between old age and diapause.
Insulin-Like Peptide 8
ILP8 is relaxin-like and binds the leucine-rich repeat-containing G protein-coupled receptor 3 (Lgr3), as well as InR (Colombani et al., 2015; Garelli et al., 2015; Vallejo et al., 2015). Lgr3 is expressed throughout the organism, including the plasma membranes of the IPCs where it can be bound to modify insulin signaling. ilp8 expression typically follows the metamorphic peaks of ecdysone, but is also induced through JNK signaling coordinated by Eiger, the TNFα ligand homolog in Drosophila (Sanchez et al., 2019). The latter scenario occurs in the imaginal disks through disturbance of tissue growth via genetic or mechanical injury to the organism during development (Colombani et al., 2012; Garelli et al., 2012; Ray and Lakhotia, 2017; Gontijo and Garelli, 2018). ILP8 delays development by inhibiting the production of PTTH, thereby modifying the release of ecdysone and allowing time to repair the damaged tissue before progressing to the next molt (Colombani et al., 2015; Ray and Lakhotia, 2017; Gontijo and Garelli, 2018). The role of ILP8 in diapause has not yet been explored, but as a stress-responsive peptide with the ability to delay development and upregulate repair processes, it is worthwhile to consider ILP8 in the diapause context. Accordingly, ilp8 supports survival during starvation and regulates reproduction in young adults (Ables et al., 2016). Loss of ilp8 reduces fecundity, though this appears to be caused by a defect in oviposition, rather than ovarian development (Liao and Nässel, 2020). In contrast, a separate study found that activation of Lgr3-expressing neurons in the abdominal ganglion of female flies decreases fecundity and discourages mating behavior (Meissner et al., 2016). The discrepancy is likely due to the difference in signaling locale, as well as the unknown molecular events that ILP8 causes by binding to InR. Taken together, ILP8 controls characteristics of diapause at various developmental stages. ILP8 may also contribute to longevity during diapause through its known roles increasing stress resistance. More research is needed to determine the place of ILP8 in the regulation of lifespan.
Insulin-Like Peptide 5
Although little is known about the function of ILP5, the majority of published work suggests it responds in a manner similar to ILP2 (Géminard et al., 2009; Wigby et al., 2011; Bai et al., 2012; Schiesari et al., 2016; Toprak, 2020). However, ILP5 is unique in certain respects. ilp5 is expressed in the IPCs, as well as the renal tubes and ovarian follicles, whereas ilp2 is excluded from these tissues (Ikeya et al., 2002; Okamoto et al., 2009; Söderberg et al., 2011). Intriguingly, ilp5 is the only Drosophila ILP that is strongly reduced under dietary restriction (Min et al., 2008; Bai et al., 2012). This effect appears in larvae, IPCs, and principal cells of the renal tubes (Min et al., 2008; Géminard et al., 2009; Bai et al., 2012; Okamoto and Nishimura, 2015). In the IPCs, ILP5 becomes sequestered during dietary restriction while its mRNA transcript decreases (Géminard et al., 2009). mRNA levels of ilp5 have not yet been tested in the renal tubes, but ILP5 itself is shown to decrease in this tissue (Söderberg et al., 2011). Loss of ilp5 is correlated with, but does not cause, the lifespan extension phenotype observed with dietary restriction (Min et al., 2008). Rather, recent work shows that ilp5 supports proper growth during poor nutrient conditions, inviting the possibility of a role for ILP5 in diapause (Okamoto and Nishimura, 2015). ILP5 mediates growth in response to the presence of amino acids in the fat body (Min et al., 2008; Broughton et al., 2010; Okamoto and Nishimura, 2015; Post and Tatar, 2016). It follows that ilp5 expression is negatively regulated by FoxO and ILP6, two factors expressed during diapause and other non-feeding stages. Repression of ilp5 may constitute part of a metabolic signaling pathway responsible for diapause induction. Supporting this, ilp5-null mutants have delayed time to pupariation (Okamoto and Nishimura, 2015). Loss of ilp5 is also associated with reduced mating physiology and behavior, as well as increased survival during desiccation and oxidative stress (Söderberg et al., 2011; Wigby et al., 2011; Schiesari et al., 2016). ilp5/ilp2 double mutants exhibit enhanced diapause inducibility, though this effect was not tested in single mutants, demonstrating the need for more direct evidence about the function of ILP5 in diapause (Schiesari et al., 2016).
Insulin-Like Peptides 7 and 4
Least is known about ILP7 and especially ILP4. Recent work shows that, in addition to ILP8, ILP7 is able to bind Lgr3 (Meissner et al., 2016; Prince et al., 2021). ILP7 acts upstream of IPC-derived ILPs to maintain IIS while the availability of dietary amino acids is low. This pathway is particularly relevant during diapause conditions which do not support the proliferation of protein-rich food sources (yeast on rotting fruit). Indeed, Drosophila show preference for plant-based food sources under conditions of cold temperatures (Brankatschk et al., 2014, 2018). Therefore, it may be the case that ILP7 plays a similar, yet distinct role to ILP5. ILP7 may regulate lifespan in diapause through upstream modulation of IPC-derived ILPs such as the pro-aging ILP2 or the pro-longevity ILP1.
Concluding Statement on Insulin
Though diapause preparations help shield the organism from environmental stress, ILP signaling must remain dynamic throughout the phases of diapause to accommodate challenges both expected and unexpected (Denlinger, 2002; Sim and Denlinger, 2013; Chatterjee et al., 2014; Kubrak et al., 2014). This statement contrasts previous assertions that IIS is generally suppressed in diapause. However, as research on diapause has developed, more studies have examined IIS from a greater diversity of angles, creating a more complicated and realistic perspective of diapause regulation. The physiological state at the time of diapause induction is not the same as the state throughout, nor upon termination. Recent work suggests that diapause is best construed as an alternative phase of life during which energy is not constantly conserved, but metabolized in a more judicious way.
Adipokinetic Hormone
Adipokinetic hormone, the functional insect homolog to glucagon, is responsible for the mobilization of energy stores from the fat body (Isabel et al., 2005; Mochanová et al., 2018). During early phases of Drosophila diapause, akh mRNA increases as mRNA levels of ilp2, ilp3, and ilp5 decrease (Kubrak et al., 2014; Sinclair and Marshall, 2018; Post et al., 2019). The corresponding metabolic effects of these changes be observed in IPC-ablated flies, suggesting that the transcriptional profile induced via IPC ablation may share certain features with that of diapause (Ikeya et al., 2002; Reyes-DelaTorre et al., 2012). Indeed, genetic upregulation of AKH has been observed to cause similar phenotypes as in diapause, including longevity and starvation resistance (Waterson et al., 2014; Kučerová et al., 2016; Hughson et al., 2021). Though the diapause-associated increase in AKH might be seen as counterintuitive, given the typical diapause preparatory accumulation of energy stores, AKH is likely upregulated in early diapause to support the production of trehalose (Isabel et al., 2005; Hahn and Denlinger, 2011; Li et al., 2015). Trehalose is glucose disaccharide that functions as a cryoprotectant and energy source in diapause (Elbein et al., 2003; Ohtake and Wang, 2011; Teets and Denlinger, 2013). Additionally, trehalose is reported to induce autophagy and longevity, and confer resistance to infection and desiccation in non-insect models (Kyryakov et al., 2012; Tapia et al., 2015; Belzile et al., 2016; Rusmini et al., 2018; Seo et al., 2018; Yu et al., 2021). In insects, however, there is currently a lack of evidence to definitively determine whether trehalose confers the longevity associated with akh expression (Broughton et al., 2008). This discrepancy may be explained by the fact that the synthesis of trehalose is also supported by FoxO; instead of AKH, FoxO may upregulate trehalose production in parallel to other FoxO-dependent, beneficial effects observed in non-insect models (Hibshman et al., 2017). Alternatively, FoxO may promote trehalose production (or longevity) through the upregulation of AKH, which could confer longevity through means other than trehalose (Bednářová et al., 2015, 2018). One proposed mechanism involves Target of Brain Insulin (Tobi) (Buch et al., 2008). Tobi is an AKH-responsive alpha-glucosidase responsible for hydrolyzation of advanced glycation end products (AGEs), which are associated with diseases of old age in various model organisms (Oudes et al., 1998; Semba et al., 2010; Chaudhuri et al., 2018). In a transcriptomic study examining the mechanism of longevity in E(z) mutants, tobi was the most highly differentially expressed gene. Despite this, overexpression of tobi was found to diminish lifespan in flies (Buch et al., 2008; Moskalev et al., 2019).
The molecular mechanism underlying the pro-longevity effects of AKH in insects remains mysterious. Bednářová et al. (2015) showed this is achieved at least in part through activation of FoxO, indicating that diapause-like phenotypes induced by AKH are coordinated through feedback with IIS. During development, AKH is secreted from a subset of cells in the corpora cardiaca during periods of perceived nutritional deficiency to maintain metabolic homeostasis and resist starvation (Sinclair and Marshall, 2018). Recent work from Hughson et al. (2021), found that AKH during development helps regulate the timing of progression through molts by integrating environmental cues about nutrient availability. Authors observed that AKH signaling indeed participates in the endocrine regulation of development by signaling through projections of the corpora cardiaca that innervate the PG, suggesting additional interplay in developmental progress or diapause initiation through ecdysone. Supporting this notion, larvae without the AKH-responsive locus dgc2 have delayed timing to pupariation when exposed to low nutrient conditions (Hughson et al., 2021).
Juvenile Hormone
Though it has long been established that changes in the titers of juvenile hormones (JH) are associated with reproductive diapause, these studies are largely based on anatomical observations following injection or topical application (Denlinger et al., 2012). In most insect species, the induction of diapause coincides with a marked decrease in circulating JH (Agui et al., 1991; Hiroyoshi et al., 2017; Ma et al., 2021). In fewer species, however, diapause occurs with an increase in JH (Yin and Chippendale, 1973; Denlinger et al., 2012; Bebas et al., 2018). Research into the molecular regulation of JH in diapause is still in its infancy. JH is most well-studied during development as a metamorphic antagonist and activator of IIS (Minakuchi et al., 2008; Konopova et al., 2011; Kayukawa et al., 2016). The synthesis of JH takes place in the corpora allata (CA), a component of the ring gland that is retained in adulthood, under a complex and poorly understood regulatory network controlled by 20HE, as well as circadian rhythm-associated clock genes, allatotropin, allatostatin, and nutritional signals conveyed via IIS/TOR (Ikeno et al., 2010; Shin et al., 2012; Abdel-latief and Hoffmann, 2014; Kang et al., 2014; Ojima et al., 2018; Guo et al., 2021; Yang et al., 2021). From the CA, JH enters the hemolymph and travels to target tissues via juvenile hormone-binding protein, where it then utilizes the receptors Methoprene-tolerant (Met) and Germ cell-expressed (Gce), leading to the transcription and activation of anti-metamorphic targets such as Krüppel-homolog 1 and Hairy (Shin et al., 2012; Jindra et al., 2013; Gujar and Palli, 2016; Kayukawa et al., 2016; Zhang et al., 2019; Yang et al., 2021). Met is also capable of limiting metamorphic transcription further upstream through direct interaction with EcR and USP (Bitra and Palli, 2009; Zhao et al., 2014).
Upregulation of JH biosynthetic genes occurs in between molts and is responsible for the stimulation of growth to achieve critical mass (Truman and Riddiford, 2007; Borras-Castells et al., 2017). Conversely, JH is degraded rapidly at the onset of metamorphosis by a series of enzymes that convert active JH to JH acid diol and JH diol phosphate (Li et al., 2005; Lyu et al., 2019). Degradation of JH is also maintained during most instances of insect diapause, which has varied effects depending on the organism’s developmental stage (Denlinger et al., 2012; Fu et al., 2016; Tian et al., 2021). Specifically, the effects of low JH include the enhancement of innate immunity genes, desensitization to 20HE through reduction of a JH-inducible ecdysone competence factor, and the suppression of reproductive development and organismal growth via downregulation of IIS (Zhu et al., 2003, 2010; Flatt et al., 2008; Sheng et al., 2011; Mirth et al., 2014; Kayukawa et al., 2016; Borras-Castells et al., 2017). The JH-IIS axis has not yet been thoroughly explored, in spite of its relevance for both insect diapause and aging biology. In Drosophila, loss of JH signaling via CA ablation causes diapause phenotypes such as slow growth and longevity in a FoxO-dependent manner (Tatar et al., 2001; Mirth et al., 2014). Reciprocally, perturbations in IIS contribute to loss of JH (Tu et al., 2005). In Aedes aegypti, transcript levels of amino acid and sugar transporters are lower in diapause, likely contributing to reduced IIS through the low JH characteristic of diapause (Zhu et al., 2010). Evidence from tsetse flies also suggests an intersection of environmental stress, low JH and insulin, though more research is needed to understand how conserved the relationship between JH and IIS is across taxa (Baumann et al., 2013).
By contrast, the role of JH in reproduction is better understood. JH controls ovarian maturation in conjunction with ecdysone through the production of the oocyte yolk protein vitellogenin (Chinzei et al., 1982; Wyatt and Davey, 1996; Sheng et al., 2011; Yamamoto et al., 2013). This function was shown to be mediated by IIS in Tribolium castaneum (Sheng et al., 2011; Xu et al., 2013). It follows that the loss of reproduction in adult diapause is JH-dependent, although studies in Colaphellus bowringi and Melinda pusilla suggest JH alone is insufficient to control the non-reproductive adult diapause phenotype (Agui et al., 1991; Guo et al., 2019). Knockdown of diapause-associated JH degradation genes did not completely rescue ovarian development in diapause-destined bowringi females (Guo et al., 2019). Similarly, supplementation with JH analog restored ovarian development in diapausing pusilla females in a manner dependent on diapause phase (Agui et al., 1991). JH appears to be primarily regulated at the level of synthesis and degradation, though these results may still be explained through JH if downstream JH signaling components are lower in diapause (Gilbert et al., 2000; Tian et al., 2021). Of note is the observation that diapause traits other than reproduction were ameliorated upon knockdown of JH degradation genes, highlighting the expansive and complicated roles of JH in diapause. Complementing this idea, it has been shown that JH is also insufficient to control the timing of metamorphosis in early (larval) instars (Tan et al., 2005; Smykal et al., 2014). This may be related to the observation that diapause incidence in pre-adult molts tends to depend more on ecdysone than JH, assuming some mechanistic similarities between metamorphosis and diapause, although this relationship is not well conserved (Batz et al., 2019). In general, while the main outcomes of each insect endocrine molecule are similar across taxa, little is conserved in exactly how these hormones function together (Flatt et al., 2005; Mirth et al., 2014).
Among other diapause phenotypes, loss of JH increases lifespan in a multitude of species (Herman and Tatar, 2001; Yamamoto et al., 2013). Thus far little effort has been dedicated to understanding the molecular basis for longevity associated with low JH. It was previously accepted that longevity in diapause (and in broader contexts) resulted from a tradeoff with reproduction; the organism devotes energetic resources toward either maintenance of the gonads or the somatic tissues (Flatt and Kawecki, 2007). However, it has since been demonstrated that this “tradeoff” is coincidental, as lifespan can be uncoupled from reproductive capacity in plentiful ways (Corona et al., 2007; Yamamoto et al., 2013; Flatt and Partridge, 2018; Maklakov and Chapman, 2019; Moskalev et al., 2019). Given that diapause typically begins through downregulation of JH synthesis, it is reasonable to consider that loss of JH extends lifespan by inducing biological programs typically reserved for diapause. Yet, while genetic or surgical manipulations interrupt JH synthesis throughout the lifespan of the organism, levels of JH fluctuate during diapause (Denlinger et al., 1984; Jiang et al., 2011). Natural periods of JH elevation in diapause do not result in diapause termination and the organism remains extraordinarily long-lived, often far beyond the lifespan of insects unable to synthesize JH. Therefore, the JH-dependent mechanisms of longevity may borrow from, but do not replicate, mechanisms of longevity in diapause. Modification to IIS might represent one mode by which JH-null mutants exhibit extended lifespan in a way that borrows from programs of diapause (Cambron et al., 2021). As mentioned, the precise mechanisms linking JH signaling to IIS are poorly defined. Other modes include modifications to lipid metabolism, immunity, protein stability, mTOR, Jun-N-terminal kinase (JNK), or cell cycle/developmental programs dependent on Janus kinase/signal transducer and activator of transcription (JAK/STAT), all of which have been significantly noted in transcriptomic analyses of diapausing insects (Poelchau et al., 2013; Kubrak et al., 2014; Yocum et al., 2015; Kankare et al., 2016; Santos et al., 2018; Cambron et al., 2021). Unfortunately, these pathways have yet to be adequately explored at the intersection of diapause and lifespan. Employment of omics tools over the past several years has generated a wealth of information that will continue to provide avenues for future research into the molecular regulation of lifespan in diapause.
Prothoracicotropic Hormone
Literature is scarce on the subject of prothoracicotropic hormone (PTTH) in diapause, but it is nonetheless important to discuss in this review for its intersection with more well-studied modes of diapause induction and exit. The traditional regulatory interaction between ecdysone and JH is mediated through PTTH and its receptor, Torso (Rewitz et al., 2009). As an activator of ecdysone biosynthesis, PTTH-producing neurons innervate the PG. Juvenile hormone binds and inhibits PTTH-producing neurons in between molts to limit the production of ecdysone. When JH is degraded at critical weight, PTTH-producing neurons become disinhibited, causing release of PTTH (Mirth et al., 2014). Subsequent binding to Torso located on the PG activates Ras/Raf/ERK signaling downstream, which increases intracellular cAMP to promote ecdysone biosynthesis.
However, recent work has prompted reanalysis of the canonical ecdysone synthesis pathway (Cruz et al., 2020; Pan and O’Connor, 2021). In both Drosophila and Bombyx mori, null mutants of PTTH or Torso will delay metamorphic transition from larvae to pupae, but will not arrest development indefinitely, implying the dispensability of PTTH for ecdysone biosynthesis in at least some insects (Uchibori-Asano et al., 2017). Similarly, about 50% of Drosophila with ablated PTTH-producing neurons will still undergo metamorphosis, albeit with significant delay. These findings strongly suggest another mode apart from PTTH is responsible for managing ecdysone at developmental transitions.
Cruz et al. (2020) have demonstrated that Egf signaling fills the dispensability gap of PTTH by activating Ras/Raf/ERK in parallel. Unlike Ptth-null mutants, Egfr-null mutants arrest development and do not resume. Importantly, this was shown to be dependent on ecdysone vesicle trafficking and biosynthesis. These results suggest Egf signaling may be the primary mode of regulation of ecdysone biosynthesis at developmental transitions. Additionally, Pan and O’Connor (2021) have identified several more candidate signaling pathways apart from PTTH to fine-tune metamorphic timing via ecdysone biosynthesis. Loss of anaplastic lymphoma kinase (Alk) and PDGF and VEGF receptor-related (Pvr) in the PG causes developmental delay in Drosophila. Ligands for these receptors originate from the PTTH-producing neurons, implying Alk, Pvr, and Torso have redundant functions in regulating ecdysone (Pan and O’Connor, 2021).
Under this model, PTTH is best construed as a means by which insects fine-tune the production of ecdysone in response to situational cues. This revision has clear implications for diapause, as one situation wherein environmental changes prompt adjustments to ecdysone signaling that occur outside the typical developmental program. This position is made stronger through the examination of previous work linking PTTH to growth regulators ILP8 and the glial amino acid transporter Sobremesa (Sbm) (Colombani et al., 2012; Galagovsky et al., 2018; Delanoue and Romero, 2020). Both ILP8 and Sbm suppress PTTH in response to various stressors to prevent metamorphosis under unfavorable conditions. ILP8 may also suppress PTTH during diapause, given the metabolic similarities between metamorphosis and diapause, as well as the fact that circulating PTTH is often low during diapause (Denlinger et al., 2012). Alternatively, in species that exhibit high ecdysone during diapause initiation, PTTH may serve to interrupt JH-dependent growth in between molts when Egf signaling is low.
To date, there has been no obvious role for PTTH in diapause, perhaps due to the inconsistent role of ecdysone across species. Recent advances have begun to paint a more complex and accurate picture of ecdysone regulation that will change the portrayal of PTTH in future literature.
Future Directions and Conclusion
Diapause has evolved to incorporate information from every feature of seasonal change that could hinder the organism’s path to reproductive success. Considering this, the complex nature of the diapause endocrine program is to be expected. Peptide hormones from distant tissues must communicate with signaling programs elsewhere to coordinate responses in behavior, metabolism, growth, cuticle structure, dietary preferences, and more, in ways that generally run counter to the normal trajectory of development. Diapause cannot be achieved without careful timing and balance of hormonal transitions. This is best demonstrated through the well-documented developmental lethality of topical JH, or the many partial diapause phenotypes achievable through piecewise interventions in endocrine signaling (Spielman and Williams, 1966; Shaaya and Pisarev, 1986). Evolution has arrived at this balance on many occasions, given the different—and sometimes opposing—ways that different species initiate diapause. Indeed, the extension of diapause into vertebrate systems such as bats, rodents, fish, birds, bears and marsupials gives hope that later work will compare mechanisms of diapause across phyla (Ptak et al., 2012; Deng et al., 2018; Rasweiler and Badwaik, 2020).
Partial diapause is readily inducible through manipulations in IIS, diet, and other aspects of physiology. However, even interventions of major upstream regulators (InR deletion, IPC ablation, CA removal, CC stimulation), do not recapitulate diapause entirely. Diapause endocrinology is a study of interactions. It is not yet fully understood how most hormones interact throughout the phases of diapause, nor through which pathways, to arrive at homeostasis. Valuable studies have begun to connect these pieces, though more translational work is needed to construct a cohesive network of endocrine in diapause. In a similar sense, lifespan is a multi-faceted trait whose length depends on species-specific adaptations, in addition to environmental ones (Rasweiler and Badwaik, 2020). Much has been accomplished in recent years to characterize the molecular events responsible for these adaptations. Still, even more could be achieved through their examination in light of evolution. The aim of this review is to poise diapause endocrinology in a way that provides context to the question of how a species arrives at its normal lifespan. Diapause is a natural case of lifespan plasticity; the mechanisms that determine a species’ lifespan are dramatically altered in a very conserved manner, making them able to be probed scientifically. Without this context, discoveries about the determination of lifespan are rarer and more difficult to understand, as they lack the evolutionary perspective needed to describe the extension in a systemic way.
Canonical regulators of aging are implicated in diapause. Insofar as both aging and diapause involve changes to most aspects of physiology, significant overlap is expected. However, beyond the shared categories of physiology, the specific ways in which physiology is altered in diapause reflects physiological changes in laboratory extensions of lifespan. For example, somatic reduction of mTOR activity is known to extend lifespan outside the immediate context of diapause, and is also observed in diapause (Kapahi et al., 2004; Liu et al., 2017; Cohen, 2018). Other pathways beyond the scope of this review are regulated similarly, such as the retention of genomic stability or the induction of longevity-associated microRNAs (Saravanakumar et al., 2010; Liu et al., 2012; Vijg and Suh, 2013; Batz et al., 2017; Gendron and Pletcher, 2017; Reynolds et al., 2017). These similarities suggest that evolutionarily conserved aspects of diapause are the natural context for why laboratory manipulations extend lifespan and increase stress resistance. However, laboratory manipulations of lifespan do not replicate the extreme longevity of diapause. As mentioned, this may be attributed to the fact that laboratory manipulations of lifespan induce partial diapause, but fail to capture the intricate balance of hormones required for full diapause.
Other insect peptide hormones and neuropeptides that were not included in this review are likely regulated in diapause-specific manners (Nässel and Zandawala, 2020). However, less is known about the specific functions of these peptides in diapause. The purpose of this review is to speculate about roles in longevity for diapause-associated hormones; therefore, it would be inappropriate to do so using a peptide whose role in diapause is already speculative. The exception to this statement is the diapause hormone of B. mori (Hasegawa, 1964; Jiang et al., 2019). Secretion of diapause hormone is promoted in oocytes destined for diapause by environmental perception of the mother pre-deposition. Significant work has dissected the regulation of B. mori diapause in relation to numerous aspects of physiology, including other endocrine molecules, but thus far diapause hormone appears to be exclusive to moths (Ichikawa et al., 1997; Cui et al., 2021; Tsuchiya et al., 2021). While diapause hormone likely impacts lifespan, research in the biology of aging most clearly benefits from examination of mechanisms in diapause that are well-conserved. For this reason, diapause hormone is not examined here in the context of lifespan.
Diapause represents a fantastic model system to understand the complex intersection of environmental stimuli, endocrine regulation, development and longevity. From current literature, it can be inferred that a complete understanding of diapause requires integration of knowledge from domains in biology that are often studied separately. Advances in omics technology provide a highly informative and efficient means to accomplish this. An increasing number of omics experiments under various conditions relevant to, or directly observing, insect diapause are mapping the contributions of individual hormones to diapause traits. Comparing these studies with data from single interventions in longevity could help identify mechanisms of lifespan plasticity in diapause, while supplying evolutionary context for how lifespan is determined in non-diapausing species. However, metanalytical work such as this is rarely performed, and even within the same species, there is little consistency in experimental design and choice of analysis. These are obstacles that must be controlled for. Comparative omics could discern conserved mechanisms from non-conserved ones that define the diapause endocrine program across species. Future work will reveal much about how lifespan evolved as a plastic component in the larger system of diapause.
Author Contributions
The author confirms being the sole contributor of this work and has approved it for publication.
Funding
This work was funded by the NIH grants T32AG041688 and R37AG024360.
Conflict of Interest
The author declares that the research was conducted in the absence of any commercial or financial relationships that could be construed as a potential conflict of interest.
Publisher’s Note
All claims expressed in this article are solely those of the authors and do not necessarily represent those of their affiliated organizations, or those of the publisher, the editors and the reviewers. Any product that may be evaluated in this article, or claim that may be made by its manufacturer, is not guaranteed or endorsed by the publisher.
Abbreviations
JH, juvenile hormone; IIS, insulin/insulin-like signaling; AKH, adipokinetic hormone; PTTH, prothoracicotropic hormone; ILP, insulin-like peptide; 20HE, 20-hydroxyecdysone; PG, prothoracic gland; CA, corpora allata; CC, corpora cardiaca; IPCs, insulin producing cells.
References
Abdel-latief, M., and Hoffmann, K. H. (2014). Functional activity of allatotropin and allatostatin in the pupal stage of a holometablous insect. Tribolium castaneum (Coleoptera, Tenebrionidae). Peptides 53, 172–184. doi: 10.1016/j.peptides.2013.10.007
Ables, E. T., Hwang, G. H., Finger, D. S., Hinnant, T. D., and Drummond-Barbosa, D. (2016). A genetic mosaic screen reveals ecdysone-responsive genes regulating Drosophila oogenesis. G3 6, 2629–2642. doi: 10.1534/g3.116.028951
Agui, N., Mihara, M., and Kurahashi, H. (1991). Effect of juvenile hormone analogue on ovarian development of the reproductive-diapausing parasitic fly, Melinda pusilla. Med. Entomol. Zool. 42, 311–317. doi: 10.7601/mez.42.311
Ahmad, M., He, L., and Perrimon, N. (2020). Regulation of insulin and adipokinetic hormone/glucagon production in flies. WIRES Dev. Biol. 9:e360. doi: 10.1002/wdev.360
Alejandro, E. U., Bozadjieva, N., Blandino-Rosano, M., Wasan, M. A., Elghazi, L., Vadrevu, S., et al. (2017). Overexpression of kinase-dead mtor impairs glucose homeostasis by regulating insulin secretion and not β-Cell mass. Diabetes 66, 2150–2162. doi: 10.2337/db16-1349
Almudi, I., Poernbacher, I., Hafen, E., and Stocker, H. (2013). The Lnk/SH2B adaptor provides a fail-safe mechanism to establish the Insulin receptor-Chico interaction. Cell Commun. Signal. 11:26. doi: 10.1186/1478-811X-11-26
Altintas, O., Park, S., and Lee, S.-J. V. (2016). The role of insulin/IGF-1 signaling in the longevity of model invertebrates, C. elegans and D. melanogaster. BMB Rep. 49, 81–92. doi: 10.5483/BMBRep.2016.49.2.261
Ashburner, M. (1973). Sequential gene activation by ecdysone in polytene chromosomes of Drosophila melanogaster: I. dependence upon ecdysone concentration. Dev. Biol. 35, 47–61. doi: 10.1016/0012-1606(73)90006-7
Ashburner, M. (1990). Puffs, genes, and hormones revisited. Cell 61, 1–3. doi: 10.1016/0092-8674(90)90205-s
Bai, H., Kang, P., and Tatar, M. (2012). Drosophila insulin-like peptide-6 (dilp6) expression from fat body extends lifespan and represses secretion of Drosophila insulin-like peptide-2 from the brain. Aging Cell 11, 978–985. doi: 10.1111/acel.12000
Barber, A. F., Erion, R., Holmes, T. C., and Sehgal, A. (2016). Circadian and feeding cues integrate to drive rhythms of physiology in Drosophila insulin-producing cells. Genes Dev. 30, 2596–2606. doi: 10.1101/gad.288258.116
Batz, Z. A., Brent, C. S., Marias, M. R., Sugijanto, J., and Armbruster, P. A. (2019). Juvenile hormone iii but not 20-hydroxyecdysone regulates the embryonic diapause of Aedes albopictus. Front. Physiol. 10:1352. doi: 10.3389/fphys.2019.01352
Batz, Z. A., Goff, A. C., and Armbruster, P. A. (2017). MicroRNAs are differentially abundant during Aedes albopictus diapause maintenance but not diapause induction. Insect Mol. Biol. 26, 721–733. doi: 10.1111/imb.12332
Baumann, A. A., Benoit, J. B., Michalkova, V., Mireji, P., Attardo, G. M., Moulton, J. K., et al. (2013). Juvenile hormone and insulin suppress lipolysis between periods of lactation during tsetse fly pregnancy. Mol. Cell Endocrinol. 372, 30–41. doi: 10.1016/j.mce.2013.02.019
Bebas, P., Cymborowski, B., Kazek, M., and Polanska, M. A. (2018). Effects of larval diapause and the juvenile hormone analog, fenoxycarb, on testis development and spermatogenesis in the wax moth. Galleria mellonella (Lepidoptera: Pyralidae). EJE 115, 400–417. doi: 10.14411/eje.2018.040
Beckstead, R. B., Lam, G., and Thummel, C. S. (2007). Specific transcriptional responses to juvenile hormone and ecdysone in Drosophila. Insect Biochem. Mol. Biol. 37, 570–578. doi: 10.1016/j.ibmb.2007.03.001
Bednářová, A., Kodrík, D., and Krishnan, N. (2015). Knockdown of adipokinetic hormone synthesis increases susceptibility to oxidative stress in Drosophila–a role for dFoxO? Comp. Biochem. Physiol. C Toxicol. Pharmacol. 171, 8–14. doi: 10.1016/j.cbpc.2015.03.006
Bednářová, A., Tomèala, A., Mochanová, M., Kodrík, D., and Krishnan, N. (2018). Disruption of adipokinetic hormone mediated energy homeostasis has subtle effects on physiology. behavior and lipid status during aging in Drosophila. Front. Physiol. 9:949. doi: 10.3389/fphys.2018.00949
Belzile, J.-P., Sabalza, M., Craig, M., Clark, A. E., Morello, C. S., and Spector, D. H. (2016). Trehalose, an mTOR-Independent inducer of autophagy, inhibits human cytomegalovirus infection in multiple cell types. J. Virol. 90, 1259–1277. doi: 10.1128/JVI.02651-15
Bitra, K., and Palli, S. R. (2009). Interaction of proteins involved in ecdysone and juvenile hormone signal transduction. Arch. Insect Biochem. Physiol. 70, 90–105. doi: 10.1002/arch.20281
Borras-Castells, F., Nieva, C., Maestro, J. L., Maestro, O., Belles, X., and Martín, D. (2017). Juvenile hormone biosynthesis in adult Blattella germanica requires nuclear receptors Seven-up and FTZ-F1. Sci. Rep. 7:40234. doi: 10.1038/srep40234
Brankatschk, M., Dunst, S., Nemetschke, L., and Eaton, S. (2014). Delivery of circulating lipoproteins to specific neurons in the Drosophila brain regulates systemic insulin signaling. ELife 3:e02862. doi: 10.7554/eLife.02862
Brankatschk, M., Gutmann, T., Knittelfelder, O., Palladini, A., Prince, E., Grzybek, M., et al. (2018). A temperature-dependent switch in feeding preference improves drosophila development and survival in the cold. Dev. Cell 46, 781.e–793.e. doi: 10.1016/j.devcel.2018.05.028
Brogiolo, W., Stocker, H., Ikeya, T., Rintelen, F., Fernandez, R., and Hafen, E. (2001). An evolutionarily conserved function of the Drosophila insulin receptor and insulin-like peptides in growth control. Curr. Biol. 11, 213–221. doi: 10.1016/S0960-9822(01)00068-9
Broughton, S. J., Piper, M. D. W., Ikeya, T., Bass, T. M., Jacobson, J., Driege, Y., et al. (2005). Longer lifespan, altered metabolism, and stress resistance in Drosophila from ablation of cells making insulin-like ligands. PNAS 102, 3105–3110. doi: 10.1073/pnas.0405775102
Broughton, S. J., Slack, C., Alic, N., Metaxakis, A., Bass, T. M., Driege, Y., et al. (2010). DILP-producing median neurosecretory cells in the Drosophila brain mediate the response of lifespan to nutrition. Aging Cell 9, 336–346. doi: 10.1111/j.1474-9726.2010.00558.x
Broughton, S., Alic, N., Slack, C., Bass, T., Ikeya, T., Vinti, G., et al. (2008). Reduction of DILP2 in Drosophila triages a metabolic phenotype from lifespan revealing redundancy and compensation among DILPs. PLoS One 3:e3721. doi: 10.1371/journal.pone.0003721
Buch, S., Melcher, C., Bauer, M., Katzenberger, J., and Pankratz, M. J. (2008). Opposing effects of dietary protein and sugar regulate a transcriptional target of Drosophila insulin-like peptide signaling. Cell Metab. 7, 321–332. doi: 10.1016/j.cmet.2008.02.012
Cambron, L. D., Yocum, G. D., Yeater, K. M., and Greenlee, K. J. (2021). Overwintering conditions impact insulin pathway gene expression in diapausing Megachile rotundata. Comp. Biochem. Physiol. A Mol. Integr. Physiol. 256:110937. doi: 10.1016/j.cbpa.2021.110937
Chatterjee, D., Katewa, S. D., Qi, Y., Jackson, S. A., Kapahi, P., and Jasper, H. (2014). Control of metabolic adaptation to fasting by dILP6-induced insulin signaling in Drosophila oenocytes. PNAS 111, 17959–17964. doi: 10.1073/pnas.1409241111
Chaudhuri, J., Bains, Y., Guha, S., Kahn, A., Hall, D., Bose, N., et al. (2018). The role of advanced glycation end products in aging and metabolic diseases: bridging association and causality. Cell Metab. 28, 337–352. doi: 10.1016/j.cmet.2018.08.014
Chell, J. M., and Brand, A. H. (2010). Nutrition-responsive glia control exit of neural stem cells from quiescence. Cell 143, 1161–1173. doi: 10.1016/j.cell.2010.12.007
Chinzei, Y., White, B. N., and Wyatt, G. R. (1982). Vitellogenin mRNA in locust fat body: identification, isolation, and quantitative changes induced by juvenile hormone. Can. J. Biochem. 60, 243–251. doi: 10.1139/o82-029
Chowański, S., Walkowiak-Nowicka, K., Winkiel, M., Marciniak, P., Urbański, A., and Pacholska-Bogalska, J. (2021). Insulin-Like peptides and cross-talk with other factors in the regulation of insect metabolism. Front. Physiol. 12:973. doi: 10.3389/fphys.2021.701203
Cohen, A. A. (2018). Aging across the tree of life: the importance of a comparative perspective for the use of animal models in aging. Biochim. Biophys. Acta 1864, 2680–2689. doi: 10.1016/j.bbadis.2017.05.028
Colombani, J., Andersen, D. S., and Léopold, P. (2012). Secreted peptide dilp8 coordinates Drosophila tissue growth with developmental timing. Science 336, 582–585. doi: 10.1126/science.1216689
Colombani, J., Andersen, D. S., Boulan, L., Boone, E., Romero, N., Virolle, V., et al. (2015). Drosophila Lgr3 couples organ growth with maturation and ensures developmental stability. Curr. Biol. 25, 2723–2729. doi: 10.1016/j.cub.2015.09.020
Colombani, J., Bianchini, L., Layalle, S., Pondeville, E., Dauphin-Villemant, C., Antoniewski, C., et al. (2005). Antagonistic actions of ecdysone and insulins determine final size in Drosophila. Science 310, 667–670. doi: 10.1126/science.1119432
Colombani, J., Raisin, S., Pantalacci, S., Radimerski, T., Montagne, J., and Léopold, P. (2003). A nutrient sensor mechanism controls Drosophila growth. Cell 114, 739–749. doi: 10.1016/s0092-8674(03)00713-x
Corona, M., Velarde, R. A., Remolina, S., Moran-Lauter, A., Wang, Y., Hughes, K. A., et al. (2007). Vitellogenin, juvenile hormone, insulin signaling, and queen honey bee longevity. PNAS 104, 7128–7133. doi: 10.1073/pnas.0701909104
Cruz, J., Martín, D., and Franch-Marro, X. (2020). Egfr signaling is a major regulator of ecdysone biosynthesis in the drosophila prothoracic gland. Curr. Biol. 30, 1547.e–1554.e. doi: 10.1016/j.cub.2020.01.092
Cui, W.-Z., Qiu, J.-F., Dai, T.-M., Chen, Z., Li, J.-L., Liu, K., et al. (2021). Circadian clock gene period contributes to diapause via GABAeric-diapause hormone pathway in bombyx mori. Biology 10:842. doi: 10.3390/biology10090842
Davidson, B., and Swalla, B. J. (2002). A molecular analysis of ascidian metamorphosis reveals activation of an innate immune response. Development 129, 4739–4751. doi: 10.1242/dev.129.20.4739
Delanoue, R., and Romero, N. M. (2020). Growth and maturation in development: a fly’s perspective. Int. J. Mol. Sci. 21:1260. doi: 10.3390/ijms21041260
Delanoue, R., Meschi, E., Agrawal, N., Mauri, A., Tsatskis, Y., McNeill, H., et al. (2016). Drosophila insulin release is triggered by adipose stunted ligand to brain methuselah receptor. Science 353, 1553–1556. doi: 10.1126/science.aaf8430
Delanoue, R., Slaidina, M., and Léopold, P. (2010). The steroid hormone ecdysone controls systemic growth by repressing dMyc function in Drosophila fat cells. Dev. Cell 18, 1012–1021. doi: 10.1016/j.devcel.2010.05.007
Deng, L., Li, C., Chen, L., Liu, Y., Hou, R., and Zhou, X. (2018). Research advances on embryonic diapause in mammals. Anim. Reprod. Sci. 198, 1–10. doi: 10.1016/j.anireprosci.2018.09.009
Denlinger, D. L. (2002). Regulation of diapause. Annu. Rev. Entomol. 47, 93–122. doi: 10.1146/annurev.ento.47.091201.145137
Denlinger, D. L., Shukla, M., and Faustini, D. L. (1984). Juvenile hormone involvement in pupal diapause of the flesh fly Sarcophaga Crassipalpis: regulation of infradian cycles of O2 consumption. J. Exp. Biol. 109, 191–199. doi: 10.1242/jeb.109.1.191
Denlinger, D. L., Valaitis, A. P., and Lee, K.-Y. (1997). Further evidence that diapause in the gypsy moth. Lymantria dispar, is regulated by ecdysteroids: a comparison of diapause and nondiapause strains. J. Insect Physiol. 43, 897–903. doi: 10.1016/s0022-1910(97)00054-1
Denlinger, D. L., Yocum, G. D., and Rinehart, J. P. (2012). “10-Hormonal Control of Diapause,” in Insect Endocrinology, ed. L. I. Gilbert (San Diego, CA: Academic Press), 430–463. doi: 10.1016/B978-0-12-384749-2.10010-X
Doğan, C., Güney, G., Güzel, K. K., Can, A., Hegedus, D. D., and Toprak, U. (2021). What You eat matters: nutrient inputs alter the metabolism and neuropeptide expression in egyptian cotton leaf worm, Spodoptera littoralis (Lepidoptera: Noctuidae). Front. Physiol. 12. Available online at: https://www.frontiersin.org/article/10.3389/fphys.2021.773688 (accessed January 19, 2022). doi: 10.3389/fphys.2021.773688
Elbein, A. D., Pan, Y. T., Pastuszak, I., and Carroll, D. (2003). New insights on trehalose: a multifunctional molecule. Glycobiology 13, 17R–27R. doi: 10.1093/glycob/cwg047
Flatt, T., and Kawecki, T. J. (2007). Juvenile hormone as a regulator of the trade-off between reproduction and life span in Drosophila melanogaster. Evolution 61, 1980–1991. doi: 10.1111/j.1558-5646.2007.00151.x
Flatt, T., and Partridge, L. (2018). Horizons in the evolution of aging. BMC Biol. 16:93. doi: 10.1186/s12915-018-0562-z
Flatt, T., Heyland, A., Rus, F., Porpiglia, E., Sherlock, C., Yamamoto, R., et al. (2008). Hormonal regulation of the humoral innate immune response in Drosophila melanogaster. J. Exp. Biol. 211, 2712–2724. doi: 10.1242/jeb.014878
Flatt, T., Tu, M.-P., and Tatar, M. (2005). Hormonal pleiotropy and the juvenile hormone regulation of Drosophila development and life history. Bioessays 27, 999–1010. doi: 10.1002/bies.20290
Franceschi, C., Garagnani, P., Parini, P., Giuliani, C., and Santoro, A. (2018). Inflammaging: a new immune–metabolic viewpoint for age-related diseases. Nat. Rev. Endocrinol. 14, 576–590. doi: 10.1038/s41574-018-0059-4
Fridell, Y.-W. C., Hoh, M., Kréneisz, O., Hosier, S., Chang, C., Scantling, D., et al. (2009). Increased uncoupling protein (UCP) activity in Drosophila insulin-producing neurons attenuates insulin signaling and extends lifespan. Aging 1, 699–713. doi: 10.18632/aging.100067
Fu, K.-Y., Li, Q., Zhou, L.-T., Meng, Q.-W., Lü, F.-G., Guo, W.-C., et al. (2016). Knockdown of juvenile hormone acid methyl transferase severely affects the performance of Leptinotarsa decemlineata (Say) larvae and adults. Pest Manag. Sci. 72, 1231–1241. doi: 10.1002/ps.4103
Galagovsky, D., Depetris-Chauvin, A., Manière, G., Geillon, F., Berthelot-Grosjean, M., Noirot, E., et al. (2018). Sobremesa L-type amino acid transporter expressed in glia is essential for proper timing of development and brain growth. Cell Rep. 24, 3156.e–3166.e. doi: 10.1016/j.celrep.2018.08.067
Garelli, A., Gontijo, A. M., Miguela, V., Caparros, E., and Dominguez, M. (2012). Imaginal discs secrete insulin-like peptide 8 to mediate plasticity of growth and maturation. Science 336, 579–582. doi: 10.1126/science.1216735
Garelli, A., Heredia, F., Casimiro, A. P., Macedo, A., Nunes, C., Garcez, M., et al. (2015). Dilp8 requires the neuronal relaxin receptor Lgr3 to couple growth to developmental timing. Nat. Commun. 6:8732. doi: 10.1038/ncomms9732
Gariou-Papalexiou, A., Mintzas, A. C., and Zacharopoulou, A. (2001). Ecdysone-regulated chromosome puffing in the salivary glands of the Mediterranean fruit fly, Ceratitis capitata. Genome 44, 752–762. doi: 10.1139/g01-068
Garschall, K., and Flatt, T. (2018). The interplay between immunity and aging in Drosophila. F1000Res 7:160. doi: 10.12688/f1000research.13117.1
Garsin, D. A., Villanueva, J. M., Begun, J., Kim, D. H., Sifri, C. D., Calderwood, S. B., et al. (2003). Long-lived C. elegans daf-2 mutants are resistant to bacterial pathogens. Science 300:1921. doi: 10.1126/science.1080147
Géminard, C., Rulifson, E. J., and Léopold, P. (2009). Remote control of insulin secretion by fat cells in Drosophila. Cell Metab. 10, 199–207. doi: 10.1016/j.cmet.2009.08.002
Gendron, C. M., and Pletcher, S. D. (2017). MicroRNAs mir-184 and let-7 alter Drosophila metabolism and longevity. Aging Cell 16, 1434–1438. doi: 10.1111/acel.12673
Ghbeish, N., Tsai, C.-C., Schubiger, M., Zhou, J. Y., Evans, R. M., and McKeown, M. (2001). The dual role of ultraspiracle, the Drosophila retinoid X receptor, in the ecdysone response. PNAS 98, 3867–3872. doi: 10.1073/pnas.061437798
Gibbs, A. G., and Markow, T. A. (2001). Effects of Age on Water Balance in Drosophila Species. Physiol. Biochem. Zool. 74, 520–530. doi: 10.1086/322162
Gibbs, A., Mousseau, T. A., and Crowe, J. H. (1991). Genetic and acclimatory variation in biophysical properties of insect cuticle lipids. Proc. Natl. Acad. Sci. U.S.A. 88, 7257–7260. doi: 10.1073/pnas.88.16.7257
Gilbert, L. I., Granger, A., and Roe, R. M. (2000). The juvenile hormones: historical facts and speculations on future research directions. Insect Biochem. Mol. Biol. 30, 617–644. doi: 10.1016/S0965-1748(00)00034-5
Gontijo, A. M., and Garelli, A. (2018). The biology and evolution of the Dilp8-Lgr3 pathway: a relaxin-like pathway coupling tissue growth and developmental timing control. Mech. Dev. 154, 44–50. doi: 10.1016/j.mod.2018.04.005
Grönke, S., Clarke, D.-F., Broughton, S., Andrews, T. D., and Partridge, L. (2010). Molecular evolution and functional characterization of drosophila insulin-like peptides. PLoS Genetics 6:e1000857. doi: 10.1371/journal.pgen.1000857
Gu, S.-H., Lin, J.-L., Lin, P.-L., and Chen, C.-H. (2009). Insulin stimulates ecdysteroidogenesis by prothoracic glands in the silkworm, Bombyx mori. Insect Biochem. Mol. Biol. 39, 171–179. doi: 10.1016/j.ibmb.2008.10.012
Gu, Y., Lindner, J., Kumar, A., Yuan, W., and Magnuson, M. A. (2011). Rictor/mTORC2 is essential for maintaining a balance between beta-cell proliferation and cell size. Diabetes 60, 827–837. doi: 10.2337/db10-1194
Gujar, H., and Palli, S. R. (2016). Krüppel homolog 1 and E93 mediate Juvenile hormone regulation of metamorphosis in the common bed bug, Cimex lectularius. Sci. Rep. 6:26092. doi: 10.1038/srep26092
Guo, S., Sun, D., Tian, Z., Liu, W., Zhu, F., and Wang, X.-P. (2019). The limited regulatory roles of juvenile hormone degradation pathways in reproductive diapause preparation of the cabbage beetle, Colaphellus bowringi. J. Insect Physiol. 119:103967. doi: 10.1016/j.jinsphys.2019.103967
Guo, S., Tian, Z., Wu, Q.-W., King-Jones, K., Liu, W., Zhu, F., et al. (2021). Steroid hormone ecdysone deficiency stimulates preparation for photoperiodic reproductive diapause. PLoS Genetics 17:e1009352. doi: 10.1371/journal.pgen.1009352
Hahn, D. A., and Denlinger, D. L. (2011). Energetics of insect diapause. Annu. Rev. Entomol. 56, 103–121. doi: 10.1146/annurev-ento-112408-085436
Hasegawa, K. (1964). Studies on the mode of action of the diapause hormone in the silkworm. Bombyx Mori L: II. content of diapause hormone in the suboesophageal ganglion. J. Exp. Biol. 41, 855–863. doi: 10.1242/jeb.41.4.855
Haselton, A., Sharmin, E., Schrader, J., Sah, M., Poon, P., and Fridell, Y.-W. C. (2010). Partial ablation of adult Drosophilainsulin-producing neurons modulates glucose homeostasis and extends life span without insulin resistance. Cell Cycle 9, 3135–3143. doi: 10.4161/cc.9.15.12458
Hayakawa, Y. (1991). Structure of a growth-blocking peptide present in parasitized insect hemolymph. J. Biol. Chem. 266, 7982–7984. doi: 10.1016/S0021-9258(18)92927-3
Hentze, J. L., Moeller, M. E., Jørgensen, A. F., Bengtsson, M. S., Bordoy, A. M., Warren, J. T., et al. (2013). Accessory gland as a site for prothoracicotropic hormone controlled ecdysone synthesis in adult male insects. PLoS One 8:e55131. doi: 10.1371/journal.pone.0055131
Herboso, L., Oliveira, M. M., Talamillo, A., Pérez, C., González, M., Martín, D., et al. (2015). Ecdysone promotes growth of imaginal discs through the regulation of Thor in D. melanogaster. Sci. Rep. 5:12383. doi: 10.1038/srep12383
Herman, W. S., and Tatar, M. (2001). Juvenile hormone regulation of longevity in the migratory monarch butterfly. Proc. Biol. Sci. 268, 2509–2514. doi: 10.1098/rspb.2001.1765
Hibshman, J. D., Doan, A. E., Moore, B. T., Kaplan, R. E., Hung, A., Webster, A. K., et al. (2017). Daf-16/FoxO promotes gluconeogenesis and trehalose synthesis during starvation to support survival. ELife 6:e30057. doi: 10.7554/eLife.30057
Hiroyoshi, S., Reddy, G. V. P., and Mitsuhashi, J. (2017). Effects of juvenile hormone analogue (methoprene) and 20-hydroxyecdysone on reproduction in Polygonia c-aureum (Lepidoptera: Nymphalidae) in relation to adult diapause. J. Comp. Physiol. A Neuroethol. Sens. Neural. Behav. Physiol. 203, 635–647. doi: 10.1007/s00359-017-1179-3
Holtof, M., Lenaerts, C., Cullen, D., and Vanden Broeck, J. (2019). Extracellular nutrient digestion and absorption in the insect gut. Cell Tissue Res. 377, 397–414. doi: 10.1007/s00441-019-03031-9
Hughson, B. N., Shimell, M., and O’Connor, M. B. (2021). AKH signaling in D. melanogaster alters larval development in a nutrient-dependent manner that influences adult metabolism. Front. Physiol. 12:42. doi: 10.3389/fphys.2021.619219
Ichikawa, T., Aoki, S., and Shimizu, I. (1997). Neuroendocrine control of diapause hormone secretion in the silkworm, Bombyx mori. J. Insect Physiol. 43, 1101–1109. doi: 10.1016/s0022-1910(97)00083-8
Ikeno, T., Tanaka, S. I., Numata, H., and Goto, S. G. (2010). Photoperiodic diapause under the control of circadian clock genes in an insect. BMC Biol. 8:116. doi: 10.1186/1741-7007-8-116
Ikeya, T., Galic, M., Belawat, P., Nairz, K., and Hafen, E. (2002). Nutrient-dependent expression of insulin-like peptides from neuroendocrine cells in the CNS contributes to growth regulation in Drosophila. Curr. Biol. 12, 1293–1300. doi: 10.1016/s0960-9822(02)01043-6
Isabel, G., Martin, J.-R., Chidami, S., Veenstra, J. A., and Rosay, P. (2005). AKH-producing neuroendocrine cell ablation decreases trehalose and induces behavioral changes in Drosophila. Am. J. Physiol. Regul. Integr. Comp. Physiol. 288, R531–R538. doi: 10.1152/ajpregu.00158.2004
Itskov, P. M., and Ribeiro, C. (2013). The dilemmas of the gourmet fly: the molecular and neuronal mechanisms of feeding and nutrient decision making in Drosophila. Front. Neurosci. 7:12. doi: 10.3389/fnins.2013.00012
Jiang, T., Chen, Y., Tan, Z., Li, J., Qian, P., Tang, S., et al. (2019). Expression analysis and functional identification of several genes related to diapause in Bombyx mori. Dev. Growth Differ. 61, 150–157. doi: 10.1111/dgd.12589
Jiang, X., Huang, S., and Luo, L. (2011). Juvenile hormone changes associated with diapause induction, maintenance, and termination in the beet webworm, Loxostege sticticalis (lepidoptera: pyralidae). Arch. Insect Biochem. Physiol. 77, 134–144. doi: 10.1002/arch.20429
Jindra, M., Palli, S. R., and Riddiford, L. M. (2013). The juvenile hormone signaling pathway in insect development. Annu. Rev. Entomol. 58, 181–204. doi: 10.1146/annurev-ento-120811-153700
Kang, D. S., Denlinger, D. L., and Sim, C. (2014). Suppression of allatotropin simulates reproductive diapause in the mosquito Culex pipiens. J. Insect Physiol. 64, 48–53. doi: 10.1016/j.jinsphys.2014.03.005
Kankare, M., Parker, D. J., Merisalo, M., Salminen, T. S., and Hoikkala, A. (2016). Transcriptional differences between diapausing and non-diapausing d. montana females reared under the same photoperiod and temperature. PLoS One 11:e0161852. doi: 10.1371/journal.pone.0161852
Kapahi, P., Kaeberlein, M., and Hansen, M. (2017). Dietary restriction and lifespan: lessons from invertebrate models. Ageing Res. Rev. 39, 3–14. doi: 10.1016/j.arr.2016.12.005
Kapahi, P., Zid, B. M., Harper, T., Koslover, D., Sapin, V., and Benzer, S. (2004). Regulation of lifespan in Drosophila by modulation of genes in the TOR signaling pathway. Curr. Biol. 14, 885–890. doi: 10.1016/j.cub.2004.03.059
Karim, F. D., Guild, G. M., and Thummel, C. S. (1993). The Drosophila broad-complex plays a key role in controlling ecdysone-regulated gene expression at the onset of metamorphosis. Development 118, 977–988.
Karp, X. (2021). Hormonal regulation of diapause and development in nematodes, insects, and fishes. Front. Ecol. Evol. 9:657. doi: 10.3389/fevo.2021.735924
Katewa, S. D., Demontis, F., Kolipinski, M., Hubbard, A., Gill, M. S., Perrimon, N., et al. (2012). Intramyocellular fatty-acid metabolism plays a critical role in mediating responses to dietary restriction in Drosophila melanogaster. Cell Metab. 16, 97–103. doi: 10.1016/j.cmet.2012.06.005
Kayukawa, T., Nagamine, K., Ito, Y., Nishita, Y., Ishikawa, Y., and Shinoda, T. (2016). Krüppel Homolog 1 inhibits insect metamorphosis via direct transcriptional repression of broad-complex, a pupal specifier gene. J. Biol. Chem. 291, 1751–1762. doi: 10.1074/jbc.M115.686121
Kemirembe, K., Liebmann, K., Bootes, A., Smith, W. A., and Suzuki, Y. (2012). Amino acids and TOR signaling promote prothoracic gland growth and the initiation of larval molts in the tobacco hornworm Manduca sexta. PLoS One 7:e44429. doi: 10.1371/journal.pone.0044429
Kim, J., and Neufeld, T. P. (2015). Dietary sugar promotes systemic TOR activation in Drosophila through AKH-dependent selective secretion of Dilp3. Nat. Commun. 6:6846. doi: 10.1038/ncomms7846
Konopova, B., Smykal, V., and Jindra, M. (2011). Common and distinct roles of juvenile hormone signaling genes in metamorphosis of holometabolous and hemimetabolous insects. PLoS One 6:e28728. doi: 10.1371/journal.pone.0028728
Koyama, T., and Mirth, C. K. (2016). Growth-blocking peptides as nutrition-sensitive signals for insulin secretion and body size regulation. PLoS Biol. 14:e1002392. doi: 10.1371/journal.pbio.1002392
Koyama, T., Mendes, C. C., and Mirth, C. K. (2013). Mechanisms regulating nutrition-dependent developmental plasticity through organ-specific effects in insects. Front. Physiol. 4:263. doi: 10.3389/fphys.2013.00263
Kramer, J. M., Davidge, J. T., Lockyer, J. M., and Staveley, B. E. (2003). Expression of Drosophila FOXO regulates growth and can phenocopy starvation. BMC Dev. Biol. 3:5. doi: 10.1186/1471-213X-3-5
Kréneisz, O., Chen, X., Fridell, Y.-W. C., and Mulkey, D. K. (2010). Glucose increases activity and Ca2+ in adult Drosophila Insulin producing cells. Neuroreport 21, 1116–1120. doi: 10.1097/WNR.0b013e3283409200
Kubrak, O. I., Kučerová, L., Theopold, U., and Nässel, D. R. (2014). The sleeping beauty: how reproductive diapause affects hormone signaling, metabolism, immune response and somatic maintenance in Drosophila melanogaster. PLoS One 9:e113051. doi: 10.1371/journal.pone.0113051
Kučerová, L., Kubrak, O. I., Bengtsson, J. M., Strnad, H., Nylin, S., Theopold, U., et al. (2016). Slowed aging during reproductive dormancy is reflected in genome-wide transcriptome changes in Drosophila melanogaster. BMC Genomics 17:50. doi: 10.1186/s12864-016-2383-1
Kuo, T.-H., Yew, J. Y., Fedina, T. Y., Dreisewerd, K., Dierick, H. A., and Pletcher, S. D. (2012). Aging modulates cuticular hydrocarbons and sexual attractiveness in Drosophila melanogaster. J. Exp. Biol. 215, 814–821. doi: 10.1242/jeb.064980
Kyryakov, P., Beach, A., Richard, V., Burstein, M., Leonov, A., Levy, S., et al. (2012). Caloric restriction extends yeast chronological lifespan by altering a pattern of age-related changes in trehalose concentration. Front. Physiol. 3:256. doi: 10.3389/fphys.2012.00256
Lee, C.-Y., Wendel, D. P., Reid, P., Lam, G., Thummel, C. S., and Baehrecke, E. H. (2000). E93 directs steroid-triggered programmed cell death in Drosophila. Mol. Cell 6, 433–443. doi: 10.1016/S1097-2765(00)00042-3
Li, A., and Denlinger, D. L. (2009). Pupal cuticle protein is abundant during early adult diapause in the mosquito Culex pipiens. J. Med. Entomol. 46, 1382–1386. doi: 10.1603/033.046.0618
Li, S., Torre-Muruzabal, T., Søgaard, K. C., Ren, G. R., Hauser, F., Engelsen, S. M., et al. (2013). Expression patterns of the Drosophila neuropeptide CCHamide-2 and its receptor may suggest hormonal signaling from the gut to the brain. PLoS One 8:e76131. doi: 10.1371/journal.pone.0076131
Li, S., Zhang, Q.-R., Xu, W.-H., and Schooley, D. A. (2005). Juvenile hormone diol kinase, a calcium-binding protein with kinase activity, from the silkworm, Bombyx mori. Insect Biochem. Mol. Biol. 35, 1235–1248. doi: 10.1016/j.ibmb.2005.06.005
Li, Y., Zhang, L., Chen, H., Koštál, V., Simek, P., Moos, M., et al. (2015). Shifts in metabolomic profiles of the parasitoid Nasonia vitripennis associated with elevated cold tolerance induced by the parasitoid’s diapause, host diapause and host diet augmented with proline. Insect Biochem. Mol. Biol. 63, 34–46. doi: 10.1016/j.ibmb.2015.05.012
Li, Y.-L., Yao, Y.-X., Zhao, Y.-M., Di, Y.-Q., and Zhao, X.-F. (2021). The steroid hormone 20-hydroxyecdysone counteracts insulin signaling via insulin receptor dephosphorylation. J. Biol. Chem. 296:100318. doi: 10.1016/j.jbc.2021.100318
Liao, S., and Nässel, D. R. (2020). Drosophila insulin-like peptide 8 (dilp8) in ovarian follicle cells regulates ovulation and metabolism. Front. Endocrinol. (Lausanne) 11:461. doi: 10.3389/fendo.2020.00461
Lin, Y. J., Seroude, L., and Benzer, S. (1998). Extended life-span and stress resistance in the Drosophila mutant methuselah. Science 282, 943–946. doi: 10.1126/science.282.5390.943
Ling, L., and Raikhel, A. S. (2018). Serotonin signaling regulates insulin-like peptides for growth, reproduction, and metabolism in the disease vector Aedes aegypti. PNAS 115, E9822–E9831. doi: 10.1073/pnas.1808243115
Ling, L., and Raikhel, A. S. (2021). Cross-talk of insulin-like peptides, juvenile hormone, and 20-hydroxyecdysone in regulation of metabolism in the mosquito Aedes aegypti. PNAS 118: e2023470118. doi: 10.1073/pnas.2023470118
Liu, F., Li, K., Cai, W., Zhao, J., Zou, Y., and Hua, H. (2017). Knockdown of TOR causing ovarian diapause in a genetically stable brachypterous strain of Nilaparvata lugens. Arch. Insect Biochem. Physiol. 95:e21400. doi: 10.1002/arch.21400
Liu, N., Landreh, M., Cao, K., Abe, M., Hendriks, G.-J., Kennerdell, J. R., et al. (2012). The microRNA miR-34 modulates ageing and neurodegeneration in Drosophila. Nature 482, 519–523. doi: 10.1038/nature10810
Liu, Y., Liao, S., Veenstra, J. A., and Nässel, D. R. (2016). Drosophila insulin-like peptide 1 (DILP1) is transiently expressed during non-feeding stages and reproductive dormancy. Sci. Rep. 6:26620. doi: 10.1038/srep26620
Lockey, K. H. (1988). Lipids of the insect cuticle: origin, composition and function. Comp. Biochem. Physiol. B Comp. Biochem. 89, 595–645. doi: 10.1016/0305-0491(88)90305-7
Lourido, F., Quenti, D., Salgado-Canales, D., and Tobar, N. (2021). Domeless receptor loss in fat body tissue reverts insulin resistance induced by a high-sugar diet in Drosophila melanogaster. Sci. Rep. 11:3263. doi: 10.1038/s41598-021-82944-4
Lyu, Z., Li, Z., Cheng, J., Wang, C., Chen, J., and Lin, T. (2019). Suppression of gene juvenile hormone diol kinase delays pupation in Heortia vitessoides Moore. Insects 10:278. doi: 10.3390/insects10090278
Ma, H.-Y., Li, Y.-Y., Li, L., Tan, Y., and Pang, B.-P. (2021). Regulation of juvenile hormone on summer diapause of geleruca daurica and its pathway analysis. Insects 12:237. doi: 10.3390/insects12030237
Maklakov, A. A., and Chapman, T. (2019). Evolution of ageing as a tangle of trade-offs: energy versus function. Proc. R. Soc. B Biol. Sci. 286:20191604. doi: 10.1098/rspb.2019.1604
Manière, G., Alves, G., Berthelot-Grosjean, M., and Grosjean, Y. (2020). Growth regulation by amino acid transporters in Drosophila larvae. Cell. Mol. Life Sci. 77, 4289–4297. doi: 10.1007/s00018-020-03535-6
Manière, G., Ziegler, A. B., Geillon, F., Featherstone, D. E., and Grosjean, Y. (2016). Direct sensing of nutrients via a LAT1-like transporter in Drosophila insulin-producing cells. Cell Rep. 17, 137–148. doi: 10.1016/j.celrep.2016.08.093
Meissner, G. W., Luo, S. D., Dias, B. G., Texada, M. J., and Baker, B. S. (2016). Sex-specific regulation of Lgr3 in Drosophila neurons. PNAS 113, E1256–E1265. doi: 10.1073/pnas.1600241113
Meschi, E., Léopold, P., and Delanoue, R. (2019). An EGF-responsive neural circuit couples insulin secretion with nutrition in Drosophila. Dev. Cell 48, 76.e–86.e. doi: 10.1016/j.devcel.2018.11.029
Min, K.-J., Yamamoto, R., Buch, S., Pankratz, M., and Tatar, M. (2008). Drosophila lifespan control by dietary restriction independent of insulin-like signaling. Aging Cell 7, 199–206. doi: 10.1111/j.1474-9726.2008.00373.x
Minakuchi, C., Namiki, T., Yoshiyama, M., and Shinoda, T. (2008). RNAi-mediated knockdown of juvenile hormone acid O-methyltransferase gene causes precocious metamorphosis in the red flour beetle Tribolium castaneum. FEBS J. 275, 2919–2931. doi: 10.1111/j.1742-4658.2008.06428.x
Mirth, C. K., Tang, H. Y., Makohon-Moore, S. C., Salhadar, S., Gokhale, R. H., Warner, R. D., et al. (2014). Juvenile hormone regulates body size and perturbs insulin signaling in Drosophila. PNAS 111, 7018–7023. doi: 10.1073/pnas.1313058111
Mochanová, M., Tomèala, A., Svobodová, Z., and Kodrík, D. (2018). Role of adipokinetic hormone during starvation in Drosophila. Comp. Biochem. Physiol. B Biochem. Mol. Biol. 226, 26–35. doi: 10.1016/j.cbpb.2018.08.004
Morimoto, A. M., Jordan, K. C., Tietze, K., Britton, J. S., O’Neill, E. M., and Ruohola-Baker, H. (1996). Pointed, an ETS domain transcription factor, negatively regulates the EGF receptor pathway in Drosophila oogenesis. Development 122, 3745–3754.
Moskalev, A. A., Shaposhnikov, M. V., Zemskaya, N. V., Koval, L. À, Schegoleva, E. V., Guvatova, Z. G., et al. (2019). Transcriptome analysis of long-lived drosophila melanogaster e(z) mutants sheds light on the molecular mechanisms of longevity. Sci. Rep. 9:9151. doi: 10.1038/s41598-019-45714-x
Nässel, D. R., and Zandawala, M. (2020). Hormonal axes in Drosophila: regulation of hormone release and multiplicity of actions. Cell Tissue Res. 382, 233–266. doi: 10.1007/s00441-020-03264-z
Nässel, D., Kubrak, O., Liu, Y., Luo, J., and Lushchak, O. (2013). Factors that regulate insulin producing cells and their output in Drosophila. Front. Physiol. 4:252. doi: 10.3389/fphys.2013.00252
Nghiem, D., Gibbs, A. G., Rose, M. R., and Bradley, T. J. (2000). Postponed aging and desiccation resistance in Drosophila melanogaster. Exp. Gerontol. 35, 957–969. doi: 10.1016/S0531-5565(00)00163-7
Niwa, R., and Niwa, Y. S. (2014). Enzymes for ecdysteroid biosynthesis: their biological functions in insects and beyond. Biosci. Biotechnol. Biochem. 78, 1283–1292. doi: 10.1080/09168451.2014.942250
Noh, M. Y., Muthukrishnan, S., Kramer, K. J., and Arakane, Y. (2016). Cuticle formation and pigmentation in beetles. Curr. Opin. Insect Sci. 17, 1–9. doi: 10.1016/j.cois.2016.05.004
O’Neill, E. M., Rebay, I., Tjian, R., and Rubin, G. M. (1994). The activities of two Ets-related transcription factors required for drosophila eye development are modulated by the Ras/MAPK pathway. Cell 78, 137–147. doi: 10.1016/0092-8674(94)90580-0
Ohtake, S., and Wang, Y. J. (2011). Trehalose: current use and future applications. J. Pharm. Sci. 100, 2020–2053. doi: 10.1002/jps.22458
Ojima, N., Hara, Y., Ito, H., and Yamamoto, D. (2018). Genetic dissection of stress-induced reproductive arrest in Drosophila melanogaster females. PLoS Genetics 14:e1007434. doi: 10.1371/journal.pgen.1007434
Okamoto, N., and Nishimura, T. (2015). Signaling from glia and cholinergic neurons controls nutrient-dependent production of an insulin-like peptide for Drosophila body growth. Dev. Cell 35, 295–310. doi: 10.1016/j.devcel.2015.10.003
Okamoto, N., Nakamori, R., Murai, T., Yamauchi, Y., Masuda, A., and Nishimura, T. (2013). A secreted decoy of InR antagonizes insulin/IGF signaling to restrict body growth in Drosophila. Genes Dev. 27, 87–97. doi: 10.1101/gad.204479.112
Okamoto, N., Viswanatha, R., Bittar, R., Li, Z., Haga-Yamanaka, S., Perrimon, N., et al. (2018). A membrane transporter is required for steroid hormone uptake in Drosophila. Dev. Cell 47, 294.e–305.e. doi: 10.1016/j.devcel.2018.09.012
Okamoto, N., Yamanaka, N., Satake, H., Saegusa, H., Kataoka, H., and Mizoguchi, A. (2009). An ecdysteroid-inducible insulin-like growth factor-like peptide regulates adult development of the silkmoth Bombyx mori. FEBS J. 276, 1221–1232. doi: 10.1111/j.1742-4658.2008.06859.x
Ono, H. (2014). Ecdysone differentially regulates metamorphic timing relative to 20-hydroxyecdysone by antagonizing juvenile hormone in Drosophila melanogaster. Dev. Biol. 391, 32–42. doi: 10.1016/j.ydbio.2014.04.004
Ostojic, I., Boll, W., Waterson, M. J., Chan, T., Chandra, R., Pletcher, S. D., et al. (2014). Positive and negative gustatory inputs affect Drosophila lifespan partly in parallel to dFOXO signaling. Proc. Natl. Acad. Sci. U.S.A. 111, 8143–8148. doi: 10.1073/pnas.1315466111
Oudes, A. J., Herr, C. M., Olsen, Y., and Fleming, J. E. (1998). Age-dependent accumulation of advanced glycation end-products in adult Drosophila melanogaster. Mech. Ageing Dev. 100, 221–229. doi: 10.1016/s0047-6374(97)00146-2
Pan, X., and O’Connor, M. B. (2021). Coordination among multiple receptor tyrosine kinase signals controls Drosophila developmental timing and body size. Cell Rep. 36:109644. doi: 10.1016/j.celrep.2021.109644
Park, S., Alfa, R. W., Topper, S. M., Kim, G. E. S., Kockel, L., and Kim, S. K. (2014). A genetic strategy to measure circulating Drosophila insulin reveals genes regulating insulin production and secretion. PLoS Genet. 10:e1004555. doi: 10.1371/journal.pgen.1004555
Parkhitko, A. A., Ramesh, D., Wang, L., Leshchiner, D., Filine, E., Binari, R., et al. (2020). Downregulation of the tyrosine degradation pathway extends Drosophila lifespan. ELife 9:e58053. doi: 10.7554/eLife.58053
Petryk, A., Warren, J. T., Marqués, G., Jarcho, M. P., Gilbert, L. I., Kahler, J., et al. (2003). Shade is the Drosophila P450 enzyme that mediates the hydroxylation of ecdysone to the steroid insect molting hormone 20-hydroxyecdysone. Proc. Natl. Acad. Sci. U.S.A. 100, 13773–13778. doi: 10.1073/pnas.2336088100
Poelchau, M. F., Reynolds, J. A., Elsik, C. G., Denlinger, D. L., and Armbruster, P. A. (2013). RNA-Seq reveals early distinctions and late convergence of gene expression between diapause and quiescence in the Asian tiger mosquito, Aedes albopictus. J. Exp. Biol. 216, 4082–4090. doi: 10.1242/jeb.089508
Post, S., and Tatar, M. (2016). Nutritional geometric profiles of Insulin/IGF expression in Drosophila melanogaster. PLoS One 11:e0155628. doi: 10.1371/journal.pone.0155628
Post, S., Karashchuk, G., Wade, J. D., Sajid, W., De Meyts, P., and Tatar, M. (2018). Drosophila Insulin-Like Peptides DILP2 and DILP5 differentially stimulate cell signaling and glycogen phosphorylase to regulate longevity. Front. Endocrinol. 9:245. doi: 10.3389/fendo.2018.00245
Post, S., Liao, S., Yamamoto, R., Veenstra, J. A., Nässel, D. R., and Tatar, M. (2019). Drosophila insulin-like peptide dilp1 increases lifespan and glucagon-like Akh expression epistatic to dilp2. Aging Cell 18:e12863. doi: 10.1111/acel.12863
Prince, E., Kretzschmar, J., Trautenberg, L. C., Broschk, S., and Brankatschk, M. (2021). DIlp7-Producing neurons regulate insulin-producing cells in Drosophila. Front. Physiol. 12:1159. doi: 10.3389/fphys.2021.630390
Ptak, G. E., Tacconi, E., Czernik, M., Toschi, P., Modlinski, J. A., and Loi, P. (2012). Embryonic diapause is conserved across mammals. PLoS One 7:e33027. doi: 10.1371/journal.pone.0033027
Rajan, A., and Perrimon, N. (2010). Steroids make you bigger? Fat chance says myc. Cell Metab. 2, 7–9. doi: 10.1016/j.cmet.2010.06.003
Rajan, A., and Perrimon, N. (2012). Drosophila cytokine unpaired 2 regulates physiological homeostasis by remotely controlling insulin secretion. Cell 151, 123–137. doi: 10.1016/j.cell.2012.08.019
Rasweiler, J., and Badwaik, N. (2020). Embryonic diapause in the short-tailed fruit bat, Carollia perspicillata: why this is postimplantational. Biosci. Proc. 10:8. doi: 10.1530/biosciprocs.10.008
Rauschenbach, I. Yu, Sukhanova, M. Zh, Hirashima, A., Sutsugu, E., and Kuano, E. (2000). Role of the ecdysteroid system in the regulation of drosophila reproduction under environmental stress. Dokl. Biol. Sci. 375, 641–643. doi: 10.1023/A:1026610425973
Ray, M., and Lakhotia, S. C. (2017). High JNK following Ras/Rpr/Tak1 over-expression in eye discs of Drosophila reduces post-pupariation ecdysone via Dilp8 causing early pupal death. bioRxiv [Preprint]. doi: 10.1101/049882
Ren, G. R., Hauser, F., Rewitz, K. F., Kondo, S., Engelbrecht, A. F., Didriksen, A. K., et al. (2015). CCHamide-2 is an orexigenic brain-gut peptide in Drosophila. PLoS One 10:e0133017. doi: 10.1371/journal.pone.0133017
Rewitz, K. F., Yamanaka, N., Gilbert, L. I., and O’Connor, M. B. (2009). The insect neuropeptide PTTH activates receptor tyrosine kinase torso to initiate metamorphosis. Science 326, 1403–1405. doi: 10.1126/science.1176450
Reyes-DelaTorre, A., Peña-Rangel, M. T., and Riesgo-Escovar, J. R. (2012). “Carbohydrate metabolism in Drosophila: reliance on the disaccharide trehalose,” in Carbohydrates-Comprehensive Studies on Glycobiology and Glycotechnology, ed. C. Chang (London: IntechOpen). doi: 10.5772/50633
Reynolds, J. A., Peyton, J. T., and Denlinger, D. L. (2017). Changes in microRNA abundance may regulate diapause in the flesh fly, Sarcophaga bullata. Insect Biochem. Mol. Biol. 84, 1–14. doi: 10.1016/j.ibmb.2017.03.002
Rinehart, J. P., Cikra-Ireland, R. A., Flannagan, R. D., and Denlinger, D. L. (2001). Expression of ecdysone receptor is unaffected by pupal diapause in the flesh fly, Sarcophaga crassipalpis, while its dimerization partner, USP, is downregulated. J. Insect Physiol. 47, 915–921. doi: 10.1016/S0022-1910(01)00064-6
Rulifson, E. J., Kim, S. K., and Nusse, R. (2002). Ablation of insulin-producing neurons in flies: growth and diabetic phenotypes. Science 296, 1118–1120. doi: 10.1126/science.1070058
Rus, F., Flatt, T., Tong, M., Aggarwal, K., Okuda, K., Kleino, A., et al. (2013). Ecdysone triggered PGRP-LC expression controls Drosophila innate immunity. EMBO J. 32, 1626–1638. doi: 10.1038/emboj.2013.100
Rusmini, P., Cortese, K., Crippa, V., Cristofani, R., Cicardi, M. E., Ferrari, V., et al. (2018). Trehalose induces autophagy via lysosomal-mediated TFEB activation in models of motoneuron degeneration. Autophagy 15, 631–651. doi: 10.1080/15548627.2018.1535292
Russell, S., and Ashburner, M. (1996). “3 - Ecdysone-Regulated chromosome puffing in drosophila melanogaster,” in Metamorphosis Cell Biology, eds L. I. Gilbert, J. R. Tata, and B. G. Atkinson (San Diego, CA: Academic Press), 109–144. doi: 10.1016/B978-012283245-1/50005-1
Rusten, T. E., Lindmo, K., Juhász, G., Sass, M., Seglen, P. O., Brech, A., et al. (2004). Programmed autophagy in the Drosophila fat body is induced by ecdysone through regulation of the PI3K pathway. Dev. Cell 7, 179–192. doi: 10.1016/j.devcel.2004.07.005
Ruzzi, L. R., Schilman, P. E., San Martin, A., Lew, S. E., Gelb, B. D., and Pagani, M. R. (2020). The phosphatase CSW controls life span by insulin signaling and metabolism throughout adult life in Drosophila. Front. Genetics 11:364. doi: 10.3389/fgene.2020.00364
Sanchez, J. A., Mesquita, D., Ingaramo, M. C., Ariel, F., Milán, M., and Dekanty, A. (2019). Eiger/TNFα-mediated Dilp8 and ROS production coordinate intra-organ growth in Drosophila. PLoS Genet. 15:e1008133. doi: 10.1371/journal.pgen.1008133
Sangüesa, G., Roglans, N., Baena, M., Velázquez, A. M., Laguna, J. C., and Alegret, M. (2019). mTOR is a key protein involved in the metabolic effects of simple sugars. Int. J. Mol. Sci. 20:1117. doi: 10.3390/ijms20051117
Santos, P. K. F., de Souza Araujo, N., Françoso, E., Zuntini, A. R., and Arias, M. C. (2018). Diapause in a tropical oil-collecting bee: molecular basis unveiled by RNA-Seq. BMC Genomics 19:305. doi: 10.1186/s12864-018-4694-x
Saravanakumar, R., Ponnuvel, K. M., and Qadri, S. M. H. (2010). Genetic stability analysis of diapause-induced multivoltine silkworm Bombyx mori germplasm using inter simple sequence repeat markers. Entomol. Exp. Appl. 135, 170–176. doi: 10.1111/j.1570-7458.2010.00978.x
Schiesari, L., Andreatta, G., Kyriacou, C. P., O’Connor, M. B., and Costa, R. (2016). The insulin-like proteins dILPs-2/5 determine diapause inducibility in Drosophila. PLoS One 11:e0163680. doi: 10.1371/journal.pone.0163680
Semaniuk, U., Strilbytska, O., Malinovska, K., Storey, K. B., Vaiserman, A., Lushchak, V., et al. (2021). Factors that regulate expression patterns of insulin-like peptides and their association with physiological and metabolic traits in Drosophila. Insect Biochem. Mol. Biol. 135:103609. doi: 10.1016/j.ibmb.2021.103609
Semba, R. D., Nicklett, E. J., and Ferrucci, L. (2010). Does accumulation of advanced glycation end products contribute to the aging phenotype? J. Gerontol. A 65A, 963–975. doi: 10.1093/gerona/glq074
Seo, Y., Kingsley, S., Walker, G., Mondoux, M. A., and Tissenbaum, H. A. (2018). Metabolic shift from glycogen to trehalose promotes lifespan and healthspan in Caenorhabditis elegans. Proc. Natl. Acad. Sci. U.S.A. 115, E2791–E2800. doi: 10.1073/pnas.1714178115
Shaaya, E., and Pisarev, V. (1986). The lethal effects of three insect juvenile hormone analogues on the developmental stages of Ephestia cautella (Wlk.) (Lepidoptera: Phycitidae). J. Stored Prod. Res. 22, 125–129. doi: 10.1016/0022-474X(86)90004-4
Shamim, G., Ranjan, S. K., Pandey, D. M., and Ramani, R. (2014). Biochemistry and biosynthesis of insect pigments. EJE 111, 149–164. doi: 10.14411/eje.2014.021
Shaul, Y. D., and Seger, R. (2007). The MEK/ERK cascade: from signaling specificity to diverse functions. Biochim. Biophys. Acta 1773, 1213–1226. doi: 10.1016/j.bbamcr.2006.10.005
Sheng, Z., Xu, J., Bai, H., Zhu, F., and Palli, S. R. (2011). Juvenile hormone regulates vitellogenin gene expression through insulin-like peptide signaling pathway in the red flour beetle, Tribolium castaneum. J. Biol. Chem. 286, 41924–41936. doi: 10.1074/jbc.M111.269845
Shimobayashi, M., and Hall, M. N. (2016). Multiple amino acid sensing inputs to mTORC1. Cell Res. 26, 7–20. doi: 10.1038/cr.2015.146
Shin, S. W., Zou, Z., Saha, T. T., and Raikhel, A. S. (2012). BHLH-PAS heterodimer of methoprene-tolerant and Cycle mediates circadian expression of juvenile hormone-induced mosquito genes. PNAS 109, 16576–16581. doi: 10.1073/pnas.1214209109
Shingleton, A. W., Das, J., Vinicius, L., and Stern, D. L. (2005). The temporal requirements for insulin signaling during development in Drosophila. PLoS Biol. 3:e289. doi: 10.1371/journal.pbio.0030289
Siddle, K. (2012). Molecular basis of signaling specificity of insulin and IGF receptors: neglected corners and recent advances. Front. Endocrinol. 3:34. doi: 10.3389/fendo.2012.00034
Sim, C., and Denlinger, D. L. (2008). Insulin signaling and FOXO regulate the overwintering diapause of the mosquito Culex pipiens. Proc. Natl. Acad. Sci. U.S.A. 105, 6777–6781. doi: 10.1073/pnas.0802067105
Sim, C., and Denlinger, D. L. (2013). Insulin signaling and the regulation of insect diapause. Front. Physiol. 4:189. doi: 10.3389/fphys.2013.00189
Simon, A. F., Shih, C., Mack, A., and Benzer, S. (2003). Steroid control of longevity in Drosophila melanogaster. Science 299, 1407–1410. doi: 10.1126/science.1080539
Sinclair, B. J., and Marshall, K. E. (2018). The many roles of fats in overwintering insects. J. Exp. Biol. 221:jeb161836. doi: 10.1242/jeb.161836
Slaidina, M., Delanoue, R., Gronke, S., Partridge, L., and Léopold, P. (2009). A Drosophila insulin-like peptide promotes growth during nonfeeding states. Dev. Cell 17, 874–884. doi: 10.1016/j.devcel.2009.10.009
Sloth Andersen, A., Hertz Hansen, P., Schäffer, L., and Kristensen, C. (2000). A new secreted insect protein belonging to the immunoglobulin superfamily binds insulin and related peptides and inhibits their activities. J. Biol. Chem. 275, 16948–16953. doi: 10.1074/jbc.M001578200
Smykal, V., Daimon, T., Kayukawa, T., Takaki, K., Shinoda, T., and Jindra, M. (2014). Importance of juvenile hormone signaling arises with competence of insect larvae to metamorphose. Dev. Biol. 390, 221–230. doi: 10.1016/j.ydbio.2014.03.006
Söderberg, J. A. E., Birse, R. T., and Nässel, D. R. (2011). Insulin production and signaling in renal tubules of Drosophila is under control of tachykinin-related peptide and regulates stress resistance. PLoS One 6:e19866. doi: 10.1371/journal.pone.0019866
Solano, F. (2014). Melanins: Skin Pigments and Much More—Types, Structural Models, Biological Functions, and Formation Routes. Hoboken, NJ: Wiley. doi: 10.1155/2014/498276
Spielman, A., and Williams, C. M. (1966). Lethal effects of synthetic juvenile hormone on larvae of the yellow fever mosquito, Aedes aegypti. Science 154, 1043–1044. doi: 10.1126/science.154.3752.1043
Stefanatos, R., Sriram, A., Kiviranta, E., Mohan, A., Ayala, V., Jacobs, H. T., et al. (2012). Dj-1β regulates oxidative stress, insulin-like signaling and development in Drosophila melanogaster. Cell Cycle 11, 3876–3886. doi: 10.4161/cc.22073
Stocker, A. J., and Pavan, C. (1977). Developmental puffing patterns in salivary gland chromosomes of Rhynchosciara hollaenderi. Chromosoma 62, 17–47. doi: 10.1007/BF00328438
Suo, S., Culotti, J. G., and Tol, H. H. M. V. (2009). Dopamine suppresses octopamine signaling in C. elegans: possible involvement of dopamine in the regulation of lifespan. Aging 1, 870–874. doi: 10.18632/aging.100097
Suzawa, M., Muhammad, N. M., Joseph, B. S., and Bland, M. L. (2019). The toll signaling pathway targets the insulin-like peptide Dilp6 to inhibit growth in Drosophila. Cell Rep. 28, 1439.e–1446.e. doi: 10.1016/j.celrep.2019.07.015
Tan, A., Tanaka, H., Tamura, T., and Shiotsuki, T. (2005). Precocious metamorphosis in transgenic silkworms overexpressing juvenile hormone esterase. PNAS 102, 11751–11756. doi: 10.1073/pnas.0500954102
Tanabe, K., Itoh, M., and Tonoki, A. (2017). Age-Related changes in insulin-like signaling lead to intermediate-term memory impairment in Drosophila. Cell Rep. 18, 1598–1605. doi: 10.1016/j.celrep.2017.01.053
Tanaka, Y. (2013). The different effects of ecdysone and 20-hydroxyecdysone on the induction of larval ecdysis in the silkworm, Bombyx mori (Lepidoptera: Bombycidae). EJE 92, 155–160.
Tapia, H., Young, L., Fox, D., Bertozzi, C. R., and Koshland, D. (2015). Increasing intracellular trehalose is sufficient to confer desiccation tolerance to Saccharomyces cerevisiae. Proc. Natl. Acad. Sci. U.S.A. 112, 6122–6127. doi: 10.1073/pnas.1506415112
Tatar, M., Kopelman, A., Epstein, D., Tu, M.-P., Yin, C.-M., and Garofalo, R. S. (2001). A mutant Drosophila insulin receptor homolog that extends life-span and impairs neuroendocrine function. Science 292, 107–110. doi: 10.1126/science.1057987
Teets, N. M., and Denlinger, D. L. (2013). Physiological mechanisms of seasonal and rapid cold-hardening in insects. Physiol. Entomol. 38, 105–116. doi: 10.1111/phen.12019
Teleman, A. A. (2009). Molecular mechanisms of metabolic regulation by insulin in Drosophila. Biochem. J. 425, 13–26. doi: 10.1042/BJ20091181
Terashima, J., Takaki, K., Sakurai, S., and Bownes, M. (2005). Nutritional status affects 20-hydroxyecdysone concentration and progression of oogenesis in Drosophila melanogaster. J. Endocrinol. 187, 69–79. doi: 10.1677/joe.1.06220
Tian, Z., Guo, S., Li, J.-X., Zhu, F., Liu, W., and Wang, X.-P. (2021). Juvenile hormone biosynthetic genes are critical for regulating reproductive diapause in the cabbage beetle. Insect Biochem. Mol. Biol. 139:103654. doi: 10.1016/j.ibmb.2021.103654
Toprak, U. (2020). The role of peptide hormones in insect lipid metabolism. Front. Physiol. 11:434. doi: 10.3389/fphys.2020.00434
Traverse, S., Seedorf, K., Paterson, H., Marshall, C. J., Cohen, P., and Ullrich, A. (1994). EGF triggers neuronal differentiation of PC12 cells that overexpress the EGF receptor. Curr. Biol. 4, 694–701. doi: 10.1016/S0960-9822(00)00154-8
Truman, J. W., and Riddiford, L. M. (2007). The morphostatic actions of juvenile hormone. Insect Biochem. Mol. Biol. 37, 761–770. doi: 10.1016/j.ibmb.2007.05.011
Tsai, C.-C., Kao, H.-Y., Yao, T.-P., McKeown, M., and Evans, R. M. (1999). SMRTER, a Drosophila nuclear receptor coregulator. Reveals that EcR-mediated repression is critical for development. Mol. Cell 4, 175–186. doi: 10.1016/S1097-2765(00)80365-2
Tsuchiya, R., Kaneshima, A., Kobayashi, M., Yamazaki, M., Takasu, Y., Sezutsu, H., et al. (2021). Maternal GABAergic and GnRH/corazonin pathway modulates egg diapause phenotype of the silkworm Bombyx mori. Proc. Natl. Acad. Sci. U.S.A. 118:e2020028118. doi: 10.1073/pnas.2020028118
Tsun, Z.-Y., Bar-Peled, L., Chantranupong, L., Zoncu, R., Wang, T., Kim, C., et al. (2013). The folliculin tumor suppressor is a GAP for the RagC/D GTPases that signal amino acid levels to mTORC1. Mol. Cell 52, 495–505. doi: 10.1016/j.molcel.2013.09.016
Tu, M.-P., Yin, C.-M., and Tatar, M. (2005). Mutations in insulin signaling pathway alter juvenile hormone synthesis in Drosophila melanogaster. Gen. Comp. Endocrinol. 142, 347–356. doi: 10.1016/j.ygcen.2005.02.009
Uchibori-Asano, M., Kayukawa, T., Sezutsu, H., Shinoda, T., and Daimon, T. (2017). Severe developmental timing defects in the prothoracicotropic hormone (PTTH)-deficient silkworm, Bombyx mori. Insect Biochem. Mol. Biol. 87, 14–25. doi: 10.1016/j.ibmb.2017.06.007
Uyehara, C. M., and McKay, D. J. (2019). Direct and widespread role for the nuclear receptor EcR in mediating the response to ecdysone in Drosophila. PNAS 116, 9893–9902. doi: 10.1073/pnas.1900343116
Vallejo, D. M., Juarez-Carreño, S., Bolivar, J., Morante, J., and Dominguez, M. (2015). A brain circuit that synchronizes growth and maturation revealed through Dilp8 binding to Lgr3. Science 350:aac6767. doi: 10.1126/science.aac6767
Varghese, J., Lim, S. F., and Cohen, S. M. (2010). Drosophila miR-14 regulates insulin production and metabolism through its target, sugarbabe. Genes Dev. 24, 2748–2753. doi: 10.1101/gad.1995910
Veenstra, J. A., Agricola, H.-J., and Sellami, A. (2008). Regulatory peptides in fruit fly midgut. Cell Tissue Res. 334, 499–516. doi: 10.1007/s00441-008-0708-3
Vijg, J., and Suh, Y. (2013). Genome instability and aging. Annu. Rev. Physiol. 75, 645–668. doi: 10.1146/annurev-physiol-030212-183715
Walkiewicz, M. A., and Stern, M. (2009). Increased insulin/insulin growth factor signaling advances the onset of metamorphosis in Drosophila. PLoS One 4:e5072. doi: 10.1371/journal.pone.0005072
Waterson, M. J., Chung, B. Y., Harvanek, Z. M., Ostojic, I., Alcedo, J., and Pletcher, S. D. (2014). Water sensor ppk28 modulates Drosophila lifespan and physiology through AKH signaling. PNAS 111, 8137–8142. doi: 10.1073/pnas.1315461111
Werz, C., Köhler, K., Hafen, E., and Stocker, H. (2009). The Drosophila SH2B family adaptor Lnk acts in parallel to chico in the insulin signaling pathway. PLoS Genet. 5:e1000596. doi: 10.1371/journal.pgen.1000596
Wigby, S., Slack, C., Grönke, S., Martinez, P., Calboli, F. C. F., Chapman, T., et al. (2011). Insulin signalling regulates remating in female Drosophila. Proc. R. Soc. B Biol. Sci. 278, 424–431. doi: 10.1098/rspb.2010.1390
Wyatt, G. R., and Davey, K. G. (1996). “Cellular and molecular actions of juvenile hormone. II. roles of juvenile hormone in adult insects,” in Advances in Insect Physiology, ed. P. D. Evans (Cambridge, MA: Academic Press), 1–155. doi: 10.1016/S0065-2806(08)60030-2
Xu, J., Sheng, Z., and Palli, S. R. (2013). Juvenile hormone and insulin regulate trehalose homeostasis in the red flour beetle, Tribolium castaneum. PLoS Genet. 9:e1003535. doi: 10.1371/journal.pgen.1003535
Yamada, T., Habara, O., Kubo, H., and Nishimura, T. (2018). Fat body glycogen serves as a metabolic safeguard for the maintenance of sugar levels in Drosophila. Development 145:dev158865. doi: 10.1242/dev.158865
Yamamoto, R., Bai, H., Dolezal, A. G., Amdam, G., and Tatar, M. (2013). Juvenile hormone regulation of Drosophila aging. BMC Biol. 11:85. doi: 10.1186/1741-7007-11-85
Yamamoto, R., Palmer, M., Koski, H., Curtis-Joseph, N., and Tatar, M. (2021). Aging modulated by the Drosophila insulin receptor through distinct structure-defined mechanisms. Genetics 217:iyaa037. doi: 10.1093/genetics/iyaa037
Yamanaka, N., Rewitz, K. F., and O’Connor, M. B. (2013). Ecdysone control of developmental transitions: lessons from Drosophila research. Annu. Rev. Entomol. 58, 497–516. doi: 10.1146/annurev-ento-120811-153608
Yang, Y., Zhao, T., Li, Z., Qian, W., Peng, J., Wei, L., et al. (2021). Histone H3K27 methylation–mediated repression of Hairy regulates insect developmental transition by modulating ecdysone biosynthesis. PNAS 118:e2101442118. doi: 10.1073/pnas.2101442118
Yao, T.-P., Forman, B. M., Jiang, Z., Cherbas, L., Chen, J.-D., McKeown, M., et al. (1993). Functional ecdysone receptor is the product of EcR and Ultraspiracle genes. Nature 366, 476–479. doi: 10.1038/366476a0
Yao, T.-P., Segraves, W. A., Oro, A. E., McKeown, M., and Evans, R. M. (1992). Drosophila ultraspiracle modulates ecdysone receptor function via heterodimer formation. Cell 71, 63–72. doi: 10.1016/0092-8674(92)90266-F
Yenush, L., Fernandez, R., Myers, M. G., Grammer, T. C., Sun, X. J., Blenis, J., et al. (1996). The Drosophila insulin receptor activates multiple signaling pathways but requires insulin receptor substrate proteins for DNA synthesis. Mol. Cell. Biol. 16, 2509–2517. doi: 10.1128/MCB.16.5.2509
Yin, C.-M., and Chippendale, G. M. (1973). Juvenile hormone regulation of the larval diapause of the Southwestern corn borer, Diatraea grandiosella. J. Insect Physiol. 19, 2403–2420. doi: 10.1016/0022-1910(73)90244-8
Yocum, G. D., Rinehart, J. P., Horvath, D. P., Kemp, W. P., Bosch, J., Alroobi, R., et al. (2015). Key molecular processes of the diapause to post-diapause quiescence transition in the alfalfa leafcutting bee Megachile rotundata identified by comparative transcriptome analysis. Physiol. Entomol. 40, 103–112. doi: 10.1111/phen.12093
Yu, R., Cao, X., Sun, L., Zhu, J.-Y., Wasko, B. M., Liu, W., et al. (2021). Inactivating histone deacetylase HDA promotes longevity by mobilizing trehalose metabolism. Nat. Commun. 12:1981. doi: 10.1038/s41467-021-22257-2
Zhang, W., Liang, G., Ma, L., Jiang, T., and Xiao, H. (2019). Dissecting the Role of Juvenile Hormone Binding Protein in Response to Hormone and Starvation in the Cotton Bollworm, Helicoverpa armigera (Hübner) (Lepidoptera: Noctuidae). J. Econ. Entomol. 112, 1411–1417. doi: 10.1093/jee/toz027
Zhang, W., Thompson, B. J., Hietakangas, V., and Cohen, S. M. (2011). MAPK/ERK signaling regulates insulin sensitivity to control glucose metabolism in Drosophila. PLoS Genetics 7:e1002429. doi: 10.1371/journal.pgen.1002429
Zhao, W.-L., Liu, C.-Y., Liu, W., Wang, D., Wang, J.-X., and Zhao, X.-F. (2014). Methoprene-tolerant 1 regulates gene transcription to maintain insect larval status. J. Mol. Endocrinol. 53, 93–104. doi: 10.1530/JME-14-0019
Zhao, X., and Karpac, J. (2017). Muscle directs diurnal energy homeostasis through a myokine-dependent hormone module in Drosophila. Curr. Biol. 27, 1941.e–1955.e. doi: 10.1016/j.cub.2017.06.004
Zheng, W., Ocorr, K., and Tatar, M. (2020). Extracellular matrix induced by steroids and aging through a G-protein-coupled receptor in a Drosophila model of renal fibrosis. Dis. Model. Mech. 13:dmm041301. doi: 10.1242/dmm.041301
Zheng, W., Rus, F., Hernandez, A., Kang, P., Goldman, W., Silverman, N., et al. (2018). Dehydration triggers ecdysone-mediated recognition-protein priming and elevated anti-bacterial immune responses in Drosophila Malpighian tubule renal cells. BMC Biol. 16:60. doi: 10.1186/s12915-018-0532-5
Zhu, J., Busche, J. M., and Zhang, X. (2010). Identification of juvenile hormone target genes in the adult female mosquitoes. Insect Biochem. Mol. Biol. 40, 23–29. doi: 10.1016/j.ibmb.2009.12.004
Zhu, J., Chen, L., and Raikhel, A. S. (2003). Posttranscriptional control of the competence factor βFTZ-F1 by juvenile hormone in the mosquito Aedes aegypti. PNAS 100, 13338–13343. doi: 10.1073/pnas.2234416100
Ziegler, A. B., Manière, G., and Grosjean, Y. (2018). JhI-21 plays a role in Drosophila insulin-like peptide release from larval IPCs via leucine transport. Sci. Rep. 8:1908. doi: 10.1038/s41598-018-20394-1
Keywords: diapause, endocrine, hormone, lifespan, aging, longevity, ecdysone, insulin
Citation: Hutfilz C (2022) Endocrine Regulation of Lifespan in Insect Diapause. Front. Physiol. 13:825057. doi: 10.3389/fphys.2022.825057
Received: 29 November 2021; Accepted: 25 January 2022;
Published: 15 February 2022.
Edited by:
Julie A. Reynolds, The Ohio State University, United StatesReviewed by:
Umut Toprak, Ankara University, TurkeyYuyan Li, Institute of Plant Protection, Chinese Academy of Agricultural Sciences (CAAS), China
Copyright © 2022 Hutfilz. This is an open-access article distributed under the terms of the Creative Commons Attribution License (CC BY). The use, distribution or reproduction in other forums is permitted, provided the original author(s) and the copyright owner(s) are credited and that the original publication in this journal is cited, in accordance with accepted academic practice. No use, distribution or reproduction is permitted which does not comply with these terms.
*Correspondence: Corinne Hutfilz, Y29yaW5uZV9odXRmaWx6QGJyb3duLmVkdQ==