- 1CNRS, INSERM, Institute for Research on Cancer and Aging, Nice, Université Côte d’Azur, Nice, France
- 2Institut Fédératif de Recherche – Ressources Marines (MARRES), Université Côte d’Azur, Nice, France
- 3UFR 927, Sorbonne Université, Paris, France
The cnidarian-dinoflagellate symbiosis is a mutualistic intracellular association based on the photosynthetic activity of the endosymbiont. This relationship involves significant constraints and requires co-evolution processes, such as an extensive capacity of the holobiont to counteract pro-oxidative conditions induced by hyperoxia generated during photosynthesis. In this study, we analyzed the capacity of Anemonia viridis cells to deal with pro-oxidative conditions by in vivo and in vitro approaches. Whole specimens and animal primary cell cultures were submitted to 200 and 500 μM of H2O2 during 7 days. Then, we monitored global health parameters (symbiotic state, viability, and cell growth) and stress biomarkers (global antioxidant capacity, oxidative protein damages, and protein ubiquitination). In animal primary cell cultures, the intracellular reactive oxygen species (ROS) levels were also evaluated under H2O2 treatments. At the whole organism scale, both H2O2 concentrations didn’t affect the survival and animal tissues exhibited a high resistance to H2O2 treatments. Moreover, no bleaching has been observed, even at high H2O2 concentration and after long exposure (7 days). Although, the community has suggested the role of ROS as the cause of bleaching, our results indicating the absence of bleaching under high H2O2 concentration may exculpate this specific ROS from being involved in the molecular processes inducing bleaching. However, counterintuitively, the symbiont compartment appeared sensitive to an H2O2 burst as it displayed oxidative protein damages, despite an enhancement of antioxidant capacity. The in vitro assays allowed highlighting an intrinsic high capacity of isolated animal cells to deal with pro-oxidative conditions, although we observed differences on tolerance between H2O2 treatments. The 200 μM H2O2 concentration appeared to correspond to the tolerance threshold of animal cells. Indeed, no disequilibrium on redox state was observed and only a cell growth decrease was measured. Contrarily, the 500 μM H2O2 concentration induced a stress state, characterized by a cell viability decrease from 1 day and a drastic cell growth arrest after 7 days leading to an uncomplete recovery after treatment. In conclusion, this study highlights the overall high capacity of cnidarian cells to cope with H2O2 and opens new perspective to investigate the molecular mechanisms involved in this peculiar resistance.
Introduction
The evolutionary success of symbiotic cnidarians is based on a mutualism with dinoflagellates of the family Symbiodiniaceae. The symbionts, living inside the gastrodermal host cells, find a protected and stable environment and benefit from inorganic compounds provided by the animal cells (e.g., nitrogen, phosphorus, and sulfate) for their photosynthetic activity. Conversely, the animal host benefits from the organic compounds produced by algal photosynthesis (e.g., glucose and subsequently amino-acids, lipids) and largely transferred from the alga to the animal cell (Davy et al., 2012). This partner cooperation allows autotrophy to the animal host, leading to the colonization of oligotrophic waters by the symbiotic holobiont.
Concomitantly with those advantages, some constraints appear, especially the photosynthetic-dependent production of oxygen in the animal tissue. Such oxygen production causes diurnal hyperoxia condition in a symbiotic cnidarian, leading to a pro-oxidant state with reactive oxygen species (ROS) overproduction (Dykens et al., 1992; Richier et al., 2003; Saragosti et al., 2010; Shaked and Armoza-Zvuloni, 2013). Both partners have the pathways for cross-regulating the intracellular redox state, especially by ROS detoxication through a full suite of antioxidant enzymes to avoid cellular damages (Shick and Dykens, 1985; Richier et al., 2003, 2005; Plantivaux et al., 2004; Furla et al., 2005; Merle et al., 2007; Pey et al., 2017).
The study of ROS sensitivity in these organisms is also of environmental interest. Environmental perturbations (especially variations in temperature and UV radiation) induce oxidative stress that may lead in extreme cases to symbiosis breakdown, a process commonly called bleaching. Thus, under stressful oxidative conditions, Symbiodiniaceae can be eliminated from or exit the host through different cellular processes, like exocytosis, cell detachment, necrosis or apoptosis (see for review Suggett and Smith, 2020). Oxidative stress is known to induce specific cellular damages such as DNA modification (DNA adducts), lipid peroxidation and protein oxidation. In symbiotic cnidarians, several biochemical biomarkers (e.g., protein carbonylation, lipid peroxidation, and protein ubiquitination) were validated in studies following imbalances between ROS overproduction and antioxidant defenses during environmental stress, resulting in the disruption of the symbiotic association (e.g., Lesser and Farrell, 2004; Richier et al., 2006; Pey et al., 2011).
Among ROS, hydrogen peroxide (H2O2) is a relatively stable chemical formed from O2 and is naturally present in the aquatic systems (Ndungu et al., 2019) with concentrations ranging from 0.3 μM in the water column to 4 μM in intertidal areas (Abele-Oeschger et al., 1997). It originates from marine biota (Hansel and Diaz, 2021) or is carried by the rain (Cooper et al., 1987). In all the organisms, intracellular H2O2 levels can reach tens of micromolar and is generated during normal cellular metabolism (i.e., photosynthesis and respiration) playing crucial roles in the intracellular signaling such as hypoxic signal transduction, cell differentiation and proliferation as well as for immune responses (Halliwell et al., 2000; Apel and Hirt, 2004; Giorgio et al., 2007). At high production levels, the H2O2 effect can be mitigated by several antioxidant defenses including peroxidases, catalases, thioredoxin reductase, peroxiredoxins, and glutathione S-transferases family enzymes which can lead to rapidly decreasing intracellular H2O2 concentrations. However, if cellular redox homeostasis cannot be maintained, H2O2 leads to reversible and irreversible oxidative modifications of proteins (e.g., carbonylation), enhancing protein ubiquitination and subsequent proteasome activation. In addition, cell cycle arrest or apoptosis could also be observed (see for review Fulda et al., 2010). Although the impact of H2O2 has been more widely investigated in mammalian cells and particularly in tumor cells (see for review Lennicke et al., 2015), studies have shown a similar impact in marine invertebrates, such as bivalves or polychaetes (Abele-Oeschger et al., 1994; McDonagh and Sheehan, 2006, 2007; Da Rosa et al., 2008; Friedman et al., 2018; Nguyen, 2020).
In the coral symbiont, H2O2 has been shown to be a by-product of photosynthesis processes (Suggett et al., 2008; Armoza-Zvuloni and Shaked, 2014). Thanks to its cell-permeable properties, H2O2 may diffuse from algal to animal host cells. Interestingly, some studies reported a release of H2O2 from non-stressed corals (Armoza-Zvuloni and Shaked, 2014) and an extracellular production by the dynamics of the superoxide anion (Saragosti et al., 2010). Therefore, due to their symbiosis lifestyle, animal host cells are daily exposed to H2O2, raising the question of their intrinsic potential to resist a massive influx of H2O2. Nevertheless, in excess, ROS (including H2O2) cause negative impact (mostly on protein and lipids) on the symbiont, leading to photosynthesis impairment, even if no bleaching phenomenon is induced (Higuchi et al., 2009; Roberty et al., 2015, 2016).
Anemonia viridis is a temperate sea anemone deeply studied as biological model of the cnidarian-dinoflagellate symbiosis. Its enzymatic antioxidant properties, tissue distribution and regulation have been intensively investigated (Hawkridge et al., 2000; Richier et al., 2003, 2005; Plantivaux et al., 2004; Merle et al., 2007; Ganot et al., 2011; Pey et al., 2017). In addition, the sensitivity of A. viridis to thermal and UV stresses has been well described and some mechanisms of bleaching have been decrypted, including oxidative stress and apoptosis (Richier et al., 2006; Moya et al., 2012). Recently, we succeeded in the establishment of primary cell cultures from A. viridis exhibiting a gastrodermal signature (Barnay-Verdier et al., 2013; Ventura et al., 2018; Fricano et al., 2020). Thus, to test the hypothesis of an intrinsic resistance of animal cells to H2O2, we exposed A. viridis specimens and primary cell cultures at the same H2O2 concentrations (200 and 500 μM) during the same periods of time (24 h and 7 days), and compared the respective responses. For each treatment, we monitored global health parameters (symbiotic state, viability and cell growth) and stress biomarkers (global antioxidant capacity, oxidative protein damages, and protein ubiquitination). This allowed us to assess the cnidarian cell susceptibility to H2O2 exposure, highlighting the putative influence of the tissue organization or/and of the presence of symbionts.
Materials and Methods
Biological Material
Anemonia viridis Specimens
Specimens of A. viridis (Forskal 1775) were collected (prefectural authorization n107; 28 February 2019) from “Plage des ondes,” Antibes, France, (43°33′17″ N, 7°07′17.7″ E), and maintained in a closed-circuit aquarium with artificial seawater (ASW, Prodibio Expert Reef Salt) at 36–38‰ at 18.0 ± 0.5°C with weekly water changes. A LED bar (450 nm—Deckey LED aquarium) provided light at a constant saturating irradiance of 100 μmol m–2s–1 (measured using a special sensor QSL-100, Biospherical Instruments Inc., San Diego, CA, United States) on a 12 h:12 h (light:dark) photoperiod. Sea anemones were fed once a week with oysters.
Gastrodermal Primary Cell Cultures
Independent primary cell cultures were obtained from different A. viridis individuals and maintained as described in Ventura et al. (2018) and Fricano et al. (2020). Briefly, cells were cultured in the dark in a thermo-regulated incubator (POL-EKO-APARATURA, Poland) at 20.0 ± 0.5°C. The optimized culture medium was replaced weekly and consisted of 20% GMIM (Gibco, Carlsbad, CA, United States), 5% fetal bovine serum (FBS; PAA/GE Healthcare, Chicago, IL, United States), 1% kanamycin (100 μg/mL, Sigma-Aldrich), 1% amphotericin B (2.5 μg/mL; Interchim, Montluçon, France), 1% antibiotic antimycotic solution (Sigma-Aldrich), 1% L-glutamate (Sigma-Aldrich), and 71% of filtered ASW. The medium was adapted in respect to the Mediterranean Seawater characteristics (i.e., salinity 40 ppt and pH 8.1).
Hydrogen Peroxide Experimental Design
In vivo Experiments
Eight specimens of A. viridis were kept individually in 5 L tanks under controlled conditions. Six tentacles were cut from each specimen after 24 h and 7 days to assess the control condition. For 200 and 500 μM H2O2 treatments, a solution of H2O2 (Sigma-Aldrich) was added in each tank (200 μM for four of them and 500 μM for the four others). After 24 h and 7 days of treatment, six tentacles were cut from each specimen to assess the treatment condition. All tentacles from the different time points and conditions were longitudinally opened and the gastroderm (containing the dinoflagellates) was manually separated from the epiderm. A centrifugation at 1,000 × g for 5 min was used to separate the gastrodermal compartment from the dinoflagellates fraction (Richier et al., 2003).
In vitro Experiments
The cell response to H2O2 treatment was assessed on at least three independent primary cell cultures. Cells seeded in 12-well plates were exposed to H2O2 treatment (Sigma-Aldrich) starting with a dose-response experiment (between 0 and 2 mM) for 7 days. Another set of experiments was performed by incubating cells to 0 (control), 200 and 500 μM H2O2 for short term (1, 2, and 6 h) or long term (1 day or 7 days) exposure. We then conducted resilience experiments by reseeding the treated cells in their normal culture medium for 7 days to assess their recovery capacity. Three wells, for each condition and time point, were used for all analyses.
Symbiotic State Assessment of A. viridis Specimens
The density of the endosymbionts from the genus Philozoon sp. (LaJeunesse et al., 2021) was assessed according to Zamoum and Furla (2012). Briefly, two tentacles were cut from each animal before and after H2O2 treatment in each condition (0, 200, or 500 μM H2O2) and then put in 2 M solution of NaOH and incubated at 37°C for 1 h to dissolve all animal tissue. To determine symbiont density, three replicate samples were counted using a modified Neubauer hemocytometer (Sigma-Aldrich). The remaining extract was used to determine the protein content from which we normalized symbiont density.
Protein Extractions
Gastrodermal cells from primary cultures and the animal’s epidermal and gastrodermal tissues were all separately placed in 200 μL of lysis extraction buffer (HEPES 25 mM, MgCl2 5 mM, EDTA 5 mM, DTT 5 mM, and PMSF 2 mM) and homogenized. Tissue samples were potterized, and both tissue and cell samples were sonicated and centrifuged to obtain the cytosoluble protein content. The total protein concentration of each sample was then assessed by a Bradford assay (Bradford reagent; SIGMA-ALDRICH), using 0–2 μg/ml bovine serum albumin solutions as standard curve.
Cell Viability and Growth Rate of Cultivated Cells
Cell viability was assessed by evaluating the membrane integrity using the Evans blue method. Therefore, viable cells (unstained) and dead cells (stained) were identified and counted on a Neubauer improved hemocytometer (Sigma-Aldrich) using an optic microscope (Zeiss Axio Imager Z1). The cell viability was defined as the percentage of viable cells relative to total cells (i.e., viable and dead cells). Cell growth rate was assessed every week using the cells counts with Evans blue method, as previously described in Ventura et al. (2018) and Fricano et al. (2020). The following formula was then used to calculate the weekly growth rate:
Total Oxidative Scavenging Capacity Assay
The oxygen radical scavenging activities of protein samples were determined using fluorometric assay according to Naguib (2000). Protein samples are incubated with phosphate buffer (75 mM pH 7.5), in presence of 180 nM 6-carboxyfluorescein as fluorescent probe and 36 mM AAPH [2,2′-azobis(2-amidinopropane) dihydrochloride] as the peroxyl radical generator. In the assay, Trolox [6-hydroxy-2,5,7,8-tetramethylchroman-2-carboxylic acid] is used as antioxidant calibrator. Fluorescence recordings were performed in black microplates (96-wells Greiner Bio-One), and fluorescence decay was measured by spectrofluorometer (Safas, Monaco) every minute for a total duration of 1 h at an excitation/emission wavelength of 520/495 nm. Relative antioxidant activities of protein samples (tested in duplicate) were measured by comparison with Trolox standard. Results were expressed in Trolox equivalents and represented in the figures as a ratio to the control condition.
Protein Carbonylation Analysis
Carbonyl content of the cytosolic extractions was measured using an ELISA assay and spectrophotometry, according to Buss et al. (1997). Protein derivatization was done by adding a dinitrophenylhydrazine (DNP) solution (10 mM in 6 M guanidine hydrochloride, 0.5 M potassium phosphate buffer) to the protein samples. The ELISA assay used anti-DNP antibody produced in rabbit (1:500; Sigma-Aldrich) and anti-rabbit Ig (1:2000; Bio-Rad). 0–100% reduced bovine serum albumin (BSA) were used as standard curve. Carbonyl content of protein samples was expressed in nanomoles per milligram of protein and was then represented in the figures as a ratio to the control condition.
Ubiquitin Conjugates Dot-Blot Analysis
Ubiquitinated proteins were assayed, according to Haas and Bright (1985), by dot-blotting 3 μg of protein samples fixed to a nitrocellulose membrane, which was incubated with primary antibody (1:1000; Mono- and polyubiquitinylated conjugates recombinant monoclonal antibody; Enzo Life Sciences). The membrane was next incubated with the secondary antibody (1:5000; anti-mouse antibody; Sigma-Aldrich). After chemiluminescence revelation (ECL), levels of spot density were measured using image analysis on GeneTools (SynGene). Levels of ubiquitinated proteins were obtained after normalization by spot density measured after amido black solution staining. Results were represented in the figures as a ratio to the control condition.
Detection of Intracellular Reactive Oxygen Species
Intracellular ROS were detected in cultivated cells treated or not with H2O2 using a sensitive fluorescent probe that penetrates the cell and, when oxidized by intracellular free radicals, binds to DNA, emitting a more intense green fluorescence (CellROX™ Green Reagent, Invitrogen). We followed the manufacturer’s instructions. Briefly, 10 μL of a 500 μM solution of CellROX™ Green were added to each treated well during 30 min at room temperature in the dark. After rinsing, cells were resuspended in 200 μL of PBS 0.6 M. Samples were then analyzed by flow cytometry (CytoFLEX LX, Beckman Coulter), excited at 488 nm and detected at 515–530 nm. For data analysis, we selected the green-fluorescent cells (CellROX-positive cells) within the population of interest. The population of interest corresponds to the cell population from which the analysis has been conducted after hierarchical exclusion of debris and doublets. For each experiment, controls (i.e., untreated cells) allowed to evaluate the basal intracellular ROS level in the cell cultures.
Statistical Analysis
For all cellular and biochemical markers evaluated in this study, the effect of the different treatments was analyzed with a two-way ANOVA test or Kruskal–Wallis test followed by, respectively, a Tukey’s or Dunn post hoc, depending on the homoscedasticity of the data set. All experiments, i.e., in vivo and in vitro, were conducted on at least three independent biological replicates.
Results
Hydrogen Peroxide Effects on A. viridis Specimens
To determine the susceptibility of A. viridis specimen to H2O2, we monitored the sign of bleaching, tissue necrosis and potential mortality during 7 days of exposure. No sign of tissue necrosis or mortality of the A. viridis specimens have been observed during H2O2 exposure. In addition, no differences in the symbiotic state have been measured since the quantity of symbionts, Philozoon sp. genus, per mg of protein remained the same after 7 days of treatment with both H2O2 concentrations (Figure 1). In addition, the sea anemones were also monitored several weeks after the treatment, and no signs of bleaching or disease were observed (data not shown).
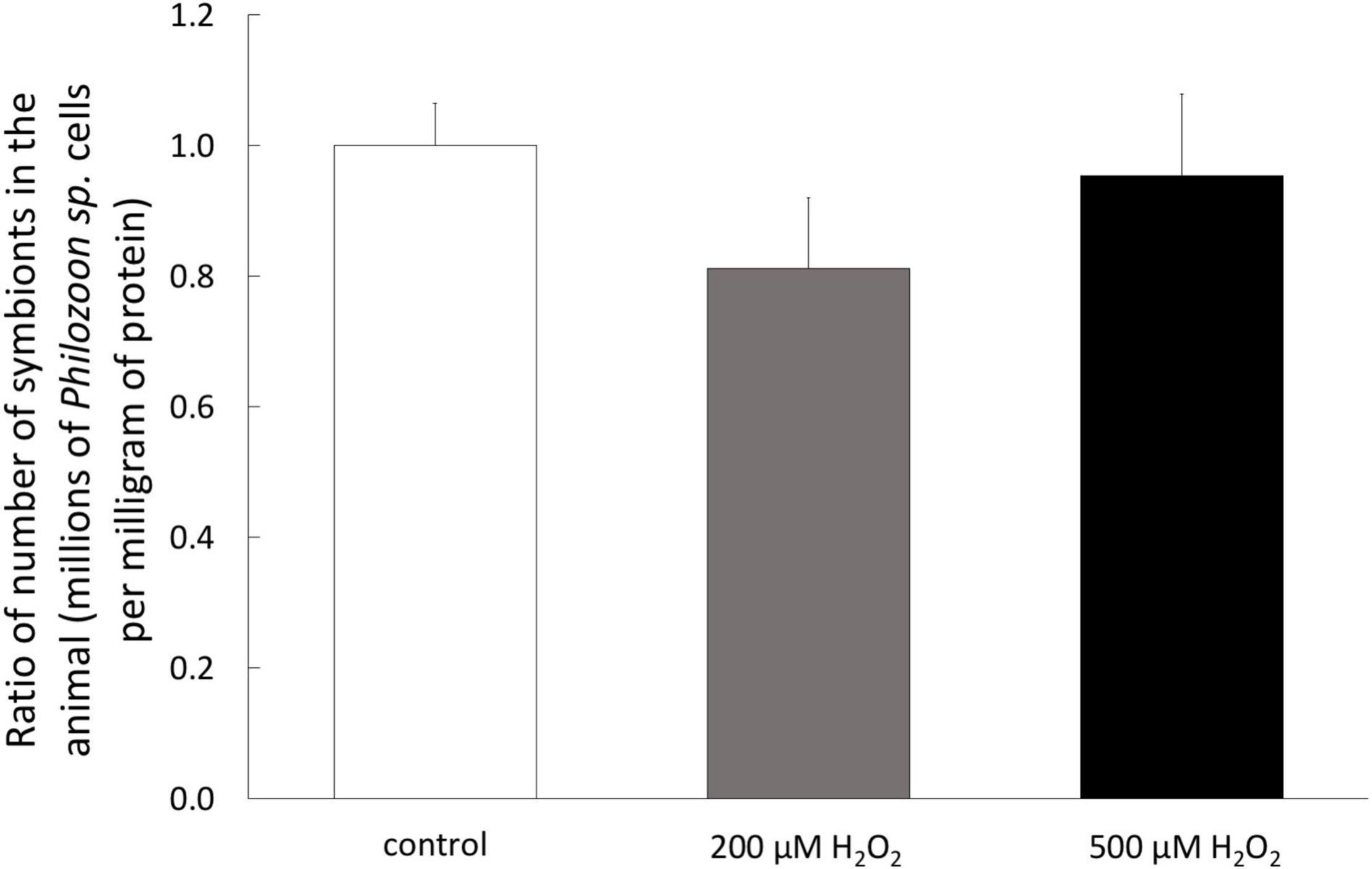
Figure 1. Symbiotic state of A. viridis specimens under H2O2 treatments. Content of Philozoon sp. symbionts in the tentacles of A. viridis before and after 7 days of 0 μM (control, white bars) 200 μM (gray bars) and 500 μM (black bars). At day 0, the A. viridis specimens hosted 2.3 × 106 symbiont mg–1. Data are represented as means with standard error bars.
Hydrogen Peroxide Effects on Stress Biomarkers of A. viridis Tissues Under Treatments
To analyze the impact of H2O2 at the tissue level, we assessed three biochemical stress markers (TOSC, carbonylation, and ubiquitination) known to be associated with oxidative stress conditions, in the three tissue compartments (epiderm, gastroderm, and symbiont) separately. In the epiderm, we only observed a slight but significant decrease of TOSC after 7 days of 200 μM H2O2 (Figures 2A–C). It is noteworthy that after 1 day of 200 μM H2O2, we observed a trend of increasing protein damages (carbonylation and ubiquitination), which totally disappeared after 7 days.
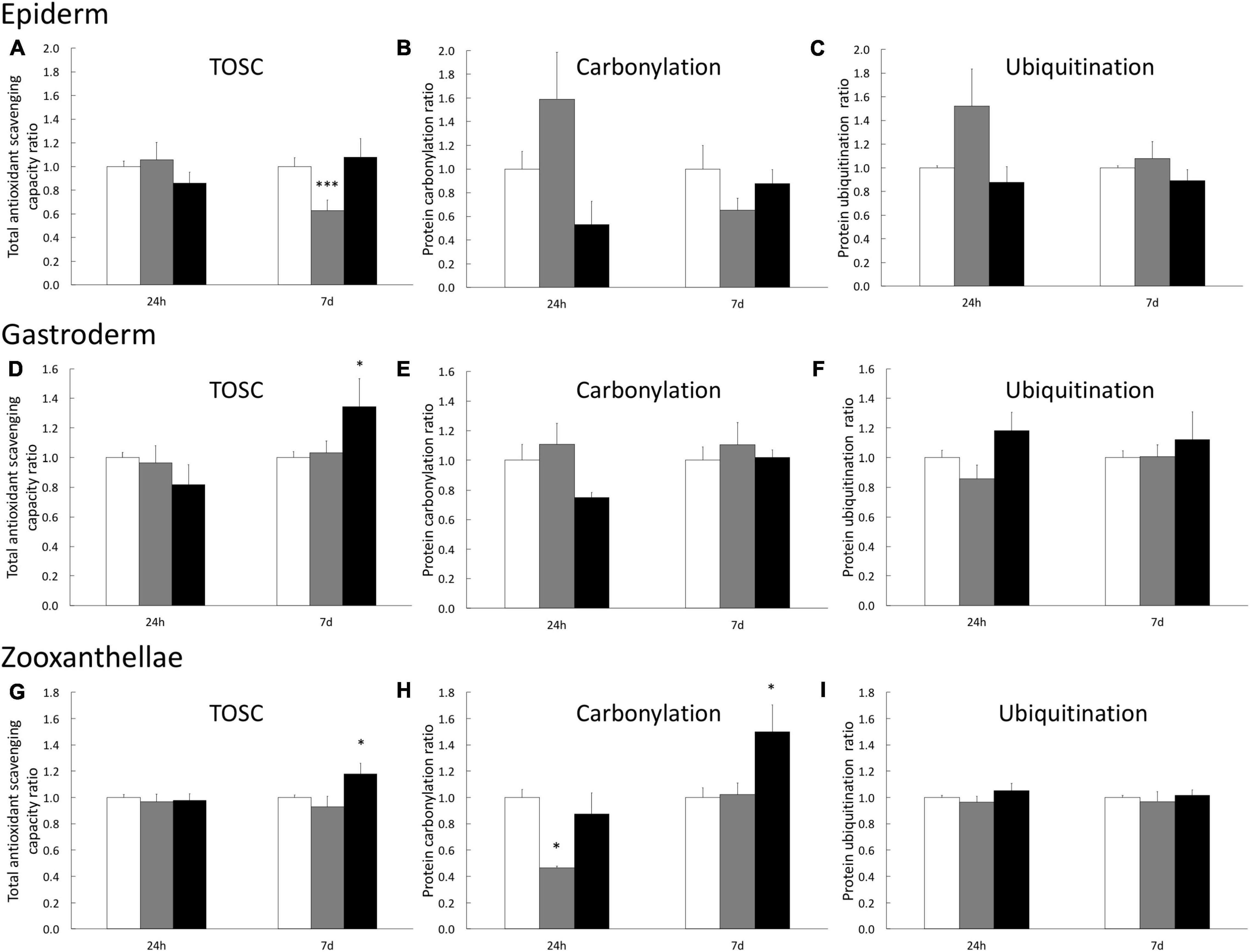
Figure 2. Stress biomarkers in A. viridis tissues under H2O2 treatments. Measurements have been performed separately on animal’s epiderm extracts (A–C), on animal’s gastroderm extract (D–F) and on symbiont extracts (G–I) from the gastroderm. Total Oxidative Scavenging Capacity (A,D,G), protein carbonylation (B,E,H) and protein ubiquitination (C,F,I) were measured under 0 μM H2O2 (control; white bars), 200 μM H2O2 (gray bars) and 500 μM H2O2 (black bars). Data are expressed relative to the control condition (ratio of the control condition). Means values with standard error bars are presented. Significant differences between control and stress condition are expressed by *p < 0.05 and ***p < 0.001.
In the gastrodermal compartment (i.e., animal tissue), we only observed an effect of H2O2 treatments after 7 days of 500 μM H2O2, inducing a 34% increase of TOSC (Figure 2D) which is sufficient to avoid protein damages (Figures 2E,F). Finally, the analysis on the symbiont fraction showed an increase of TOSC (17% higher than the control), associated with an increase of protein carbonylation level (50% higher than the control) after 7 days at 500 μM H2O2 (Figures 2G,H). No impact on symbiont protein ubiquitination has been measured (Figure 2I). Taken together these data suggest a higher capacity of cnidarian animal cells to cope with H2O2 and prevent protein damages compared to the algal symbiont.
Dose Response of Gastrodermal Cultivated Cells to Hydrogen Peroxide
To gain more insight in the capacity of the animal gastrodermal compartment to cope with ROS we first assessed the overall toxicity of H2O2 using gastrodermal cell cultures from A. viridis. More precisely, we applied a range of H2O2 concentrations from 100 μM to 2 mM for 7 days and assessed cellular growth and viability (Figure 3). Without any treatment, the mean viability of cultivated cells was 93% ± 1.17 and the weekly growth rate was 18.7 ± 4.5. At 100 μM, no effect on viability and growth rate was observed. The first significant effects on both parameters appeared at 200 μM (10% viability and 42% growth rate decreases) and drastically exacerbated at 500 μM. With a concentration of 500 μM H2O2, the viability was decreased by 58% (EC50 = 400.8 μM) and the growth was totally arrested. These results led us to direct further experiments toward the concentrations of 200 and 500 μM H2O2, where the response of the cells seemed pivotal and allowing the comparison with the in vivo experiments.
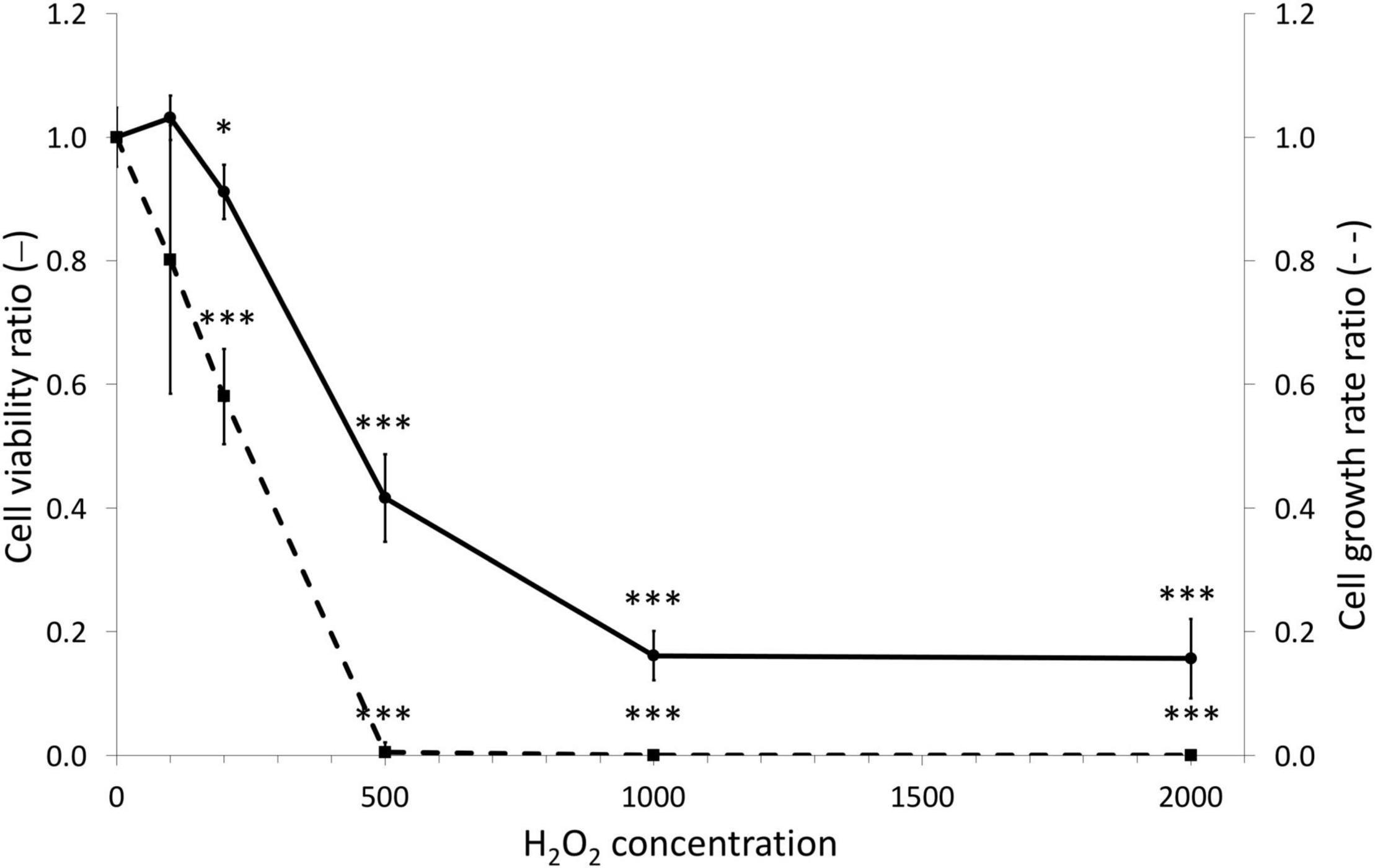
Figure 3. Dose viability and growth responses of gastrodermal cultivated cells under H2O2 treatments. A. viridis gastrodermal cultivated cells were treated with H2O2 at different concentrations for 7 days before measuring cell viability (solid line) and cell growth rate (dashed line). Cell viability and growth rate are expressed relative to the control condition (ratio of the control condition). Means values with standard error bars are presented. Significant differences between control and stress condition are expressed by *p < 0.05 and ***p < 0.001.
Hydrogen Peroxide Effects on Oxidative Status of Gastrodermal Cultivated Cells
To test whether H2O2 treatments induced an intracellular ROS increase, we stained cultivated cells with specific fluorescent probe (CellROX™ Green) allowing to quantify the positive cells for CellROX™ Green. The level of intracellular ROS was thus correlated to the percentage of positive cells for CellROX™ Green. Interestingly, this analysis revealed that although an increasing trend, there was no significant difference between the control (no treatment) and the cells treated with 200 μM H2O2, whatever the time point (Figure 4). However, there was a significant increase of ROS-positive cells (2.7 times higher in average, 11.2% vs. 4.1%) within the cells treated with 500 μM H2O2 from 1 h until 7 days of treatment.
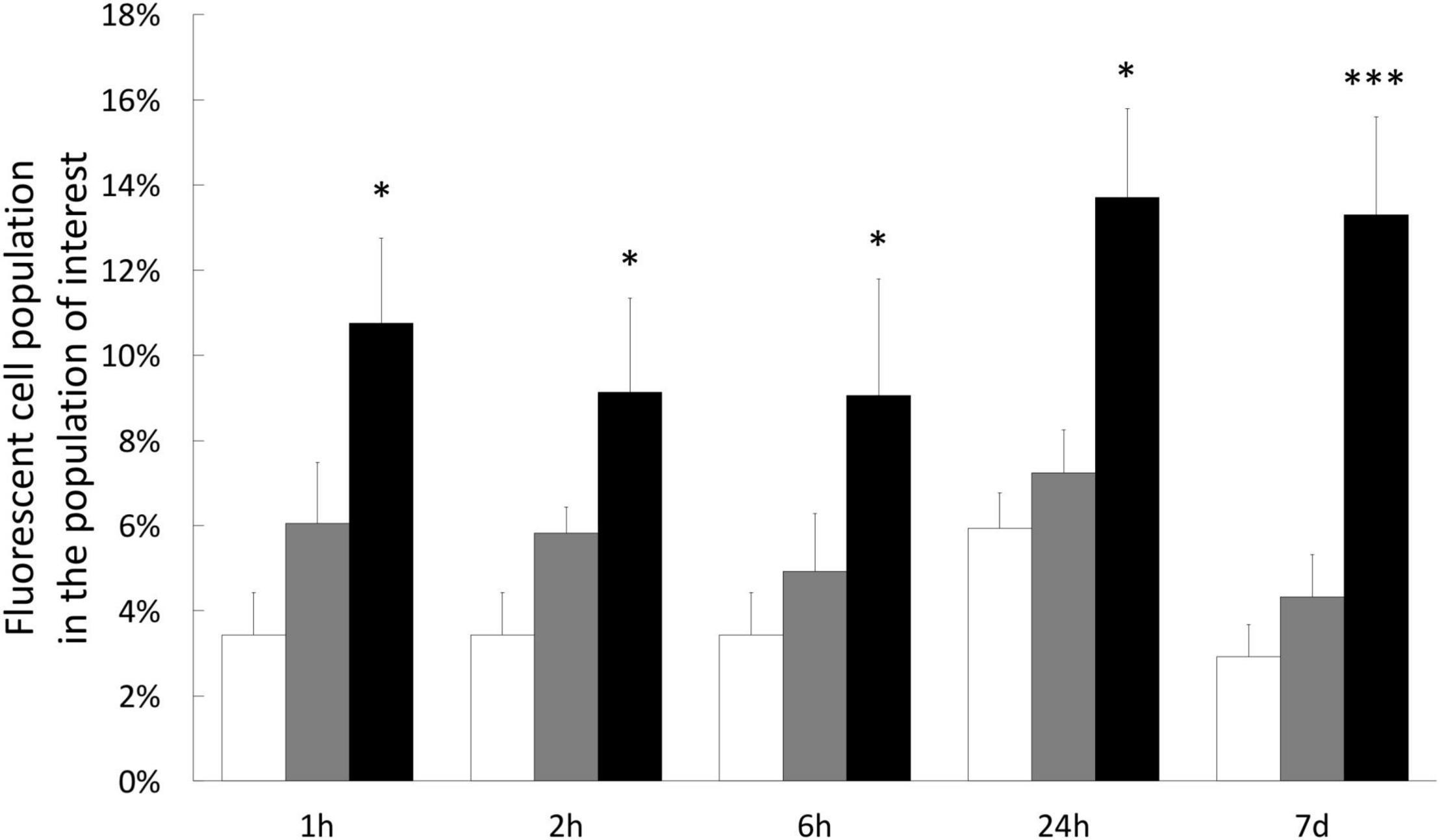
Figure 4. Quantification of intracellular ROS in gastrodermal cultivated cells under H2O2 treatments. Intracellular content of ROS was measured by staining cells with CellROX Green Reagent after 1 h, 2 h, 6 h, 1 day, or 7 days with 0 μM H2O2 (control condition; white bars), 200 μM H2O2 (gray bars) or 500 μM H2O2 (black bars). Results represent the percentage of fluorescent cells (CellROX-positive cells), within the population of interest, quantified by flow cytometry. Data are represented as means with standard error bars. Significant differences between control and treatments are expressed by *p < 0.05 and ***p < 0.001.
Hydrogen Peroxide Effects on Cellular Parameters Under Treatments and Resilience Phase
We next wondered whether H2O2 exposures induce a stress at the cellular level by monitoring cellular parameters during treatments. In addition, we also evaluated the resilience capacity of treated cells by following the same parameters after a week-recovery phase. Firstly, the analysis of the cell viability, during 1–6 h of exposures, showed no impact of 200 or 500 μM H2O2 treatments (see Supplementary Figure 1). At 200 μM, there was only a light significant decrease in cell viability (9%) after 7 days of exposure, which was totally recovered after the resilience period (Figure 5A). At 500 μM, a 28% decrease was observed at 1 day that worsened after 7 days (58% of decrease, Figure 5A), confirming our results obtained during the dose-response assay. After a resilience period, only a partial recovery was observed (71% of the control condition), suggesting that not all the cells that survived may be able to compensate the H2O2–induced stress. In line with this hypothesis, the analysis of the cell population by flow-cytometry showed, after 7 days of 500 μM H2O2, a modification of cell-population pattern associated with a significant increase of autofluorescence (in average: 3.86% vs. 0.53% for the control condition; p-value = 0.021; Figure 6), which could be reflecting cell apoptosis.
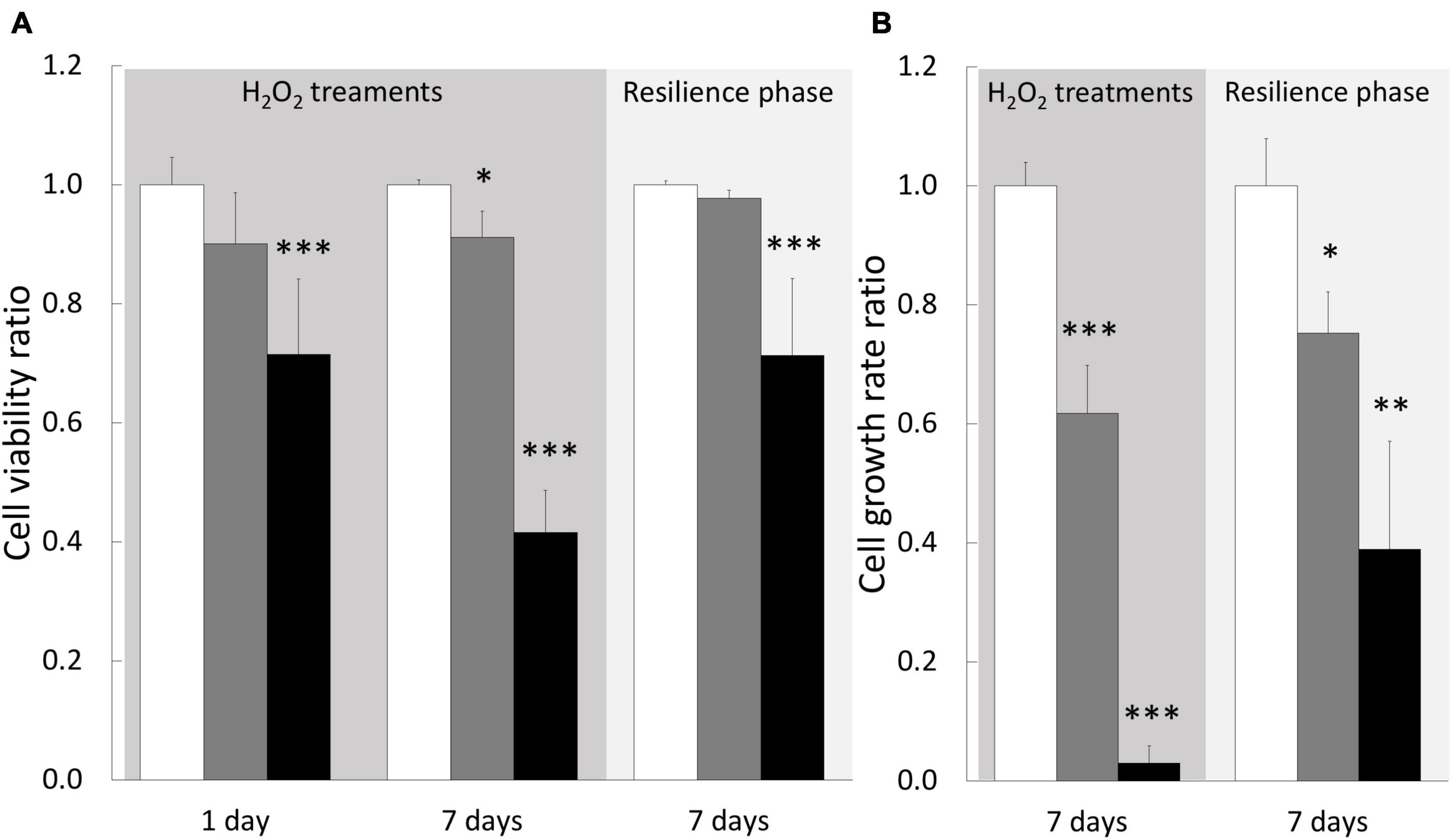
Figure 5. Cellular parameters of gastrodermal cultivated cells under H2O2 treatments and resilience phase. Cell viability (A) and cell growth rate (B) were measured under cell treatment with H2O2 at 200 and 500 μM and during resilience phase (back to control condition for 7 days): 0 μM H2O2 (control condition; white bars), 200 μM H2O2 (gray bars) and 500 μM H2O2 (black bars). Cell viability and growth rate are expressed relative to the control condition (ratio of the control condition). Data are represented as means with standard error bars. Significant differences between control and treatments are expressed by *p < 0.05, **p < 0.01, and ***p < 0.001.
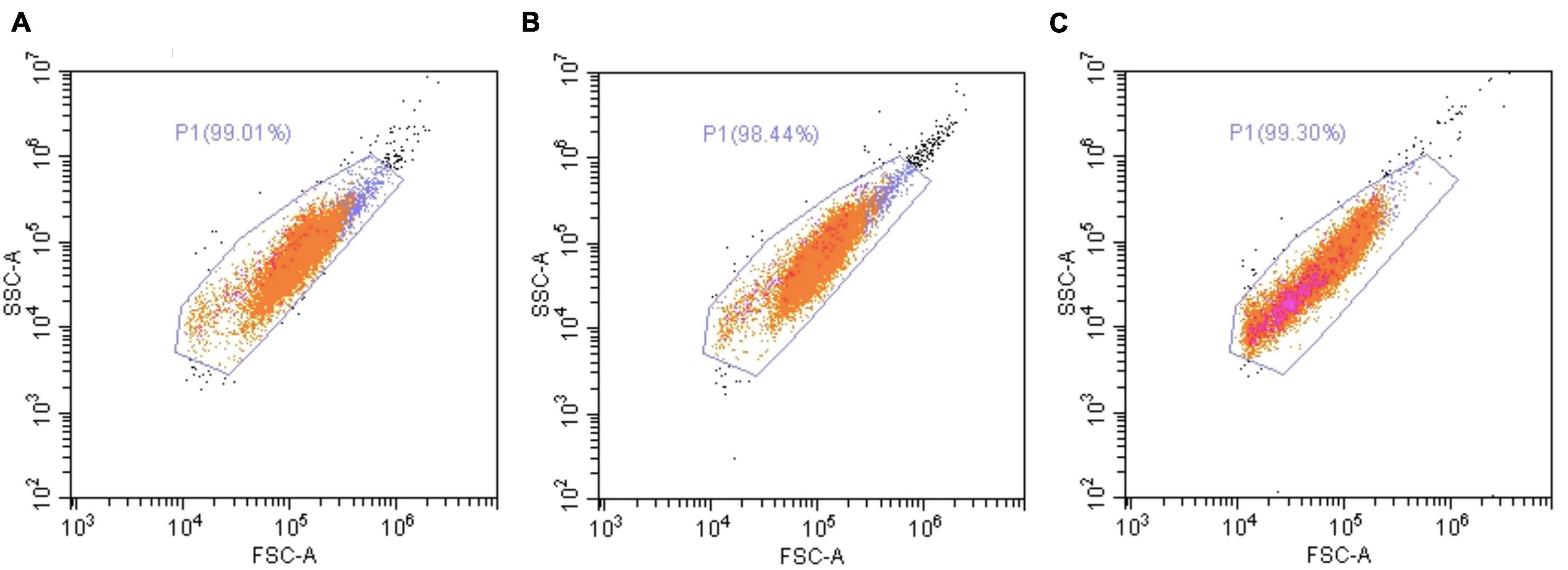
Figure 6. Flow cytometry analysis of A. viridis cultivated cells under a 7-days treatment with H2O2. Flow cytometry analysis were performed on cultivated cell population by excitation at 488 nm and detection at 515–530 nm, after 7 days of incubation without H2O2 (A) or with 200 μM (B) or 500 μM (C) of H2O2. Representative dot plots of all experiments performed are shown. Orange dots: cells of interest; pink dots: green-autofluorescent cells.
The growth rate measurements showed a significant impact of both treatments, 200 and 500 μM H2O2, with a dose-dependent response as we observed a decrease of 38 and 97%, respectively (Figure 5B). This H2O2 dose-dependent response was maintained after the resilience period with a partial growth rate recovery for both treatments (75 and 39% of control, respectively, for 200 and 500 μM H2O2). Taken together these data suggested that 200 μM H2O2 can be considered as the tolerance threshold of cultivated cnidarian cells while 500 μM H2O2 can represent a critical concentration inducing deleterious cellular injuries.
Hydrogen Peroxide Effects on Stress Biomarkers Under Treatments and Resilience Phase
To determine the cellular response induced by H2O2 treatments on cultivated cells we analyzed biochemical stress markers (TOSC, carbonylation, and ubiquitination). After 1–6 h of treatment, we observed no significant effect on any of the three biomarkers (Supplementary Figure 2). However, after 24 h of treatment TOSC was impaired at both 200 and 500 μM H2O2. A full recovery was observed after 7 days of treatment and maintained during the resilience phase (Figure 7A), suggesting an acclimation of the cells to the pro-oxidant conditions. No signs of protein damage (protein carbonylation and ubiquitination) were measured after 24 h. However, the 200 μM H2O2 treatment induced an increase of ubiquitinated proteins after 7 days (Figure 7C). This effect was totally abolished after the resilience period (Figure 7C). These data suggest that H2O2 exposures, even at 500 μM and after 7 days, didn’t lead to persistent oxidative damages on proteins in cnidarian cultivated cells.
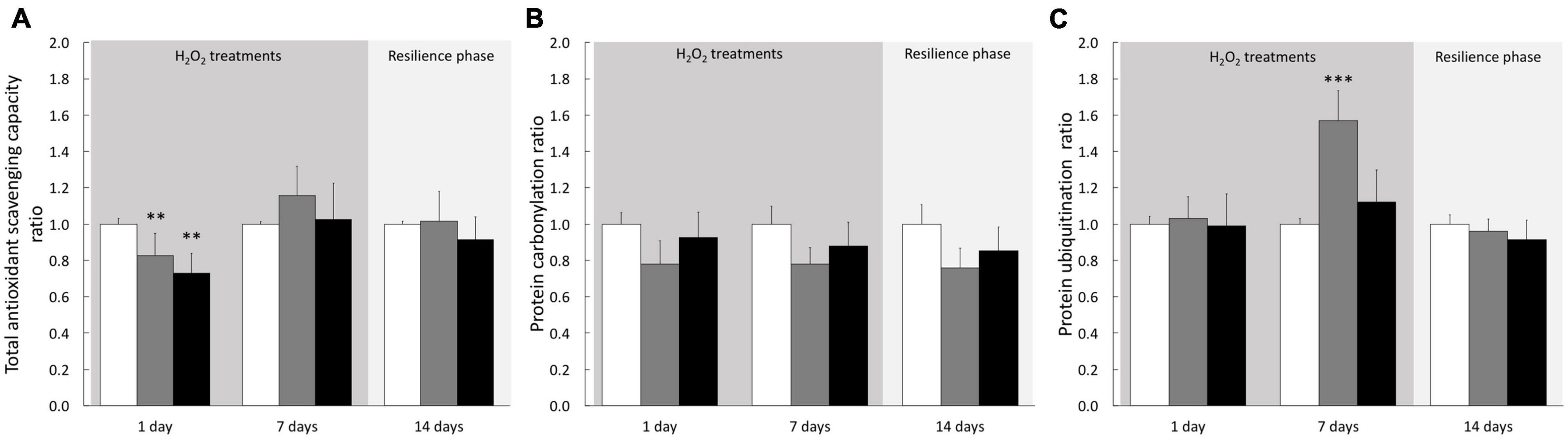
Figure 7. Stress biomarkers in A. viridis cultivated cells under H2O2 treatments and resilience phase. Total Oxidative Scavenging Capacity, TOSC (A), protein carbonylation (B) and protein ubiquitination (C) were measured under cell treatment with H2O2 at 200 and 500 μM and during resilience phase (back to control condition for 7 days): 0 μM H2O2 (control; white bars), 200 μM H2O2 (gray bars) and 500 μM H2O2 (black bars). Data are expressed relative to control condition (ratio of the control condition). Means values with standard error bars are presented. Significant differences between control and treatments are expressed by **p < 0.01 and ***p < 0.001.
Discussion
Extended Tolerance of Symbiotic Cnidarians to Hydrogen Peroxide
In this study, we used H2O2 to induce pro-oxidative condition and to investigate the stress response in the symbiotic sea anemone A. viridis at both whole animal and cellular scales. The H2O2 concentrations used in the present study correspond to extremely high levels never measured in the seawater. However, rainwater can temporally induced increase of H2O2 to tens of micromolar (Ndungu et al., 2019; Jones and Lee, 2020). Benthic marine organisms from coastal areas are therefore regularly facing H2O2, that could lead to oxidative stress (Abele-Oeschger et al., 1997). Indeed, among ROS, H2O2 is the most abundant and long-lived in sea water and contrary to other ROS, H2O2 could rapidly diffuse across membranes (see for review Halliwell and Gutteridge, 2015).
In our study model, at both whole animal and cellular scales, 200 μM of H2O2 did not create a condition of stress, since very weak impact was observed in global health: no sign of mortality or bleaching on specimens (Figure 1), no intracellular ROS accumulation (Figure 4) and only very slight effect on viability after 7 days exposure in cell cultures (Figure 5). In addition, no effect was observed on any stress biomarkers we tested (Figures 2, 7). In cell cultures, we nevertheless measured a significant cellular growth arrest (Figure 5), reflecting a common feature of stress response and could be interpreted as an usual resistance mechanism (see for review Davies, 1999, 2000).
H2O2 usually represents a threat for most organisms. Indeed, in diverse mammalian cell lines, a cytotoxic effect of H2O2 could be observed from 60 μM (Coyle et al., 2006) with drastic and irreversible impacts (i.e., apoptosis and necrosis) induced at 400 μM H2O2 (Xiang et al., 2016). Some studies performed on marine invertebrates showed that micromolar concentrations of H2O2, ranging from 0.5 to 20 μM, could impact the metabolism of the whole animal. For example, a 40% drop in O2 consumption was observed in the Polychaete Nereis diversicolor under 5 μM of H2O2 (Abele-Oeschger et al., 1994), while higher level of H2O2 (50 μM) can cause oxidative damages (i.e., lipid peroxidation), as observed in another Polychaete species, Laenoereis acuta (Da Rosa et al., 2008).
Contrasting with those results, our study highlighted an extended tolerance of symbiotic cnidarian facing even greater H2O2 concentrations. This resistance to H2O2 is, however, not a general cnidarian feature as it has been shown that concentrations exceeding 163 μM H2O2 caused mortality of the non-symbiotic sea anemone Nematostella vectensis (Friedman et al., 2018). Therefore, these results reinforced the hypothesis of the adaptation of symbiotic cnidarians to pro-oxidative conditions, due to their lifestyle with a photosynthetic symbiont. Indeed, it has been already highlighted that symbiotic cnidarians exhibited a wide diversity of biochemical antioxidant actors, compared to non-symbiotic species (Furla et al., 2005). For instance, higher number of superoxide dismutase (SOD) isoforms was identified in the symbiotic cnidarian A. viridis compared to the non-symbiotic one Actinia schmidti. In complement, another comparative analysis showed that the glutathione peroxidase (GPx) isoforms were less numerous in the non-symbiotic sea anemone N. vectensis than in the symbiotic sea anemone A. viridis (Pey et al., 2017). To confirm the hypothesis of the adaptative process, it will be required to extend the comparison of the antioxidant battalion between symbiotic and non-symbiotic cnidarians at multiple scales, even by including the non-enzymatic actors.
Importance of Host Cells to Hydrogen Peroxide Defense
In the present study, H2O2 exposure on whole organism affected mainly the endosymbiont, Philozoon sp. genus (LaJeunesse et al., 2021), rather than the animal host tissues. Although antioxidant defenses were stimulated after 7 days, an increase of protein carbonylation was measured in the symbiont fraction, whereas no increase was observed in the animal compartments (Figure 2). The susceptibility of free-living algae to H2O2 is well documented and highlighted an important heterogeneity in H2O2 response. For example, although the cyanobacterium Synechococcus aeruginosus tolerated until 2 mM H2O2, another cyanobacterium species, Microcystis aeruginosa, was affected by around 20 μM (EC50) and the diatoms Navicula seminulum by 200 μM (Drábková et al., 2007). Few studies have been performed on Symbiodiniaceae sensitivity to ROS and again they highlighted species-specific impacts on photosynthesis (Wietheger et al., 2015; Roberty et al., 2016). For example, cultured Symbiodinium microadriaticum strain showed high resistance to 1 mM H2O2, compared to Fugacium kawagutii showing drastic damage to photosystem function at the same H2O2 concentration (Wietheger et al., 2015). In addition, light exposure increased the photosynthesis impairment of cultured Symbiodiniaceae from 30 min of >1 mM H2O2 exposure (Wietheger et al., 2015).
Protected inside the gastrodermal host cell, the endosymbiont would not have shown any signs of oxidative stress under H2O2 exposure, but the sensitivity assessed in our study did not confirm this assumption. This sensitivity to H2O2 could, however, be correlated to previous studies demonstrating that Symbiodiniaceae living in hospite present a reduction of the antioxidant enzymatic defenses (i.e., SOD, catalases or peroxidases) compared to the free-living condition (Lesser and Shick, 1989; Richier et al., 2005; Pey et al., 2017). Nevertheless, it has been shown that in hospite, Symbiodiniaceae harbor a higher surface volume of thylakoid lamellae (Lesser and Shick, 1990), increasing definitely the photosystem density and consequently the source of ROS associated with electron chain transports (Saragosti et al., 2010).
These data, in addition with measurements of H2O2 diffusion from the symbiont (Suggett et al., 2008; Armoza-Zvuloni and Shaked, 2014), support the conclusion that, in hospite, the redox homeostasis of the symbiont is bolstered by the antioxidant defenses of the animal host cells. For example, in A. viridis it has been frequently observed that the animal compartment constitutes the major contributor to the holobiont antioxidant potential, with higher amount of antioxidant defenses compared to the symbiont fraction (Richier et al., 2003, 2005; Plantivaux et al., 2004; Merle et al., 2007; Pey et al., 2017). This agrees with studies performed on other symbiotic cnidarian species (Yakovleva et al., 2004; Levy et al., 2006; Krueger et al., 2015). By consequence, an experimental burst of H2O2 leads to more deleterious effects in the endosymbiont than in animal cells, illustrating their capacity to cope with H2O2.
No Bleaching Induction by Hydrogen Peroxide
Even at highest H2O2 exposure, no bleaching was observed neither during, nor after the exposure period in the treated A. viridis specimens (Figure 1). Interestingly, despite the protein damages observed in the symbiont, the equilibrium of the symbiosis was maintained. It has been largely documented in symbiotic cnidarians that stress-induced bleaching (e.g., thermal stress) is linked with the over-production of ROS by the endosymbiont, leading to significant oxidative damages in the host cells (see for review Suggett and Smith, 2020). Due to its permeable properties and its overproduction in several strains of Symbiodiniaceae exposed to thermal stress (Lesser, 1996; Suggett et al., 2008; Roberty et al., 2015), this ROS has then been suggested to be responsible of oxidative stress occurring in host cells during bleaching events. However, in this study, the absence of bleaching and of oxidative damages in host cells under H2O2 exposures can support the conclusion that H2O2 may not be the most important ROS associated with coral bleaching.
Limits of the Resistance
At cellular level, pro-oxidative condition can elicit a broad spectrum of responses from proliferation to growth arrest, or senescence and cell death, depending on the cell capacity to overcome the stress by repairing or removing damaged molecules. The observed effect reflects the balance between intracellular pathways activated in response to the oxidative injury and can vary significantly with the concentration of the oxidant agent and the treatment exposure. In our in vitro study model, 500 μM of H2O2 induced a decrease of cell viability, particularly pronounced after 7 days of treatment and associated with a drastic growth arrest (Figure 5).
This response could be explained by a strategy of the “sacrifice” signaling pathway set up by the cells to eliminate the most damaged cell population (Davies, 2000). Indeed, a change in the cell population pattern was highlighted after 7 days at 500 μM H2O2 (Figure 6) and no protein damages were observed in these surviving cells (Figure 7). Nevertheless, the increase of autofluorescence measured in surviving cells after 7 days at 500 μM H2O2 (Figure 6) might be correlated with changes in metabolic activity of mitochondria that cells undergo during apoptosis, as it has been previously observed in mammalian cells (Levitt et al., 2006). In addition, the partial recovery of cell growth after the resilience period (Figure 5) suggested a non-reversible impact of 500 μM H2O2 injury on surviving cells, whose mechanisms should be deeply addressed in the future.
Finally, comparing the in vitro and the in vivo approaches, we highlighted that the sensitivity of A. viridis gastrodermal cells at 500 μM of H2O2 exposure is less pronounced in the gastrodermal tissue than in the isolated cultivated cells (Figures 2, 7). This is likely due to the contribution of the tissue organization thanks to host cell/cell communication, more efficient turnover of damaged cells and/or by a signaling pathway linked to the presence of the symbiont.
Moving forward thanks to the in vitro cnidarian cell culture, an ambitious perspective of this study will be to disentangle the mechanisms of H2O2 resistance of cnidarian cells and more specifically to assess the impact of other ROS (as the superoxide anion and the hydroxyl radical), thus contributing to decipher the adaptative tools that have evolved for a successful symbiosis stability and conversely to better understand the bleaching processes.
Data Availability Statement
The original contributions presented in the study are included in the article/Supplementary Material, further inquiries can be directed to the corresponding author/s.
Author Contributions
PC did all the investigations (experimental work) and statistical analyses. CF contributed to the obtaining of cell cultures and their maintenance. CF and GT initialized the H2O2 experiments on cell cultures. PF and SB-V designed and supervised the research. PC, ER, SB-V, and PF wrote the manuscript. All authors read and agreed to the published version of the manuscript.
Funding
This work was supported by a doctoral fellowship (CIFRE) from SO.F.I.A Cosmétiques company and the Association Nationale de la Recherche et de la Technologie (ANRT) to PC.
Conflict of Interest
The authors declare that the research was conducted in the absence of any commercial or financial relationships that could be construed as a potential conflict of interest.
Publisher’s Note
All claims expressed in this article are solely those of the authors and do not necessarily represent those of their affiliated organizations, or those of the publisher, the editors and the reviewers. Any product that may be evaluated in this article, or claim that may be made by its manufacturer, is not guaranteed or endorsed by the publisher.
Acknowledgments
The authors greatly acknowledge Ludovic Cervera of the CYTOMED facility (IRCAN’s Flow Cytometry Facility that was supported by the FEDER, Ministère de l’Enseignement Supérieur, Région Provence-Alpes-Côte d’Azur, Conseil Départemental 06, ITMO Cancer Aviesan, Cancéropole PACA, INCa, CNRS, and INSERM). The authors are also grateful to Aldine Amiel from the ANTIAGE facility [IRCAN’s Marine Invertebrate Facility for (Anti-)Aging Research] as well as Thamilla Zamoum, Brigitte Poderini, and Maxence Burtin, for the maintenance of the aquarium and the monitoring and feeding of Anemonia viridis specimens. Finally, the authors would like to thank particularly Thalassa Marine Research & Environmental Awareness (Patricia Ventura and Alexis Pey) for the sampling of the sea anemones.
Supplementary Material
The Supplementary Material for this article can be found online at: https://www.frontiersin.org/articles/10.3389/fphys.2022.819111/full#supplementary-material
References
Abele-Oeschger, D., Oeschger, R., and Theede, H. (1994). Biochemical adaptations of Nereis diversicolor (Polychaeta) to temporarily increased hydrogen peroxide levels in intertidal sandflats. Mar. Ecol. Progr. Ser. 106, 101–110. doi: 10.3354/meps106101
Abele-Oeschger, D., Tüg, H., and Röttgers, R. (1997). Dynamics of UV-driven hydrogen peroxide formation on an intertidal sandflat. Limnol. Oceanogr. 42, 1406–1415. doi: 10.4319/lo.1997.42.6.1406
Apel, K., and Hirt, H. (2004). Reactive oxygen species: metabolism, oxidative stress, and signal transduction. Annu. Rev. Plant Biol. 55, 373–399. doi: 10.1146/annurev.arplant.55.031903.141701
Armoza-Zvuloni, R., and Shaked, Y. (2014). Release of hydrogen peroxide and antioxidants by the coral Stylophora pistillata to its external milieu. Biogeosciences 11, 4587–4598. doi: 10.5194/bg-11-4587-2014
Barnay-Verdier, S., Dall’Osso, D., Joli, N., Olivré, J., Priouzeau, F., Zamoum, T., et al. (2013). Establishment of primary cell culture from the temperate symbiotic cnidarian, Anemonia viridis. Cytotechnology 65, 697–704. doi: 10.1007/s10616-013-9566-2
Buss, H., Chan, T. P., Sluis, K. B., Domigan, N. M., and Winterbourn, C. C. (1997). Protein Carbonyl Measurement by a Sensitive ELISA Method. Free Radic. Biol. Med. 23, 361–366. doi: 10.1016/S0891-5849(97)00104-4
Cooper, W. J., Saltzman, E. S., and Zika, R. G. (1987). The contribution of rainwater to variability in surface ocean hydrogen peroxide. J. Geophys. Res. Oceans 92, 2970–2980. doi: 10.1029/JC092iC03p02970
Coyle, C. H., Martinez, L. J., Coleman, M. C., Spitz, D. R., Weintraub, N. L., and Kader, K. N. (2006). Mechanisms of H2O2-induced oxidative stress in endothelial cells. Free Radic. Biol. Med. 40, 2206–2213. doi: 10.1016/j.freeradbiomed.2006.02.017
Da Rosa, C. E., Bianchini, A., and Monserrat, J. M. (2008). Antioxidant responses of Laeonereis acuta (Polychaeta) after exposure to hydrogen peroxide. Braz. J. Med. Biol. Res. 41, 117–121. doi: 10.1590/s0100-879x2008000200007
Davies, K. J. (1999). The Broad Spectrum of Responses to Oxidants in Proliferating Cells: a New Paradigm for Oxidative Stress. IUBMB Life 48, 41–47. doi: 10.1080/713803463
Davies, K. J. (2000). Oxidative stress, antioxidant defenses, and damage removal, repair, and replacement systems. IUBMB Life 50, 279–289. doi: 10.1080/713803728
Davy, S. K., Allemand, D., and Weis, V. M. (2012). Cell Biology of Cnidarian-Dinoflagellate Symbiosis. Microbiol. Mol. Biol. Rev. 76, 229–261. doi: 10.1128/MMBR.05014-11
Drábková, M., Admiraal, W., and Maršálek, B. (2007). Combined Exposure to Hydrogen Peroxide and LightSelective Effects on Cyanobacteria, Green Algae, and Diatoms. Environ. Sci. Technol. 41, 309–314. doi: 10.1021/es060746i
Dykens, J. A., Shick, J. M., Benoit, C., Buettner, G. R., and Winston, G. W. (1992). Oxygen radical production in the sea anemone Anthopleura elegantissima and its endosymbiotic algae. J. Exp. Biol. 168, 219–241. doi: 10.1242/jeb.168.1.219
Fricano, C., Röttinger, E., Furla, P., and Barnay-Verdier, S. (2020). Cnidarian Cell Cryopreservation: a Powerful Tool for Cultivation and Functional Assays. Cells 9:2541. doi: 10.3390/cells9122541
Friedman, L. E., Gilmore, T. D., and Finnerty, J. R. (2018). Intraspecific variation in oxidative stress tolerance in a model cnidarian: differences in peroxide sensitivity between and within populations of Nematostella vectensis. PLoS One 13:e0188265. doi: 10.1371/journal.pone.0188265
Fulda, S., Gorman, A. M., Hori, O., and Samali, A. (2010). Cellular Stress Responses: cell Survival and Cell Death. Int. J. Cell Biol. 2010:214074. doi: 10.1155/2010/214074
Furla, P., Allemand, D., Shick, J. M., Ferrier-Pagès, C., Richier, S., Plantivaux, A., et al. (2005). The Symbiotic Anthozoan: a Physiological Chimera between Alga and Animal. Integr. Comp. Biol. 45, 595–604. doi: 10.1093/icb/45.4.595
Ganot, P., Moya, A., Magnone, V., Allemand, D., Furla, P., and Sabourault, C. (2011). Adaptations to Endosymbiosis in a Cnidarian-Dinoflagellate Association: differential Gene Expression and Specific Gene Duplications. PLoS Genet. 7:e1002187. doi: 10.1371/journal.pgen.1002187
Giorgio, M., Trinei, M., Migliaccio, E., and Pelicci, P. G. (2007). Hydrogen peroxide: a metabolic by-product or a common mediator of ageing signals? Nat. Rev. Mol. Cell Biol. 8, 722–728. doi: 10.1038/nrm2240
Haas, A. L., and Bright, P. M. (1985). The immunochemical detection and quantitation of intracellular ubiquitin-protein conjugates. J. Biol. Chem. 260, 12464–12473. doi: 10.1016/S0021-9258(17)38895-6
Halliwell, B., Clement, M. V., and Long, L. H. (2000). Hydrogen peroxide in the human body. FEBS Lett. 486, 10–13. doi: 10.1016/S0014-5793(00)02197-9
Halliwell, B., and Gutteridge, J. M. C. (2015). Free Radicals in Biology and Medicine, 5th Edn. Oxford: Oxford University Press.
Hansel, C. M., and Diaz, J. M. (2021). Production of Extracellular Reactive Oxygen Species by Marine Biota. Ann. Rev. Mar. Sci. 13, 177–200. doi: 10.1146/annurev-marine-041320-102550
Hawkridge, J. M., Pipe, R. K., and Brown, B. E. (2000). Localisation of antioxidant enzymes in the cnidarians Anemonia viridis and Goniopora stokesi. Mar. Biol. 137, 1–9. doi: 10.1007/s002270000324
Higuchi, T., Fujimura, H., Ikota, H., Arakaki, T., and Oomori, T. (2009). The effects of hydrogen peroxide on metabolism in the coral Goniastrea aspera. J. Exp. Mar. Biol. Ecol. 370, 48–55. doi: 10.1016/j.jembe.2008.11.013
Jones, M. R., and Lee, K. (2020). Precipitation of hydrogen peroxide during winter storms and summer typhoons. Sci. Total Environ. 733:139377. doi: 10.1016/j.scitotenv.2020.139377
Krueger, T., Hawkins, T. D., Becker, S., Pontasch, S., Dove, S., Hoegh-Guldberg, O., et al. (2015). Differential coral bleaching—Contrasting the activity and response of enzymatic antioxidants in symbiotic partners under thermal stress. Comp. Biochem. Physiol. A Mol. Integr. Physiol. 190, 15–25. doi: 10.1016/j.cbpa.2015.08.012
LaJeunesse, T. C., Wiedenmann, J., Casado-Amezúa, P., D’Ambra, I., Turnham, K. E., Nitschke, M. R., et al. (2021). Revival of Philozoon Geddes for host-specialized dinoflagellates, ‘zooxanthellae’, in animals from coastal temperate zones of northern and southern hemispheres. Eur. J. Phycol. 0, 1–15. doi: 10.1080/09670262.2021.1914863
Lennicke, C., Rahn, J., Lichtenfels, R., Wessjohann, L. A., and Seliger, B. (2015). Hydrogen peroxide - production, fate and role in redox signaling of tumor cells. Cell Commun. Signal 13:39. doi: 10.1186/s12964-015-0118-6
Lesser, M., and Farrell, J. (2004). Exposure to solar radiation increases damage to both host tissues and algal symbionts of corals during thermal stress. Coral Reefs 23, 367–377. doi: 10.1007/s00338-004-0392-z
Lesser, M. P. (1996). Elevated temperatures and ultraviolet radiation cause oxidative stress and inhibit photosynthesis in ymbiotic dinoflagellates. Limnol. Oceanogr. 41, 271–283. doi: 10.4319/lo.1996.41.2.0271
Lesser, M. P., and Shick, J. M. (1989). Effects of irradiance and ultraviolet radiation on photoadaptation in the zooxanthellae of Aiptasia pallida: primary production, photoinhibition, and enzymic defenses against oxygen toxicity. Mar. Biol. 102, 243–255. doi: 10.1007/BF00428286
Lesser, M. P., and Shick, J. M. (1990). Effects of visible and ultraviolet radiation on the ultrastructure of zooxanthellae (Symbiodinium sp.) in culture and in situ. Cell Tissue Res. 261, 501–508. doi: 10.1007/BF00313529
Levitt, J. M., Baldwin, A., Papadakis, A., Puri, S., Xylas, J., Münger, K., et al. (2006). Intrinsic fluorescence and redox changes associated with apoptosis of primary human epithelial cells. JBO 11:064012. doi: 10.1117/1.2401149
Levy, O., Achituv, Y., Yacobi, Y. Z., Stambler, N., and Dubinsky, Z. (2006). The impact of spectral composition and light periodicity on the activity of two antioxidant enzymes (SOD and CAT) in the coral Favia favus. J. Exp. Mar. Biol. Ecol. 328, 35–46. doi: 10.1016/j.jembe.2005.06.018
McDonagh, B., and Sheehan, D. (2006). Redox proteomics in the blue mussel Mytilus edulis: carbonylation is not a pre-requisite for ubiquitination in acute free radical-mediated oxidative stress. Aquat. Toxicol. 79, 325–333. doi: 10.1016/j.aquatox.2006.06.020
McDonagh, B., and Sheehan, D. (2007). Effect of oxidative stress on protein thiols in the blue mussel Mytilus edulis: proteomic identification of target proteins. Proteomics 7, 3395–3403. doi: 10.1002/pmic.200700241
Merle, P. L., Sabourault, C., Richier, S., Allemand, D., and Furla, P. (2007). Catalase characterization and implication in bleaching of a symbiotic sea anemone. Free Radic. Biol. Med. 42, 236–246. doi: 10.1016/j.freeradbiomed.2006.10.038
Moya, A., Ganot, P., Furla, P., and Sabourault, C. (2012). The transcriptomic response to thermal stress is immediate, transient and potentiated by ultraviolet radiation in the sea anemone Anemonia viridis. Mol. Ecol. 21, 1158–1174. doi: 10.1111/j.1365-294X.2012.05458.x
Naguib, Y. M. A. (2000). A Fluorometric Method for Measurement of Oxygen Radical-Scavenging Activity of Water-Soluble Antioxidants. Anal. Biochem. 284, 93–98. doi: 10.1006/abio.2000.4691
Ndungu, L. K., Steele, J. H., Hancock, T. L., Bartleson, R. D., Milbrandt, E. C., Parsons, M. L., et al. (2019). Hydrogen peroxide measurements in subtropical aquatic systems and their implications for cyanobacterial blooms. Ecol. Eng. 138, 444–453. doi: 10.1016/j.ecoleng.2019.07.011
Nguyen, L. (2020). The Effects of Photosymbiosis on Gene Expression in the Facultatively Symbiotic Coral Astrangia poculata, With a Focus on NF-Kappab Signaling and Antioxidant Enzymes. Available Online at: https://open.bu.edu/handle/2144/41678 [accessed Dec 13, 2021].
Pey, A., Zamoum, T., Allemand, D., Furla, P., and Merle, P.-L. (2011). Depth-dependant thermotolerance of the symbiotic Mediterranean gorgonian Eunicella singularis: evidence from cellular stress markers. J. Exp. Mar. Biol. Ecol. 404, 73–78. doi: 10.1016/j.jembe.2011.05.007
Pey, A., Zamoum, T., Christen, R., Merle, P.-L., and Furla, P. (2017). Characterization of glutathione peroxidase diversity in the symbiotic sea anemone Anemonia viridis. Biochimie 132, 94–101. doi: 10.1016/j.biochi.2016.10.016
Plantivaux, A., Furla, P., Zoccola, D., Garello, G., Forcioli, D., Richier, S., et al. (2004). Molecular characterization of two CuZn-superoxide dismutases in a sea anemone. Free Radic. Biol. Med. 37, 1170–1181. doi: 10.1016/j.freeradbiomed.2004.06.043
Richier, S., Furla, P., Plantivaux, A., Merle, P.-L., and Allemand, D. (2005). Symbiosis-induced adaptation to oxidative stress. J. Exp. Biol. 208, 277–285. doi: 10.1242/jeb.01368
Richier, S., Merle, P.-L., Furla, P., Pigozzi, D., Sola, F., and Allemand, D. (2003). Characterization of superoxide dismutases in anoxia- and hyperoxia-tolerant symbiotic cnidarians. Biochim. Biophys. Acta Gen. Subj. 1621, 84–91. doi: 10.1016/S0304-4165(03)00049-7
Richier, S., Sabourault, C., Courtiade, J., Zucchini, N., Allemand, D., and Furla, P. (2006). Oxidative stress and apoptotic events during thermal stress in the symbiotic sea anemone, Anemonia viridis. FEBS J. 273, 4186–4198. doi: 10.1111/j.1742-4658.2006.05414.x
Roberty, S., Fransolet, D., Cardol, P., Plumier, J.-C., and Franck, F. (2015). Imbalance between oxygen photoreduction and antioxidant capacities in Symbiodinium cells exposed to combined heat and high light stress. Coral Reefs 34, 1063–1073. doi: 10.1007/s00338-015-1328-5
Roberty, S., Furla, P., and Plumier, J.-C. (2016). Differential antioxidant response between two Symbiodinium species from contrasting environments. Plant Cell Environ. 39, 2713–2724. doi: 10.1111/pce.12825
Saragosti, E., Tchernov, D., Katsir, A., and Shaked, Y. (2010). Extracellular Production and Degradation of Superoxide in the Coral Stylophora pistillata and Cultured Symbiodinium. PLoS One 5:e12508. doi: 10.1371/journal.pone.0012508
Shaked, Y., and Armoza-Zvuloni, R. (2013). Dynamics of hydrogen peroxide in a coral reef: sources and sinks. J. Geophys. Res. Biogeosci. 118, 1793–1801. doi: 10.1002/2013JG002483
Shick, J. M., and Dykens, J. A. (1985). Oxygen detoxification in algal-invertebrate symbioses from the Great Barrier Reef. Oecologia 66, 33–41. doi: 10.1007/BF00378549
Suggett, D. J., and Smith, D. J. (2020). Coral bleaching patterns are the outcome of complex biological and environmental networking. Glob. Chang. Biol. 26, 68–79. doi: 10.1111/gcb.14871
Suggett, D. J., Warner, M. E., Smith, D. J., Davey, P., Hennige, S., and Baker, N. R. (2008). Photosynthesis and Production of Hydrogen Peroxide by Symbiodinium (Pyrrhophyta) Phylotypes with Different Thermal Tolerances. J. Phycol. 44, 948–956. doi: 10.1111/j.1529-8817.2008.00537.x
Ventura, P., Toullec, G., Fricano, C., Chapron, L., Meunier, V., Röttinger, E., et al. (2018). Cnidarian Primary Cell Culture as a Tool to Investigate the Effect of Thermal Stress at Cellular Level. Mar. Biotechnol. 20, 144–154. doi: 10.1007/s10126-017-9791-3
Wietheger, A., Fisher, P. L., Gould, K. S., and Davy, S. K. (2015). Sensitivity to oxidative stress is not a definite predictor of thermal sensitivity in symbiotic dinoflagellates. Mar. Biol. 162, 2067–2077. doi: 10.1007/s00227-015-2736-3
Xiang, J., Wan, C., Guo, R., and Guo, D. (2016). Is Hydrogen Peroxide a Suitable Apoptosis Inducer for All Cell Types? Biomed. Res. Int. 2016:7343965. doi: 10.1155/2016/7343965
Yakovleva, I., Bhagooli, R., Takemura, A., and Hidaka, M. (2004). Differential susceptibility to oxidative stress of two scleractinian corals: antioxidant functioning of mycosporine-glycine. Comp. Biochem. Physiol. B Biochem. Mol. Biol. 139, 721–730. doi: 10.1016/j.cbpc.2004.08.016
Keywords: Anemonia viridis, in vitro cell cultures, oxidative stress, hydrogen peroxide, cnidarian
Citation: Cotinat P, Fricano C, Toullec G, Röttinger E, Barnay-Verdier S and Furla P (2022) Intrinsically High Capacity of Animal Cells From a Symbiotic Cnidarian to Deal With Pro-Oxidative Conditions. Front. Physiol. 13:819111. doi: 10.3389/fphys.2022.819111
Received: 20 November 2021; Accepted: 10 January 2022;
Published: 10 February 2022.
Edited by:
Davide Seveso, University of Milano-Bicocca, ItalyReviewed by:
Tingting Xiang, University of North Carolina at Charlotte, United StatesJohn Robert Finnerty, Boston University, United States
Copyright © 2022 Cotinat, Fricano, Toullec, Röttinger, Barnay-Verdier and Furla. This is an open-access article distributed under the terms of the Creative Commons Attribution License (CC BY). The use, distribution or reproduction in other forums is permitted, provided the original author(s) and the copyright owner(s) are credited and that the original publication in this journal is cited, in accordance with accepted academic practice. No use, distribution or reproduction is permitted which does not comply with these terms.
*Correspondence: Paola Furla, cGFvbGEuZnVybGFAdW5pY2UuZnI=
†Present address: Gaëlle Toullec, Laboratory for Biological Geochemistry, School of Architecture, Civil and Environmental Engineering, Ecole Polytechnique Fédérale de Lausanne, Lausanne, Switzerland
‡These authors have contributed equally to this work
§These authors share senior authorship