- 1Unit of Histology and Medical Embryology, Department of Anatomy, Histology, Forensic Medicine and Orthopedics, Sapienza University of Rome, Rome, Italy
- 2Biological Adaptation and Ageing, Institut de Biologie Paris-Seine, Sorbonne Université, Paris, France
- 3Institute of Nanotechnology (Nanotec), National Research Council, Rome, Italy
Skeletal muscle plays a major role in controlling body mass and metabolism: it is the most abundant tissue of the body and a major source of humoral factors; in addition, it is primarily responsible for glucose uptake and storage, as well as for protein metabolism. Muscle acts as a metabolic hub, in a crosstalk with other organs and tissues, such as the liver, the brain, and fat tissue. Cytokines, adipokines, and myokines are pivotal mediators of such crosstalk. Many of these circulating factors modulate histone deacetylase (HDAC) expression and/or activity. HDACs form a numerous family of enzymes, divided into four classes based on their homology to their orthologs in yeast. Eleven family members are considered classic HDACs, with a highly conserved deacetylase domain, and fall into Classes I, II, and IV, while class III members are named Sirtuins and are structurally and mechanistically distinct from the members of the other classes. HDACs are key regulators of skeletal muscle metabolism, both in physiological conditions and following metabolic stress, participating in the highly dynamic adaptative responses of the muscle to external stimuli. In turn, HDAC expression and activity are closely regulated by the metabolic demands of the skeletal muscle. For instance, NAD+ levels link Class III (Sirtuin) enzymatic activity to the energy status of the cell, and starvation or exercise affect Class II HDAC stability and intracellular localization. SUMOylation or phosphorylation of Class II HDACs are modulated by circulating factors, thus establishing a bidirectional link between HDAC activity and endocrine, paracrine, and autocrine factors. Indeed, besides being targets of adipo-myokines, HDACs affect the synthesis of myokines by skeletal muscle, altering the composition of the humoral milieu and ultimately contributing to the muscle functioning as an endocrine organ. In this review, we discuss recent findings on the interplay between HDACs and circulating factors, in relation to skeletal muscle metabolism and its adaptative response to energy demand. We believe that enhancing knowledge on the specific functions of HDACs may have clinical implications leading to the use of improved HDAC inhibitors for the treatment of metabolic syndromes or aging.
Introduction
Skeletal muscle significantly affects body mass and energy consumption, being characterized by a highly dynamic response to energy demand (Baskin et al., 2015). Indeed, skeletal muscle is the most significant metabolic organ, accounting for up to 30% of resting energy expenditure (Zurlo et al., 1990). Considered a key site for glucose uptake and storage, and a reservoir of amino acids, skeletal muscle exerts a crucial role in modulating energy and protein metabolism throughout the body (Turner et al., 2014; Ahima and Park, 2015). Adult skeletal muscles have a diverse pattern of myofibers (Staron, 1997): the slow-twitch, or type I, myofibers are characterized by a higher content of mitochondria and have an oxidative metabolism, as opposed to the fast-twitch, or type II, myofibers, which mostly rely on a glycolytic metabolism (Schiaffino and Reggiani, 2011; Talbot and Maves, 2016). Metabolic demand or a disease state both affect the composition of the skeletal muscle fiber type. For instance, insulin resistance correlates with an increase in the anaerobic and glycolytic capacities of muscle, similarly to what happens in obesity and diabetes (Simoneau et al., 1995). On the other hand, age-related sarcopenia is characterized by an increase in the relative amount of slow-twitch fibers and by the atrophy of the fast ones (Gannon et al., 2009; Daou et al., 2020; Berardi et al., 2021).
The mechanisms whereby skeletal muscle mediates metabolic changes in other tissues is a clinically relevant, dynamic avenue of investigation. An increasingly high number of muscle-derived soluble molecules is known to induce a plethora of effects in the whole body and to mediate inter-organ cross-talk (Severinsen and Pedersen, 2020) and numerous studies also reveal that changes in skeletal muscle factors can modulate metabolism systemically (Chen et al., 2010; Correia et al., 2015; Pereira et al., 2017). The expression of many muscle-derived soluble factors is reported as being altered in disease, including in cancer, type 2 diabetes, obesity, or heart failure (Liu et al., 2015; Berezin et al., 2021; Evans et al., 2021; Pin et al., 2021), suggesting the importance of these factors in the crosstalk between muscle and other organs/tissues in pathological conditions. However, the metabolic adaptations of the skeletal muscle, up to now often merely seen as a consequence of a disease state, may be exploited in a pro-active way to treat metabolic disorders. In this scenario, exercise training as a preventive and therapeutic strategy in several diseases has already been proposed (Leal et al., 2018; de Lima et al., 2020; Severinsen and Pedersen, 2020). Indeed, muscle contraction represents a major physiological stimulus to activate the release of a variety of soluble factors, in a time- and intensity-dependent fashion (Hutchinson et al., 2019; Kurgan et al., 2019; Piccirillo, 2019; Florin et al., 2020).
Histone deacetylases (HDACs) work as epigenetic factors by repressing gene transcription through the removal of acetyl groups from the tails of histone proteins or, alternatively, modulate various cellular responses through their deacetylase activity on non-histone proteins (Glozak et al., 2005; Hyndman and Knepper, 2017). We find it intriguing that HDACs modify gene expression in the skeletal muscle tissue in response to humoral factors, and that, in turn, several HDACs modulate the expression of soluble factors, ultimately affecting their levels in the circulation. The fact that the genetic expression of secreted products is regulated by epigenetic factors adds up to the complexity of the regulatory network controlling myokine production. Also, that multiple tissues are responsible for the coordinated production of the same endocrine factors, such as certain adipo-myokines, highlights this phenomenon in all its complexity.
In this review article, first we introduce HDACs (paragraph 2) and their key role in skeletal muscle metabolic responses (paragraph 3), then we review the most recent findings on how myokines directly or indirectly regulate metabolic reprogramming (paragraph 4): in particular, we focus on those myokines that affect HDAC expression and activity (paragraph 5) and, vice-versa, on those HDACs that affect myokine synthesis and release (paragraph 6); lastly, we conclude with the clinical implications of the use of HDAC inhibitors (paragraph 7).
The Histone Deacetylases and Their Classification
Chromatin is a highly organized nucleoprotein complex, mainly composed by DNA and histone proteins that form nucleosomes. The DNA transcription rate depends, among other factors, on changes in the structure of the nucleosomes. Highly compact and dense chromatin, known as heterochromatin, is associated with the repression of transcriptional activity; whereas open chromatin, or euchromatin, is associated with transcriptional activation. The packaging of chromatin depends mainly on modifications, including acetylation, of the N-terminal histone tails, which protrude from the nucleosome. Histone acetylation levels are regulated through the interplay between two families of enzymes, namely histone acetyltransferases (HATs) and HDACs, and chromatin (Peserico and Simone, 2011). The deacetylation of histones increases their positive charge and consequently their affinity to the DNA, inducing the formation of a compacted, transcriptionally repressed chromatin structure. However, HDACs also target non-histone proteins and finely tune their activity and function by means of deacetylation (Bannister and Kouzarides, 2011; Peserico and Simone, 2011; Hyndman and Knepper, 2017).
To date, 18 different mammalian HDACs have been identified and divided into four classes according to their sequence similarity to yeast counterparts (Seto and Yoshida, 2014; Park and Kim, 2020): Class I HDACs (HDAC1, 2, 3, and 8), Class II HDACs (HDAC4, 5, 6, 7, 9, and 10), Class III HDACs (Sirtuins), which differ from the others because they use NAD+ as a cofactor rather than Zn2+, and Class IV HDACs (HDAC11). Class II HDACs is further divided into two subgroups, Class IIa (HDAC4, 5, 7, and 9) and Class IIb HDACs (HDAC6 and 10), which differ significantly in many biological aspects, from subcellular localization to their involvement in diseases. Whereas most Class I HDACs are ubiquitously expressed and localized in the nucleus, the Class II HDACs are characterized by tissue-specific expression and stimulus-dependent nucleus-to-cytoplasm shuttling (Haberland et al., 2009). Class III HDACs, which are ubiquitously expressed in human tissues (Frye, 1999), have differential subcellular localizations: SIRT1, SIRT6, and SIRT7 are primarily detected in the nucleus, SIRT2 is mainly found in the cytosol, while SIRT3, SIRT4, and SIRT5 are present exclusively in mitochondria (Michishita et al., 2005). HDAC11, the only member of Class IV, seems to be mainly expressed in the kidney, heart, brain, skeletal muscle, and testis, and is localized in the nucleus of the cell (Michishita et al., 2005).
Histone deacetylases control essential phenomena such as cell cycle progression, cell survival and differentiation (Moresi et al., 2016). Consistently, HDAC deregulation plays a key role in many pathologies, including cancer, neurological, inflammatory and cardiac diseases, as well as metabolic and neuromuscular disorders (Haberland et al., 2009; Volmar and Wahlestedt, 2015; Li and Seto, 2016; Pigna et al., 2019). The involvement of HDACs in the regulation of skeletal muscle metabolism is highlighted below.
Histone Deacetylases Are Key Regulators of Skeletal Muscle Metabolism
The interplay between epigenetics and skeletal muscle metabolism has been thoroughly described (Sharples et al., 2016; Tzika et al., 2018; Jacques et al., 2019). In particular, HDAC activity orchestrates the metabolic reprogramming of skeletal muscle in response to physiological challenges and in several disease states (Howlett and McGee, 2016; Bianchi et al., 2017; Astratenkova and Rogozkin, 2019).
The role of HDACs in muscle metabolism has been extensively studied over the years. Early evidence revealed that Class IIa HDACs have a main role in regulating myofiber identity in physiological conditions. Indeed, Class IIa HDACs associate with MEF2 transcription factors ultimately repressing their transcriptional activity thereby regulating the metabolism of oxidative, slow-twitch myofibers (Wu et al., 2000; Potthoff et al., 2007). In particular, HDAC5 directly binds to the promoter region of GLUT4, repressing the transcription of this gene. This ultimately leads to the shift of muscle fibers toward oxidative metabolism promoting their glucose uptake (McGee et al., 2008).
The Class I members HDAC1 and HDAC2 are essential to skeletal muscle metabolism and homeostasis in physiological conditions. The deletion of HDAC1 and HDAC2 in skeletal muscle induces a shift toward a more oxidative metabolism and higher energy expenditure, caused by defects in the autophagy flux (Moresi et al., 2012). More recently, an important role in muscle energy metabolism was demonstrated for HDAC3 by means of its muscle-specific deletion: this causes an insulin resistance associated with higher endurance and fatigue resistance, due to a decrease in the uptake of glucose and an increase in the consumption of lipids (Hong et al., 2017; Song et al., 2019). The involvement of Class I HDACs in skeletal muscle metabolism was further demonstrated through the pharmacological inhibition of this class (Galmozzi et al., 2013), validating previous findings obtained through genetic approaches.
Being nicotinamide adenine dinucleotide (NAD+)-dependent enzymes, Sirtuins control gene transcription in response to changes in nutrient availability and energy demand. Indeed, an energy deficit increases the cellular NAD+/NADH ratio, thus activating Sirtuins which promote mitochondrial biogenesis and fatty acid oxidation in skeletal muscle, by deacetylating both histone and non-histone proteins (Houtkooper et al., 2012), as a response to the low energy condition (Ryall, 2012). It is worth noting that the deletion of SIRT1 in mice has modest effects on skeletal muscle metabolism (Menzies et al., 2013), implying a biological redundancy of this adaptative response.
In addition to regulating metabolic homeostasis in the whole body, by controlling insulin sensitivity and glucose tolerance (Bhaskara, 2018), the Class IV member HDAC11 has been recently reported to play a crucial role in skeletal muscle metabolism and function (Hurtado et al., 2021). HDAC11 localizes in muscle mitochondria and its deletion increases mitochondrial content, causing a glycolytic-to-oxidative muscle fiber switch (Hurtado et al., 2021).
Metabolic stresses, such as exercise, obesity, or a high fat diet (HFD), induce skeletal muscle adaptations through HDACs. Exercise modulates skeletal muscle metabolism via epigenetic reprogramming, partially through the phosphorylation-dependent nuclear export of Class II HDACs (McGee and Hargreaves, 2011). In addition, exercise also affects Sirtuin activity, as shown by the fact that a number of histone and non-histone Sirtuin targets are deacetylated during exercise (Cantó et al., 2009). Furthermore, obesity and HFD induce epigenetic reprogramming in skeletal muscle, involving Class I and II HDACs (Berdeaux et al., 2007; Sun et al., 2011; Moresi et al., 2012), although, the underlying mechanisms are not yet fully known.
Given the crucial role of HDACs in the regulation of muscle metabolism in various pathophysiological conditions, it is not surprising that not only HDACs in skeletal muscle are targeted by soluble factors, but also that they regulate the production of myokines affecting other tissues. The role played by myokines and adipo-myokines is outlined in the next paragraph, in which we describe how these factors of muscle and non-muscle origin are able to reprogram the metabolic activity of several tissues, ultimately determining metabolic adaptations in the whole body.
Myokines as Mediators of Metabolic Reprogramming
Skeletal muscle is an important source of secreted factors exerting autocrine, paracrine and endocrine effects (Di Felice et al., 2020; Florin et al., 2020). This skeletal muscle secretome varies in response to ever-changing metabolic demand, dependent on variable physiological and pathological conditions (Lecompte et al., 2017; Florin et al., 2020). Among the numerous circulating factors involved in the regulation of systemic metabolism we included in our overview those that are expressed in the skeletal muscle and also have a proved link to HDACs.
Myostatin was the first characterized skeletal muscle-derived circulating protein (McPherron et al., 1997), originally identified in mice as a regulator of muscle growth via a negative feedback. Further studies clarified that myostatin targets other tissues in addition to muscle. For instance, myostatin is involved in the crosstalk between skeletal muscle and adipose tissue, thereby modulating insulin sensitivity and fat accumulation, both in animals and humans (Argilés et al., 2012). Moreover, myostatin targets bone, affecting osteogenic differentiation and enhancing resorption, modulating overall bone mass (Hamrick et al., 2006, 2007). Follistatin is a myostatin-binding protein and an inhibitor of myostatin activity, capable of promoting muscle growth (Yakabe et al., 2020). In humans, following exercise circulating follistatin increases and targets several organs, regulating pancreatic cell function and survival and promoting insulin resistance in skeletal muscle and adipose tissue (Willis et al., 2019). In this regard, we have shown that the mechanical stretch of murine myotubes per se alters the amount of myokines produced by muscle cells in vitro, including follistatin (Baccam et al., 2019).
In the search for a consensus term to define peptides produced and released by skeletal muscle, the word “myokine” referring to Interleukin 6 (IL-6) was first introduced in 2003 (Pedersen et al., 2003). Ten years later, the term “adipo-myokine” was coined to indicate those myokines, such as IL-6, that are also expressed by adipose tissue (Raschke and Eckel, 2013). In humans, the level of circulating IL-6 is increased after exercise (Northoff and Berg, 1991), mainly due to its secretion by skeletal muscle (Steensberg et al., 2000). Studies conducted in mice and humans highlighted that in response to physical exercise IL-6 exerts a positive role in modulating muscle mass, insulin secretion and lipid metabolism (Rosendal et al., 2005; Serrano et al., 2008; Ellingsgaard et al., 2011; da Silva Vasconcelos and Fernanda Salla, 2018). In addition, IL-6 produces anti-inflammatory effects, inhibiting the expression of Tumor Necrosis Factor-α (TNFα) and Interleukin-1 (IL-1) (Pedersen et al., 2003). However, besides the above mentioned positive effects, other studies highlighted the pleiotropic functions of this factor and revealed the deleterious effect of IL-6 on muscle homeostasis, showing that it is also a promoter of muscle wasting (Moresi et al., 2019). Indeed, IL-6 overexpression induces muscle atrophy in transgenic mice (Tsujinaka et al., 1996) and perturbs redox homeostasis (Forcina et al., 2019). Consistently, while the acute induction of IL-6 promotes muscle growth, sustained and elevated levels of IL-6 have been associated with muscle wasting in several human catabolic conditions (Yudkin et al., 2000; Kern et al., 2001; Pradhan et al., 2001; Ershler and Keller, 2003). All of the above shows how important it is to consider the complexity of a system where the kinetics and the source tissue of a given factor significantly influence its effects. To this regard IL-6 is emblematic and all these aspects should be taken into account when discussing the possible clinical implications of adipo-myokines.
Metrnl and BAIBA are two myokines whose expression is regulated by the transcriptional factor peroxisome proliferator-activated receptor-gamma coactivator-1α (PGC-1α) (Boström et al., 2012; Rao et al., 2014) and induced upon exercise in murine and human skeletal muscle (Rao et al., 2014; Roberts et al., 2014). These myokines target many tissues, exerting numerous physiological functions, such as promoting neural and osteocyte differentiation, preserving pancreatic β-cell functions and browning white adipose tissue (WAT), in addition to regulating glucose uptake and energy expenditure in skeletal muscle (Zheng et al., 2016; Darvin et al., 2018; Tanianskii et al., 2019; Rabiee et al., 2020).
Among other myokines, irisin production (Maak et al., 2021) has been shown to be induced by exercise in mice and humans (Boström et al., 2012; Jedrychowski et al., 2015). Studies with gain- (Boström et al., 2012) and loss- (Xiong et al., 2019) of function approaches demonstrate the ability of irisin to mediate the positive effects of physical activity in mice. These effects were not confirmed in humans (Maak et al., 2021).
The role of irisin, or even its existence in humans (Albrecht et al., 2015), is still debated, mainly in relation to: (1) the accuracy and reliability of the methods used to detect circulating irisin levels (Albrecht et al., 2015; Jedrychowski et al., 2015; Pourteymour et al., 2017; Bretland et al., 2021); (2) the positive effects of irisin on target organs or tissues (Raschke et al., 2013; Elsen et al., 2014; Maak et al., 2021). The debate on irisin exemplifies how difficult it is to study the crosstalk between tissues.
Metabolic stresses, such as acute bouts of exercise, fasting and caloric restriction, induce the expression of ANGPTL4 in human skeletal muscle (Catoire et al., 2014), likely by increasing plasma free-fatty acid levels and activating the peroxisome proliferator-activated receptor-δ (PPAR-δ) (Staiger et al., 2009). ANGPTL4 stimulates lipolysis in WAT, determining a shift from fat storage to fat release, and triggers AMP-activated protein kinase (AMPK) in skeletal muscle, thereby mediating an increase in its mitochondrial oxidative capacity and ATP production (Norheim et al., 2014; Chang et al., 2018; Li et al., 2020). It should be noted that besides skeletal muscle, adipose tissue and the liver express higher levels of ANGPTL4 following exercise, likely contributing even more than skeletal muscle to ANGPTL4 exercise-mediated effects (Norheim et al., 2014). Interestingly, human studies showed that ANGPTL4 mRNA in skeletal muscle is lower in men than in women at basal conditions, probably due to differences in the utilization of glucose and fat mass in the two genders (Barja-Fernandez et al., 2018; Li et al., 2020). Moreover, a positive correlation between muscle ANGPTL4, obesity and glucose metabolism has been reported: indeed, ANGPTL4 muscle and serum levels are significantly higher in obese patients with an abnormal glucose tolerance (Barja-Fernandez et al., 2018; Li et al., 2020).
Fibroblast growth factor 21 targets several organs including: (1) the liver, where it activates important metabolic responses, such as the induction of hepatic fatty acid oxidation, ketogenesis, and gluconeogenesis; (2) the pancreas, where it is necessary to maintain the digestive and endocrine functions of this organ; (3) WAT, where it promotes insulin sensitivity, glucose uptake, fatty acid storage, and oxidative capacity (Cuevas-Ramos et al., 2019; Martínez-Garza et al., 2019; Keinicke et al., 2020; Watanabe et al., 2020; Xie and Leung, 2020). Several cellular stress-signals, such as autophagy impairment, mitochondrial dysfunction and ER stress, induce FGF21 release from murine skeletal muscle (Kim and Lee, 2014; Pereira et al., 2017; Oost et al., 2019). All these induce FGF21 expression via the activation of transcription factor 4 (ATF4) (Keipert et al., 2014; Miyake et al., 2016). In addition, both mammalian target of rapamycin (mTOR) complex 1 (mTORC1) and mTORC2 have been reported to induce FGF21 expression in murine skeletal muscle (Guridi et al., 2015; Pereira et al., 2017). Furthermore, the secretion of FGF21 from human skeletal muscle is strongly induced upon exercise or in pathophysiological conditions including in mitochondrial myopathies and aging, suggesting the involvement of muscle-derived FGF21 in both healthy and pathological conditions (Hanks et al., 2015; Tanimura et al., 2016; Villarroya et al., 2018; Tezze et al., 2019). Despite its beneficial effect on body metabolism, the therapeutic role of FGF21 to the advantage of human health is still under debate, due to its paradoxical effects on different tissues. Indeed, FGF21 has negative effects on bone mass and mineral density both in mice and humans (Wei et al., 2012; Fazeli et al., 2015). Moreover, the long-term increase of circulating FGF21, triggered in skeletal muscle by stress conditions, contributes to systemic inflammation, precocious senescence, and premature death in mice (Tezze et al., 2017). Additional studies are required to clarify the specific role of FGF21 secreted from other organs and its effect on the whole body under physiological and pathological conditions.
Data available on rodents and humans highlight a rapid increase of IL-15 in circulation in response to exercise, improving whole body insulin sensitivity and decreasing adiposity (Carbó et al., 2001; Nadeau and Aguer, 2019). The data regarding IL-15 expression and release from skeletal muscle upon exercise in humans are controversial, probably due to the use of different exercise protocols in different studies or to differences in the subjects analyzed (Nielsen et al., 2007). In rats, autocrine IL-15 release induces a shift toward an oxidative skeletal muscle phenotype through the induction of PPAR-d and PGC-1α (Carbó et al., 2001; Busquets et al., 2006; Pistilli and Quinn, 2013), contributing to the beneficial adaptations to exercise of the metabolism (Hennigar et al., 2017). IL-15 also inhibits muscle protein degradation (Pistilli et al., 2007), however, since its levels decline progressively with aging, this contributes to age-related sarcopenia (Quinn, 2008; Quinn et al., 2010). In addition, IL-15 directly targets adipose tissue modulating the WAT mass and lipid metabolism probably because it decreases lipoprotein lipase (LPL) and leptin activities (Alvarez et al., 2002). A positive effect of a greater amount of circulating IL-15 on bone mineral content has been reported, likely due to the ability of IL-15 to interfere with TNF-α signaling (Quinn et al., 2009).
The expression of myonectin in murine skeletal muscle is regulated by the availability of nutrients and by exercise (Seldin et al., 2012; Laurens et al., 2020). In addition, patients with type 2 diabetes mellitus or those affected by obesity exhibit higher levels of circulating myonectin compared to healthy individuals (Li et al., 2018). Circulating myonectin has been shown to decrease the levels of circulating non-esterified fatty acid, through the promotion of fatty acid uptake both in hepatocytes and in adipocytes (Seldin et al., 2012). Moreover, the exercise-induced myonectin release from skeletal muscle protects cardiac myocytes from apoptosis, leading to a reduction of acute myocardial ischemia-reperfusion injury in mice (Otaka et al., 2017, 2018). Taken together, these data underline the role of myonectin in regulating systemic fatty acid metabolism and in mediating a cross-talk between muscle and other metabolic compartments (Seldin et al., 2012), suggesting possible therapeutic applications.
Brain-derived neurotrophic factor is a member of the neurotrophic factor family, whose expression is increased in human and rat skeletal muscles in response to muscle contraction (Dupont-Versteegden et al., 2004; Matthews et al., 2009). In human skeletal muscle, BDNF activates AMPK and promotes lipid oxidation following exercise (Matthews et al., 2009). In addition to skeletal muscle, exercise greatly increases the expression and release of BDNF from the brain, in both humans and mice (Liu and Nusslock, 2018), which, most likely, accounts for most of the BDNF-mediated effects, since the brain is the major source of this soluble factor.
The aforementioned studies taken together indicate that major metabolic adaptations to physiological or pathological alterations are regulated by soluble factors, mediating a complex crosstalk involving a network of tissues. Many of these circulating factors involve changes in HDAC expression or activation in skeletal muscle. The next paragraph focuses on this aspect.
Myokines Affect Histone Deacetylase Expression and Activity
Myokines and other soluble factors control tissue homeostasis through a variety of signaling pathways including the regulation of HDAC expression or activity (Table 1). For instance, a correlation between the exercise-induced hormone irisin, known to regulate energy metabolism and to protect against numerous diseases, and HDAC4 has already been proposed. In rodents irisin protects the cardiomyocytes of rats exposed to hypoxic stress (Zhao et al., 2016) and the myocardium of type II diabetic db/db mice (Zhao et al., 2016; Wang et al., 2020). This protective role of irisin correlates with the degradation of HDAC4 (Zhao et al., 2016; Wang et al., 2020), which causes cell death and mitochondrial dysfunction in these cells. These findings highlight the principle, in rodents at least, that a systemic factor, namely irisin, produces its effects partially involving epigenetic mechanisms. Since the relevance of irisin-mediated effects in humans is still debated, more studies are needed to clarify whether the protective role of irisin on muscle in our species exists, and whether it involves epigenetic mechanisms.
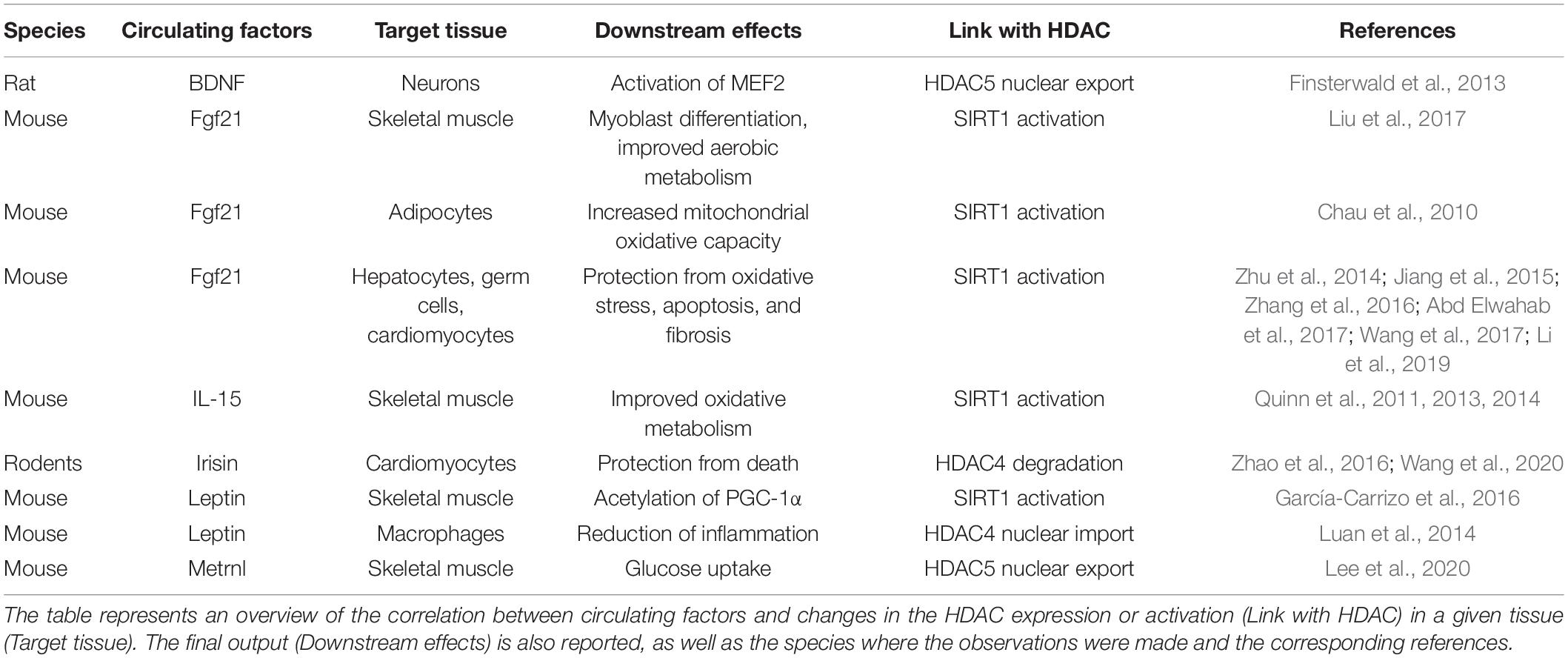
Table 1. Circulating factors affect histone deacetylase (HDAC) expression or activity in various tissues.
The secretion of Metrnl by skeletal muscle, increased during muscle contractions, improves glucose tolerance by inducing glucose uptake via the AMPK-dependent phosphorylation of HDAC5, a transcriptional repressor of Glut4 in mice (Lee et al., 2020): the phosphorylated HDAC5 interacts with 14-3-3 proteins, is sequestrated in the cytoplasm, and results in the activation of Glut4 transcription (Figure 1). Glucose uptake by the musculature represents a paradigmatic behavior, which usually occurs in response to the classic insulin signaling. The interesting aspect of the alternative Metrnl signaling stems from the fact that the upregulation of the Glut4 gene involves an energy-sensing factor, AMPK, as well as an epigenetic factor, HDAC5. In addition, the delivery of recombinant Metrnl improves glucose tolerance in mice with HFD-induced obesity or type 2 diabetes (Lee et al., 2020), suggesting the potential use of this myokine as a therapeutic tool to treat metabolic disorders.
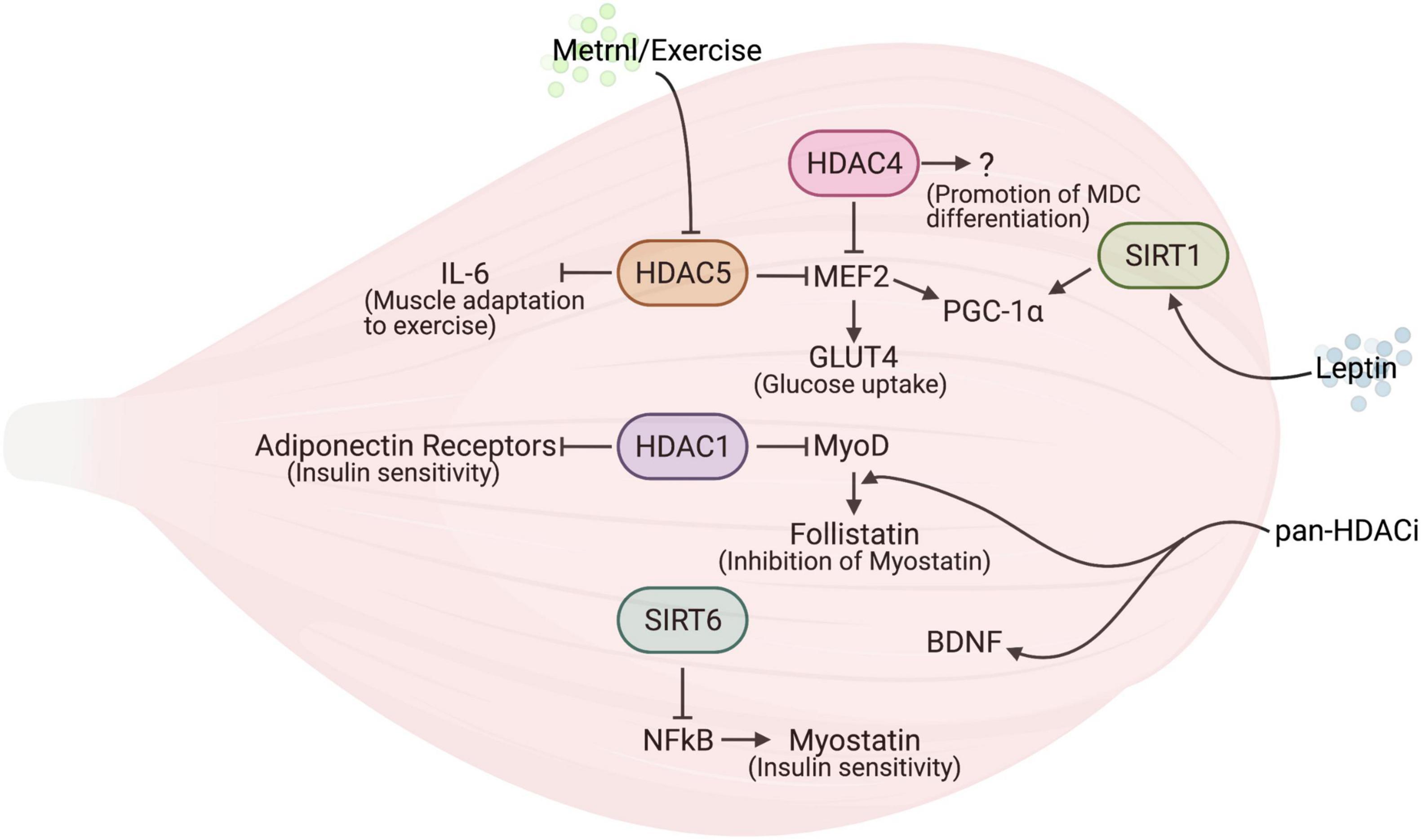
Figure 1. The interplay between histone deacetylases (HDACs) and myokines in skeletal muscle. The reciprocal regulation of HDACs and myokines modulates skeletal muscle metabolism.
Fibroblast growth factor 21 activates a SIRT1-AMPK-PGC1α pathway in skeletal muscle, thereby promoting myoblast differentiation and aerobic metabolism, and is also possibly involved in starvation-induced muscle atrophy (Liu et al., 2017). In adipocytes the same axis has been proven to be responsible for increasing mitochondrial oxidative capacity (Chau et al., 2010). Additionally, the protective role of the FGF21-SIRT1 axis in different pathological conditions has been reported in multiple cell types, suggesting that this pathway may be a potential target for therapeutic approaches. Indeed, FGF21 activates SIRT1 in hepatocytes, thereby ameliorating multiple metabolic parameters in alcoholic fatty liver disease (Zhu et al., 2014) or cafeteria-diet induced steatohepatitis (Abd Elwahab et al., 2017). In addition, FGF21 also activates SIRT1 in germ cells reducing their oxidative stress and apoptosis in diabetes (Jiang et al., 2015). A similar TGF21-induced SIRT1 activation in cardiomyocytes has a protective effect on type 1 diabetes-induced inflammation and fibrosis in the heart (Zhang et al., 2016), on doxorubicin-induced cardiomyopathy (Wang et al., 2017) as well as on angiotensin-II induced cardiomyocyte apoptosis and cardiac hypertrophy (Li et al., 2019).
Interleukin 15, a myokine which contributes to the benefits of physical exercise on muscle metabolism, is responsible for the post-exercise induction of SIRT1 in muscle, which, in turn, mediates many of the metabolic effects of physical exercise (Quinn et al., 2013, 2014). IL-15 Tg mice also exhibit an induction of the SIRT1 protein and changes in oxidative metabolism, which may be involved in their phenotype, i.e., the resistance to diet-induced obesity and higher insulin-sensitivity (Quinn et al., 2011).
Differently from FGF21 and IL-15, ANGPTL4 represses Sirt1 expression in hepatocytes, thus mediating the inflammatory response in acute lung injury (Guo et al., 2015).
Leptin has been shown to target multiple tissues, including skeletal muscle. Evidence of a direct effect of leptin on muscle cells comes from in vitro experiments showing that, in murine myotubes, leptin induces the expression of genes involved in metabolism (including PGC-1α, uncoupling protein 3, muscle carnitine palmitoyltransferase 1) in addition to that of myokines such as IL-6 and IL-15 (Nozhenko et al., 2015). Interestingly, leptin affects SIRT1-mediated PGC-1α deacetylation (García-Carrizo et al., 2016; Figure 1), even though contradictory data have been reported. This is probably due to dissimilar leptin and nutrient concentrations in the culture media, as well as to different timing of the analyses. In vivo, leptin receptor deficiency in skeletal muscle leads to muscle atrophy and compromised primary myoblast proliferation and differentiation (Arounleut et al., 2013), indicating a possible role for this adipokine in skeletal muscle homeostasis. Whether or not leptin influences adult skeletal muscle via modulating HDACs has not been investigated yet. However, an initial link between leptin and HDACs has been reported: indeed, the administration of leptin in conjunction with a HFD induces HDAC4 dephosphorylation and its nuclear import in macrophages in white adipose tissue, thereby reducing inflammation (Luan et al., 2014). The molecular mechanism described for leptin-induced HDAC dephosphorylation consists in a catecholamine-dependent increase in cAMP, triggered by leptin, which inhibits salt-inducible kinases. This ultimately promotes HDAC4 dephosphorylation; the dephosphorylated HDAC4 then shuttles to the nucleus where it inhibits NF-κB transcriptional activity on pro-inflammatory genes (Luan et al., 2014).
Although experimental evidence in skeletal muscle cells is still lacking, it is interesting to note that BDNF activates the salt-inducible kinase 1 (SIK1), a member of the AMPK family, in rat neurons, leading to the phosphorylation-dependent nuclear extrusion of HDAC5 and the consequent MEF2-dependent gene activation (Finsterwald et al., 2013). It would be important to investigate this signaling in skeletal muscles as well, since it may underly the beneficial effects of BDNF on muscle metabolism.
So far, we have reported that myokines control the metabolic shifts occurring in multiple tissues in response to physiological or pathological stress conditions, and that, at least in muscle tissues, myokines are capable of inducing epigenetic responses through HDACs. Conversely, HDACs influence the release of several factors from skeletal muscle, as outlined next.
Histone Deacetylases Affect Myokine Synthesis
Histone deacetylases regulate the expression of chemokines, cytokines and adipo-myokines, or that of their receptors, under physiological and pathological conditions in several tissues (Gatla et al., 2019). In particular, recent studies highlighted this role of HDACs in skeletal muscle—and in other cells to a lesser extent (Table 2). A paramount example of this is the fact that HDAC4 regulates factors secreted by skeletal muscle upon injury, thus regulating muscle-derived cell (MDC) myogenic potential and muscle regeneration (Renzini et al., 2018). Indeed, the deletion of HDAC4 in skeletal muscle hampers skeletal muscle regeneration, even though MDCs from HDAC4 KO mice differentiate well when cultured in vitro, i.e., isolated from their muscle niche. Conversely, sera from injured HDAC4 KO mice negatively affect MDC differentiation, which proves that HDAC4 affects the release of soluble factors from skeletal muscle upon injury, which, in turn, are important for muscle regeneration (Renzini et al., 2018; Figure 1). The characterization of the secretome of HDAC4 KO will clarify this new important function of HDAC4 in skeletal muscle and provide new insights for a potential therapeutic application to muscle regeneration.
Myostatin is a member of the transforming growth factor-beta (TGF-beta) superfamily, expressed and released by skeletal muscle and that negatively influences muscle growth (McPherron et al., 1997). Myostatin expression is controlled by SIRT6, which regulates the binding of NF-kB to the myostatin promoter (Samant et al., 2017; Figure 1). Indeed, SIRT6 KO mice display muscle degeneration and atrophy, in addition to reduced fat and bone density. Conversely, the SIRT6 overexpression counteracts cytokine-induced myostatin expression in muscle cells, promoting myogenesis. Therefore, increasing SIRT6 activity or expression has been proposed as a potential therapeutic tool to counteract many disease states characterized by muscle cachexia, thanks to the ability of SIRT6 to influence myostatin expression and possibly act on multiple tissues.
Follistatin is a secreted protein able to bind with and neutralize the actions of many members of the TGF-beta family of proteins (Patel, 1998). General Pan-HDAC inhibitors (HDACi), such as valproic acid (VPA) or Trichostatin-A (TSA), have been shown to increase the expression of follistatin in muscle cells, through the binding of MyoD to the follistatin promoter, a process which is fostered by local hyperacetylation (Figure 1); in turn, the release of the soluble factor follistatin counteracts myostatin activity, enhancing myogenesis (Iezzi et al., 2004). Further studies clarified that pan-HDACi improve muscle regeneration in dystrophy by increasing the expression of follistatin in both muscle stem cells and fibro-adipogenic progenitors (Minetti et al., 2006; Mozzetta et al., 2013)—a finding that led scientists to suggest the use of HDACi in clinical trials for the treatment of Duchenne Muscular Dystrophy. Although pan-HDACi increase the expression of follistatin in cancer cachexia as well, this is not sufficient to prevent muscle wasting in this disease state (Bonetto et al., 2009). Also, pan-HDACi have been shown to increase BDNF expression in the skeletal muscles and spinal cord in a murine model of Spinal Muscular Atrophy (SMA) (Avila et al., 2007; Figure 1). Since BDNF is an important contraction-induced secreted factor for the neurogenesis, growth, and survival of neurons (Murawska-Ciałowicz et al., 2021), its increase may explain the beneficial effects of HDACi on SMA progression.
Interleukin 6 was recognized in the past as a principal mediator of muscle adaptation to exercise (Ostrowski et al., 1998). Muscle contractions induce an increase of IL-6 gene expression that leads to a release of IL-6 protein in the circulation (Reihmane and Dela, 2013). An epigenetic modulation of IL-6 expression driven by HDAC5 has been recently reported in exercised skeletal muscle. Genetic murine models helped clarify the metabolic changes, including that of glucose metabolism, regulated by the HDAC5 activity on the IL-6 promoter (Klymenko et al., 2020): in resting conditions, HDAC5 negatively modulates IL-6 transcription by removing histone H3 acetylation at lysine 9 (AcH3K9), while upon exercise, HDAC5 is exported to the cytoplasm and results in an increased expression of GLUT4, thus prompting skeletal muscle cells to uptake glucose in response to insulin (Klymenko et al., 2020; Figure 1).
Histone deacetylases regulate ANGPTL4 expression in different cell types. ANGPTL4 is a secreted glycoprotein which inhibits the activity of lipoprotein lipases, thereby increasing circulating triglyceride levels (Fernández-Hernando and Suárez, 2020). HDACi sodium butyrate activates lipolysis in pigs, in part by downregulating ANGPTL4 gene expression in the adipose tissue (Zhang et al., 2021). In human cells, HDAC3 mediates a NCOR-dependent transcriptional repression of the ANGPTL4 gene, as shown by using a renal cancer cell line, while additional investigations are needed to unveil the potential role of HDAC in regulating ANGPTL4 expression in skeletal muscle.
Different HDACs exert opposite effects on the expression of Fgf21, an important regulator of carbohydrate and lipid metabolism, in different cell types. HDAC2 and HDAC3 inhibit Fgf21 expression in glia cells, thus regulating the outgrowth of their processes (Leng et al., 2016), and in adipocytes, affecting fatty acid utilization and ketogenesis (Li et al., 2012). On the contrary, SIRT1 induces Fgf21 expression in hepatocytes, preventing fat-induced liver steatosis while promoting white adipose tissue browning and higher energy expenditure (Li et al., 2014). SIRT1 also induces Fgf21 expression in the heart, improving energy metabolism and increasing the contractile efficiency of pressure-overloaded hearts (Furukawa et al., 2020).
A correlation between SIRT1 expression and irisin serum levels has been reported in type 2 diabetic patients and mice (Safarpour et al., 2020; Jiang et al., 2021), suggesting the involvement of SIRT1 in PGC-1α-mediated irisin release. The treatment of obese type 2 diabetes patients with vitamin D also induces higher serum levels of SIRT1 and irisin, further pointing to the SIRT1-mediated release of irisin (Safarpour et al., 2020). The SIRT1 activator resveratrol, alone or in combination with all-trans retinoic acid (ATRA), significantly induces the expression of irisin in skeletal muscle cells (RongXia et al., 2017; Abedi-Taleb et al., 2019). Consistently, metformin, another compound activating SIRT1, increases irisin expression and its release from skeletal muscle in diabetic mice (Yang et al., 2015). In spite of the limited evidence to date, it seems likely that the positive effects of SIRT1 activation on body metabolism (Feige et al., 2008) are at least in part due to its ability to increase the levels of circulating irisin.
In skeletal muscle HDACs regulate signaling in response to adiponectin. This is an adipokine involved in controlling glucose levels and fatty acid breakdown (Yamauchi et al., 2001), which, interestingly, also plays a role in skeletal muscle (Fruebis, 2001). Here HDAC1 represses the transcription of the genes encoding adiponectin receptors and uncoupling protein 2 and 3. Since the latter are important mitochondrial proteins involved in heat generation and energy expenditure, the inhibition of HDAC1 contributes to the beneficial effects of the pan-HDACi sodium butyrate on HFD-induced obesity in mice (Jian et al., 2016; Figure 1).
Based on the substantial experimental evidence above, it is apparent that myokines, cytokines and adipokines control the homeostatic and metabolic responses of tissues at organismal level, while their dysregulation is responsible for many diseases. Since HDACs affect the synthesis and ultimately the release of these factors in various organs or, vice-versa, modulate the organ response to endocrine factors through the regulation of their receptors, HDACs are very promising pharmaceutical targets. However, the first attempts to interfere with the activity of HDACs by means of HDACi must be further supported by additional investigations on the specific role of each HDAC family member. This approach alone will provide a solid ground for the development of HDACi-based therapies for human diseases. The studies on HDACi performed so far are summarized below.
Clinical Implications of the Use of Histone Deacetylase Inhibitors
Several preclinical studies highlighted the positive effects of HDACi in regulating the whole-body metabolism in various diabetic or insulin-resistance rodent models, thus providing the rationale for the inclusion of HDACi in clinical trials targeting metabolic disorders. For instance, pan-HDACi sodium butyrate and TSA, which improve insulin sensitivity and energy expenditure in HFD-fed mice (Gao et al., 2009; Li et al., 2012; Lin et al., 2012; Henagan et al., 2015; Xu et al., 2020), increase the liver expression of FGF21 and its serum concentration (Li et al., 2012). Consequently, FGF21 mediates the increase in fatty acid utilization and ketogenesis, in response to butyrate treatment, causing resistance to HFD. An added value offered by this study is the fact that it represents an additional proof of principle that many HDACi effects on body metabolism may be achieved through the regulation of soluble factors, such as FGF21 itself. Butyrate improves glucose and pyruvate tolerance in aged mice, reducing fat and preserving skeletal muscle mass (Walsh et al., 2015)—a result that suggests the use of HDACi to counter aging. Butyrate also increases mitochondrial biogenesis and oxygen consumption in the skeletal muscle of aged mice; notably, the use of this HDACi does not affect the HDAC4-myogenin axis, which is significant in neurogenic muscle atrophy (Moresi et al., 2010). In addition, another pan-HDACi, Scriptaid, modulates adaptative metabolic responses to exercise (Gaur et al., 2016). Considering the beneficial effects of exercise on aging, all these findings further suggest new future applications for HDACi in the context of sarcopenia (Pasyukova and Vaiserman, 2017).
In spite of the numerous positive effects that HDACi exert on metabolism control, additional considerations are needed concerning the use of pan-HDACi in clinical trials for metabolic disorders. The use of general HDACi is usually discouraged, since their use is normally associated with numerous side effects and since they target a broad range of proteins. This makes drawing any biological conclusion on the action of specific HDACs difficult. Indeed, contradictory data were reported on the effectiveness of specific Classes of HDACi in ameliorating insulin resistance in obese mice (Sharma and Taliyan, 2016), probably due to the different concentrations used in the various studies or to the fact that Class II enzymatic activity depends on Class I HDACs, which can bias the effects of HDACi (Fischle et al., 2002).
Thus, further investigations should be carried out, both at the pre-clinical and clinical level, before proposing the unspecific pharmacological inhibition of this important enzyme family. The two major avenues of research in this field should be aimed at defining: (1) expression and activity of the different members of the HDAC family, under pathophysiological conditions, and (2) the functions of each single HDAC member in different tissues. Only by undertaking this approach will it be possible to design enzyme- and tissue-specific pharmacological treatments.
Conclusion
Myokines are mediators of the crosstalk between skeletal muscle and other tissues of pivotal importance for body metabolism and they support the central role of skeletal muscle as a metabolic hub. HDACs not only are regulators of muscle metabolism, they also affect other tissues, including liver and fat tissues, by controlling myokine production. The effects of this production can be muscle -centered, as is the case of myostatin and related factors, or have a broader action, such as in the case of pro- and anti-inflammatory cytokines. In turn, HDAC activity is regulated in response to the humoral milieu, a fact which suggests how important epigenetic control is to fine-tune the genetic response of tissues to hormones or other circulating factors. Given the broad and pleiotropic effects of HDACs, these may be targets in the treatment of metabolic and systemic pathological conditions.
Author Contributions
VM conceived the manuscript. AR and MD’O generated the figure. DC supervised the content of the text. All authors contributed to the writing.
Funding
VM and DC are supported by the Fondi Ateneo Sapienza. DC is supported by Emergence SiRIC Curamus 2021.
Conflict of Interest
The authors declare that the research was conducted in the absence of any commercial or financial relationships that could be construed as a potential conflict of interest.
Publisher’s Note
All claims expressed in this article are solely those of the authors and do not necessarily represent those of their affiliated organizations, or those of the publisher, the editors and the reviewers. Any product that may be evaluated in this article, or claim that may be made by its manufacturer, is not guaranteed or endorsed by the publisher.
Acknowledgments
The authors are grateful to Anna Luisa Mazzotti for the editing of the manuscript.
Abbreviations
AcH3K9, Acetyl-Histone H3 (Lys9); AMPK, AMP-activated protein kinase; ANGPTL4, Angiopoietin-like protein 4; ATF4, Activate transcription factor 4; AP-1, Activator protein 1; BAIBA, β-aminoisobutyric acid; BDNF, Brain-derived neurotrophic factor; cAMP, Cyclic adenosine monophosphate; ER, Endoplasmic Reticulum; FGF-21, Fibroblast growth factor 21; GLUT4, Glucose transporter type 4; HAT, Histone Acetyltransferases; HDAC, Histone Deacetylases; HDACi, HDAC inhibitors; HFD, High fat diet; IL-1, Interleukin 1; IL-6, Interleukin 6; IL-15, Interleukin 15; IKK, Nuclear factor-kB Kinase; JNK, c-JunN-terminal kinase; LPL, Lipoprotein lipase; MDC, Muscle-derived cells; MEF2, Myocyte enhancer factor-2; Metrnl, Meteorin-like; mTOR, Mammalian target of rapamycin; MyoD, Myoblast determination protein 1; NAD, Nicotinamide adenine dinucleotide; NFkB, Nuclear factor kappa-light-chain-enhancer of activated B cells; TNFα, Tumor Necrosis Factor-α; TSA, Trichostatin-A; PGC-1α, Peroxisome proliferator-activated receptor-gamma coactivator-1α; PPAR-δ, Peroxisome proliferator-activated receptor-δ; SIK1, Salt-inducible kinase 1; SIRT, Sirtuin; STAT3, Signal Transducer And Activator Of Transcription 3; VPA, Valproic acid; WAT, White adipose tissue.
References
Abd Elwahab, A. H., Ramadan, B. K., Schaalan, M. F., and Tolba, A. M. (2017). A novel role of SIRT1/FGF-21 in taurine protection against cafeteria diet-induced steatohepatitis in rats. Cell. Physiol. Biochem. 43, 644–659. doi: 10.1159/000480649
Abedi-Taleb, E., Vahabi, Z., Sekhavati-Moghadam, E., Khedmat, L., Jazayeri, S., and Saboor-Yaraghi, A. A. (2019). Upregulation of FNDC5 gene expression in C2C12 cells after single and combined treatments of resveratrol and ATRA. Lipids Health Dis. 18:181. doi: 10.1186/S12944-019-1128-Y
Ahima, R. S., and Park, H. K. (2015). Connecting myokines and metabolism. Endocrinol. Metab. 30, 235–245. doi: 10.3803/EnM.2015.30.3.235
Albrecht, E., Norheim, F., Thiede, B., Holen, T., Ohashi, T., Schering, L., et al. (2015). Irisin – a myth rather than an exercise-inducible myokine. Sci. Rep. 5:8889. doi: 10.1038/srep08889
Alvarez, B., Carbó, N., López-Soriano, J., Drivdahl, R. H., Busquets, S., López-Soriano, F. J., et al. (2002). Effects of interleukin-15 (IL-15) on adipose tissue mass in rodent obesity models: evidence for direct IL-15 action on adipose tissue. Biochim. Biophys. Acta Gen. Subj. 1570, 33–37. doi: 10.1016/S0304-4165(02)00148-4
Argilés, J. M., Orpí, M., Busquets, S., and López-Soriano, F. J. (2012). Myostatin: more than just a regulator of muscle mass. Drug Discov. Today 17, 702–709. doi: 10.1016/j.drudis.2012.02.001
Arounleut, P., Bowser, M., Upadhyay, S., Shi, X. M., Fulzele, S., Johnson, M. H., et al. (2013). Erratum: absence of functional leptin receptor isoforms in the POUND (Leprdb/lb) mouse is associated with muscle atrophy and altered myoblast proliferation and differentiation. PLoS One 8:8. doi: 10.1371/annotation/3a7d6e24-137c-4603-93ca-879bec7fab80
Astratenkova, I. V., and Rogozkin, V. A. (2019). The role of acetylation/deacetylation of histones and transcription factors in regulating metabolism in skeletal muscles. Neurosci. Behav. Physiol. 49, 281–288. doi: 10.1007/s11055-019-00730-2
Avila, A. M., Burnett, B. G., Taye, A. A., Gabanella, F., Knight, M. A., Hartenstein, P., et al. (2007). Trichostatin A increases SMN expression and survival in a mouse model of spinal muscular atrophy. J. Clin. Invest. 117, 659–671. doi: 10.1172/JCI29562
Baccam, A., Benoni-Sviercovich, A., Rocchi, M., Moresi, V., Seelaender, M., Li, Z., et al. (2019). The mechanical stimulation of myotubes counteracts the effects of tumor-derived factors through the modulation of the activin/follistatin ratio. Front. Physiol. 10:401. doi: 10.3389/fphys.2019.00401
Bannister, A. J., and Kouzarides, T. (2011). Regulation of chromatin by histone modifications. Cell Res. 21, 381–395. doi: 10.1038/cr.2011.22
Barja-Fernandez, S., Moreno-Navarrete, J. M., Folgueira, C., Xifra, G., Sabater, M., Castelao, C., et al. (2018). Plasma ANGPTL-4 is associated with obesity and glucose tolerance: cross-sectional and longitudinal findings. Mol. Nutr. Food Res. 62:e1800060. doi: 10.1002/mnfr.201800060
Baskin, K. K., Winders, B. R., and Olson, E. N. (2015). Muscle as a “mediator” of systemic metabolism. Cell Metab. 21, 237–248. doi: 10.1016/j.cmet.2014.12.021
Berardi, E., Madaro, L., Lozanoska-Ochser, B., Adamo, S., Thorrez, L., Bouche, M., et al. (2021). A pound of flesh: what cachexia is and what it is not. Diagnostics 11:116. doi: 10.3390/diagnostics11010116
Berdeaux, R., Goebel, N., Banaszynski, L., Takemori, H., Wandless, T., Shelton, G. D., et al. (2007). SIK1 is a class II HDAC kinase that promotes survival of skeletal myocytes. Nat. Med. 13, 597–603. doi: 10.1038/nm1573
Berezin, A. E., Berezin, A. A., and Lichtenauer, M. (2021). Myokines and heart failure: challenging role in adverse cardiac remodeling, myopathy, and clinical outcomes. Dis. Markers 2021:6644631. doi: 10.1155/2021/6644631
Bhaskara, S. (2018). Histone deacetylase 11 as a key regulator of metabolism and obesity. EBioMedicine 35, 27–28. doi: 10.1016/j.ebiom.2018.08.008
Bianchi, M., Renzini, A., Adamo, S., and Moresi, V. (2017). Coordinated actions of microRNAs with other epigenetic factors regulate skeletal muscle development and adaptation. Int. J. Mol. Sci. 18:840. doi: 10.3390/ijms18040840
Bonetto, A., Penna, F., Minero, V., Reffo, P., Bonelli, G., Baccino, F., et al. (2009). Deacetylase inhibitors modulate the myostatin/follistatin axis without improving cachexia in tumor-bearing mice. Curr. Cancer Drug Targets 9, 608–616. doi: 10.2174/156800909789057015
Boström, P., Wu, J., Jedrychowski, M. P., Korde, A., Ye, L., Lo, J. C., et al. (2012). A PGC1-α-dependent myokine that drives brown-fat-like development of white fat and thermogenesis. Nature 481, 463–468. doi: 10.1038/nature10777
Bretland, K. A., Lin, L., Bretland, K. M., Smith, M. A., Fleming, S. M., and Dengler-Crish, C. M. (2021). Irisin treatment lowers levels of phosphorylated tau in the hippocampus of pre-symptomatic female but not male htau mice. Neuropathol. Appl. Neurobiol. 47, 967–978. doi: 10.1111/NAN.12711
Busquets, S., Figueras, M., Almendro, V., López-Soriano, F. J., and Argilés, J. M. (2006). Interleukin-15 increases glucose uptake in skeletal muscle An antidiabetogenic effect of the cytokine. Biochim. Biophys. Acta Gen. Subj. 1760, 1613–1617. doi: 10.1016/j.bbagen.2006.09.001
Cantó, C., Gerhart-Hines, Z., Feige, J. N., Lagouge, M., Noriega, L., Milne, J. C., et al. (2009). AMPK regulates energy expenditure by modulating NAD+ metabolism and SIRT1 activity. Nature 458, 1056–1060. doi: 10.1038/nature07813
Carbó, N., López-Soriano, J., Costelli, P., Alvarez, B., Busquets, S., Baccino, F. M., et al. (2001). Interleukin-15 mediates reciprocal regulation of adipose and muscle mass: a potential role in body weight control. Biochim. Biophys. Acta Gen. Subj. 1526, 17–24. doi: 10.1016/S0304-4165(00)00188-4
Catoire, M., Alex, S., Paraskevopulos, N., Mattijssen, F., Evers-Van Gogh, I., Schaart, G., et al. (2014). Fatty acid-inducible ANGPTL4 governs lipid metabolic response to exercise. Proc. Natl. Acad. Sci. U. S. A. 111, E1043–52. doi: 10.1073/pnas.1400889111
Chang, H., Kwon, O., Shin, M.-S., Kang, G. M., Leem, Y. H., Lee, C. H., et al. (2018). Role of Angptl4/Fiaf in exercise-induced skeletal muscle AMPK activation. J. Appl. Physiol. 125, 715–722. doi: 10.1152/japplphysiol.00984.2016
Chau, M. D., Gao, J., Yang, Q., Wu, Z., and Gromada, J. (2010). Fibroblast growth factor 21 regulates energy metabolism by activating the AMPK–SIRT1–PGC-1α pathway. Proc. Natl. Acad. Sci. U. S. A. 107, 12553–12558. doi: 10.1073/PNAS.1006962107
Chen, W., Zhang, X., Birsoy, K., and Roeder, R. G. (2010). A muscle-specific knockout implicates nuclear receptor coactivator MED1 in the regulation of glucose and energy metabolism. Proc. Natl. Acad. Sci. U. S. A. 107, 10196–10201. doi: 10.1073/pnas.1005626107
Correia, J. C., Ferreira, D. M., and Ruas, J. L. (2015). Intercellular: local and systemic actions of skeletal muscle PGC-1s. Trends Endocrinol. Metab. 26, 305–314. doi: 10.1016/j.tem.2015.03.010
Cuevas-Ramos, D., Mehta, R., and Aguilar-Salinas, C. A. (2019). Fibroblast growth factor 21 and browning of white adipose tissue. Front. Physiol. 10:37. doi: 10.3389/fphys.2019.00037
da Silva Vasconcelos, E., and Fernanda Salla, R. (2018). Role of interleukin-6 and interleukin-15 in exercise. MOJ Immunol. 6:00185. doi: 10.15406/MOJI.2018.06.00185
Daou, N., Hassani, M., Matos, E., De Castro, G. S., Costa, R. G. F., Seelaender, M., et al. (2020). Displaced myonuclei in cancer cachexia suggest altered innervation. Int. J. Mol. Sci. 21:1092. doi: 10.3390/ijms21031092
Darvin, P., Toor, S. M., Sasidharan Nair, V., and Elkord, E. (2018). Immune checkpoint inhibitors: recent progress and potential biomarkers. Exp. Mol. Med. 50:165. doi: 10.1038/s12276-018-0191-1
de Lima, E. A., Teixeira, A. A., de, S., Biondo, L. A., Diniz, T. A., Silveira, L. S., et al. (2020). Exercise reduces the resumption of tumor growth and proteolytic pathways in the skeletal muscle of mice following chemotherapy. Cancers (Basel) 12:3466. doi: 10.3390/cancers12113466
Di Felice, V., Coletti, D., and Seelaender, M. (2020). Editorial: myokines, adipokines, cytokines in muscle pathophysiology. Front. Physiol. 11:592856. doi: 10.3389/fphys.2020.592856
Dupont-Versteegden, E. E., Houlé, J. D., Dennis, R. A., Zhang, J., Knox, M., Wagoner, G., et al. (2004). Exercise-induced gene expression in soleus muscle is dependent on time after spinal cord injury in rats. Muscle Nerve 29, 73–81. doi: 10.1002/MUS.10511
Ellingsgaard, H., Hauselmann, I., Schuler, B., Habib, A. M., Baggio, L. L., Meier, D. T., et al. (2011). Interleukin-6 enhances insulin secretion by increasing glucagon-like peptide-1 secretion from L cells and alpha cells. Nat. Med. 17, 1481–1489. doi: 10.1038/nm.2513
Elsen, M., Raschke, S., and Eckel, J. (2014). Browning of white fat: does irisin play a role in humans? J. Endocrinol. 222, R25–R38. doi: 10.1530/JOE-14-0189
Ershler, W. B., and Keller, E. T. (2003). Age-associated increased interleukin-6 gene expression, late-life diseases, and frailty. Annu. Rev. Med. 51, 245–270. doi: 10.1146/ANNUREV.MED.51.1.245
Evans, W. S., Blumenthal, J. B., Heilman, J. M., Ryan, A. S., and Prior, S. J. (2021). Effects of exercise training with weight loss on skeletal muscle expression of angiogenic factors in overweight and obese older men. J. Appl. Physiol. (1985) 131, 56–63. doi: 10.1152/JAPPLPHYSIOL.00084.2021
Fazeli, P. K., Faje, A. T., Cross, E. J., Lee, H., Rosen, C. J., Bouxsein, M. L., et al. (2015). Serum FGF-21 levels are associated with worsened radial trabecular bone microarchitecture and decreased radial bone strength in women with anorexia nervosa. Bone 77, 6–11. doi: 10.1016/j.bone.2015.04.001
Feige, J. N., Lagouge, M., Canto, C., Strehle, A., Houten, S. M., Milne, J. C., et al. (2008). Specific SIRT1 activation mimics low energy levels and protects against diet-induced metabolic disorders by enhancing fat oxidation. Cell Metab. 8, 347–358. doi: 10.1016/J.CMET.2008.08.017
Fernández-Hernando, C., and Suárez, Y. (2020). ANGPTL4: a multifunctional protein involved in metabolism and vascular homeostasis. Curr. Opin. Hematol. 27, 206–213. doi: 10.1097/MOH.0000000000000580
Finsterwald, C., Carrard, A., and Martin, J.-L. (2013). Role of salt-inducible kinase 1 in the activation of MEF2-dependent transcription by BDNF. PLoS One 8:e54545. doi: 10.1371/journal.pone.0054545
Fischle, W., Dequiedt, F., Hendzel, M. J., Guenther, M. G., Lazar, M. A., Voelter, W., et al. (2002). Enzymatic activity associated with class II HDACs is dependent on a multiprotein complex containing HDAC3 and SMRT/N-CoR. Mol. Cell 9, 45–57. doi: 10.1016/S1097-2765(01)00429-4
Florin, A., Lambert, C., Sanchez, C., Zappia, J., Durieux, N., Tieppo, A. M., et al. (2020). The secretome of skeletal muscle cells: a systematic review. Osteoarthr. Cartil. Open 2:100019. doi: 10.1016/j.ocarto.2019.100019
Forcina, L., Miano, C., Scicchitano, B. M., Rizzuto, E., Berardinelli, M. G., De Benedetti, F., et al. (2019). Increased circulating levels of interleukin-6 affect the redox balance in skeletal muscle. Oxid. Med. Cell. Longev. 2019, 1–13. doi: 10.1155/2019/3018584
Fruebis, J. (2001). Proteolytic cleavage product of 30-kDa adipocyte complement-related protein increases fatty acid oxidation in muscle and causes weight loss in mice. Proc. Natl. Acad. Sci. U. S. A. 98, 2005–2010. doi: 10.1073/pnas.041591798
Frye, R. A. (1999). Characterization of five human cDNAs with homology to the yeast SIR2 gene: sir2-like proteins (Sirtuins) metabolize NAD and may have protein ADP-ribosyltransferase activity. Biochem. Biophys. Res. Commun. 260, 273–279. doi: 10.1006/bbrc.1999.0897
Furukawa, N., Koitabashi, N., Matsui, H., Sunaga, H., Umbarawan, Y., Syamsunarno, M. R. A. A., et al. (2020). DPP-4 inhibitor induces FGF21 expression via sirtuin 1 signaling and improves myocardial energy metabolism. Heart Vessels 361, 136–146. doi: 10.1007/S00380-020-01711-Z
Galmozzi, A., Mitro, N., Ferrari, A., Gers, E., Gilardi, F., Godio, C., et al. (2013). Inhibition of class I histone deacetylases unveils a mitochondrial signature and enhances oxidative metabolism in skeletal muscle and adipose tissue. Diabetes 62, 732–742. doi: 10.2337/db12-0548
Gannon, J., Doran, P., Kirwan, A., and Ohlendieck, K. (2009). Drastic increase of myosin light chain MLC-2 in senescent skeletal muscle indicates fast-to-slow fibre transition in sarcopenia of old age. Eur. J. Cell Biol. 88, 685–700. doi: 10.1016/j.ejcb.2009.06.004
Gao, Z., Yin, J., Zhang, J., Ward, R. E., Martin, R. J., Lefevre, M., et al. (2009). Butyrate improves insulin sensitivity and increases energy expenditure in mice. Diabetes 58, 1509–1517. doi: 10.2337/db08-1637
García-Carrizo, F., Nozhenko, Y., Palou, A., and Rodríguez, A. M. (2016). Leptin effect on acetylation and phosphorylation of Pgc1α in muscle cells associated With Ampk and Akt activation in high-glucose medium. J. Cell. Physiol. 231, 641–649. doi: 10.1002/jcp.25109
Gatla, H. R., Muniraj, N., Thevkar, P., Yavvari, S., Sukhavasi, S., and Makena, M. R. (2019). Regulation of chemokines and cytokines by histone deacetylases and an update on histone decetylase inhibitors in human diseases. Int. J. Mol. Sci. 20:1110. doi: 10.3390/ijms20051110
Gaur, V., Connor, T., Sanigorski, A., Martin, S. D., Bruce, C. R., Henstridge, D. C., et al. (2016). Disruption of the class IIa HDAC corepressor complex increases energy expenditure and lipid oxidation. Cell Rep. 16, 2802–2810. doi: 10.1016/j.celrep.2016.08.005
Glozak, M. A., Sengupta, N., Zhang, X., and Seto, E. (2005). Acetylation and deacetylation of non-histone proteins. Gene 363, 15–23. doi: 10.1016/J.GENE.2005.09.010
Guo, L., Li, S., Zhao, Y., Qian, P., Ji, F., Qian, L., et al. (2015). Silencing angiopoietin-like protein 4 (ANGPTL4) protects against lipopolysaccharide-induced acute lung injury via regulating SIRT1/NF-kB pathway. J. Cell. Physiol. 230, 2390–2402. doi: 10.1002/JCP.24969
Guridi, M., Tintignac, L. A., Lin, S., Kupr, B., Castets, P., and Rüegg, M. A. (2015). Activation of mTORC1 in skeletal muscle regulates whole-body metabolism through FGF21. Sci. Signal. 8:ra113. doi: 10.1126/scisignal.aab3715
Haberland, M., Montgomery, R. L., and Olson, E. N. (2009). The many roles of histone deacetylases in development and physiology: implications for disease and therapy. Nat. Rev. Genet. 10, 32–42. doi: 10.1038/nrg2485
Hamrick, M. W., Samaddar, T., Pennington, C., and McCormick, J. (2006). Increased muscle mass with myostatin deficiency improves gains in bone strength with exercise. J. Bone Miner. Res. 21, 477–483. doi: 10.1359/JBMR.051203
Hamrick, M. W., Shi, X., Zhang, W., Pennington, C., Thakore, H., Haque, M., et al. (2007). Loss of myostatin (GDF8) function increases osteogenic differentiation of bone marrow-derived mesenchymal stem cells but the osteogenic effect is ablated with unloading. Bone 40, 1544–1553. doi: 10.1016/j.bone.2007.02.012
Hanks, L. J., Gutiérrez, O. M., Bamman, M. M., Ashraf, A., McCormick, K. L., and Casazza, K. (2015). Circulating levels of fibroblast growth factor-21 increase with age independently of body composition indices among healthy individuals. J. Clin. Transl. Endocrinol. 2, 77–82. doi: 10.1016/j.jcte.2015.02.001
Henagan, T. M., Stefanska, B., Fang, Z., Navard, A. M., Ye, J., Lenard, N. R., et al. (2015). Sodium butyrate epigenetically modulates high-fat diet-induced skeletal muscle mitochondrial adaptation, obesity and insulin resistance through nucleosome positioning. Br. J. Pharmacol. 172, 2782–2798. doi: 10.1111/bph.13058
Hennigar, S. R., McClung, J. P., and Pasiakos, S. M. (2017). Nutritional interventions and the IL-6 response to exercise. FASEB J. 31, 3719–3728. doi: 10.1096/FJ.201700080R
Hong, S., Zhou, W., Fang, B., Lu, W., Loro, E., Damle, M., et al. (2017). Dissociation of muscle insulin sensitivity from exercise endurance in mice by HDAC3 depletion. Nat. Med. 23, 223–234. doi: 10.1038/nm.4245
Houtkooper, R. H., Pirinen, E., and Auwerx, J. (2012). Sirtuins as regulators of metabolism and healthspan. Nat. Rev. Mol. Cell Biol. 13, 225–238. doi: 10.1038/nrm3293
Howlett, K. F., and McGee, S. L. (2016). Epigenetic regulation of skeletal muscle metabolism. Clin. Sci. 130, 1051–1063. doi: 10.1042/CS20160115
Hurtado, E., Núñez-Álvarez, Y., Muñoz, M., Gutiérrez-Caballero, C., Casas, J., Pendás, A. M., et al. (2021). HDAC11 is a novel regulator of fatty acid oxidative metabolism in skeletal muscle. FEBS J. 288, 902–919. doi: 10.1111/febs.15456
Hutchinson, K. A., Mohammad, S., Garneau, L., McInnis, K., Aguer, C., and Adamo, K. B. (2019). Examination of the myokine response in pregnant and non-pregnant women following an acute bout of moderate-intensity walking. Front. Physiol. 10:1188. doi: 10.3389/fphys.2019.01188
Hyndman, K. A., and Knepper, M. A. (2017). Dynamic regulation of lysine acetylation: the balance between acetyltransferase and deacetylase activities. Am. J. Physiol. Ren. Physiol. 313, F842–F846. doi: 10.1152/ajprenal.00313.2017
Iezzi, S., Di Padova, M., Serra, C., Caretti, G., Simone, C., Maklan, E., et al. (2004). Deacetylase inhibitors increase muscle cell size by promoting myoblast recruitment and fusion through induction of follistatin. Dev. Cell 6, 673–684. doi: 10.1016/S1534-5807(04)00107-8
Jacques, M., Hiam, D., Craig, J., Barrès, R., Eynon, N., and Voisin, S. (2019). Epigenetic changes in healthy human skeletal muscle following exercise– a systematic review. Epigenetics 14, 633–648. doi: 10.1080/15592294.2019.1614416
Jedrychowski, M. P., Wrann, C. D., Paulo, J. A., Gerber, K. K., Szpyt, J., Robinson, M. M., et al. (2015). Detection and quantitation of circulating human irisin by tandem mass spectrometry. Cell Metab. 22, 734–740. doi: 10.1016/J.CMET.2015.08.001
Jian, H., Yimin, J., Shifng, P., Longfei, J., Huifang, L., Zhenqiang, H., et al. (2016). Butyrate alleviates high fat diet-induced obesity through activation of adiponectin-mediated pathway and stimulation of mitochondrial function in the skeletal muscle of mice. Oncotarget 7, 56071–56082. doi: 10.18632/oncotarget.11267
Jiang, S., Piao, L., Ma, E. B., Ha, H., and Huh, J. Y. (2021). Associations of circulating irisin with FNDC5 expression in fat and muscle in type 1 and type 2 diabetic mice. Biomolecules 11, 1–14. doi: 10.3390/BIOM11020322
Jiang, X., Chen, J., Zhang, C., Zhang, Z., Tan, Y., Feng, W., et al. (2015). The protective effect of FGF21 on diabetes-induced male germ cell apoptosis is associated with up-regulated testicular AKT and AMPK/Sirt1/PGC-1α signaling. Endocrinology 156, 1156–1170. doi: 10.1210/EN.2014-1619
Keinicke, H., Sun, G., Mentzel, C. M. J., Fredholm, M., John, L. M., Andersen, B., et al. (2020). FGF21 regulates hepatic metabolic pathways to improve steatosis and inflammation. Endocr. Connect. 9, 755–768. doi: 10.1530/EC-20-0152
Keipert, S., Ost, M., Johann, K., Imber, F., Jastroch, M., van Schothorst, E. M., et al. (2014). Skeletal muscle mitochondrial uncoupling drives endocrine cross-talk through the induction of FGF21 as a myokine. Am. J. Physiol. Endocrinol. Metab. 306, E469–E482. doi: 10.1152/ajpendo.00330.2013
Kern, P. A., Ranganathan, S., Li, C., Wood, L., and Ranganathan, G. (2001). Adipose tissue tumor necrosis factor and interleukin-6 expression in human obesity and insulin resistance. Am. J. Physiol. Endocrinol. Metab. 280, E745–E751. doi: 10.1152/AJPENDO.2001.280.5.E745
Kim, K. H., and Lee, M. S. (2014). FGF21 as a stress hormone: the roles of FGF21 in stress adaptation and the treatment of metabolic disease. Diabetes Metab. J. 38, 245–251. doi: 10.4093/dmj.2014.38.4.245
Klymenko, O., Brecklinghaus, T., Dille, M., Springer, C., de Wendt, C., Altenhofen, D., et al. (2020). Histone deacetylase 5 regulates interleukin 6 secretion and insulin action in skeletal muscle. Mol. Metab. 42:101062. doi: 10.1016/j.molmet.2020.101062
Kurgan, N., Noaman, N., Pergande, M. R., Cologna, S. M., Coorssen, J. R., and Klentrou, P. (2019). Changes to the human serum proteome in response to high intensity interval exercise: a sequential top-down proteomic analysis. Front. Physiol. 10:362. doi: 10.3389/fphys.2019.00362
Laurens, C., Bergouignan, A., and Moro, C. (2020). Exercise-released myokines in the control of energy metabolism. Front. Physiol. 11:91. doi: 10.3389/fphys.2020.00091
Leal, L. G., Lopes, M. A., and Batista, M. L. (2018). Physical exercise-induced myokines and muscle-adipose tissue crosstalk: a review of current knowledge and the implications for health and metabolic diseases. Front. Physiol. 9:1307. doi: 10.3389/fphys.2018.01307
Lecompte, S., Abou-Samra, M., Boursereau, R., Noel, L., and Brichard, S. M. (2017). Skeletal muscle secretome in Duchenne muscular dystrophy: a pivotal anti-inflammatory role of adiponectin. Cell. Mol. Life Sci. 7413, 2487–2501. doi: 10.1007/S00018-017-2465-5
Lee, J. O., Byun, W. S., Kang, M. J., Han, J. A., Moon, J., Shin, M. J., et al. (2020). The myokine meteorin-like (metrnl) improves glucose tolerance in both skeletal muscle cells and mice by targeting AMPKα2. FEBS J. 287, 2087–2104. doi: 10.1111/febs.15301
Leng, Y., Wang, J., Wang, Z., Liao, H.-M., Wei, M., Leeds, P., et al. (2016). Valproic acid and other HDAC inhibitors upregulate FGF21 gene expression and promote process elongation in glia by inhibiting HDAC2 and 3. Int. J. Neuropsychopharmacol. 19:pyw035. doi: 10.1093/IJNP/PYW035
Li, G., Zhang, H., and Ryan, A. S. (2020). Skeletal muscle angiopoietin-like protein 4 and glucose metabolism in older adults after exercise and weight loss. Metabolites 10:354. doi: 10.3390/metabo10090354
Li, H., Gao, Z., Zhang, J., Ye, X., Xu, A., Ye, J., et al. (2012). Sodium butyrate stimulates expression of fibroblast growth factor 21 in liver by inhibition of histone deacetylase 3. Diabetes 61, 797–806. doi: 10.2337/db11-0846
Li, K., Liao, X., Wang, K., Mi, Q., Zhang, T., Jia, Y., et al. (2018). Myonectin predicts the development of type 2 diabetes. J. Clin. Endocrinol. Metab. 103, 139–147. doi: 10.1210/jc.2017-01604
Li, S., Zhu, Z., Xue, M., Yi, X., Liang, J., Niu, C., et al. (2019). Fibroblast growth factor 21 protects the heart from angiotensin II-induced cardiac hypertrophy and dysfunction via SIRT1. Biochim. Biophys. Acta Mol. Basis Dis. 1865, 1241–1252. doi: 10.1016/J.BBADIS.2019.01.019
Li, Y., and Seto, E. (2016). HDACs and HDAC inhibitors in cancer development and therapy. Cold Spring Harb. Perspect. Med. 6:a026831. doi: 10.1101/cshperspect.a026831
Li, Y., Wong, K., Giles, A., Jiang, J., Lee, J. W., Adams, A. C., et al. (2014). Hepatic SIRT1 attenuates hepatic steatosis and controls energy balance in mice by inducing fibroblast growth factor 21. Gastroenterology 146, 539–49.e7. doi: 10.1053/J.GASTRO.2013.10.059
Lin, H. V., Frassetto, A., Kowalik, E. J. Jr., Nawrocki, A. R., Lu, M. M., Kosinski, J. R., et al. (2012). Butyrate and propionate protect against diet-induced obesity and regulate gut hormones via free fatty acid receptor 3-independent mechanisms. PLoS One 7:e35240. doi: 10.1371/journal.pone.0035240
Liu, J. J., Foo, J. P., Liu, S., and Lim, S. C. (2015). The role of fibroblast growth factor 21 in diabetes and its complications: a review from clinical perspective. Diabetes Res. Clin. Pract. 108, 382–389. doi: 10.1016/J.DIABRES.2015.02.032
Liu, P. Z., and Nusslock, R. (2018). Exercise-mediated neurogenesis in the hippocampus via BDNF. Front. Neurosci. 12:52. doi: 10.3389/FNINS.2018.00052
Liu, X., Wang, Y., Hou, L., Xiong, Y., and Zhao, S. (2017). Fibroblast Growth Factor 21 (FGF21) promotes formation of aerobic myofibers via the FGF21-SIRT1-AMPK-PGC1α Pathway. J. Cell. Physiol. 232, 1893–1906. doi: 10.1002/JCP.25735
Luan, B., Goodarzi, M. O., Phillips, N. G., Guo, X., Chen, Y. D. I., Yao, J., et al. (2014). Leptin-mediated increases in catecholamine signaling reduce adipose tissue inflammation via activation of macrophage HDAC4. Cell Metab. 19, 1058–1065. doi: 10.1016/j.cmet.2014.03.024
Maak, S., Norheim, F., Drevon, C. A., and Erickson, H. P. (2021). Progress and Challenges in the Biology of FNDC5 and Irisin. Endocr. Rev. 42, 436–456. doi: 10.1210/ENDREV/BNAB003
Martínez-Garza, Ú., Torres-Oteros, D., Yarritu-Gallego, A., Marrero, P. F., Haro, D., and Relat, J. (2019). Fibroblast growth factor 21 and the adaptive response to nutritional challenges. Int. J. Mol. Sci. 20:4692. doi: 10.3390/ijms20194692
Matthews, V. B., Åström, M. B., Chan, M. H. S., Bruce, C. R., Krabbe, K. S., Prelovsek, O., et al. (2009). Brain-derived neurotrophic factor is produced by skeletal muscle cells in response to contraction and enhances fat oxidation via activation of AMP-activated protein kinase. Diabetologia 52, 1409–1418. doi: 10.1007/s00125-009-1364-1
McGee, S. L., and Hargreaves, M. (2011). Histone modifications and exercise adaptations. J. Appl. Physiol. 110, 258–263. doi: 10.1152/japplphysiol.00979.2010
McGee, S. L., Van Denderen, B. J. W., Howlett, K. F., Mollica, J., Schertzer, J. D., Kemp, B. E., et al. (2008). AMP-activated protein kinase regulates GLUT4 transcription by phosphorylating histone deacetylase 5. Diabetes 57, 860–867. doi: 10.2337/db07-0843
McPherron, A. C., Lawler, A. M., and Lee, S. J. (1997). Regulation of skeletal muscle mass in mice by a new TGF-beta superfamily member. Nature 387, 83–90. doi: 10.1038/387083A0
Menzies, K. J., Singh, K., Saleem, A., and Hood, D. A. (2013). Sirtuin 1-mediated effects of exercise and resveratrol on mitochondrial biogenesis. J. Biol. Chem. 288, 6968–6979. doi: 10.1074/jbc.M112.431155
Michishita, E., Park, J. Y., Burneskis, J. M., Barrett, J. C., and Horikawa, I. (2005). Evolutionarily conserved and nonconserved cellular localizations and functions of human SIRT proteins. Mol. Biol. Cell 16, 4623–4635. doi: 10.1091/mbc.E05-01-0033
Minetti, G. C., Colussi, C., Adami, R., Serra, C., Mozzetta, C., Parente, V., et al. (2006). Functional and morphological recovery of dystrophic muscles in mice treated with deacetylase inhibitors. Nat. Med. 12, 1147–1150. doi: 10.1038/nm1479
Miyake, M., Nomura, A., Ogura, A., Takehana, K., Kitahara, Y., Takahara, K., et al. (2016). Skeletal muscle-specific eukaryotic translation initiation factor 2α phosphorylation controls amino acid metabolism and fibroblast growth factor 21-mediated non-cell-autonomous energy metabolism. FASEB J. 30, 798–812. doi: 10.1096/fj.15-275990
Moresi, V., Adamo, S., and Berghella, L. (2019). The JAK/STAT pathway in skeletal muscle pathophysiology. Front. Physiol. 10:500. doi: 10.3389/fphys.2019.00500
Moresi, V., Carrer, M., Grueter, C. E., Rifki, O. F., Shelton, J. M., Richardson, J. A., et al. (2012). Histone deacetylases 1 and 2 regulate autophagy flux and skeletal muscle homeostasis in mice. Proc. Natl. Acad. Sci. U. S. A. 109, 1649–1654. doi: 10.1073/pnas.1121159109
Moresi, V., Marroncelli, N., Pigna, E., and Adamo, S. (2016). “Epigenetics of muscle disorders,” in Medical Epigenetics, ed. T. Tollefsbol (Amsterdam: Elsevier Inc), 315–333. doi: 10.1016/B978-0-12-803239-8.00018-1
Moresi, V., Williams, A. H., Meadows, E., Flynn, J. M., Potthoff, M. J., McAnally, J., et al. (2010). Myogenin and Class II HDACs control neurogenic muscle atrophy by inducing E3 ubiquitin ligases. Cell 143, 35–45. doi: 10.1016/j.cell.2010.09.004
Mozzetta, C., Consalvi, S., Saccone, V., Tierney, M., Diamantini, A., Mitchell, K. J., et al. (2013). Fibroadipogenic progenitors mediate the ability of HDAC inhibitors to promote regeneration in dystrophic muscles of young, but not old Mdx mice. EMBO Mol. Med. 5, 626–639. doi: 10.1002/emmm.201202096
Murawska-Ciałowicz, E., Wiatr, M., Ciałowicz, M., de Assis, G. G., Borowicz, W., Rocha-Rodrigues, S., et al. (2021). BDNF impact on biological markers of depression-role of physical exercise and training. Int. J. Environ. Res. Public Health 18:7553. doi: 10.3390/IJERPH18147553
Nadeau, L., and Aguer, C. (2019). Interleukin-15 as a myokine: mechanistic insight into its effect on skeletal muscle metabolism. Appl. Physiol. Nutr. Metab. 44, 229–238. doi: 10.1139/apnm-2018-0022
Nielsen, A. R., Mounier, R., Plomgaard, P., Mortensen, O. H., Penkowa, M., Speerschneider, T., et al. (2007). Expression of interleukin-15 in human skeletal muscle – effect of exercise and muscle fibre type composition. J. Physiol. 584, 305–12. doi: 10.1113/JPHYSIOL.2007.139618
Norheim, F., Hjorth, M., Langleite, T. M., Lee, S., Holen, T., Bindesbøll, C., et al. (2014). Regulation of angiopoietin-like protein 4 production during and after exercise. Physiol. Rep. 2:e12109. doi: 10.14814/phy2.12109
Northoff, H., and Berg, A. (1991). Immunologic mediators as parameters of the reaction to strenuous exercise. Int. J. Sports Med. 12, S9–S15. doi: 10.1055/S-2007-1024743
Nozhenko, Y., Rodríguez, A. M., and Palou, A. (2015). Leptin rapidly induces the expression of metabolic and myokine genes in C2C12 muscle cells to regulate nutrient partition and oxidation. Cell. Physiol. Biochem. 35, 92–103. doi: 10.1159/000369678
Oost, L. J., Kustermann, M., Armani, A., Blaauw, B., and Romanello, V. (2019). Fibroblast growth factor 21 controls mitophagy and muscle mass. J. Cachexia Sarcopenia Muscle 10, 630–642. doi: 10.1002/jcsm.12409
Ostrowski, K., Rohde, T., Zacho, M., Asp, S., and Pedersen, B. K. (1998). Evidence that interleukin-6 is produced in human skeletal muscle during prolonged running. J. Physiol. 508, 949–953. doi: 10.1111/j.1469-7793.1998.949bp.x
Otaka, N., Shibata, R., Ohashi, K., Murohara, T., and Ouchi, N. (2017). P5393Myonectin/CTRP15 protects against myocardial ischemia-reperfusion injury. Eur. Heart J. 38:5393. doi: 10.1093/eurheartj/ehx493.P5393
Otaka, N., Shibata, R., Ohashi, K., Uemura, Y., Kambara, T., Enomoto, T., et al. (2018). Myonectin is an exercise-induced Myokine that protects the heart from ischemia-reperfusion injury. Circ. Res. 123, 1326–1338. doi: 10.1161/CIRCRESAHA.118.313777
Park, S. Y., and Kim, J. S. (2020). A short guide to histone deacetylases including recent progress on class II enzymes. Exp. Mol. Med. 52, 204–212. doi: 10.1038/s12276-020-0382-4
Pasyukova, E. G., and Vaiserman, A. M. (2017). HDAC inhibitors: a new promising drug class in anti-aging research. Mech. Ageing Dev. 166, 6–15. doi: 10.1016/j.mad.2017.08.008
Patel, K. (1998). Follistatin. Int. J. Biochem. Cell Biol. 30, 1087–1093. doi: 10.1016/S1357-2725(98)00064-8
Pedersen, B. K., Steensberg, A., Fischer, C., Keller, C., Keller, P., Plomgaard, P., et al. (2003). Searching for the exercise factor: is IL-6 a candidate? J Muscle Res Cell Motil. 24, 113–119. doi: 10.1023/A:1026070911202
Pereira, R. O., Tadinada, S. M., Zasadny, F. M., Oliveira, K. J., Pires, K. M. P., Olvera, A., et al. (2017). OPA1 deficiency promotes secretion of FGF21 from muscle that prevents obesity and insulin resistance. EMBO J. 36, 2126–2145. doi: 10.15252/embj.201696179
Peserico, A., and Simone, C. (2011). Physical and functional HAT/HDAC interplay regulates protein acetylation balance. J. Biomed. Biotechnol. 2011, 1–10. doi: 10.1155/2011/371832
Piccirillo, R. (2019). Exercise-induced myokines with therapeutic potential for muscle wasting. Front. Physiol. 10:287. doi: 10.3389/fphys.2019.00287
Pigna, E., Simonazzi, E., Sanna, K., Bernadzki, K. M., Proszynski, T., Heil, C., et al. (2019). Histone deacetylase 4 protects from denervation and skeletal muscle atrophy in a murine model of amyotrophic lateral sclerosis. EBioMedicine 40, 717–732. doi: 10.1016/j.ebiom.2019.01.038
Pin, F., Bonewald, L. F., and Bonetto, A. (2021). Role of myokines and osteokines in cancer cachexia. Exp. Biol. Med. (Maywood) 246, 2118–2127. doi: 10.1177/15353702211009213
Pistilli, E. E., and Quinn, L. S. (2013). From anabolic to oxidative: reconsidering the roles of IL-15 and IL-15Rα in skeletal muscle. Exerc. Sport Sci. Rev. 41, 100–106. doi: 10.1097/JES.0b013e318275d230
Pistilli, E. E., Siu, P. M., and Alway, S. E. (2007). Interleukin-15 responses to aging and unloading-induced skeletal muscle atrophy. Am. J. Physiol. Physiol. 292, C1298–C1304. doi: 10.1152/ajpcell.00496.2006
Potthoff, M. J., Wu, H., Arnold, M. A., Shelton, J. M., Backs, J., McAnally, J., et al. (2007). Histone deacetylase degradation and MEF2 activation promote the formation of slow-twitch myofibers. J. Clin. Invest. 117, 2459–2467. doi: 10.1172/JCI31960
Pourteymour, S., Eckardt, K., Holen, T., Langleite, T., Lee, S., Jensen, J., et al. (2017). Global mRNA sequencing of human skeletal muscle: search for novel exercise-regulated myokines. Mol. Metab. 6, 352–365. doi: 10.1016/J.MOLMET.2017.01.007
Pradhan, A. D., Manson, J. E., Rifai, N., Buring, J. E., and Ridker, P. M. (2001). C-Reactive protein, interleukin 6, and risk of developing type 2 diabetes mellitus. JAMA 286, 327–334. doi: 10.1001/JAMA.286.3.327
Quinn, L. S. (2008). Interleukin-15: a muscle-derived cytokine regulating fat-to-lean body composition1,2. J. Anim. Sci. 86, E75–E83. doi: 10.2527/jas.2007-0458
Quinn, L. S., Anderson, B. G., Conner, J. D., and Wolden-Hanson, T. (2013). IL-15 overexpression promotes endurance, oxidative energy metabolism, and muscle PPARδ, SIRT1, PGC-1α, and PGC-1β expression in male mice. Endocrinology 154, 232–245. doi: 10.1210/EN.2012-1773
Quinn, L. S., Anderson, B. G., Conner, J. D., Pistilli, E. E., and Wolden-Hanson, T. (2011). Overexpression of interleukin-15 in mice promotes resistance to diet-induced obesity, increased insulin sensitivity, and markers of oxidative skeletal muscle metabolism. Int. J. Interf. Cytokine Mediat. Res. 3, 29–42. doi: 10.2147/IJICMR.S19007
Quinn, L. S., Anderson, B. G., Conner, J. D., Wolden-Hanson, T., and Marcell, T. J. (2014). IL-15 is required for postexercise induction of the pro-oxidative mediators PPARδ and SIRT1 in male mice. Endocrinology 155, 143–155. doi: 10.1210/EN.2013-1645
Quinn, L. S., Anderson, B. G., Strait-Bodey, L., and Wolden-Hanson, T. (2010). Serum and muscle interleukin-15 levels decrease in aging mice: correlation with declines in soluble interleukin-15 receptor alpha expression. Exp. Gerontol. 45, 106–112. doi: 10.1016/j.exger.2009.10.012
Quinn, L. S., Anderson, B. G., Strait-Bodey, L., Stroud, A. M., and Argués, J. M. (2009). Oversecretion of interleukin-15 from skeletal muscle reduces adiposity. Am. J. Physiol. Endocrinol. Metab. 296, E191–202. doi: 10.1152/ajpendo.90506.2008
Rabiee, F., Lachinani, L., Ghaedi, S., Nasr-Esfahani, M. H., Megraw, T. L., and Ghaedi, K. (2020). New insights into the cellular activities of Fndc5/Irisin and its signaling pathways. Cell Biosci. 10:51. doi: 10.1186/s13578-020-00413-3
Rao, R. R., Long, J. Z., White, J. P., Svensson, K. J., Lou, J., Lokurkar, I., et al. (2014). Meteorin-like is a hormone that regulates immune-adipose interactions to increase beige fat thermogenesis. Cell 157, 1279–1291. doi: 10.1016/j.cell.2014.03.065
Raschke, S., and Eckel, J. (2013). Adipo-Myokines: two sides of the same coin–Mediators of inflammation and mediators of exercise. Mediators Inflamm. 2013:320724. doi: 10.1155/2013/320724
Raschke, S., Elsen, M., Gassenhuber, H., Sommerfeld, M., Schwahn, U., Brockmann, B., et al. (2013). Evidence against a beneficial effect of irisin in humans. PLoS One 8:e73680. doi: 10.1371/JOURNAL.PONE.0073680
Reihmane, D., and Dela, F. (2013). Interleukin-6: possible biological roles during exercise. Eur. J. Sport Sci. 14, 242–250. doi: 10.1080/17461391.2013.776640
Renzini, A., Marroncelli, N., Noviello, C., Moresi, V., and Adamo, S. (2018). HDAC4 regulates skeletal muscle regeneration via soluble factors. Front. Physiol. 9:1387. doi: 10.3389/fphys.2018.01387
Roberts, L. D., Boström, P., O’Sullivan, J. F., Schinzel, R. T., Lewis, G. D., Dejam, A., et al. (2014). β-aminoisobutyric acid induces browning of white fat and hepatic β-oxidation and is inversely correlated with cardiometabolic risk factors. Cell Metab. 19, 96–108. doi: 10.1016/J.CMET.2013.12.003
RongXia, W., TaoYan, Y., WanQiu, Z., ShuJing, L., JunWang, T., Xue, D., et al. (2017). Effect of resveratrol on mRNA expression of chicken myokines. Chin. J. Vet. Sci. 37, 364–368.
Rosendal, L., Søgaard, K., Kjær, M., Sjøgaard, G., Langberg, H., and Kristiansen, J. (2005). Increase in interstitial interleukin-6 of human skeletal muscle with repetitive low-force exercise. J. Appl. Physiol. 98, 477–481. doi: 10.1152/JAPPLPHYSIOL.00130.2004
Ryall, J. G. (2012). The role of sirtuins in the regulation of metabolic homeostasis in skeletal muscle. Curr. Opin. Clin. Nutr. Metab. Care 15, 561–566. doi: 10.1097/MCO.0b013e3283590914
Safarpour, P., Daneshi-Maskooni, M., Vafa, M., Nourbakhsh, M., Janani, L., Maddah, M., et al. (2020). Vitamin D supplementation improves SIRT1, Irisin, and glucose indices in overweight or obese type 2 diabetic patients: a double-blind randomized placebo-controlled clinical trial. BMC Fam. Pract. 21:26. doi: 10.1186/S12875-020-1096-3/TABLES/6
Samant, S. A., Kanwal, A., Pillai, V. B., Bao, R., and Gupta, M. P. (2017). The histone deacetylase SIRT6 blocks myostatin expression and development of muscle atrophy. Sci. Rep. 7:11877. doi: 10.1038/s41598-017-10838-5
Schiaffino, S., and Reggiani, C. (2011). Fiber types in mammalian skeletal muscles. Physiol. Rev. 91, 1447–1531. doi: 10.1152/physrev.00031.2010
Seldin, M. M., Peterson, J. M., Byerly, M. S., Wei, Z., and Wong, G. W. (2012). Myonectin (CTRP15), a novel myokine that links skeletal muscle to systemic lipid homeostasis. J. Biol. Chem. 287, 11968–11980. doi: 10.1074/jbc.M111.336834
Serrano, A. L., Baeza-Raja, B., Perdiguero, E., Jardí, M., and Muñoz-Cánoves, P. (2008). Interleukin-6 is an essential regulator of satellite cell-mediated skeletal muscle hypertrophy. Cell Metab. 7, 33–44. doi: 10.1016/j.cmet.2007.11.011
Seto, E., and Yoshida, M. (2014). Erasers of histone acetylation: the histone deacetylase enzymes. Cold Spring Harb. Perspect. Biol. 6:a018713. doi: 10.1101/cshperspect.a018713
Severinsen, M. C. K., and Pedersen, B. K. (2020). Muscle–organ crosstalk: the emerging roles of myokines. Endocr. Rev. 41, 594–609. doi: 10.1210/ENDREV/BNAA016
Sharma, S., and Taliyan, R. (2016). Histone deacetylase inhibitors: future therapeutics for insulin resistance and type 2 diabetes. Pharmacol. Res. 113, 320–326. doi: 10.1016/j.phrs.2016.09.009
Sharples, A. P., Stewart, C. E., and Seaborne, R. A. (2016). Does skeletal muscle have an ‘epi’-memory? The role of epigenetics in nutritional programming, metabolic disease, aging and exercise. Aging Cell 15, 603–616. doi: 10.1111/acel.12486
Simoneau, J., Colberg, S. R., Thaete, F. L., and Kelley, D. E. (1995). Skeletal muscle glycolytic and oxidative enzyme capacities are determinants of insulin sensitivity and muscle composition in obese women. FASEB J. 9, 273–278. doi: 10.1096/fasebj.9.2.7781930
Song, S., Wen, Y., Tong, H., Loro, E., Gong, Y., Liu, J., et al. (2019). The HDAC3 enzymatic activity regulates skeletal muscle fuel metabolism. J. Mol. Cell Biol. 11, 133–143. doi: 10.1093/jmcb/mjy066
Staiger, H., Haas, C., Machann, J., Werner, R., Weisser, M., Schick, F., et al. (2009). Muscle-derived angiopoietin-like protein 4 is induced by fatty acids via peroxisome proliferator-activated receptor (PPAR)-δ and is of metabolic relevance in humans. Diabetes 58, 579–589. doi: 10.2337/db07-1438
Staron, R. S. (1997). Human skeletal muscle fiber types: delineation, development, and distribution. Can. J. Appl. Physiol. 22, 307–327. doi: 10.1139/h97-020
Steensberg, A., van Hall, G., Osada, T., Sacchetti, M., Saltin, B., and Pedersen, B. K. (2000). Production of interleukin-6 in contracting human skeletal muscles can account for the exercise-induced increase in plasma interleukin-6. J. Physiol. 529, 237–42. doi: 10.1111/J.1469-7793.2000.00237.X
Sun, Z., Singh, N., Mullican, S. E., Everett, L. J., Li, L., Yuan, L., et al. (2011). Diet-induced lethality due to deletion of the Hdac3 gene in heart and skeletal muscle. J. Biol. Chem. 286, 33301–33309. doi: 10.1074/jbc.M111.277707
Talbot, J., and Maves, L. (2016). Skeletal muscle fiber type: using insights from muscle developmental biology to dissect targets for susceptibility and resistance to muscle disease. Wiley Interdiscip. Rev. Dev. Biol. 5, 518–534. doi: 10.1002/wdev.230
Tanianskii, D. A., Jarzebska, N., Birkenfeld, A. L., O’sullivan, J. F., and Rodionov, R. N. (2019). Beta-aminoisobutyric acid as a novel regulator of carbohydrate and lipid metabolism. Nutrients 11:524. doi: 10.3390/nu11030524
Tanimura, Y., Aoi, W., Takanami, Y., Kawai, Y., Mizushima, K., Naito, Y., et al. (2016). Acute exercise increases fibroblast growth factor 21 in metabolic organs and circulation. Physiol. Rep. 4:e12828. doi: 10.14814/phy2.12828
Tezze, C., Romanello, V., and Sandri, M. (2019). FGF21 as modulator of metabolism in health and disease. Front. Physiol. 10:419. doi: 10.3389/fphys.2019.00419
Tezze, C., Romanello, V., Desbats, M. A., Fadini, G. P., Albiero, M., Favaro, G., et al. (2017). Age-associated loss of OPA1 in muscle impacts muscle mass, metabolic homeostasis, systemic inflammation, and epithelial senescence. Cell Metab. 25, 1374–1389.e6. doi: 10.1016/j.cmet.2017.04.021
Tsujinaka, T., Fujita, J., Ebisui, C., Yano, M., Kominami, E., Suzuki, K., et al. (1996). Interleukin 6 receptor antibody inhibits muscle atrophy and modulates proteolytic systems in interleukin 6 transgenic mice. J. Clin. Invest. 97, 244–249. doi: 10.1172/JCI118398
Turner, N., Cooney, G. J., Kraegen, E. W., and Bruce, C. R. (2014). Fatty acid metabolism, energy expenditure and insulin resistance in muscle. J. Endocrinol. 220, T61–T79. doi: 10.1530/JOE-13-0397
Tzika, E., Dreker, T., and Imhof, A. (2018). Epigenitics and metabolism in health and disease. Front. Genet. 9:361. doi: 10.3389/fgene.2018.00361
Villarroya, J., Gallego-Escuredo, J. M., Delgado-Anglés, A., Cairó, M., Moure, R., Gracia Mateo, M., et al. (2018). Aging is associated with increased FGF21 levels but unaltered FGF21 responsiveness in adipose tissue. Aging Cell 17:e12822. doi: 10.1111/acel.12822
Volmar, C. H., and Wahlestedt, C. (2015). Histone deacetylases (HDACs) and brain function. Neuroepigenetics 1, 20–27. doi: 10.1016/j.nepig.2014.10.002
Walsh, M. E., Bhattacharya, A., Sataranatarajan, K., Qaisar, R., Sloane, L., Rahman, M. M., et al. (2015). The histone deacetylase inhibitor butyrate improves metabolism and reduces muscle atrophy during aging. Aging Cell 14, 957–970. doi: 10.1111/acel.12387
Wang, J., Zhao, Y. T., Zhang, L., Dubielecka, P. M., Zhuang, S., Qin, G., et al. (2020). Irisin improves myocardial performance and attenuates insulin resistance in spontaneous mutation (Leprdb) mice. Front. Pharmacol. 11:769. doi: 10.3389/fphar.2020.00769
Wang, S., Wang, Y., Zhang, Z., Liu, Q., and Gu, J. (2017). Cardioprotective effects of fibroblast growth factor 21 against doxorubicin-induced toxicity via the SIRT1/LKB1/AMPK pathway. Cell Death Dis. 8:e3018. doi: 10.1038/cddis.2017.410
Watanabe, M., Singhal, G., Fisher, F. M., Beck, T. C., Morgan, D. A., Socciarelli, F., et al. (2020). Liver-derived FGF21 is essential for full adaptation to ketogenic diet but does not regulate glucose homeostasis. Endocrine 67, 95–108. doi: 10.1007/s12020-019-02124-3
Wei, W., Dutchak, P. A., Wang, X., Ding, X., Wang, X., Bookout, A. L., et al. (2012). Fibroblast growth factor 21 promotes bone loss by potentiating the effects of peroxisome proliferator-activated receptor γ. Proc. Natl. Acad. Sci. U. S. A. 109, 3143–3148. doi: 10.1073/pnas.1200797109
Willis, S. A., Sargeant, J. A., Thackray, A. E., Yates, T., Stensel, D. J., Aithal, G. P., et al. (2019). Effect of exercise intensity on circulating hepatokine concentrations in healthy men. Appl. Physiol. Nutr. Metab. 44, 1065–1072. doi: 10.1139/apnm-2018-0818
Wu, H., Naya, F. J., McKinsey, T. A., Mercer, B., Shelton, J. M., Chin, E. R., et al. (2000). MEF2 responds to multiple calcium-regulated signals in the control of skeletal muscle fiber type. EMBO J. 19, 1963–1973. doi: 10.1093/emboj/19.9.1963
Xie, T., and Leung, P. S. (2020). Roles of FGF21 and irisin in obesity-related diabetes and pancreatic diseases. J. Pancreatol. 3, 29–34. doi: 10.1097/JP9.0000000000000039
Xiong, Y., Wu, Z., Zhang, B., Wang, C., Mao, F., Liu, X., et al. (2019). Fndc5 loss-of-function attenuates exercise-induced browning of white adipose tissue in mice. FASEB J. 33, 5876–5886. doi: 10.1096/FJ.201801754RR
Xu, F., Liu, J., Na, L., and Chen, L. (2020). Roles of epigenetic modifications in the differentiation and function of pancreatic β-Cells. Front. Cell Dev. Biol. 8:748. doi: 10.3389/fcell.2020.00748
Yakabe, M., Hosoi, T., Akishita, M., and Ogawa, S. (2020). Updated concept of sarcopenia based on muscle–bone relationship. J. Bone Miner. Metab. 38, 7–13. doi: 10.1007/s00774-019-01048-2
Yamauchi, T., Kamon, J., Waki, H., Terauchi, Y., Kubota, N., Hara, K., et al. (2001). The fat-derived hormone adiponectin reverses insulin resistance associated with both lipoatrophy and obesity. Nat. Med. 7, 941–946. doi: 10.1038/90984
Yang, Z., Chen, X., Chen, Y., and Zhao, Q. (2015). PGC-1 mediates the regulation of metformin in muscle irisin expression and function. Am. J. Transl. Res. 7, 1850–1859.
Yudkin, J. S., Kumari, M., Humphries, S. E., and Mohamed-Ali, V. (2000). Inflammation, obesity, stress and coronary heart disease: is interleukin-6 the link? Atherosclerosis 148, 209–214. doi: 10.1016/S0021-9150(99)00463-3
Zhang, H., Ren, E., Xu, R., and Su, Y. (2021). Transcriptomic responses induced in muscle and adipose tissues of growing pigs by intravenous infusion of sodium butyrate. Biology (Basel) 10:559. doi: 10.3390/BIOLOGY10060559
Zhang, J., Cheng, Y., Gu, J., Wang, S., Zhou, S., Wang, Y., et al. (2016). Fenofibrate increases cardiac autophagy via FGF21/SIRT1 and prevents fibrosis and inflammation in the hearts of Type 1 diabetic mice. Clin. Sci. 130, 625–641. doi: 10.1042/CS20150623
Zhao, Y. T., Wang, H., Zhang, S., Du, J., Zhuang, S., and Zhao, T. C. (2016). Irisin ameliorates hypoxia/reoxygenation-induced injury through modulation of histone deacetylase 4. PLoS One 11:e0166182. doi: 10.1371/journal.pone.0166182
Zheng, S. L., Li, Z. Y., Song, J., Liu, J. M., and Miao, C. Y. (2016). Metrnl: a secreted protein with new emerging functions. Acta Pharmacol. Sin. 37, 571–579. doi: 10.1038/aps.2016.9
Zhu, S., Ma, L., Wu, Y., Ye, X., Zhang, T., Zhang, Q., et al. (2014). FGF21 treatment ameliorates alcoholic fatty liver through activation of AMPK-SIRT1 pathway. Acta Biochim. Biophys. Sin. (Shanghai) 46, 1041–1048. doi: 10.1093/ABBS/GMU097
Keywords: HDACs, tissue crosstalk, soluble factors, epigenetics, HDAC inhibitors (HDACi)
Citation: Renzini A, D’Onghia M, Coletti D and Moresi V (2022) Histone Deacetylases as Modulators of the Crosstalk Between Skeletal Muscle and Other Organs. Front. Physiol. 13:706003. doi: 10.3389/fphys.2022.706003
Received: 06 May 2021; Accepted: 31 January 2022;
Published: 18 February 2022.
Edited by:
Bruno Bastide, Lille University of Science and Technology, FranceReviewed by:
Tomohiro Nakamura, Osaka Institute of Technology, JapanGiuseppe Banfi, Galeazzi Orthopedic Institute, Scientific Institute for Research, Hospitalization and Healthcare (IRCCS), Italy
Copyright © 2022 Renzini, D’Onghia, Coletti and Moresi. This is an open-access article distributed under the terms of the Creative Commons Attribution License (CC BY). The use, distribution or reproduction in other forums is permitted, provided the original author(s) and the copyright owner(s) are credited and that the original publication in this journal is cited, in accordance with accepted academic practice. No use, distribution or reproduction is permitted which does not comply with these terms.
*Correspondence: Dario Coletti, ZGFyaW8uY29sZXR0aUB1bmlyb21hMS5pdA==