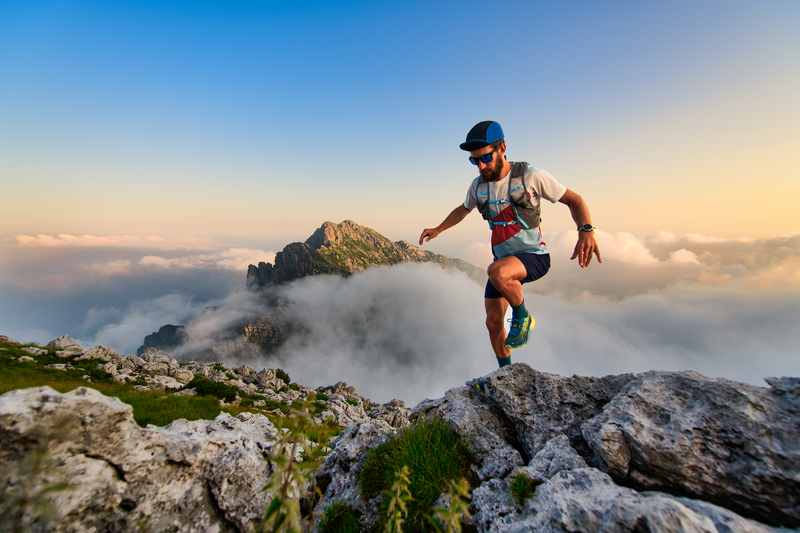
95% of researchers rate our articles as excellent or good
Learn more about the work of our research integrity team to safeguard the quality of each article we publish.
Find out more
REVIEW article
Front. Physiol. , 09 January 2023
Sec. Invertebrate Physiology
Volume 13 - 2022 | https://doi.org/10.3389/fphys.2022.1112278
This article is part of the Research Topic Physiological Events Associated with Pesticide-Resistance View all 6 articles
Threatening the global community is a wide variety of potential threats, most notably invasive pest species. Invasive pest species are non-native organisms that humans have either accidentally or intentionally spread to new regions. One of the most effective and first lines of control strategies for controlling pests is the application of insecticides. These toxic chemicals are employed to get rid of pests, but they pose great risks to people, animals, and plants. Pesticides are heavily used in managing invasive pests in the current era. Due to the overuse of synthetic chemicals, numerous invasive species have already developed resistance. The resistance development is the main reason for the failure to manage the invasive species. Developing pesticide resistance management techniques necessitates a thorough understanding of the mechanisms through which insects acquire insecticide resistance. Insects use a variety of behavioral, biochemical, physiological, genetic, and metabolic methods to deal with toxic chemicals, which can lead to resistance through continuous overexpression of detoxifying enzymes. An overabundance of enzymes causes metabolic resistance, detoxifying pesticides and rendering them ineffective against pests. A key factor in the development of metabolic resistance is the amplification of certain metabolic enzymes, specifically esterases, Glutathione S-transferase, Cytochromes p450 monooxygenase, and hydrolyses. Additionally, insect guts offer unique habitats for microbial colonization, and gut bacteria may serve their hosts a variety of useful services. Most importantly, the detoxification of insecticides leads to resistance development. The complete knowledge of invasive pest species and their mechanisms of resistance development could be very helpful in coping with the challenges and effectively developing effective strategies for the control of invasive species. Integrated Pest Management is particularly effective at lowering the risk of chemical and environmental contaminants and the resulting health issues, and it may also offer the most effective ways to control insect pests.
Invasive species pose risks to human health, food security, the survival of endangered species, economic loss, and ecosystem stability (Venette et al., 2021). Invasive pest species are also known by the names non-native, exotic, non-indigenous, and introduced. The human race is responsible for its introduction, whether on purpose, by accident, or through trade. Besides the obvious danger to biodiversity, these introduced species also pose a significant risk to national biosecurity (Rao et al., 2018; Raghuteja et al., 2022). Species can now be transported to new regions of the world at an extraordinary pace due to the rapid expansion of the world’s commercial and social networking. Awareness of the negative effects of invasive species on local biodiversity and the resulting economic loss in many nations has grown over the past two decades (Pimentel et al., 2005; Zhang et al., 2022). Recent research into the evolutionary genetics of invasive species has found that the ability to adapt to natural selection may be more important for the successful invasion of some introduced species than widespread physiological plasticity or tolerance. Therefore, it can unintentionally boost the invasive species’ evolutionary potential and enable its quick growth and geographical distribution in the area of introduction (Lavergne and Molofsky, 2007; Marbuah et al., 2014; Siddiqui et al., 2021; Venette et al., 2021; Hafeez et al., 2022b).
As arthropods spread outside of their natural habitats, they meet naïve animals and host plants as well as population-controlling pathogens, parasitoids, and predators. Opportunities to dominate and modify invaded areas arise when antagonistic and coevolutionary relationships among invasive species are disrupted. The first introduction of invasive species (Grape phylloxera pest, Daktulosphaira vitifoliae) was reported in Europe about the middle of the 19th century. At the same time, the emerald ash borer, Agrilus planipennis, landed in North America and Europe in the early twenties. The fall armyworm, Spodoptera frugiperda, devours maize in Africa, causing a direct impact on food or the environment (Sileshi et al., 2019), Darwin’s finches in the Galapagos were under attack by invading flies (Philornis downsi) (Fessl and Tebbich, 2002; Koop et al., 2021), or red turpentine beetle (Dendroctonus valens) has a devastating effect on China’s pine forests (Sun et al., 2013). Indirect effects can be more subtle, such as when the brown marmorated stink bug (Halyomorpha halys) spreads an aflatoxin-producing fungus in the United States (Opoku et al., 2019), or In South America, dengue fever is spread by the Asian tiger mosquito (Aedes albopictus) (Ricas Rezende et al., 2020), and Xylella fastidiosa, a non-native bacteria, is spread via spittlebugs (Occhibove et al., 2020). Indirect consequences on water, health, and the environment may result from measures taken in response to arthropod invasions, particularly if widespread use of pesticides is required (Milano and Chèvre, 2019).
Each year, the world’s food supply faces a 10%–30% loss due to insect pests (Oerke, 2006) and exotic species-related damages worth $20 billion (Pimentel et al., 2000). That is why it is so important to keep the pests that have affected our economy under control (Savary et al., 2012). Insecticide use is thought to be the most effective pest control technique. Pesticides are heavily used in managing the pest in the current era. Consequently, one billion kilos of chemicals are used annually to combat crop pests (Alavanja, 2009; Drees et al., 2013). In several countries, pesticide usage has increased, i.e., Britain (18,000 tons), Italy (62,000 tons), Germany (4,800 tons), Poland (2,400 tons), and China (273,375 tons) (FAOSTAT Food and Agriculture Organization of the United Nations, 2020). Despite their effectiveness, synthetic pesticides have raised questions about resistance to pests, environmental degradation, and potential health impacts are all reasonable concerns (Du et al., 2020).
Insecticides are used to eliminate pests and disease-carrying insects in farming. Several insecticides, including organophosphates, pyrethroids, and neonicotinoids, play pivotal roles in pest management (Abdulahi et al., 2011). One of the most prominent examples of micro-evolution is the development of pest resistance due to the widespread usage of insecticides. More than 500 distinct pest species have been documented as having developed resistance to insecticides, according to previous research (Connor et al., 2011). Numerous pests, like corn earthworms and others, feed on a wide variety of crops around the world (cotton, peanuts, tobacco, etc.) and have developed resistance to insecticides. Several elements, biological, genetic, and operational, contribute to the emergence of resistance, but genetic aspects are regarded as the most helpful (Karaağaç, 2012). However, future populations showing increasing insecticide resistance make pest control more difficult (Stratonovitch et al., 2014). Insects have been found to use a variety of molecular resistance mechanisms, including metabolic and target sites.
Understanding species-level worldwide patterns are also helpful from a biosecurity aspect. Resistance-containing species pose a significant concern as intruders as they can build new populations that are already evolved to insecticidal stress. Invasive populations of the Silverleaf white fly, Bemisia tabaci, are just one example of a historical problem in Australia (Thia et al., 2021). Similarly, after the introduction of western flower thrips, Franklieniella occidentalis, into Australia, many of the chemical pesticides used were ineffective against the insect (Reitz and Funderburk, 2012). However, numerous urban pests are resistant to pesticides (e.g., cockroaches, houseflies, mosquitoes, ants, wasps, and termites) (Acevedo et al., 2009; Liu, 2015; Mahapatro, 2017; Fardisi et al., 2019). Even though insects can negatively affect agriculture, public health, and the environment, effective methods of controlling them are lacking (Hardy, 2014).
Cumulative global knowledge of resistance mechanisms helps develop hypotheses and expectations at more localized scales. The current review literature beam spotlights available knowledge on invasive species and broadly expands on the various species that have been documented to develop a broad spectrum of resistance against various classes of chemical insecticides. Moreover, possible mechanisms of insecticide resistance in invasive species, their management strategies, and future implication are also discussed.
Pesticide-induced resistance is defined as a heritable modification in a pest population’s sensitivity to pesticides, as evident in the repeated failure of the treated chemical products to achieve the required control (Mahapatro, 2017). Resistance studies are essential in developing strategies for resistance mitigation and reducing possible insect pest outbreaks. A large number of invasive insect species have been examined and found to exhibit resistance to various classes of insecticides in China (Table 1), and in some other parts of the world (Table 2).
There is various mechanism mediating insecticide resistance development in insects. The major factors are behavioral resistance, fitness cost, reduced penetration, target resistance, and metabolic resistance. The mechanism underlying insecticide resistance in insects can generally be categorized as follows.
1) Behavioral resistance
2) Fitness cost
3) Penetration resistance
4) Target-site resistance
5) Metabolic resistance
6) Resistance-inducing operational factors
In the very first line of protection, organisms might develop strategies to lessen their exposure to the uptake of a pesticide (Dunlop et al., 2018; Lushchak et al., 2018). Insects can develop resistance to chemicals through a number of different mechanisms, but an early and important one is a behavior response (Nansen et al., 2016) (Figure 1). When exposed to a lethal toxin, insects may often cease feeding and may even leave the treated area by simply moving from one field to another or into a deeper crop canopy (De Roode and Lefèvre, 2012; IRAC, 2022).
FIGURE 1. Schematic diagram of the biological and behavioral mechanisms of insecticide resistance in invasive insects.
Behavioral resistance was well defined in a previous study by Sparks et al. (1989) as “evolved behavior that reduces an insect’s exposure to toxic compounds or that allows an insect to survive in what would otherwise be a toxic and fatal environment”. To survive, arthropod pests use behavioral processes, which involve shifts in behavior and stay away from areas that have been sprayed with insecticides (Sparks et al., 1989). Insect movement can have a significant effect on the growth of insecticide resistance. Movement characteristics of an insect cannot determine the extent of insecticide exposure, impacting the selection pressure on the insect population. It can also determine the degree to which insect populations mix in the ecosystem, thus affecting the ability of resistant ales to increase in resistance. For instance, the Colorado potato beetle (Leptinotarsa decemlineata) has limited flight capabilities, so pockets of insecticide resistance have developed in growing regions across North America (Alyokhin et al., 2008). For instance, Plutella xylostella Linnaeus, an invasive species, has developed behavioral resistance by using site selection for egg laying in adults and larval mobility variations to escape lethal dosages of foliar-applied insecticides in the field (Sarfraz et al., 2005; Zago et al., 2014). da Silva Nunes et al. (2020) found variation in the locomotor activity of P. xylostella adults between laboratory and field-collected samples. Insects collected from the field exhibited elevated activity and decreased resting time compared to controls, suggesting that they were motivated to escape from treated surfaces. Host plant evasion may have resistance traits, such as producing more eggs or preferring to oviposit on plants with less hairy and delicate cuticles, as evidenced by research demonstrating these insects have evolved to avoid insecticides (Silva and Furlong, 2012; Ang et al., 2014). Some other insects, such as female sheep blowflies (Lucillia cuprina), developed the ability to delay ovulation in the presence of a pesticide intended for their larvae (Mariath et al., 1990).
Red Imported Fire Ants (RIFA) are the best example of behavioral resistance because their excellent social system benefits their success (Dhami and Booth, 2008; Bertelsmeier et al., 2017; Jones and Robinson, 2018; Giraldo et al., 2021). Various behaviors play a role in developing resistance in RIFA, such as antibiotic secretions and adaptive immunological responses of colony members (Wilson-Rich et al., 2009), self- and mutual grooming by ant nestmates can restrict or accelerate infection and toxin transmission (Theis et al., 2015). Moreover, the effective waste removal of unhealthy materials (including dead ants). The ants regularly clean and disinfect each other and the infected ants they come into contact with (Pull et al., 2018). Examples of behavioral resistance occurred in recent studies, Wen et al. (2020) reported that invasive fire ant (Solenopsis invicta) deposited soil particles on the ant replant to avoid contact. To minimize pesticide contact, another study found that RIFA carried debris particles to cover the pesticide-treated region (Wen et al., 2021). When social ants come into touch with previously immunized nestmates, their ability to resist infection significantly improves (Konrad et al., 2018). This social transfer of infection resistance could explain how colony members’ survival rates are raised due to group life, despite the higher risks of transmission of alien agents (pesticides) that occur (Traniello et al., 2002). Pesticide resistance has been recently found in RIFA, which might be the reason for the inability to manage urban invasive ant species (Allen et al., 2018). The ineffectiveness of numerous biological and chemical control programs to control the invasive species could be attributed to a lack of understanding of ant behavior and the habits mentioned above, among several other factors.
The biological and ecological factors discussed here constantly interact to impact the risk of resistance development. Life cycle and population factors are important biological parameters.
Life cycle: An overriding aspect of insect biology that impacts an insect’s ecological interactions and the development of pesticide resistance is the insect’s life cycle (Sudo et al., 2018). Through a pest’s life cycle, there are changing interactions with its host and environment. Optimal resistance management practices rely on an understanding of these interactions. The life cycle of the insect pest is the primary factor affecting the development of insecticide resistance; in particular, the long life processes in bugs (Saulich, 2010) and short life cycle with the abundant progeny of mosquitoes which has all the properties suitable to swiftly developing resistance (Karunamoorthi and Sabesan, 2013).
The insects have different reproductive phases that influence resistance development through behavioral and genetic effects. Moreover, some ecological factors, such as environmental or host quality, will affect the reproduction phase (Helps et al., 2017). Most insects undergo sexual reproduction, involving a male and female union, and the resulting genetic recombination increases the genetic variability in a population. For example, sexual reproduction in two-spotted spider mites (Tetranychus urticae) enhanced the genetic variability in its population. It allowed for an increased potential for the development of resistance (Sun J. et al., 2022). On the other hand, asexual reproduction involves no male-female union, with the best example found in aphids (Stöck et al., 2021) (Figure 1). This will increase the reproduction rate, but it will also limit the genetic variability in a population. Multiple generations of asexually reproducing aphids, such as the green peach aphid (Myzus persicae Sulzer), are produced each year. Still, only a single sexual generation is likely to occur (Guillemaud et al., 2003). Among these reproductive phases, the insects undergo the development phase, which is a maturation process. Organisms vary a great deal in how these occur. Selection pressure can often occur throughout this life cycle phase. For example, the Housefly goes through distinct development stages (e.g., egg, pupa, adult fly) as it grows (de Jonge et al., 2020). Moreover, the sweet potato white fly, B. tabaci, has been shown to be resistant to neonicotinoids. The effects of thiamethoxam resistance selection on the life histories of B. tabaci B-biotype strains were studied by comparing those of selected and non-selected strains over multiple generations (Feng et al., 2009). The resistant individuals had shorter life spans and lower fecundity than the susceptible unselected strain. On the other hand, the nymphal stages took longer to mature in the resistant strain. Further phenotypic changes were seen in the resistant strain, with reduced body size across all instars and pupal stages compared to the susceptible strain (Feng et al., 2009). So, the life span of the insect pest, its reproductive capacity, and its surrounding habitat are crucial elements in the evolution of resistance (Naqqash et al., 2016). An insect’s lifecycle and developmental period play a crucial part in the growth of insecticide resistance (Figure 1).
Population growth: The growth of insect populations influences resistance development by determining the most successful individuals. Intense selection pressures from insecticide use, short generation time, and readily available host crops have resulted in diamondback moth (P. xylostella) resistance to almost all groups of insecticides (Taha, 2022; Venkatesan et al., 2022). The intrinsic rate of increase is the rate that an insect population will increase without external constraints. When populations are affected by biological or environmental constraints, the growth rate slows as it approaches a carrying capacity (large population size that is sustainable) (Holt, 2009). Insect populations are regulated by multiple factors, e.g., environmental conditions, host quality, and natural enemies. In the example shown, insect predator populations lag behind prey populations, but prey population decline after predator populations build up (Culshaw-Maurer et al., 2020). The invasive insects invade a new place where they do not have a natural enemy, and the environmental condition is suitable for their survival, consequently, reasons for their success. For example, Solenopsis invicta invades many countries, can increase their population, and dominates the local fauna (Morrison et al., 2004; Siddiqui et al., 2021) (Figure 1).
Generation time is also important in the time required for individuals to complete their life cycle. This ranges from multiple years per life cycle, as in the case of large pine weevil (Hylobius abietis) (Inward et al., 2012), to numerous life cycles per year in Mosquitoes (Mendoza, 2016). This allows for more genetic recombination, increasing genetic diversity and the probability of selecting resistant alleles. The population with multiple generations per year would more likely develop resistance to a pesticide because there would be more selection cycles (chances) to select for or to raise the percentage of resistant ones in the final population. For example, mosquitoes have multiple generations in short periods, which increases the possibility of the transfer of resistance alleles to the next generations and increases their genetic diversity, leading to the resistant generation (Mendoza, 2016). Another invasive species, Diamondback moths, can undergo multiple generations annually, increasing the potential for selection pressure across the population (Kliot and Ghanim, 2012) (Figure 1).
The insect cuticle comprises two major layers of polysaccharide chitin, lipids, and proteins. The chitin is in the inner procuticle, but there is no chitin in the thin outer epicuticles (Bass and Jones, 2016). By coating cuticular hydrocarbons (CHCs) generated in particular secretory cells in the epidermis called oenocytes on their epicuticle, insects are able to protect themselves from drying out (Falcon et al., 2019). Penetration resistance occurs when insects slow down the engagement of xenobiotics within their bodies. Insects create barriers against the product using their outer cuticle, which protects them against a wide spectrum of insecticides (Figure 2) (Tangtrakulwanich and Reddy, 2014; Balabanidou et al., 2016). Because of this slowing process, insecticides may take significantly longer to reach their protein targets in neuronal cells (Fang et al., 2015). Scanning electron microscopy indicated that resistant mosquitos have significantly increased cuticle thickness, with the outer epicuticle accounting for much of the entire difference in whole cuticle thickness (Balabanidou et al., 2016). Some adaptations known as penetration mechanisms slow the rate at which a pesticide is absorbed through the cuticle (Gunning et al., 1991; Ahmad et al., 2006). Evidence of penetration experimentation is frequently used to infer resistance (Ahmad et al., 2006; Puinean et al., 2010); however, in order to distinguish expression differences in cuticle-related genes between resistant and susceptible strains, transcriptomics has also been applied (Thia et al., 2021). However, minimal studies on cuticular resistance in invasive insects such as P. xylostella revealed a significant difference in cuticular microRNAs between the chlorantraniliprole resistance population and the susceptible population. They give insight into cuticular alteration during the development of resistance (Zhu et al., 2017; Shabbir et al., 2021). There is evidence of cuticular resistance in H. armigera, which successfully prevented deltamethrin adsorption in the body by generating thicker cuticles in resistant individuals compared to susceptible individuals, where insecticide percolation was substantially higher (Ahmad et al., 2006). According to one study on Amyelois transitella (Lepidoptera: Pyralidae), when an insect is repeatedly subjected to pesticide pressure, the hydrocarbon profile in the cuticle tends to change, which may help to build cuticular resistance (Ngumbi et al., 2020).
FIGURE 2. A schematic diagram represents the role of enzymes and gut microbiota in pesticide detoxification in invasive ants.
Thickening or altering the chemical makeup of the insect cuticle reduces toxicant uptake. A similar mechanism was observed in OP-resistant strains of Culex tarsalis (Whyard et al., 1994) and C. quinquefasciatus (Gong et al., 2022). The variation in pesticide transport across the cuticle of houseflies (Musca domestica Linnaeus) was also demonstrated (Malik et al., 2007). Similarly, organophosphates, methyl carbamates, pyrethroids, and neonicotinoids resistance in Tribolium castaneum (Rösner et al., 2020), OP resistance in Colorado potato beetle (Lepinotarsa decemilineata) (Yoon et al., 2022), and fenvalerate resistance in P. xylostella (Mubashir and Seram, 2022), have all been confirmed to be partly due to reduced penetration (Figure 2). This mechanism confers low levels (less than 5 - fold) of resistance and only becomes important when combined with other resistant mechanisms. On the other hand, it appears to shield several different classes of pesticides (Venkatesan et al., 2022). Comparing the amount of pesticide taken over time by resistant and susceptible types of insects is the simplest technique to calculate the penetration rate. Although, even a slight reduction in penetration might contribute significantly to the invasive insect developing resistance to the insecticide (Venkatesan et al., 2022).
Insecticides are chemicals (synthetic compounds or direct biological materials) used to control insect pests (Wojciechowska et al., 2016). However, the most successful synthetic insecticides are neuro-inhibitors (Figure 2). Despite the increasing cost and the risk of environmental contamination, these are the world’s most widely used agents of insect pest control today (Yadav et al., 2022). Our study mainly discussed commonly used four classes of organic or synthetic pesticides, and they are as follows: organochlorines, organophosphates (OPs), carbamates, and pyrethroids (Table 3). All of these pesticides target the insect’s central nervous system. For organochlorines, the neurological system is the primary target. The inhibitory neurotransmitter gamma-aminobutyric acid (GABA) attaches to a specific type of chloride channel called a GABA-gated chloride channel, and blocking these channels leads to increased excitability in neurons (Al-Kuraishy et al., 2022). The enzyme that breaks down acetylcholine, a neurotransmitter, is the target of carbamates and OPs. Other organochlorines and pyrethroids inhibit nerve impulse transmission by binding to and blocking the function of Nat channel proteins in the neuron membrane (Lopez-Suarez et al., 2022). Pyrethroids affect the sodium channels and lead to paralysis of the organism. When they attach to sodium channels, they trigger excitatory paralysis and eventually death in insects (Davies et al., 2007).
Biologically modified insects may be resistant to insecticides because they have been altered genetically to inhibit the insecticide from binding or interacting at its site of action (Fenibo et al., 2022). The second most researched resistance mechanism in a wide variety of insects is target site insensitivity. As a result of changes in the target site’s structure or accessibility, toxicants may no longer form stable bonds with them, a phenomenon known as target-site insensitivity (Venkatesan et al., 2022). Aphids have developed a resistance to pyrethroids by developing resistance at the target site (knockdown resistance-kdr) (Valmorbida et al., 2022).
There are four major types of target sites such as 1) The insensitivity of acetylcholine esterase (AChE) has been identified as an essential mechanism for pesticide resistance in numerous agricultural insect pests to carbamates and organophosphates (Renault et al., 2022). Esterases are a diverse family of enzymes responsible for breaking down a wide range of ester bonds in both endogenous and exogenous substrates (Memarizadeh et al., 2011) and play important roles in the insect’s ability to eliminate the toxic effects of insecticides. The enzyme acetylcholinesterase (AChE) is the target of both organophosphate and carbamate insecticides because of its central role in the nervous system in hydrolyzing cholinergic neurotransmitters and terminating nerve impulses (Devrnja et al., 2022). Neonicotinoids, organophosphates, and methyl carbamates act as acetylcholinesterase inhibitors or as nicotinic acetylcholine receptor agonists (Devrnja et al., 2022). Prior to inhibiting acetylcholinesterase (AChE), organophosphorus insecticides must be transformed into their oxon analogs by monooxygenases (Lorke and Petroianu, 2019) (Figure 2). 2) Sodium channel blocker insecticides (SCBIs) are a relatively new group of pesticides exemplified by metaflumizone and indoxacarb, which are commercially registered chemicals. It is well-known that SCBIs like pyrethroids and DDT intoxicate insects by blocking their access to voltage-gated sodium channels (VGSCs) (Cens et al., 2022). The sodium channel in nerve cells is disrupted by KDR (knockdown resistance). This mechanism is widely exploited in pyrethroid and DDT resistance. Kdr and super kdr are the results of a number of different mutations (Sun H. et al., 2022). 3) For insects, the nicotinic acetylcholine receptors (nAChRs) play a crucial role in learning and memory due to their participation in fast neurotransmission (Taillebois and Thany, 2022). Resistance to imidacloprid has been widely investigated in B. tabaci, Aphis gossypii, and M. persicae, due to modifications in and subunit of nAChR (Xu et al., 2022; Zhou et al., 2022). Synthetic insecticides like neonicotinoids and spinosad aim to target nAChRs because of their essential role in insect neurotransmission (Kaleem Ullah et al., 2022). d) The GABA receptor is primarily a Cl—channel, and GABA receptor molecules are essential to target locations for multiple chemically diverse kinds of pesticide-active chemicals, resulting in fipronil, cyclodienes, and avermectins resistance (Burman et al., 2022; Venkatesan et al., 2022).
Metabolic resistance is a type of resistance inferred by metabolic activities in insects that help them detoxify or break down contaminants or the ability to eliminate toxic compounds from their bodies more quickly (Venkatesan et al., 2022). Insect metabolism is critical in the development of pesticide resistance against various groups of chemical pesticides, including carbamates, organophosphates, and synthetic pyrethroids. Insects metabolize insecticides to less toxic or non-toxic forms via a mechanism called “detoxification” (Jaffar et al., 2022). Metabolic resistance is of huge importance and is also one of the most studied mechanisms in insects. Insects use their enzymatic systems to digest pesticides, and resistant populations may have more of these enzymes or enzymes with improved detoxifying capabilities (Karunaratne and Surendran, 2022). Furthermore, to become more effective, these enzyme systems may also be able to break down many different types of pesticides (Vyas et al., 2022). Detoxifying enzymes enhance and accelerate the drug resistance caused by the metabolism of insecticides (Siddiqui et al., 2022c; Siddiqui et al., 2022b). The over-produced enzymes in pests can develop protection against insecticides (Figure 2) (Rane et al., 2016; Khan et al., 2020).
Carboxylesterases (CarEs) are an adaptable class of lipolytic enzymes that catalyze the hydrolysis of esters into alcohol and acid molecules. Widespread biocatalysis, drug metabolism, and endobiotic and xenobiotic degradation rely on these enzymes (Sood et al., 2016). CarEs enzymes also detoxify environmental toxicants such as pyrethroids, a major insecticide class used worldwide (Staudinger et al., 2010). Recent studies on invasive species indicated that a greater AChE and CarE esterase activity was seen in resistant populations compared to vulnerable ones such as S. invicta (Siddiqui et al., 2022b; 2022c), H. armigera (Young et al., 2005; Wu et al., 2011), C. tarsalis (Whyard et al., 1994), and Aonidiella aurantia (Grafton-Cardwell et al., 2004) (Figure 2).
Biochemical methods using S. litura resistant strains from Korea and India have shown the role of acetylcholinesterases in pesticide resistance (Yonggyun et al., 1998; Kranthi et al., 2002; Muthusamy et al., 2011). In the same way, a carbamate-resistant strain of S. exigua from California had an AChE enzyme that was about 30 times less sensitive to methomyl than the enzyme from a sensitive laboratory strain (Byrne and Toscano, 2001). However, molecular information on the AChE point mutation exists for a number of species of Spodoptera, while it is mostly available for S. frugiperda. When comparing two strains of this species, Yu et al. (2003) found that acetylcholinesterase from a field strain taken from Florida corn fields was up to 85-fold less responsive to inhibition by CBs and Ops (Hilliou et al., 2021).
The CarE activity reported by various studies, such as alpha and beta esterase production, when exposed to fipronil, was greater in a resistant strain of the mosquito (Culex quinquefasciatus) than in a susceptible strain (Sarkar et al., 2009). Increased activity of CarE was detected in beta-cypermethrin-resistant M. domestica when compared to beta-cypermethrin-susceptible ones (Zhang et al., 2007). Moreover, elevated esterases activity was reported after insecticides exposure to the peach potato aphid (M. persicae) (Bass and Field, 2011; Venkatesan et al., 2022) and the brown planthopper (Nilaparvata lugens) (Tang et al., 2022). This means that elevated esterases can detoxify the insecticides. Gene amplification is the cause of the increased production of these enzymes (Şengül Demirak and Canpolat, 2022). In mosquitoes, amplified esterase molecules are more reactive with insecticides than non-amplified esterases (Gan et al., 2021).
Insecticide detoxification by CarE has also been reported in other insect species. For example, some previous studies have indicated an increase in the activity of CarE in honeybees following exposure to fipronil (Carvalho et al., 2013; Roat et al., 2017). Similarly, the grassland locust, Epacromius coerulipes Ivanov (Orthoptera: Acrididae), showed a significantly higher CarE activity in a strain that had evolved resistance against fipronil than in a susceptible strain (Jin et al., 2020). Insecticide metabolism studies indicated that esterases are involved in resistance mechanisms. Increased accumulation of esterase hydrolytic products in insecticide-resistant insects compared to susceptible gives evidence for the involvement of these enzymes (Jensen, 2000; Silva et al., 2012; Khan et al., 2021b; Siddiqui et al., 2022b).
Glutathione transferases (GSTs) are a broad and diversified family of enzymes that are present in almost all organisms. GST is a class of multifunctional proteins that are extremely important in the biological transformation of exogenous compounds, drug metabolism, and protection from peroxidation (Hollman et al., 2016; Dasari et al., 2018). It was discovered that the house fly M. domestica contains DDT-dehydrochlorinase as a glutathione S-transferase (Clark, 1989). They are crucial for the detoxification of both internal and external substances, intracellular movement, the generation of hormones, and the prevention of oxidative stress (Hassan et al., 2019). GST is one of the foremost detoxifying enzymes of insecticides in insects. Insecticides can be metabolized either by reductive dehydrochlorination, which is facilitated by GSTs, or by conjugation reactions with reduced glutathione, which result in water-soluble metabolites that are more easily eliminated. Additionally, they help to remove dangerous oxygen-free radical species that are formed as a result of the use of insecticides (Panini et al., 2016). Neonicotinoid resistance in the B. tabaci is correlated with constitutive overexpression of several ESTs, GSTs, UGTs, CYPs, and ABC transporters (Vassiliou et al., 2011) (Figure 2).
Several major insecticide types, such as boric acid, carbamates, organophosphorus, organochlorine, and pyrethrin-resistant in German cockroaches, have increased their GST expression levels to varying degrees (Gentz and Grace, 2006; Nasirian, 2010; Rinkevich et al., 2013). Studies on invasive fire ants show that exposure to indoxacarb, beta-cypermethrin, and fipronil causes a substantial alteration in GST activity. Additionally, the outcomes demonstrate that population resistance and sublethal concentrations substantially impact enzyme activity (Siddiqui et al., 2022c; Siddiqui et al., 2022b). OP and DDT resistance mechanisms were reported in houseflies (Ranganathan et al., 2022). In a resistant strain of M. domestica, Zhang et al. (2007) discovered that GST activity was also markedly elevated. According to Sarkar et al. (2009), the GST activity of all populations tested was greater than that of a susceptible laboratory strain. Studying orthopterans, researchers found that a particular strain of E. coerulipes had much higher GST activity than susceptible insects (Jin et al., 2020). Insecticide treatments for maize rootworms have already been linked to an increase in GST activity, according to a recent study (Souza et al., 2020). Pest resistance has already been reported in different insects against DDT and OP.
An essential supergene family, cytochrome P450 (P450 or CYP), is responsible for the metabolism or attenuation of toxicity of numerous potentially harmful substances (Zhu et al., 2017; Hafeez et al., 2022b) (Figure 2). There are four clades in insects where the CYP gene can be placed, including CYP2, CYP3, CYP4, and the mitochondrial CYP clade (Nelson, 2011; Zhang et al., 2018). Numerous insect cytochrome P450s (CYPs) have been split into eleven families (Hafeez et al., 2022b). Numerous insect CYPs make up the CYP3 clan of cytochrome P450s, which is further divided into numerous CYP9 family members known to take part in detoxifying processes linked to pesticide resistance (Schuler, 2011; Hafeez et al., 2019). P450 enzymes play an important part in the phase I process of detoxification of many different types of hazardous chemicals, including insecticides, due to their genetic diversity, broad substrate specialization, and catalytic adaptability (Kim et al., 2022). For herbivorous insects, the main mechanism of pesticide resistance is the overexpression of cytochrome P450 detoxifying enzymes (Hafeez et al., 2020b; Wu et al., 2021).
Different P450 enzymes serve different purposes in insects, such as hormone synthesis and regulation, growth and development control, or the digestion of xenobiotic substances (Zhou et al., 2010; Nelson et al., 2013). Due to their central function in pesticide metabolism, cytochrome P450s are frequently implicated in the development of insect resistance to these chemicals (Guo et al., 2012; Panini et al., 2016). One of the most prevalent processes by which insect pests develop resistance to synthetic insecticides is the upregulation of certain detoxifying P450 enzymes within the insect (Nelson, 2011; Hafeez et al., 2022a; Luo et al., 2022). Because of this, insecticide resistance may develop as a result of changes in the activity of detoxifying enzymes in insects in response to exposure to pesticides. Many insect orders, including Coleoptera, Diptera, Hemiptera, Hymenoptera, and Lepidoptera, have shown evidence of P450 overexpression leading to increased resistance to pesticides (Bass et al., 2011; Johnson et al., 2012; Liang et al., 2015; Wang et al., 2015; Chen et al., 2017; Khan et al., 2021a; Khan et al., 2021c; Hafeez et al., 2022a; Siddiqui et al., 2022b).
Invasive species, including B. tabaci, S. exigua, S. invicta, P. xylostella, etc., have been demonstrated to exhibit extremely high levels of resistance to several kinds of insecticides, and this resistance has been linked to CYP detoxifying enzymes (Lai and Su, 2011; Yu et al., 2015; Chen and Zhou, 2017; Ahmad et al., 2018; Wang et al., 2018b; Xie et al., 2018; Hafeez et al., 2019; 2020a; 2020b; 2022c; Siddiqui et al., 2022b). Increased transcriptional levels of CYP6AE97, CYP321A9, CYP9A105, CYP321A16, and CYP459 in the midgut of S. exigua larvae treated with different insecticides are just some examples of how S. exigua has developed a high level of resistance against several types of insecticides (Wang et al., 2018a; Hu et al., 2019). Moreover, the detoxification mechanism by P450 was reported in various studies. For instance, in S. invicta belonging to the order Hymenoptera, CYP was involved in the detoxification of fluralaner (Xiong et al., 2022) (Xiong et al., 2022), fipronil and beta cypermethrin (Siddiqui et al., 2022b). Similarly, in the order Diptera, D. melanogaster, C. quinquefasciatus and Anopheles funestus (Giles) (Daborn et al., 2002; Wondji et al., 2009; Itokawa et al., 2010), in the order Hemiptera, M. persicae were all reported for the amplification of P450 and insecticide resistance (Puinean et al., 2010). The lambda-cyhalothrin detoxification studies reported the overexpression of P450 in H. zae and H. armigera (order Lepidoptera) (Li et al., 2000a; 2000b; Chen et al., 2017; Hafeez et al., 2020b). Based on these results, it appears that invasive insects rely heavily on the overexpression of P450 genes in order to detoxify xenobiotics from their bodies.
Insects host a diverse microbial population that responds to environmental stresses in a dynamic way (Zhang J. et al., 2022). Similar to the insect, the associated microbiota are shaped by the process of natural selection. Changes in food scarcity and diet chemical exposure can all affect its makeup (Adair and Douglas, 2017; Akami et al., 2022). Host microbiota may help the host metabolise pesticides if the host is subjected to selection pressure from these chemicals. It is possible that this mutation is what makes the host less vulnerable to pesticides (Akami et al., 2019a; 2019b). Bacteria capable of breaking down pesticides have been found in numerous natural environments and in several insect orders, including Coleoptera (Akami et al., 2019b), Hemiptera (Kikuchi et al., 2012), Diptera (Cheng et al., 2017; Hassan et al., 2020), and Lepidoptera (Ramya et al., 2016; Almeida et al., 2017). There has been evidence that resistant strains of bacteria from the gut of P. xylostella (Xia et al., 2018) and S. frugiperda (Almeida et al., 2017) can break down many pesticides (Gomes et al., 2020). Strains of S. frugiperda were chosen because of their ability to degrade pesticides; however, these bacteria were lacking in the microbiota of susceptible, unselected larvae (Almeida et al., 2017) (Figure 2).
Insects have a complex defense system built into their digestive tracts, and this system is most likely the driving force behind the organization of gut microbiota (Siddiqui et al., 2022a). Various processes in this defense system influence the host’s tolerance and resistance to bacteria in the insect gut. Though tolerance refers to the capacity to mitigate the detrimental effects of a particular bacterial burden on the host’s health, resistance refers to the capability to lower the bacterial burden to the point where it is no longer a threat to the host’s vigor (Schneider and Ayres, 2008). Insects harboring more bacteria in their digestive systems are more tolerant to other foreign microbes and have less resistance to them than those with less diverse bacterial communities. Because of this, the systems governing gut immunity in various insects might be personalized to the host’s individual needs. Little is known about the systems that mediate tolerance, despite the fact that resistance mechanisms have been the primary focus of immunology studies (Figure 2) (Engel and Moran, 2013). However, digestive tract microbe-host interactions are frequently mutualistic or commensalism in nature. These may be of special importance to the host in terms of minimizing any harmful effects of the resident microbiota on the host.
The insects have a symbiotic microbiome in their guts that aids detoxification (Jing et al., 2020). The enzymatic detoxification method, used by invading insects and the intestinal microbiota responsible for secreting such digestive enzymes, may be used to establish a viable resistance development strategy (Figure 2). Developing resistance in a collection of organisms at the same time is undoubtedly difficult (Barbosa and Levy, 2000). Mutualistic symbiosis plays a significant role in this process because of its synergy and combined powers; the mutualistic alliance formed by two or more species adjusts to adverse conditions (such as pesticide exposure) more quickly than the individual partners of the mutualistic partnership do (Nobre and Aanen, 2012). Accordingly, any beneficial directional flow of resistance development in the system is mitigated or reversed by this adaptability.
The generation turnover of symbiotic microorganisms may overcome the barrier of invasive ants’ extended generation time, allowing for more favorable mutations or gene regulation/alteration, which could lead to pesticide resistance development. Few studies on invasive ants have provided evidence of the ants’ ability to metabolize xenobiotic compounds such as lignin, plant allele chemicals, and pesticides (Engel and Moran, 2013; Siddiqui et al., 2022b; c). Three partiti-like viruses isolated from the African armyworm (Spodoptera exempta) have been shown to increase resistance to nucleopolyhedrovirus, while the polydnavirus from parasitoid wasps can disrupt the host insect’s immune system to make sure the survivability of wasp progeny (Strand and Burke, 2013; Xu et al., 2020). Lower termites, which feed primarily on wood, require symbiotic flagellates to break down lignocelluloses and methanogenic archaea to produce methane (Ohkuma, 2008; Shi et al., 2015).
Insect microorganisms can modulate insect resistance to synthetic insecticides through direct breakdown and by stimulating the host’s detoxifying enzymes or immune system (Liu and Guo, 2019; Zhao et al., 2022). For example, the 16S rRNA gene sequencing data demonstrated a decrease in the number of the genera Enterococcus and Stenotrophomonas following polymyxin B therapy, which affected the survival rate of Bombyx mori subjected to chlorpyrifos. The host tolerance to chlorpyrifos was improved when germ-free silkworms were given S. maltophilia. This bacteria increases host acetylcholinesterase activity but cannot directly break down chlorpyrifos in the stomach (Du et al., 2020). Aeromonas hydrophila, an intestine bacteria, was found in substantially greater abundance in deltamethrin-resistant individuals of Culex pipiens. After antibiotics were used to clear the stomach of the resistant strains, the resistance level dropped by 66%, and the host’s cytochrome P450 monooxygenase (CYP450) enzyme function dropped by 58%. The resistance and CYP450 enzyme activities were recovered when A. hydrophila was supplied, suggesting that A. hydrophila promotes host resistance to deltamethrin by boosting CYP450 activity (Xing et al., 2021). Further, P. xylostella intestinal Enterococcus sp. Upregulates the appearance of an antimicrobial peptide called gloverin, which contributes to the insect’s resistance to the pesticide chlorpyrifos (Xia et al., 2018). Wolbachia proliferated in N. lugens after treatment with imidacloprid, and their removal decreased CYP450 enzyme activities and NlCYP4CE1 transcript levels. This finding supported the hypothesis that Wolbachia increases host resistance to imidacloprid by increasing the expression of the gene encoding the enzyme responsible for its metabolism, NlCYP4CE1 (Cai et al., 2021). The gut microbiome of pollinators like the honeybee (Apis mellifera) increases tolerance to pesticides like thiacloprid, tau-fluvalinate, and flumethrin by promoting the expression of genes involved in immunity and detoxification (Wu et al., 2020; Yu et al., 2021). When creating novel pest control methods or reducing pests’ vector competence, it is important to keep an eye on the role of the pests’ microbial partners. Bioremediation and the reduction of xenobiotic toxicity may be greatly aided by the discovery of insect-associated microorganisms capable of detoxifying hazardous chemicals (Mahapatro, 2017).
The resistance arises from operational factors, including the increased frequency of pesticide applications, the intensive use of insecticides with increased dosage, decreased yields because of pests, and environmental damages (Georghiou and Taylor, 1986). In addition, these factors include a low economic threshold, the repetition of the same insecticide use across multiple generations, the treatment of a large geographical area, the absence of a place of refuge, the use of long-lasting, slow-release formulations of the same insecticide, and the use of insecticides that are chemically similar to those previously used (Sarwar and Salman, 2015; Subramanyam and Hagstrum, 2018).
Increased global commerce and tourism, climate change, difficulties in protecting borders, Internet commerce, as well as other ways, and invading insects are among global concerns that impose a high economic cost with no apparent remedies in sight (Mooney and Hofgaard, 1999; Lester and Keall, 2005; Venette et al., 2021).
Managing invasive species is challenging because they are often hard to find, and the damage they do may not be noticed for a while. Another challenge is developing new strategies to detect or manage invasive species. Frequently traditional management practices are being used to manage new species, but our ability to control a new invasive species often requires developing novel tools. The last challenge is funding for invasive species control, where there is less support for interdiction activities and extremely high expenditures to launch large-scale actions against established species. Most efforts to control and study invasive species fall into two phases. The initial phases of an invasion (forecast and prevention) give way to later phases (early detection, fast response, mitigation, and management) as the invasion moves forward through the arrival, settlement, and spreading phases (Venette et al., 2021). Cost-benefit evaluations show that focusing on prevention and containment rather than damage control and ecosystem restoration is the best way to deal with invasive species (Leung et al., 2002; Lampert and Liebhold, 2021). Sociologists term the spread of alien invasive species a “wicked” problem because of the complex interplay between its many root causes (such as globalization, climate change, public ignorance, and inadequate biosecurity measures) (McNeely, 2013; Venette and Morey, 2020). Researchers may do their part to control invasive species by focusing on objectives that have widespread support and can be measured quantitatively. The costs and advantages of a proposed solution (such as a new sample plan or control system) should be clearly stated and compared to the status quo. Multiple reasons have led to an increase in the number of invasive species that have migrated over political and natural boundaries, necessitating a multifaceted approach to eradicating them.
The best strategy for combating exotic species is based on prediction and prevention. The goal is straightforward: predict which species or pathways provide unacceptable dangers and prevent them from entering a target area. Such a plan necessitates a defined indicator of success. As the number of alien arthropods keeps rising, every new invasion could be seen as a breach in biosecurity (Finch et al., 2021). However, international trade has expanded faster than new species have been introduced. This pattern implies biosecurity measures have been relatively effective (Venette and Morey, 2020) but are distant from an accurate degree of success (Saccaggi et al., 2016).
To determine whether or not a product can be legally imported, how surveillance programs should be set up, and what actions should be taken after an invasive species has been discovered in a sensitive area, biosecurity professionals will continue to rely on spatially-explicit pest risk assessments (Venette et al., 2010). Locations where an invasive species is most likely to cause damage, are described, emphasizing the environmental factors that must be present for a pest to establish. However, methods for creating these evaluations that are credible, expandable, and cost-effective are required.
Particularly in machine learning and other statistical models, the difficulty in creating models that can be successfully transferred to new space and time is becoming more widely acknowledged as a conceptual barrier (Morey and Venette, 2020). While process-based models appear promising, the sheer volume of information needed to apply them to the hundreds or even thousands of species of importance makes them impractical (Venette, 2017). Improvements in the ability to record shifts in insect population abundance, distribution, and phenology directly result from the democratization of data collecting made possible by the rise of mobile apps and open-access databases. To strengthen predictions, advances in phylogeography will allow for a more thorough accounting of invasions, the identification of invasive phenotypes, and the explicit consideration of genotype x environment relationships (Estoup and Guillemaud, 2010; Roe et al., 2019).
Early detection surveys can be more efficiently planned with the help of pest risk maps, which identify probable invasion or damage regions. Our current capacity for surveying the endangered area may be inadequate (Venette et al., 2010). More study is required, in our opinion, to determine the likelihood of finding low concentrations of invasive arthropods using a given sample strategy (Sandercock et al., 2022). Experts devised a “risk-based monitoring” method that concentrates its sampling efforts in areas with the greatest density of the invasive pest preferred and most economically valuable host plants (Prattley, 2009). As a result, we can lower the expense of detecting invasive species, while additional studies are needed to determine whether or not this method is effective for invading insects. It can be computationally challenging to utilize the strategies pioneered by Yemshanov et al. (2017, Yemshanov et al. 2019) to optimize the spatial distribution of sampling efforts.
When combined with conventional taxonomical knowledge, the recent advancements in genomes provide a method for quick validation of species identification. Additionally, genomic techniques allow for the differentiation of strains, haplotypes, or biotypes within a species (Venette et al., 2021). To better depict the geographic origins of an invading population, specific haplotype data can be used, as shown for the S. frugiperda (Goergen et al., 2016), H. halys (Xu et al., 2014), and walnut twig beetle (Pityophthorus juglandis) (Rugman-Jones et al., 2015). Precise pest origin information allows for early country selection in the search for natural enemies, typically species-specific parasitoids, which may then be released in invaded nations after being evaluated for safety and efficacy (Wyckhuys et al., 2020; Venette et al., 2021).
Following the initial settlement and expansion of invasive species, it is prudent to invest immediately in R&D to promote integrated pest management (IPM) strategies. IPM is “an ecosystem-based strategy that focuses on long-term prevention of pests or their damage through a combination of techniques such as biological control, habitat manipulation, modification of cultural practices, and use of resistant varieties (Sujatha et al., 2022). Pesticides are used only after monitoring indicates they are needed according to established guidelines, and treatments are made to remove only the target organism (Singh et al., 2018). Pest control materials are selected and applied to minimize risks to human health, beneficial and non-target organisms, and the environment” (IPM, 1996). These decision-making guidelines are applied to pest management on a regional scale rather than just on a farm or a piece of land. Ironically, many cropping systems already have IPM in place for indigenous pests. In the case of H. halys in the U.S. apple crop, for example, >$37 million was wasted related to destruction and elevated insecticide application costs because of the advent of the pest (Leskey and Nielsen, 2018). IPM solutions for invasive species often involve short- and long-term plans, just as they do when dealing with endemic pests. Pesticidal treatment is frequently prioritized first since it is effective at maintaining growers’ capital, although agreements to fund research into biocontrol, pest-resistant cultivars, and cultural measures (Radcliffe et al., 2009), physical exclusions (Rogers et al., 2016), and “attract and kill” behavior-based insect traps (Gregg et al., 2018). Developing resistance to invasive pests is becoming increasingly crucial for trees and perennial crops. Innovative technologies, such as transgenic insecticidal plants or genetic biocontrol agents facilitated by gene drives, have the potential to either complement or replace IPM programs, depending on whether or not they are granted the necessary regulatory permissions (Hutchison et al., 2010) (Maselko et al., 2017; Sudweeks et al., 2019).
Insect invasions caused by globalization have posed a severe danger to native plant and animal life, with some species becoming extinct. Because of increased international trade, more seeds and other planting materials are being transported worldwide, increasing the risk of invasive pests being introduced into new habitats and countries.
As a matter of biosecurity, it is recommended that invasive species be identified as soon as possible to control them from invading new regions. However, many underdeveloped nations are particularly lagging in early detection. Without natural predators and parasites, invasive species in their new environment can quickly spread and cause severe damage to economically important plant species and biodiversity.
Strategies to prevent or lessen the impact of future incursions should be part of any future approach to managing invasive species. It is possible to reduce the likelihood of introducing new pest species into an area if people have a general knowledge of invasive species and work together globally by sharing data about these organisms and the predators and parasites that threaten them. The increased science-based knowledge, innovation, and expertise in managing invasive species helped control them more efficiently.
The local flora and fauna, agriculture, horticulture, and the environment severely impact the harmful impacts of invasive pest species. These species negatively impact biodiversity and may also affect the nation’s economy. Scientists must work together across areas in order to detect invasive pests and analyze their ecological problems, environmental risks in different habitats, financial damage, and management alternatives. This can be done by creating generic, particular, and context-dependent action plans. This highlights the significance of the quarantine in preventing the spread of destructive alien pests.
The initial draft was written by JS, RF, HN, and BB. The manuscript was supervised and financially supported by XC and YW. The document was conceptualized and developed by JS, YX and XC. MH, MG and YW provided critical feedback and reviewed the paper. BB, MH and YX revised the manuscript. All authors have read and agreed to the final version of the manuscript.
This study was supported by the National Key Research and Development Program of China (2021YFE0107700), Science and Technology Base and Talent Project of Guangxi Province (Guike AA21196003), Guizhou Provincial Science and Technology Program (2019-1410; 2021-229; HZJD[2022]001), Outstanding Young Scientist Program of Guizhou Province (KY2021-026), Guangxi Key Laboratory of Rice Genetics and Breeding Opening Research Project (2022-36-Z01-KF12), Guizhou University Cultivation Project (2019-04), Program for Introducing Talents to Chinese Universities (111 Program; D20023), and Program for Introducing Talents to Chinese Universities (111 Program; D20023).
The authors declare that the research was conducted in the absence of any commercial or financial relationships that could be construed as a potential conflict of interest.
All claims expressed in this article are solely those of the authors and do not necessarily represent those of their affiliated organizations, or those of the publisher, the editors and the reviewers. Any product that may be evaluated in this article, or claim that may be made by its manufacturer, is not guaranteed or endorsed by the publisher.
Abdulahi G., Obeng-Ofori D., Afreh-Nuamah K., Billah M. K. (2011). Perception of Ghanaian mango farmers on the pest status and current management practices for the control of the African invader fly Bactrocera invadens (Diptera: Tephritidae). N. Y. Sci. J. 4 (2), 74–80.
Acevedo G. R., Zapater M., Toloza A. C. (2009). Insecticide resistance of house fly, Musca domestica (L.) from Argentina. Parasitol. Res. 105, 489–493. doi:10.1007/s00436-009-1425-x
Adair K. L., Douglas A. E. (2017). Making a microbiome: The many determinants of host-associated microbial community composition. Curr. Opin. Microbiol. 35, 23–29. doi:10.1016/j.mib.2016.11.002
Ahmad M., Denholm I., Bromilow R. H. (2006). Delayed cuticular penetration and enhanced metabolism of deltamethrin in pyrethroid-resistant strains of Helicoverpa armigera from China and Pakistan. Pest Manag. Sci. Former. Pestic. Sci. 62, 805–810. doi:10.1002/ps.1225
Ahmad M., Farid A., Saeed M. (2018). Resistance to new insecticides and their synergism in Spodoptera exigua (Lepidoptera: Noctuidae) from Pakistan. Crop Prot. 107, 79–86. doi:10.1016/j.cropro.2017.12.028
Akami M., Andongma A. A., Zhengzhong C., Nan J., Khaeso K., Jurkevitch E., et al. (2019a). Intestinal bacteria modulate the foraging behavior of the oriental fruit fly Bactrocera dorsalis (Diptera: Tephritidae). PLoS One 14, e0210109. doi:10.1371/journal.pone.0210109
Akami M., Njintang N. Y., Gbaye O. A., Andongma A. A., Rashid M. A., Niu C. Y., et al. (2019b). Gut bacteria of the cowpea beetle mediate its resistance to dichlorvos and susceptibility to Lippia adoensis essential oil. Sci. Rep. 9, 6435–6513. doi:10.1038/s41598-019-42843-1
Akami M., Ren X., Wang Y., Mansour A., Cao S., Qi X., et al. (2022). Host fruits shape the changes in the gut microbiota and development of Bactrocera dorsalis (Diptera: Tephritidae) larvae. Int. J. Trop. Insect Sci. 42, 2127–2141. doi:10.1007/s42690-022-00733-6
Al-Kuraishy H. M., Al-Gareeb A. I., Rauf A., Alhumaydhi F. A., Kujawska M., Batiha G. E.-S. (2022). Mechanistic insight and possible mechanism of seizure in covid-19: The nuances and focal points. CNS Neurol. Disord. Targets (Formerly Curr. Drug Targets-CNS Neurol. Disord.
Alavanja M. C. R. (2009). Introduction: Pesticides use and exposure, extensive worldwide. Rev. Environ. Health 24, 303–309. doi:10.1515/reveh.2009.24.4.303
Allen M. L., Rhoades J. H., Sparks M. E., Grodowitz M. J. (2018). Differential gene expression in red imported fire ant (Solenopsis invicta) (Hymenoptera: Formicidae) larval and pupal stages. Insects 9, 185. doi:10.3390/insects9040185
Almeida L. G. de, Moraes L. A. B. de, Trigo J. R. J. R., Omoto C., Consoli F. L., De Almeida L. G., et al. (2017). The gut microbiota of insecticide-resistant insects houses insecticide-degrading bacteria: A potential source for biotechnological exploitation. PLoS One 12, e0174754. doi:10.1371/journal.pone.0174754
Alyokhin A., Sewell G., Choban R. (2008). Reduced viability of Colorado potato beetle, Leptinotarsa decemlineata, eggs exposed to novaluron. Pest Manag. Sci. Former. Pestic. Sci. 64, 94–99. doi:10.1002/ps.1459
Ang G. C. K., Silva R., Maxwell S. L., Zalucki M. P., Furlong M. J. (2014). Contrary effects of leaf position and identity on oviposition and larval feeding patterns of the diamondback moth. Entomol. Exp. Appl. 151, 86–96. doi:10.1111/eea.12172
Balabanidou V., Kampouraki A., MacLean M., Blomquist G. J., Tittiger C., Juárez M. P., et al. (2016). Cytochrome P450 associated with insecticide resistance catalyzes cuticular hydrocarbon production in Anopheles gambiae. Proc. Natl. Acad. Sci. 113, 9268–9273. doi:10.1073/pnas.1608295113
Barbosa T. M., Levy S. B. (2000). The impact of antibiotic use on resistance development and persistence. Drug resist. updat. 3, 303–311. doi:10.1054/drup.2000.0167
Bass C., Carvalho R. A., Oliphant L., Puinean A. M., Field L. M., Nauen R., et al. (2011). Overexpression of a cytochrome P450 monooxygenase, CYP6ER1, is associated with resistance to imidacloprid in the Brown planthopper, Nilaparvata lugens. Insect Mol. Biol. 20, 763–773. doi:10.1111/j.1365-2583.2011.01105.x
Bass C., Field L. M. (2011). Gene amplification and insecticide resistance. Pest Manag. Sci. 67, 886–890. doi:10.1002/ps.2189
Bass C., Jones C. M. (2016). Mosquitoes boost body armor to resist insecticide attack. Proc. Natl. Acad. Sci. 113, 9145–9147. doi:10.1073/pnas.1610992113
Bertelsmeier C., Ollier S., Liebhold A., Keller L. (2017). Recent human history governs global ant invasion dynamics. Nat. Ecol. Evol. 1 (7), 1–8. doi:10.1038/s41559-017-0184
Borah B., Cha-hal B. S., Zhao S., Yu X. X. (1981). Trogoderma granarium in Punjab developed resistance to phosphine. Phytosanitation (03), 36–38. pang zhe pu bang gu ban pi du dui lin hua qing chan sheng le kang yao xing. 旁遮普邦谷斑皮蠹对磷化氢产生了抗药性 zhi wu jian yi 植物检疫.
Bouvier J., Cuany A., Monier C., Brosse V., Sauphanor B. (1998). Enzymatic diagnosis of resistance to deltamethrin in diapausing larvae of the codling moth, Cydia pomonella (L.). Arch. Insect Biochem. Physiol. Publ. Collab. Entomol. Soc. Am. 39, 55–64. doi:10.1002/(sici)1520-6327(1998)39:2<55::aid-arch2>3.0.co;2-1
Broadbent A. B., Pree D. J. (1997). Resistance to insecticides in populations of frankuniella occidentalis (pergande) (thysanoptera: Thripidae) from greenhouses in the niagara region of ontario. Can. Entomol. 129, 907–913. doi:10.4039/ent129907-5
Burman R. J., Rosch R. E., Wilmshurst J. M., Sen A., Ramantani G., Akerman C. J., et al. (2022). Why won’t it stop? The dynamics of benzodiazepine resistance in status epilepticus. Nat. Rev. Neurol. 18, 428–441. doi:10.1038/s41582-022-00664-3
Byrne F. J., Devonshire A. L. (1993). Insensitive acetylcholinesterase and esterase polymorphism in susceptible and resistant populations of the tobacco whitefly Bemisia tabaci (Genn.). Pestic. Biochem. Physiol. 45, 34–42. doi:10.1006/pest.1993.1005
Byrne F. J., Toscano N. C. (2001). An insensitive acetylcholinesterase confers resistance to methomyl in the beet armyworm Spodoptera exigua (Lepidoptera: Noctuidae). J. Econ. Entomol. 94, 524–528. doi:10.1603/0022-0493-94.2.524
Cai T., Zhang Y., Liu Y., Deng X., He S., Li J., et al. (2021). Wolbachia enhances expression of NlCYP4CE1 in Nilaparvata lugens in response to imidacloprid stress. Insect Sci. 28, 355–362. doi:10.1111/1744-7917.12834
Carvalho S. M., Belzunces L. P., Carvalho G. A., Brunet J., Badiou-Beneteau A. (2013). Enzymatic biomarkers as tools to assess environmental quality: A case study of exposure of the honeybee Apis mellifera to insecticides. Environ. Toxicol. Chem. 32, 2117–2124. doi:10.1002/etc.2288
Cens T., Chavanieu A., Bertaud A., Mokrane N., Estaran S., Roussel J., et al. (2022). Molecular targets of neurotoxic insecticides in Apis mellifera. Eur. J. Org. Chem. e202101531, 1–18. doi:10.1002/ejoc.202101531
Chen C., Liu Y., Shi X., Desneux N., Han P., Gao X. (2017). Elevated carboxylesterase activity contributes to the lambda-cyhalothrin insensitivity in quercetin fed Helicoverpa armigera (Hübner). PLoS One 12, e0183111. doi:10.1371/journal.pone.0183111
Chen H. M., Gao J. P., Jjiang J. Y., Peng H., Ma Y. J. (2018). Detection of the I1532 and F1534 kdr mutations and a novel mutant allele I1532T in VGSC gene in the field populations of Aedes albopictus from China. Chin. J. Vector Biol. Control 29 (2), 120.Wo guo bai wen yi wen xian chang qun ti ji dao kang xing ji yin 11532 he F1534 tu bian jian ce ji 11532T tu bian deng wei ji yin bao gao. 我国白纹伊蚊现场群体击倒抗性基因11532 和F1534 突变检测及11532T 突变等位基因报告. zhong guo mei jie sheng wu xue ji kong zhi za zhi.中国媒介生物学及控制杂志. doi:10.11853/j.issn.1003.8280.2018.02.002
Chen L., Meng Q., Li Z., Zhang S., Zeng L., Lu Y. (2015). Surveillance of drug resistance in field populations of Bactrocera dorsalis in Shenzhen area. China Plant Prot. Guide 35 (6), 63. shen zhen di qu ju xiao shi ying tian jian zhong qun kang yao xing jian ce zhong guo zhi bao dao kan 深圳地区桔小实蝇田间种群抗药性监测.中国植保导刊. doi:10.3969/j.issn.1672-6820.2015.06.017
Chen Y., Zhou S. (2017). Phylogenetic relationships based on DNA barcoding among 16 species of the ant genus formica (Hymenoptera: Formicidae) from China. J. Insect Sci. 17, 117. doi:10.1093/jisesa/iex092
Cheng D., Guo Z., Riegler M., Xi Z., Liang G., Xu Y. (2017). Gut symbiont enhances insecticide resistance in a significant pest, the oriental fruit fly Bactrocera dorsalis (Hendel). Microbiome 5, 13–12. doi:10.1186/s40168-017-0236-z
Clark A. G. (1989). The comparative enzymology of the glutathione S-transferases from non-vertebrate organisms. Comp. Biochem. Physiol. Part B Comp. Biochem. 92, 419–446. doi:10.1016/0305-0491(89)90114-4
Connor D. J., Loomis R. S., Cassman K. G. (2011). Crop ecology: Productivity and management in agricultural systems. New York: Cambridge University Press.
Culshaw-Maurer M., Sih A., Rosenheim J. A. (2020). Bugs scaring bugs: Enemy-risk effects in biological control systems. Ecol. Lett. 23, 1693–1714. doi:10.1111/ele.13601
Cutright C. R. (1954). A codling moth population resistant to DDT. J. Econ. Entomol. 47, 189–190. doi:10.1093/jee/47.1.189
da Silva Nunes G., Truzi C. C., Cardoso C. P., Vieira N. F., Ramalho D. G., de Souza J. M., et al. (2020). Temperature-dependent functional response of Euborellia annulipes (Dermaptera: Anisolabididae) preying on Plutella xylostella (Lepidoptera: Plutellidae) larvae. J. Therm. Biol. 93, 102686. doi:10.1016/j.jtherbio.2020.102686
Daborn P. J., Yen J. L., Bogwitz M. R., Le Goff G., Feil E., Jeffers S., et al. (2002). A single P450 allele associated with insecticide resistance in Drosophila. Science 80 (297), 2253–2256. doi:10.1126/science.1074170
Dasari S., Ganjayi M. S., Yellanurkonda P., Basha S., Meriga B. (2018). Role of glutathione S-transferases in detoxification of a polycyclic aromatic hydrocarbon, methylcholanthrene. Chem. Biol. Interact. 294, 81–90. doi:10.1016/j.cbi.2018.08.023
Davies T. G. E., Field L. M., Usherwood P. N. R., Williamson M. S. (2007). DDT, pyrethrins, pyrethroids and insect sodium channels. IUBMB Life 59, 151–162. doi:10.1080/15216540701352042
de Jonge N., Michaelsen T. Y., Ejbye-Ernst R., Jensen A., Nielsen M. E., Bahrndorff S., et al. (2020). Housefly (Musca domestica L.) associated microbiota across different life stages. Sci. Rep. 10, 7842–7849. doi:10.1038/s41598-020-64704-y
De Roode J. C., Lefèvre T. (2012). Behavioral immunity in insects. Insects 3, 789–820. doi:10.3390/insects3030789
Devrnja N., Milutinović M., Savić J. (2022). When scent becomes a weapon—plant essential oils as potent bioinsecticides. Sustainability 14, 6847. doi:10.3390/su14116847
Dhami M. K., Booth K. (2008). “Review of dispersal distances and landing site behaviour of Solenopsis invicta buren, red imported fire ant (RIFA),” in Cent. Biodivers. Biosecurity (Auckland, New Zeal: Sch. Biol. Sci. Univ. Auckland).
Drees B. M., Calixto A. A., Nester P. R. (2013). Integrated pest management concepts for red imported fire ants Solenopsis invicta (Hymenoptera: Formicidae). Insect Sci. 20, 429–438. doi:10.1111/j.1744-7917.2012.01552.x
Du Y., Grodowitz M. J., Chen J. (2020). Insecticidal and enzyme inhibitory activities of isothiocyanates against red imported fire ants, solenopsis invicta. Biomolecules 10, 716. doi:10.3390/biom10050716
Dunlop E. S., McLaughlin R., Adams J. V., Jones M., Birceanu O., Christie M. R., et al. (2018). Rapid evolution meets invasive species control: The potential for pesticide resistance in sea lamprey. Can. J. Fish. Aquat. Sci. 75, 152–168. doi:10.1139/cjfas-2017-0015
Engel P., Moran N. A. (2013). The gut microbiota of insects - diversity in structure and function. FEMS Microbiol. Rev. 37, 699–735. doi:10.1111/1574-6976.12025
Espinosa P. J., Bielza P., Contreras J., Lacasa A. (2002). Insecticide resistance in field populations of Frankliniella occidentalis (Pergande) in Murcia (south-east Spain). Pest Manag. Sci. 58, 967–971. doi:10.1002/ps.572
Estoup A., Guillemaud T. (2010). Reconstructing routes of invasion using genetic data: Why, how and so what? Mol. Ecol. 19, 4113–4130. doi:10.1111/j.1365-294X.2010.04773.x
Falcon T., Pinheiro D. G., Ferreira-Caliman M. J., Turatti I. C. C., Abreu F. C. P. de, Galaschi-Teixeira J. S., et al. (2019). Exploring integument transcriptomes, cuticle ultrastructure, and cuticular hydrocarbons profiles in eusocial and solitary bee species displaying heterochronic adult cuticle maturation. PLoS One 14, e0213796. doi:10.1371/journal.pone.0213796
Fang F., Wang W., Zhang D., Lv Y., Zhou D., Ma L., et al. (2015). The cuticle proteins: A putative role for deltamethrin resistance in Culex pipiens pallens. Parasitol. Res. 114, 4421–4429. doi:10.1007/s00436-015-4683-9
FAOSTAT Food and Agriculture Organization of the United Nations (2020). Pesticides use. Retrieved from: http://www.fao.org/faostat/en/#data/RP, visited (Accessed on November 22, 2020).
Fardisi M., Gondhalekar A. D., Ashbrook A. R., Scharf M. E. (2019). Rapid evolutionary responses to insecticide resistance management interventions by the German cockroach (Blattella germanica L.). Sci. Rep. 9 (1), 1–10. doi:10.1038/s41598-019-44296-y
Feng Y. T., Wu Q. J., Xu B. Y., Wang S. L., Chang X. L., Xie W., et al. (2009). Fitness costs and morphological change of laboratory-selected thiamethoxam resistance in the B-type Bemisia tabaci (Hemiptera: Aleyrodidae). J. Appl. Entomol. 133, 466–472. doi:10.1111/j.1439-0418.2009.01383.x
Fenibo E. O., Ijoma G. N., Nurmahomed W., Matambo T. (2022). The potential and green chemistry attributes of biopesticides for sustainable agriculture. Sustainability 14, 14417. doi:10.3390/su142114417
Fessl B., Tebbich S. (2002). Philornis downsi–a recently discovered parasite on the Galápagos archipelago–a threat for Darwin’s finches? Ibis Lond. 1859) 144, 445–451. doi:10.1046/j.1474-919x.2002.00076.x
Finch D. M., Butler J. L., Runyon J. B., Fettig C. J., Kilkenny F. F., Jose S., et al. (2021). Effects of climate change on invasive species.
Gan S. J., Leong Y. Q., bin Barhanuddin M. F. H., Wong S. T., Wong S. F., Mak J. W., et al. (2021). Dengue fever and insecticide resistance in Aedes mosquitoes in southeast asia: A review. Parasit. Vectors 14, 315–319. doi:10.1186/s13071-021-04785-4
Gentz M. C., Grace J. K. (2006). A review of boron toxicity in insects with an emphasis on termites. J. Agric. Urban Entomol. 23 (4), 201–207.
Georghiou G. P., Taylor C. E. (1986). Factors influencing the evolution of resistance. Pestic. Resist. Strateg. tactics Manag. 157–619. Washington, DC: National Academies Press.
Giraldo Y. M., Muscedere M. L., Traniello J. F. A. (2021). Eusociality and senescence: Neuroprotection and physiological resilience to aging in insect and mammalian systems. Front. Cell. Dev. Biol. 9, 673172. doi:10.3389/fcell.2021.673172
Goergen G., Kumar P. L., Sankung S. B., Togola A., Tamò M. (2016). First report of outbreaks of the fall armyworm Spodoptera frugiperda (JE Smith) (Lepidoptera, Noctuidae), a new alien invasive pest in West and Central Africa. PLoS One 11, e0165632. doi:10.1371/journal.pone.0165632
Gomes A. F. F., Omoto C., Cônsoli F. L. (2020). Gut bacteria of field-collected larvae of Spodoptera frugiperda undergo selection and are more diverse and active in metabolizing multiple insecticides than laboratory-selected resistant strains. J. Pest Sci. 93, 833–851. doi:10.1007/s10340-020-01202-0
Gong Y., Li M., Li T., Liu N. (2022). Molecular and functional characterization of three novel carboxylesterases in the detoxification of permethrin in the mosquito, Culex quinquefasciatus. Insect Sci. 29, 199–214. doi:10.1111/1744-7917.12927
Götte E., Rybak M. (2011). Pest control of the Western flower thrips Frankliniella occidentalis (Pergande) with proven insecticide resistance in cut flowers in greenhouses. Gesunde Pflanz. 62, 117–123. doi:10.1007/s10343-010-0229-3
Grafton-Cardwell E. E., Ouyang Y., Striggow R. A., Christiansen J. A., Black C. S. (2004). Role of esterase enzymes in monitoring for resistance of California red scale, Aonidiella aurantii (Homoptera: Diaspididae), to organophosphate and carbamate insecticides. J. Econ. Entomol. 97, 606–613. doi:10.1093/jee/97.2.606
Gregg P. C., Del Socorro A. P., Landolt P. J. (2018). Advances in attract-and-kill for agricultural pests: Beyond pheromones. Annu. Rev. Entomol. 63, 453–470. doi:10.1146/annurev-ento-031616-035040
Gu S. W., Ceng L., Liang G. W. (2015). Monitoring for insecticide resistance of melon fly populations in the field of Southern China. J. South China Agric. Univ. 36 (04), 76–80. Hua nan di qu gua shi ying tian jian zhong qun de kang yao xing jian ce. 华南地区瓜实蝇田间种群的抗药性监测 hua nan nong ye da xue xue bao. 华南农业大学学报. doi:10.7671/j.issn.1001-411X.2015.04.014
Guillemaud T., Brun A., Anthony N., Sauge M. H., Boll R., et al. (2003). Incidence of insecticide resistance alleles in sexually-reproducing populations of the peach-potato aphid Myzus persicae (Hemiptera: Aphididae) from southern France. Bull. Entomol. Res. 93, 289–297. doi:10.1079/ber2003241
Gunning R. V., Easton C. S., Balfe M. E., Ferris I. G. (1991). Pyrethroid resistance mechanisms in Australian Helicoverpa armigera. Pestic. Sci. 33, 473–490. doi:10.1002/ps.2780330410
Guo Y., Zhang J., Yu R., Zhu K. Y., Guo Y., Ma E. (2012). Identification of two new cytochrome P450 genes and RNA interference to evaluate their roles in detoxification of commonly used insecticides in Locusta migratoria. Chemosphere 87, 709–717. doi:10.1016/j.chemosphere.2011.12.061
Gutiérrez-Moreno R., Mota-Sanchez D., Blanco C. A., Whalon M. E., Terán-Santofimio H., Rodriguez-Maciel J. C., et al. (2019). Field-evolved resistance of the fall armyworm (Lepidoptera: Noctuidae) to synthetic insecticides in Puerto Rico and Mexico. J. Econ. Entomol. 112, 792–802. doi:10.1093/jee/toy372
Hafeez M., Jan S., Nawaz M., Ali E., Ali B., Qasim M., et al. (2019). Sub-lethal effects of lufenuron exposure on spotted bollworm earias vittella (fab): Key biological traits and detoxification enzymes activity. Environ. Sci. Pollut. Res. 26, 14300–14312. doi:10.1007/s11356-019-04655-8
Hafeez M., Li X., Ullah F., Zhang Z., Zhang J., Huang J., et al. (2022a). Down-regulation of P450 genes enhances susceptibility to indoxacarb and alters physiology and development of fall armyworm, Spodoptera frugipreda (Lepidoptera: Noctuidae). Front. Physiol. 13, 884447. doi:10.3389/fphys.2022.884447
Hafeez M., Li X., Zhang Z., Huang J., Wang L., Zhang J., et al. (2021). De novo transcriptomic analyses revealed some detoxification genes and related pathways responsive to noposion Yihaogong® 5% EC (Lambda-Cyhalothrin 5%) exposure in Spodoptera frugiperda third-instar larvae. Insects 12, 132–216. doi:10.3390/insects12020132
Hafeez M., Liu S., Yousaf H. K., Jan S., Wang R. L., Fernández-Grandon G. M., et al. (2020a). RNA interference-mediated knockdown of a cytochrome P450 gene enhanced the toxicity of α-cypermethrin in xanthotoxin-fed larvae of Spodoptera exigua (Hübner). Pestic. Biochem. Physiol. 162, 6–14. doi:10.1016/j.pestbp.2019.07.003
Hafeez M., Qasim M., Ali S., Yousaf H. K., Waqas M., Ali E., et al. (2020b). Expression and functional analysis of P450 gene induced tolerance/resistance to lambda-cyhalothrin in quercetin fed larvae of beet armyworm Spodoptera exigua (Hübner). Saudi J. Biol. Sci. 27, 77–87. doi:10.1016/j.sjbs.2019.05.005
Hafeez M., Ullah F., Khan M. M., Li X., Zhang Z., Shah S., et al. (2022b). Metabolic-based insecticide resistance mechanism and ecofriendly approaches for controlling of beet armyworm Spodoptera exigua: A review. Environ. Sci. Pollut. Res. 29, 1746–1762. doi:10.1007/s11356-021-16974-w
Hafeez M., Ullah F., Khan M. M., Wang Z., Gul H., Li X., et al. (2022c). Comparative low lethal effects of three insecticides on demographical traits and enzyme activity of the Spodoptera exigua (Hübner). Environ. Sci. Pollut. Res. 29, 60198–60211. doi:10.1007/s11356-022-20182-5
Hardy M. C. (2014). Resistance is not futile: It shapes insecticide discovery. Insects 5, 227–242. doi:10.3390/insects5010227
Harris C. R., Svec H. J. (1981). Colorado potato beetle resistance to carbofuran and several other insecticides in Quebec. J. Econ. Entomol. 74, 421–424. doi:10.1093/jee/74.4.421
Hassan B., Siddiqui J. A., Xu Y. (2020). Vertically transmitted gut bacteria and nutrition influence the immunity and fitness of bactrocera dorsalis larvae. Front. Microbiol. 11, 596352–596414. doi:10.3389/fmicb.2020.596352
Hassan F., Singh K. P., Ali V., Behera S., Shivam P., Das P., et al. (2019). Detection and functional characterization of sigma class GST in Phlebotomus argentipes and its role in stress tolerance and DDT resistance. Sci. Rep. 9, 19636–19715. doi:10.1038/s41598-019-56209-0
Helps J. C., Paveley N. D., van den Bosch F. (2017). Identifying circumstances under which high insecticide dose increases or decreases resistance selection. J. Theor. Biol. 428, 153–167. doi:10.1016/j.jtbi.2017.06.007
Herron G. A., James T. M. (2005). Monitoring insecticide resistance in Australian Frankliniella occidentalis Pergande (Thysanoptera: Thripidae) detects fipronil and spinosad resistance. Aust. J. Entomol. 44, 299–303. doi:10.1111/j.1440-6055.2005.00478.x
Hilliou F., Chertemps T., Maïbèche M., Le Goff G. (2021). Resistance in the genus Spodoptera: Key insect detoxification genes. Insects 12, 544. doi:10.3390/insects12060544
Hollman A. L., Tchounwou P. B., Huang H.-C. (2016). The association between gene-environment interactions and diseases involving the human GST superfamily with SNP variants. Int. J. Environ. Res. Public Health 13, 379. doi:10.3390/ijerph13040379
Holt R. D. (2009). Bringing the hutchinsonian niche into the 21st century: Ecological and evolutionary perspectives. Proc. Natl. Acad. Sci. 106, 19659–19665. doi:10.1073/pnas.0905137106
Hou J., Liu Q., Wang J., Wu Y., Li T., Gong Z. (2020). Insecticide resistance of Aedes albopictus in zhejiang Province, China. Biosci. Trends 14, 248–254. doi:10.5582/bst.2020.03194
Hough W. S. (1928). Relative resistance to arsenical poisoning of two codling moth strains. J. Econ. Entomol. 21, 325–329. doi:10.1093/jee/21.2.325
Hsu J.-C., Feng H.-T., Wu W.-J. (2004). Resistance and synergistic effects of insecticides in Bactrocera dorsalis (Diptera: Tephritidae) in Taiwan. J. Econ. Entomol. 97, 1682–1688. doi:10.1603/0022-0493-97.5.1682
Hu B., Zhang S., Ren M., Tian X., Wei Q., Mburu D. K., et al. (2019). The expression of Spodoptera exigua P450 and UGT genes: Tissue specificity and response to insecticides. Insect Sci. 26, 199–216. doi:10.1111/1744-7917.12538
Hutchison W. D., Burkness E. C., Mitchell P. D., Moon R. D., Leslie T. W., Fleischer S. J., et al. (2010). Areawide suppression of European corn borer with Bt maize reaps savings to non-Bt maize growers. Science 330 (80), 222–225. doi:10.1126/science.1190242
Immaraju J. A., Paine T. D., Bethke J. A., Robb K. L., Newman J. P. (1992). Western flower thrips (Thysanoptera: Thripidae) resistance to insecticides in coastal California greenhouses. J. Econ. Entomol. 85, 9–14. doi:10.1093/jee/85.1.9
Inward D. J. G., Wainhouse D., Peace A. (2012). The effect of temperature on the development and life cycle regulation of the pine weevil Hylobius abietis and the potential impacts of climate change. Agric. For. Entomol. 14, 348–357. doi:10.1111/j.1461-9563.2012.00575.x
Ipm U. C. (1996). What is integrated pest management (IPM). Davis, CA: Univ California Coll Agric Nat Resour.
IRAC (2022). Insecticide resistance action committee. IRAC Available at: https://irac-online.org/(Accessed November 28, 2022).
Itokawa K., Komagata O., Kasai S., Okamura Y., Masada M., Tomita T. (2010). Genomic structures of Cyp9m10 in pyrethroid resistant and susceptible strains of Culex quinquefasciatus. Insect biochem. Mol. Biol. 40, 631–640. doi:10.1016/j.ibmb.2010.06.001
Jaffar S., Ahmad S., Lu Y. (2022). Contribution of insect gut microbiota and their associated enzymes in insect physiology and biodegradation of pesticides. Front. Microbiol. 13, 979383. doi:10.3389/fmicb.2022.979383
Jensen S. E. (2000). Insecticide resistance in the Western flower thrips, Frankliniella occidentalis. Integr. Pest Manag. Rev. 5, 131–146.
Jin Y., Gao Y., Zhang H., Wang L., Yang K., Dong H. (2020). Detoxification enzymes associated with butene-fipronil resistance in Epacromius coerulipes. Pest Manag. Sci. 76, 227–235. doi:10.1002/ps.5500
Jing T.-Z., Qi F.-H., Wang Z.-Y. (2020). Most dominant roles of insect gut bacteria: Digestion, detoxification, or essential nutrient provision? Microbiome 8, 38–20. doi:10.1186/s40168-020-00823-y
Jing Z. H. U., Chen’ge L. I., Ya’nan S., Meng Z., Jinhua C., Jiawei Z. H. U., et al. (2016). Insecticide resistance status of woolly apple aphid, Eriosoma lanigerum in China. 农药学学报 18, 447.
Johnson R. M., Mao W., Pollock H. S., Niu G., Schuler M. A., Berenbaum M. R. (2012). Ecologically appropriate xenobiotics induce cytochrome P450s in Apis mellifera. PLoS One 7, e31051. doi:10.1371/journal.pone.0031051
Jones B. M., Robinson G. E. (2018). Genetic accommodation and the role of ancestral plasticity in the evolution of insect eusociality. J. Exp. Biol. 221, jeb153163. doi:10.1242/jeb.153163
Kaleem Ullah R. M., Gökçe A., Bakhsh A., Salim M., Wu H. Y., Naqqash M. N. (2022). Insights into the use of eco-friendly synergists in resistance management of Leptinotarsa decemlineata (Coleoptera: Chrysomelidae). Insects 13, 846. doi:10.3390/insects13090846
Karaagac S. U. (2012). „Insecticide resistance,” in Insecticides-advances in integrated pest management. IntechOpen. doi:10.5772/28086
Karunamoorthi K., Sabesan S. (2013). Insecticide resistance in insect vectors of disease with special reference to mosquitoes: A potential threat to global public health. Heal. Scope 2, 4–18. doi:10.17795/jhealthscope-9840
Karunaratne S., Surendran S. N. (2022). Mosquito control: A review on the past, present and future strategies. J. Natl. Sci. Found. Sri Lanka 50, 277. doi:10.4038/jnsfsr.v50i0.11244
Khan H. A. A., Akram W. (2018). Trichlorfon and spinosad resistance survey and preliminary determination of the resistance mechanism in Pakistani field strains of Bactrocera dorsalis. Sci. Rep. 8, 11223–11225. doi:10.1038/s41598-018-29622-0
Khan M. M., Hafeez M., Elgizawy K., Wang H., Zhao J., Cai W., et al. (2021a). Sublethal effects of chlorantraniliprole on paederus fuscipes (staphylinidae: Coleoptera), a general predator in paddle field. Environ. Pollut. 291, 118171. doi:10.1016/j.envpol.2021.118171
Khan M. M., Hafeez M., Siddiqui J. A., Ullah F., Shah S., Iftikhar A., et al. (2021b). Residual toxicity and sublethal effects of fenvalerate on the development and physiology of Spodoptera exigua reared on different hosts. J. King Saud. Univ. - Sci. 33, 101593. doi:10.1016/j.jksus.2021.101593
Khan M. M., Kaleem-ullah R. M., Siddiqui J. A., Ali S. (2020). Insecticide resistance and detoxification enzymes activity in nilaparvata lugens stål against neonicotinoids. J. Agric. Sci. 12, 24–36. doi:10.5539/jas.v12n5p24
Khan M. M., Khan A. H., Ali M. W., Hafeez M., Ali S., Du C., et al. (2021c). Emamectin benzoate induced enzymatic and transcriptional alternation in detoxification mechanism of predatory beetle Paederus fuscipes (Coleoptera: Staphylinidae) at the sublethal concentration. Ecotoxicology 30, 1227–1241. doi:10.1007/s10646-021-02426-1
Kikuchi Y., Hayatsu M., Hosokawa T., Nagayama A., Tago K., Fukatsu T. (2012). Symbiont-mediated insecticide resistance. Proc. Natl. Acad. Sci. U. S. A. 109, 8618–8622. doi:10.1073/pnas.1200231109
Kim I., Choi B., Park W., Kim Y., Kim B., Mun S., et al. (2022). Nuclear receptor HR96 up-regulates cytochrome P450 for insecticide detoxification in Tribolium castaneum. Pest Manag. Sci. 78, 230–239. doi:10.1002/ps.6626
Kliot A., Ghanim M. (2012). Fitness costs associated with insecticide resistance. Pest. Manag. Sci. 68, 1431–1437. doi:10.1002/ps.3395
Konrad M., Pull C. D., Metzler S., Seif K., Naderlinger E., Grasse A. V., et al. (2018). Ants avoid superinfections by performing risk-adjusted sanitary care. Proc. Natl. Acad. Sci. 115 (11), 2782–2787.
Koop J. A. H., Causton C. E., Bulgarella M., Cooper E., Heimpel G. E. (2021). Population structure of a nest parasite of Darwin’s finches within its native and invasive ranges. Conserv. Genet. 22, 11–22. doi:10.1007/s10592-020-01315-0
Kranthi K. R., Jadhav D. R., Kranthi S., Wanjari R. R., Ali S. S., Russell D. A. (2002). Insecticide resistance in five major insect pests of cotton in India. Crop Prot. 21, 449–460. doi:10.1016/s0261-2194(01)00131-4
Lai T., Su J. (2011). Assessment of resistance risk in Spodoptera exigua (Hübner)(Lepidoptera: Noctuidae) to chlorantraniliprole. Pest Manag. Sci. 67, 1468–1472. doi:10.1002/ps.2201
Lampert A., Liebhold A. M. (2021). Combining multiple tactics over time for cost-effective eradication of invading insect populations. Ecol. Lett. 24, 279–287. doi:10.1111/ele.13640
Lavergne S., Molofsky J. (2007). Increased genetic variation and evolutionary potential drive the success of an invasive grass. Proc. Natl. Acad. Sci. 104, 3883–3888. doi:10.1073/pnas.0607324104
Leskey T. C., Nielsen A. L. (2018). Impact of the invasive Brown marmorated stink bug in North America and Europe: History, biology, ecology, and management. Annu. Rev. Entomol. 63, 599–618. doi:10.1146/annurev-ento-020117-043226
Lester P. J., Keall J. B. (2005). The apparent establishment and subsequent eradication of the Australian giant bulldog ant Myrmecia brevinoda Forel (Hymenoptera: Formicidae) in New Zealand. New zeal. J. Zool. 32, 353–357. doi:10.1080/03014223.2005.9518423
Leung B., Lodge D. M., Finnoff D., Shogren J. F., Lewis M. A., Lamberti G. (2002). An ounce of prevention or a pound of cure: Bioeconomic risk analysis of invasive species. Proc. R. Soc. Lond. Ser. B Biol. Sci. 269, 2407–2413. doi:10.1098/rspb.2002.2179
Li X., Berenbaum M. R., Schuler M. A. (2000a). Molecular cloning and expression of CYP6B8: A xanthotoxin-inducible cytochrome P450 cDNA from helicoverpa zea. Insect biochem. Mol. Biol. 30, 75–84. doi:10.1016/s0965-1748(99)00102-2
Li X. C., Wang Y. Z., Han Z. J., Wang M., Ding S. Y., Dong X. H., et al. (1995). Monitoring Pectinophora gossypiella's resistance to insecticides with the incubation larvae. J. Plant Prot. 22 (1), 85–90. yong chu fu you chong jian ce mian hong ling chong dui sha chong ji de kang xing 用初孵幼虫监测棉红铃虫对杀虫剂的抗性 zhi wu bao hu xue bao 植物保护学报.
Li X., Zangerl A. R., Schuler M. A., Berenbaum M. R. (2000b). Cross-resistance to α-cypermethrin after xanthotoxin ingestion in Helicoverpa zea (Lepidoptera: Noctuidae). J. Econ. Entomol. 93, 18–25. doi:10.1603/0022-0493-93.1.18
Liang X., Xiao D., He Y., Yao J., Zhu G., Zhu K. Y. (2015). Insecticide-mediated up-regulation of cytochrome P450 genes in the red flour beetle (Tribolium castaneum). Int. J. Mol. Sci. 16, 2078–2098. doi:10.3390/ijms16012078
Liu D., Lv X. Y., Li J. S., Dong B. Y. (2005). Causes of the Trialeurodes vaporariorum outbreak in southern Liaoning and integrated control techniques. Liao ning nong ye ke xue. Liaoning Agric. Sci. 0 (03), 12–17. liao nan di qu wen shi bai fen shi da fa sheng de yuan yin ji zong he fang zhi ji shu. 辽南地区温室白粉虱大发生的原因及综合防治技术. 辽宁农业科学.
Liu N. (2015). Insecticide resistance in mosquitoes: Impact, mechanisms, and research directions. Annu. Rev. Entomol. 60, 537–559. doi:10.1146/annurev-ento-010814-020828
Liu P., Jiang W. H., Lu W. P., Li G. Q. (2011). Susceptibility of Colorado potato beetle Leptinotarsa decemlineata adults from northern Xinjiang Uygur autonomous region to 4 neonicotinoids. nong yao xue xue bao. J. Pesticide 13 (3), 271. xin jiang bei jiang ma ling shu jia chong cheng chong dui xin yan jian lei sha chong ji de min gan xing bian hua. 新疆北疆马铃薯甲虫成虫对新烟碱类杀虫剂的敏感性变化. 农药学学报.
Liu X.-D., Guo H.-F. (2019). Importance of endosymbionts Wolbachia and Rickettsia in insect resistance development. Curr. Opin. insect Sci. 33, 84–90. doi:10.1016/j.cois.2019.05.003
Liu X., Wang X., Ding X., Fu K., Tursun A., He J., et al. (2018). Resistance levels of main common chemical insecticides on different geographical populations of Lissorhoptrus oryzophilus in ecological regions in xinjiang. Xin jiang nong ye ke xue. Xinjiang Agric. Sci. 55 (10), 1847–1853. Xin jiang zhu yao dao qu bu tong dao shui xiang jia di li zhong qun dui chang yong sha chong ji kang yao xing ce ding 新疆主要稻区不同稻水象甲地理种群对常用杀虫剂抗药性测定 .新疆农业科学.
Lopez-Suarez L., Al Awabdh S., Coumoul X., Chauvet C. (2022). The SH-SY5Y human neuroblastoma cell line, a relevant in vitro cell model for investigating neurotoxicology in human: Focus on organic pollutants. Neurotoxicology 92, 131–155. doi:10.1016/j.neuro.2022.07.008
Lorke D. E., Petroianu G. A. (2019). Reversible cholinesterase inhibitors as pretreatment for exposure to organophosphates. A review. J. Appl. Toxicol. 39, 101–116. doi:10.1002/jat.3662
Luo Y.-S., Abdellah Y. A. Y., Hafeez M., Yang X., Hou W.-T., Kong X.-H., et al. (2022). Herbivore-induced tomato plant volatiles lead to the reduction of insecticides susceptibility in Spodoptera litura. Pestic. Biochem. Physiol. 187, 105215. doi:10.1016/j.pestbp.2022.105215
Lushchak V. I., Matviishyn T. M., Husak V. V., Storey J. M., Storey K. B. (2018). Pesticide toxicity: A mechanistic approach. EXCLI J. 17, 1101–1136. doi:10.17179/excli2018-1710
Malik A., Singh N., Satya S. (2007). House fly (Musca domestica): A review of control strategies for a challenging pest. J. Environ. Sci. Heal. part B 42, 453–469. doi:10.1080/03601230701316481
Marbuah G., Gren I. M., McKie B. (2014). Economics of harmful invasive species: A review. Diversity 6, 500–523. doi:10.3390/d6030500
Mariath H. A., Orton C. J., Shivas C. J. (1990). Resistance to oviposition suppression in Lucilia cuprina. Resist. Manag. Parasites Sheep, 52.
Martin N. A., Workman P. J. (1994). “Confirmation of a pesticide-resistant strain of Western flower thrips in New Zealand,” in Proceedings of the Forty Seventh New Zealand Plant Protection Conference (New Zealand: Waitangi HotelNew Zealand Plant Protection Society), 144–148.
Maselko M., Heinsch S. C., Chacón J. M., Harcombe W. R., Smanski M. J. (2017). Engineering species-like barriers to sexual reproduction. Nat. Commun. 8, 883–887. doi:10.1038/s41467-017-01007-3
McNeely J. A. (2013). “Global efforts to address the wicked problem of invasive alien species,” in Plant Invasions in Protected Areas. Invading Nature - Springer Series in Invasion Ecology, Vol. 7. Editors L. Foxcroft, P. Pyšek, D. Richardson, and P. Genovesi (Springer: Dordrecht). doi:10.1007/978-94-007-7750-7_4
Memarizadeh N., Ghadamyari M., Sajedi R. H., Jalali Sendi J. (2011). Characterization of esterases from abamectin-resistant and susceptible strains of Tetranychus urticae Koch (Acari: Tetranychidae). Int. J. Acarol. 37, 271–281. doi:10.1080/01647954.2010.517564
Mendoza A. C. (2016). Evaluation of impact of long-lasting insecticidal house screening (LLIS) on pyrethroid resistant population of the dengue vector Aedes aegypti in Mexico. PhD thesis. Liverpool, United Kingdom: The University of Liverpool.
Milano M., Chèvre N. (2019). How water quality standards for pesticides affect the classification of the chemical status of rivers, 6. Wiley Interdiscip. Rev. Water, e1375.
Moffit H. R., Westigard P. H., Mantey K. D., Van de Baan H. E. (1988). Resistance to diflubenzuron in the codling moth (Lepidoptera: Tortricidae). J. Econ. Entomol. 81, 1511–1515. doi:10.1093/jee/81.6.1511
Mooney H. A., Hofgaard A. (1999). “Biological invasions and global change,” in Invasive species and biodiversity management. Based on a selection of papers presented at the Norway/UN conference on alien species Trondheim, Norway.
Morey A. C., Venette R. C. (2020). Minimizing risk and maximizing spatial transferability: Challenges in constructing a useful model of potential suitability for an invasive insect. Ann. Entomol. Soc. Am. 113, 100–113. doi:10.1093/aesa/saz049
Morrison L. W., Porter S. D., Daniels E., Korzukhin M. D. (2004). Potential global range expansion of the invasive fire ant, Solenopsis invicta. Solenopsis invicta. Biol. Invasions 6, 183–191. doi:10.1023/b:binv.0000022135.96042.90
Mubashir S., Seram D. (2022). Insecticidal resistance in diamondback moth (Plutella xylostella): A review. Pharma Innov. J. 11 (7S), 958–962.
Muthusamy M., Shivakumar S., Karthi K., Ramkumar R. (2011). Pesticide detoxifying mechanism in field population of Spodoptera litura (Lepidoptera: Noctuidae) from south India. Egypt. Acad. J. Biol. Sci. F. Toxicol. Pest Control 3, 51–57. doi:10.21608/eajbsf.2011.17437
Nansen C., Baissac O., Nansen M., Powis K., Baker G. (2016). Behavioral avoidance - will physiological insecticide resistance level of insect strains affect their oviposition and movement responses? PLoS One 11 (3), e0149994–e0150012. doi:10.1371/journal.pone.0149994
Naqqash M. N., Gökçe A., Bakhsh A., Salim M. (2016). Insecticide resistance and its molecular basis in urban insect pests. Parasitol. Res. 115, 1363–1373. doi:10.1007/s00436-015-4898-9
Nasirian H. (2010). An overview of German cockroach, Blattella germanica, studies conducted in Iran. Pak. J. Biol. Sci. 13, 1077–1084. doi:10.3923/pjbs.2010.1077.1084
Nelson D. R., Goldstone J. V., Stegeman J. J. (2013). The cytochrome P450 Genesis locus: The origin and evolution of animal cytochrome P450s. Philos. Trans. R. Soc. B Biol. Sci. 368, 20120474. doi:10.1098/rstb.2012.0474
Nelson D. R. (2011). Progress in tracing the evolutionary paths of cytochrome P450. Biochim. Biophys. Acta (BBA)-Proteins Proteomics 1814, 14–18. doi:10.1016/j.bbapap.2010.08.008
Ngumbi E. N., Hanks L. M., Suarez A. V., Millar J. G., Berenbaum M. R. (2020). Factors associated with variation in cuticular hydrocarbon profiles in the navel orangeworm, Amyelois transitella (Lepidoptera: Pyralidae). J. Chem. Ecol. 46, 40–47. doi:10.1007/s10886-019-01129-6
Nobre T., Aanen D. K. (2012). Fungiculture or termite husbandry? The ruminant hypothesis. Insects 3, 307–323. doi:10.3390/insects3010307
Occhibove F., Chapman D. S., Mastin A. J., Parnell S. S. R., Agstner B., Mato-Amboage R., et al. (2020). Eco-epidemiological uncertainties of emerging plant diseases: The challenge of predicting Xylella fastidiosa dynamics in novel environments. Phytopathology® 110, 1740–1750. doi:10.1094/PHYTO-03-20-0098-RVW
Ohkuma M. (2008). Symbioses of flagellates and prokaryotes in the gut of lower termites. Trends Microbiol. 16, 345–352. doi:10.1016/j.tim.2008.04.004
Opoku J., Kleczewski N. M., Hamby K. A., Herbert D. A., Malone S., Mehl H. L. (2019). Relationship between invasive Brown Marmorated Stink Bug (Halyomorpha halys) and fumonisin contamination of field corn in the mid-Atlantic US. Plant Dis. 103, 1189–1195. doi:10.1094/PDIS-06-18-1115-RE
Pai H.-H., Wu S.-C., Hsu E.-L. (2005). Insecticide resistance in German cockroaches (Blattella germanica) from hospitals and households in Taiwan. Int. J. Environ. Health Res. 15, 33–40. doi:10.1080/09603120400018816
Panini M., Manicardi G. C., Moores G. D., Mazzoni E. (2016). An overview of the main pathways of metabolic resistance in insects. Invertebr. Surviv. J. 13 (1), 326–335. doi:10.25431/1824-307X/isj.v13i1.326-335
Pimentel D., Lach L., Zuniga R., Morrison D. (2000). Environmental and economic costs of nonindigenous species in the United States. Bioscience 50, 53–65. doi:10.1641/0006-3568(2000)050[0053:eaecon]2.3.co;2
Pimentel D., Zuniga R., Morrison D. (2005). Update on the environmental and economic costs associated with alien-invasive species in the United States. Ecol. Econ. 52, 273–288. doi:10.1016/j.ecolecon.2004.10.002
Prattley D. J. (2009). Risk-based suveillance in animal health: A thesis presented in partial fulfillment of the requirements for the degree of doctor of philosophy at. Palmerston North, New Zealand: Massey University.
Puinean A. M., Foster S. P., Oliphant L., Denholm I., Field L. M., Millar N. S., et al. (2010). Amplification of a cytochrome P450 gene is associated with resistance to neonicotinoid insecticides in the aphid Myzus persicae. PLOS Genet. 6, e1000999. doi:10.1371/journal.pgen.1000999
Pull C. D., Ugelvig L. V., Wiesenhofer F., Grasse A. V., Tragust S., Schmitt T., et al. (2018). Destructive disinfection of infected brood prevents systemic disease spread in ant colonies. Elife 7, e32073. doi:10.7554/eLife.32073
Qiu B., Liu L., Li X., Mathur V., Qin Z., Ren S. (2009). Genetic mutations associated with chemical resistance in the cytochrome P450 genes of invasive and native Bemisia tabaci (Hemiptera: Aleyrodidae) populations in China. Insect Sci. 16, 237–245. doi:10.1111/j.1744-7917.2009.01253.x
Radcliffe E. B., Hutchison W. D., Cancelado R. E., Hutchinson W. D., Cancelado R. E. (2009). Integrated pest management: Concepts, tactics, strategies and case studies. Cambridge University Press.
Raghuteja P. V., Rao N. B. V. C., Padma E. (2022). A review on invasive pests of horticultural crop ecosystems. Biol. Forum. Int. J. 14 (3), 1209–1217.
Ramya S. L., Venkatesan T., Srinivasa Murthy K. S., Jalali S. K., Verghese A. (2016). Detection of carboxylesterase and esterase activity in culturable gut bacterial flora isolated from diamondback moth, Plutella xylostella (Linnaeus), from India and its possible role in indoxacarb degradation. Braz. J. Microbiol. 47, 327–336. doi:10.1016/j.bjm.2016.01.012
Rane R. V., Walsh T. K., Pearce S. L., Jermiin L. S., Gordon K. H. J., Richards S., et al. (2016). Are feeding preferences and insecticide resistance associated with the size of detoxifying enzyme families in insect herbivores? Curr. Opin. insect Sci. 13, 70–76. doi:10.1016/j.cois.2015.12.001
Ranganathan M., Narayanan M., Kumarasamy S. (2022). “Importance of metabolic enzymes and their role in insecticide resistance,” in New and future development in biopesticide research: Biotechnological exploration (Springer), 243.
Rao N. B. V. C., Roshan D. R., Rao G. K., Ramanandam G. (2018). A review on rugose spiralling whitefly, Aleurodicus rugioperculatus Martin (Hemiptera: Aleyrodidae) in India. J. Pharmacogn. Phytochem. 7 (5), 948–953.
Reitz S. R., Funderburk J. (2012). “Management strategies for Western flower thrips and the role of insecticides,” in Insecticides—Pest Engineering. IntechOpen. doi:10.5772/29355
Renault D., Elfiky A., Mohamed A. (2022). Predicting the insecticide-driven mutations in a crop pest insect: Evidence for multiple polymorphisms of acetylcholinesterase gene with potential relevance for resistance to chemicals. Environ. Sci. Pollut. Res. 1–19. doi:10.1007/s11356-022-23309-w
Reyes M., Sauphanor B. (2008). Resistance monitoring in codling moth: A need for standardization. Pest Manag. Sci. Former. Pestic. Sci. 64, 945–953. doi:10.1002/ps.1588
Ricas Rezende H., Malta Romano C., Morales Claro I., Santos Caleiro G., Cerdeira Sabino E., Felix A. C., et al. (2020). First report of Aedes albopictus infected by Dengue and Zika virus in a rural outbreak in Brazil. PLoS One 15, e0229847. doi:10.1371/journal.pone.0229847
Rinkevich F. D., Du Y., Dong K. (2013). Diversity and convergence of sodium channel mutations involved in resistance to pyrethroids. Pestic. Biochem. Physiol. 106, 93–100. doi:10.1016/j.pestbp.2013.02.007
Roat T. C., Carvalho S. M., Palma M. S., Malaspina O. (2017). Biochemical response of the Africanized honeybee exposed to fipronil. Environ. Toxicol. Chem. 36, 1652–1660. doi:10.1002/etc.3699
Rodríguez M. A., Bosch D., Sauphanor B., Avilla J. (2010). Susceptibility to organophosphate insecticides and activity of detoxifying enzymes in Spanish populations of cydia pomonella Lepidoptera: Tortricidae. J. Econ. Entomol. 103, 482–491. doi:10.1603/ec09249
Roe A. D., Torson A. S., Bilodeau G., Bilodeau P., Blackburn G. S., Cui M., et al. (2019). Biosurveillance of forest insects: Part I—integration and application of genomic tools to the surveillance of non-native forest insects. J. Pest Sci. 92, 51–70. doi:10.1007/s10340-018-1027-4
Rogers M. A., Burkness E. C., Hutchison W. D. (2016). Evaluation of high tunnels for management of Drosophila suzukii in fall-bearing red raspberries: Potential for reducing insecticide use. J. Pest Sci. 89, 815–821. doi:10.1007/s10340-016-0731-1
Rösner J., Wellmeyer B., Merzendorfer H. (2020). Tribolium castaneum: A model for investigating the mode of action of insecticides and mechanisms of resistance. Curr. Pharm. Des. 26, 3554–3568. doi:10.2174/1381612826666200513113140
Rugman-Jones P. F., Seybold S. J., Graves A. D., Stouthamer R. (2015). Phylogeography of the walnut twig beetle, Pityophthorus juglandis, the vector of thousand cankers disease in North American walnut trees. PLoS One 10, e0118264. doi:10.1371/journal.pone.0118264
Saccaggi D. L., Karsten M., Robertson M. P., Kumschick S., Somers M. J., Wilson J. R. U., et al. (2016). Methods and approaches for the management of arthropod border incursions. Biol. Invasions 18, 1057–1075. doi:10.1007/s10530-016-1085-6
Sandercock B. K., Davey M. L., Endrestøl A., Blaalid R., Fossøy F., Hegre H., et al. (2022). Designing a surveillance program for early detection of alien plants and insects in Norway. Biol. Invasions 24, 1–20. doi:10.1007/s10530-022-02957-6
Sarfraz M., Keddie A. B., Dosdall L. M. (2005). Biological control of the diamondback moth, Plutella xylostella: A review. Biocontrol Sci. Technol. 15, 763–789. doi:10.1080/09583150500136956
Sarkar M., Bhattacharyya I. K., Borkotoki A., Goswami D., Rabha B., Baruah I., et al. (2009). Insecticide resistance and detoxifying enzyme activity in the principal bancroftian filariasis vector, Culex quinquefasciatus, in northeastern India. Med. Vet. Entomol. 23, 122–131. doi:10.1111/j.1365-2915.2009.00805.x
Sarwar M., Salman M. (2015). Insecticides resistance in insect pests or vectors and development of novel strategies to combat its evolution. Int. J. Bioinforma. Biomed. Eng. 1 (3), 344–215.
Saulich A. K. (2010). Long life cycles in insects. Entomol. Rev. 90, 1127–1152. doi:10.1134/s0013873810090010
Savary S., Ficke A., Aubertot J.-N., Hollier C. (2012). Crop losses due to diseases and their implications for global food production losses and food security. Food secur. 4, 519–537. doi:10.1007/s12571-012-0200-5
Schneider D. S., Ayres J. S. (2008). Two ways to survive infection: What resistance and tolerance can teach us about treating infectious diseases. Nat. Rev. Immunol. 8, 889–895. doi:10.1038/nri2432
Schuler M. A. (2011). P450s in plant–insect interactions. Biochim. Biophys. Acta (BBA)-Proteins Proteomics 1814, 36–45. doi:10.1016/j.bbapap.2010.09.012
Şengül Demirak M. Ş., Canpolat E. (2022). Plant-based bioinsecticides for mosquito control: Impact on insecticide resistance and disease transmission. Insects 13, 162. doi:10.3390/insects13020162
Shabbir M. Z., Yang X., Batool R., Yin F., Kendra P. E., Li Z.-Y. (2021). Bacillus thuringiensis and chlorantraniliprole trigger the expression of detoxification-related genes in the larval midgut of Plutella xylostella. Front. Physiol. 2113, 780255. doi:10.3389/fphys.2021.780255
Shi Y., Huang Z., Han S., Fan S., Yang H. (2015). Phylogenetic diversity of Archaea in the intestinal tract of termites from different lineages. J. Basic Microbiol. 55, 1021–1028. doi:10.1002/jobm.201400678
Siddiqui J. A., Bamisile B. S., Khan M. M., Islam W., Hafeez M., Bodlah I., et al. (2021). Impact of invasive ant species on native fauna across similar habitats under global environmental changes. Environ. Sci. Pollut. Res. 28 (39), 54362–54382. doi:10.1007/s11356-021-15961-5
Siddiqui J. A., Khan M. M., Bamisile B. S., Hafeez M., Qasim M., Rasheed M. T., et al. (2022a). Role of insect gut microbiota in pesticide degradation: A review. Front. Microbiol. 0, 870462. doi:10.3389/fmicb.2022.870462
Siddiqui J. A., Luo Y., Sheikh U. A. A., Bamisile B. S., Khan M. M., Imran M., et al. (2022b). Transcriptome analysis reveals differential effects of beta-cypermethrin and fipronil insecticides on detoxification mechanisms in Solenopsis invicta. Front. Physiol. 13, 1018731. doi:10.3389/fphys.2022.1018731
Siddiqui J. A., Zhang Y., Luo Y., Bamisile B. S., Rehman N. U., Islam W., et al. (2022c). Comprehensive detoxification mechanism assessment of red imported fire ant (Solenopsis invicta) against indoxacarb. Molecules 27, 870. doi:10.3390/molecules27030870
Sileshi G. W., Gebeyehu S., Mafongoya P. L. (2019). The threat of alien invasive insect and mite species to food security in Africa and the need for a continent-wide response. Food secur. 11, 763–775. doi:10.1007/s12571-019-00930-1
Silva A. X., Jander G., Samaniego H., Ramsey J. S., Figueroa C. C. (2012). Insecticide resistance mechanisms in the green peach aphid Myzus persicae (Hemiptera: Aphididae) I: A transcriptomic survey. PLoS One 7, e36366. doi:10.1371/journal.pone.0036366
Silva R., Furlong M. J. (2012). Diamondback moth oviposition: Effects of host plant and herbivory. Entomol. Exp. Appl. 143, 218–230. doi:10.1111/j.1570-7458.2012.01255.x
Singh R. V., Malik M., Kanojia A. K., Singode A. (2018). A review paper on adoption behavior of vegetable growers towards Pest Management Practices in Bulandshahr (UP), India. Int. J. Curr. Microbiol. Appl. Sci. 7 (7), 1364–1372. doi:10.20546/ijcmas.2018.707.162
Sood S., Sharma A., Sharma N., Kanwar S. S. (2016). Carboxylesterases: Sources, characterization and broader applications. Insight Enzym Res. 1 (1), 1–11. doi:10.21767/2573-4466.100002
Souza D., Jiménez A. V., Sarath G., Meinke L. J., Miller N. J., Siegfried B. D. (2020). Enhanced metabolism and selection of pyrethroid-resistant Western corn rootworms (Diabrotica virgifera virgifera LeConte). Pestic. Biochem. Physiol. 164, 165–172. doi:10.1016/j.pestbp.2020.01.009
Sparks T. C., Lockwood J. A., Byford R. L., Graves J. B., Leonard B. R. (1989). The role of behavior in insecticide resistance. Pestic. Sci. 26, 383–399. doi:10.1002/ps.2780260406
Staudinger J. L., Xu C., Cui Y. J., Klaassen C. D. (2010). Nuclear receptor-mediated regulation of carboxylesterase expression and activity. Expert Opin. Drug Metab. Toxicol. 6, 261–271. doi:10.1517/17425250903483215
Stöck M., Dedukh D., Reifová R., Lamatsch D. K., Starostová Z., Janko K. (2021). Sex chromosomes in meiotic, hemiclonal, clonal and polyploid hybrid vertebrates: Along the ‘extended speciation continuum. Philos. Trans. R. Soc. B 376, 20200103. doi:10.1098/rstb.2020.0103
Strand M. R., Burke G. R. (2013). Polydnavirus-wasp associations: Evolution, genome organization, and function. Curr. Opin. Virol. 3, 587–594. doi:10.1016/j.coviro.2013.06.004
Stratonovitch P., Elias J., Denholm I., Slater R., Semenov M. A. (2014). An individual-based model of the evolution of pesticide resistance in heterogeneous environments: Control of meligethes aeneus population in oilseed rape crops. PLoS One 9, e115631. doi:10.1371/journal.pone.0115631
Subramanyam B., Hagstrum D. W. (2018). “Resistance measurement and management,” in Integrated management of insects in stored products (Boca Raton, Florida: CRC Press), 331.
Sudo M., Takahashi D., Andow D. A., Suzuki Y., Yamanaka T. (2018). Optimal management strategy of insecticide resistance under various insect life histories: Heterogeneous timing of selection and interpatch dispersal. Evol. Appl. 11, 271–283. doi:10.1111/eva.12550
Sudweeks J., Hollingsworth B., Blondel D. V., Campbell K. J., Dhole S., Eisemann J. D., et al. (2019). Locally fixed alleles: A method to localize gene drive to island populations. Sci. Rep. 9 (15821), 1–10. doi:10.1038/s41598-019-51994-0
Sujatha G. S., Singh M. K., Mahanta D. K., Bala A. (2022). Pest management through ecological manipulation. Vigyan Varta 3, 40–43.
Sun H., Nomura Y., Du Y., Liu Z., Zhorov B. S., Dong K. (2022a). Characterization of two kdr mutations at predicted pyrethroid receptor site 2 in the sodium channels of Aedes aegypti and Nilaparvata lugens. Insect biochem. Mol. Biol. 148, 103814. doi:10.1016/j.ibmb.2022.103814
Sun J., Li C., Jiang J., Song C., Wang C., Feng K., et al. (2022b). Cross resistance, inheritance and fitness advantage of cyetpyrafen resistance in two-spotted spider mite, Tetranychus urticae. Tetranychus Urticae. Pestic. Biochem. Physiol. 183, 105062. doi:10.1016/j.pestbp.2022.105062
Sun J., Lu M., Gillette N. E., Wingfield M. J. (2013). Red turpentine beetle: Innocuous native becomes invasive tree killer in China. Annu. Rev. Entomol. 58, 293–311. doi:10.1146/annurev-ento-120811-153624
Taha H. S. E.-D. (2022). Teflubenzuron toxicity impact and resistance consequences on Plutella xylostella L. (Lepidoptera: Plutellidae) fitness. Asian J. Res. Rev. Agric. 42 (2), 42–49.
Taillebois E., Thany S. H. (2022). The use of insecticide mixtures containing neonicotinoids as a strategy to limit insect pests: Efficiency and mode of action. Pestic. Biochem. Physiol. 184, 105126. doi:10.1016/j.pestbp.2022.105126
Tang B., Xu K., Liu Y., Zhou Z., Karthi S., Yang H., et al. (2022). A review of physiological resistance to insecticide stress in Nilaparvata lugens. 3 Biotech. 12, 84–88. doi:10.1007/s13205-022-03137-y
Tangtrakulwanich K., Reddy G. V. P. (2014). Development of insect resistance to plant biopesticides: An overview. Adv. plant Biopestic., 47.
Theis F. J., Ugelvig L. V., Marr C., Cremer S. (2015). Opposing effects of allogrooming on disease transmission in ant societies. Philos. Trans. R. Soc. B Biol. Sci. 370, 20140108. doi:10.1098/rstb.2014.0108
Thia J. A., Hoffmann A. A., Umina P. A. (2021). Empowering Australian insecticide resistance research with genetic information: The road ahead. John Wiley & Sons.
Traniello J. F. A., Rosengaus R. B., Savoie K. (2002). The development of immunity in a social insect: Evidence for the group facilitation of disease resistance. Proc. Natl. Acad. Sci. 99, 6838–6842. doi:10.1073/pnas.102176599
Valmorbida I., Hohenstein J. D., Coates B. S., Bevilaqua J. G., Menger J., Hodgson E. W., et al. (2022). Association of voltage-gated sodium channel mutations with field-evolved pyrethroid resistant phenotypes in soybean aphid and genetic markers for their detection. Sci. Rep. 12 (1), 1–14. doi:10.1038/s41598-022-16366-1
Vassiliou V., Emmanouilidou M., Perrakis A., Morou E., Vontas J., Tsagkarakou A., et al. (2011). Insecticide resistance in Bemisia tabaci from Cyprus. Insect Sci. 18, 30–39. doi:10.1111/j.1744-7917.2010.01387.x
Venette R. C. (2017). Climate analyses to assess risks from invasive forest insects: Simple matching to advanced models. Curr. For. Rep. 3, 255–268. doi:10.1007/s40725-017-0061-4
Venette R. C., Hutchison W. D., Hutchison W. D. (2021). Invasive insect species: Global challenges, strategies & opportunities. Front. Insect Sci. 1, 8–11. doi:10.3389/finsc.2021.650520
Venette R. C., Kriticos D. J., Magarey R. D., Koch F. H., Baker R. H. A., Worner S. P., et al. (2010). Pest risk maps for invasive alien species: A roadmap for improvement. Bioscience 60, 349–362. doi:10.1525/bio.2010.60.5.5
Venette R. C., Morey A. C. (2020). Advances in understanding the ecology of invasive crop insect pests and their impact on IPM. Integr. Manag. insect pests Curr. Futur. Dev. Chapter 6. in: Editors M. Kogan, and E. A. Heinrichs. Cambridge, United Kingdom: Burleigh Dodds Science Publishing, 30. doi:10.19103/AS.2019.0047.06
Venkatesan T., Chethan B. R., Mani M. (2022). Insecticide resistance and its management in the insect pests of horticultural crops. Editors M. Mani. Springer, Singapore: Trends in Horticultural Entomology, 455–490. doi:10.1007/978-981-19-0343-4_14
Vontas J., Hernández-Crespo P., Margaritopoulos J. T., Ortego F., Feng H.-T., Mathiopoulos K. D., et al. (2011). Insecticide resistance in Tephritid flies. Pestic. Biochem. Physiol. 100, 199–205. doi:10.1016/j.pestbp.2011.04.004
Vyas T., Singh V., Kodgire P., Joshi A. (2022). Insights in detection and analysis of organophosphates using organophosphorus acid anhydrolases (OPAA) enzyme-based biosensors. Crit. Rev. Biotechnol. 1–19. doi:10.1080/07388551.2022.2052012
Wang R.-L., Staehelin C., Xia Q.-Q., Su Y.-J., Zeng R.-S. (2015). Identification and characterization of CYP9A40 from the tobacco cutworm moth (Spodoptera litura), a cytochrome P450 gene induced by plant allelochemicals and insecticides. Int. J. Mol. Sci. 16, 22606–22620. doi:10.3390/ijms160922606
Wang S., Zhang A., Li L., Men X., Zhou X., Zhai Y., et al. (2014). Insecticide resistance status of field populations of Frankliniella occidentalis (Thysanoptera: Thripidae) in China and its control strategies. Acta Entomol. Sin. 57, 621.
Wang X., Chen Y., Gong C., Yao X., Jiang C., Yang Q. (2018a). Molecular identification of four novel cytochrome P450 genes related to the development of resistance of Spodoptera exigua (Lepidoptera: Noctuidae) to chlorantraniliprole. Pest Manag. Sci. 74, 1938–1952. doi:10.1002/ps.4898
Wang X., Huang Q., Hao Q., Ran S., Wu Y., Cui P., et al. (2018b). Insecticide resistance and enhanced cytochrome P450 monooxygenase activity in field populations of Spodoptera litura from Sichuan, China. Crop Prot. 106, 110–116. doi:10.1016/j.cropro.2017.12.020
Wang Z. H., Hou W. J., Hao C. Y., Wu Q. J., Xu B. Y., Zhang Y. J. (2011). Monitoring the insecticide resistance of the field populations of Western flower thrips, Frankliniella occidentalis in Beijing area. J. Appl. Entomogr. 48 (3), 542–547. Bei jing di qu xi hua ji ma tian jian zhong qun kang yao xing jian ce. 北京地区西花蓟马田间种群抗药性监测 ying yong kun chong xue bao. 应用昆虫学报.
Welter S. C., Varela L., Freeman R. (1991). Codling moth resistance to azinphosmethyl in California. Resist. Pest Manag. Newslett 3, 12.
Wen C., Chen J., Qin W. Q., Chen X., Cai J. C., Wen J. B., et al. (2020). Red imported fire ants (Hymenoptera: Formicidae) cover inaccessible surfaces with particles to facilitate food search and transportation. Insect Sci. 28, 1816–1828. doi:10.1111/1744-7917.12891
Wen C., Sheng L., Chen J., Zhang J., Feng Y., Wang Z., et al. (2021). Red imported fire ants (Hymenoptera: Formicidae) cover the insecticide-treated surfaces with particles to reduce contact toxicity. J. Pest Sci. 95 (3), 1135–1150. doi:10.1007/s10340-021-01474-0
Whyard S., Downe A. E. R., Walker V. K. (1994). Isolation of an esterase conferring insecticide resistance in the mosquito Culex tarsalis. Insect biochem. Mol. Biol. 24, 819–827. doi:10.1016/0965-1748(94)90110-4
Wilson-Rich N., Spivak M., Fefferman N. H., Starks P. T. (2009). Genetic, individual, and group facilitation of disease resistance in insect societies. Annu. Rev. Entomol. 54, 405–423. doi:10.1146/annurev.ento.53.103106.093301
Wojciechowska M., Stepnowski P., Gołębiowski M. (2016). The use of insecticides to control insect pests. Invertebr. Surviv. J. 13 (1), 210–220. doi:10.25431/1824-307X/isj.v13i1.210-220
Wondji C. S., Irving H., Morgan J., Lobo N. F., Collins F. H., Hunt R. H., et al. (2009). Two duplicated P450 genes are associated with pyrethroid resistance in Anopheles funestus, a major malaria vector. Genome Res. 19, 452–459. doi:10.1101/gr.087916.108
Wu C., Ding C., Chen S., Wu X., Zhang L., Song Y., et al. (2021). Exposure of Helicoverpa armigera Larvae to plant volatile organic compounds induces cytochrome P450 monooxygenases and enhances larval tolerance to the insecticide methomyl. Insects 12, 238. doi:10.3390/insects12030238
Wu S., Yang Y., Yuan G., Campbell P. M., Teese M. G., Russell R. J., et al. (2011). Overexpressed esterases in a fenvalerate resistant strain of the cotton bollworm, Helicoverpa armigera. Insect biochem. Mol. Biol. 41, 14–21. doi:10.1016/j.ibmb.2010.09.007
Wu Y., Zheng Y., Chen Y., Wang S., Chen Y., Hu F., et al. (2020). Honey bee (Apis mellifera) gut microbiota promotes host endogenous detoxification capability via regulation of P450 gene expression in the digestive tract. Microb. Biotechnol. 13, 1201–1212. doi:10.1111/1751-7915.13579
Wyckhuys K. A. G., Lu Y., Zhou W., Cock M. J. W., Naranjo S. E., Fereti A., et al. (2020). Ecological pest control fortifies agricultural growth in Asia–Pacific economies. Nat. Ecol. Evol. 4, 1522–1530. doi:10.1038/s41559-020-01294-y
Xia X., Sun B., Gurr G. M., Vasseur L., Xue M., You M. (2018). Gut microbiota mediate insecticide resistance in the diamondback moth, Plutella xylostella (L.). Front. Microbiol. 9, 25. doi:10.3389/fmicb.2018.00025
Xie W., Yang X., Chen C., Yang Z., Guo L., Wang D., et al. (2018). The invasive MED/Q Bemisia tabaci genome: A tale of gene loss and gene gain. BMC Genomics 19, 68–15. doi:10.1186/s12864-018-4448-9
Xing Y. F., Liu Z. H., Zhang R. M., Zhou D., Sun Y., Ma L., et al. (2021). Effect of the midgut symbiotic Aeromonas hydrophila on the deltamethrin resistance of Culex pipiens pallens. J. Pathog. Biol. 16, 661–666.
Xiong M., Jiang W., Li G. (2010). Monitoring of insecticide resistance in larvae of Leptinotarsa decemlineata in Xinjiang Uygur autonomous region by diagnostic dose. Chin. J. Appl. Entomology 47 (04), 763–767. Zhen duan ji liang fa jian ce xin jiang wei wu er zi zhi qu ma ling shu jia chong de kang yao xing. 诊断剂量法监测新疆维吾尔自治区马铃薯甲虫的抗药性 kun chong zhi shi, 昆虫知识.
Xiong T., Ling S., quan Liu, li J., Zeng X. nian (2022). Insecticidal and P450 mediate metabolism of fluralaner against red imported fire ant, Solenopsis invicta (Hymenoptera: Formicidae). Pestic. Biochem. Physiol. 187, 105184. doi:10.1016/j.pestbp.2022.105184
Xu J., Fonseca D. M., Hamilton G. C., Hoelmer K. A., Nielsen A. L. (2014). Tracing the origin of US Brown marmorated stink bugs, Halyomorpha halys. Biol. Invasions 16, 153–166. doi:10.1007/s10530-013-0510-3
Xu P., Yang L., Yang X., Li T., Graham R. I., Wu K., et al. (2020). Novel partiti-like viruses are conditional mutualistic symbionts in their normal lepidopteran host, African armyworm, but parasitic in a novel host, Fall armyworm. PLoS Pathog. 16, e1008467. doi:10.1371/journal.ppat.1008467
Xu X., Ding Q., Wang X., Wang R., Ullah F., Gao X., et al. (2022). V101I and R81T mutations in the nicotinic acetylcholine receptor β1 subunit are associated with neonicotinoid resistance in Myzus persicae. Pest Manag. Sci. 78, 1500–1507. doi:10.1002/ps.6768
Yadav R., Singh S., Singh A. N. (2022). Biopesticides: Current status and future prospects. Proc. Int. Acad. Ecol. Environ. Sci. 12, 211.
Yan C., Qinghai F., Jingwei Z. (1998). Toxicity of several insecticides to the Liriomyza sativae Blanchard and the resistance of L. sativae to the insecticides. Wuyi Sci. J. 14, 185–188.
Yang X., Xie W., Wang S., Wu Q., Xu B., Zhou X., et al. (2014). Resistance status of the whitefly, Bemisia tabaci, in Beijing, Shandong and Hunan areas and relative levels of CYP4v2 and CYP6 CX1 mRNA expression. Plant Prot. 40 (4), 70–75. bei jing shan dong he hu nan di qu yan fen shi kang yao xing ji CYP4v2he CYP6CX1 mRNAshui ping biao da liang fen xi. 北京、山东和湖南地区烟粉虱抗药性及 CYP4v2 和CYP6CX1 mRNA水平表达量分析 zhi wu bao hu, 植物保护.
Yemshanov D., Haight R. G., Koch F. H., Lu B., Venette R., Fournier R. E., et al. (2017). Robust surveillance and control of invasive species using a scenario optimization approach. Ecol. Econ. 133, 86–98. doi:10.1016/j.ecolecon.2016.11.018
Yemshanov D., Haight R. G., Koch F. H., Venette R. C., Swystun T., Fournier R. E., et al. (2019). Optimizing surveillance strategies for early detection of invasive alien species. Ecol. Econ. 162, 87–99. doi:10.1016/j.ecolecon.2019.04.030
Yonggyun K., Cho J. R., Lee J., Kang S., Han S. C., Hong K. J., et al. (1998). Insecticide resistance in the tobacco cutworm, Spodoptera litura (Fabricius)(Lepidoptera: Noctuiae). J. Asia. Pac. Entomol. 1, 115–122. doi:10.1016/s1226-8615(08)60013-8
Yoon K. A., Kim J. H., Nauen R., Alyokhin A., Clark J. M., Lee S. H. (2022). Characterization of molecular and kinetic properties of two acetylcholinesterases from the Colorado potato beetle, Leptinotarsa decemlineata. Pestic. Biochem. Physiol. 185, 105137. doi:10.1016/j.pestbp.2022.105137
Young S. J., Gunning R. V., Moores G. D. (2005). The effect of piperonyl butoxide on pyrethroid-resistance-associated esterases in Helicoverpa armigera (H??bner) (Lepidoptera: Noctuidae). Pest Manag. Sci. 61, 397–401. doi:10.1002/ps.996
Yu L., Tang W., He W., Ma X., Vasseur L., Baxter S. W., et al. (2015). Characterization and expression of the cytochrome P450 gene family in diamondback moth, Plutella xylostella (L.). Sci. Rep. 5, 8952–8959. doi:10.1038/srep08952
Yu L., Yang H., Cheng F., Wu Z., Huang Q., He X., et al. (2021). Honey bee Apis mellifera larvae gut microbial and immune, detoxication responses towards flumethrin stress. Environ. Pollut. 290, 118107. doi:10.1016/j.envpol.2021.118107
Yu S. J. (1991). Insecticide resistance in the fall armyworm, Spodoptera frugiperda (JE Smith). Pestic. Biochem. Physiol. 39, 84–91. doi:10.1016/0048-3575(91)90216-9
Yu S. J., Nguyen S. N., Abo-Elghar G. E. (2003). Biochemical characteristics of insecticide resistance in the fall armyworm, Spodoptera frugiperda (JE Smith). Pestic. Biochem. Physiol. 77, 1–11. doi:10.1016/s0048-3575(03)00079-8
Zago H. B., Siqueira H. A. A., Pereira E. J. G., Picanço M. C., Barros R. (2014). Resistance and behavioural response of Plutella xylostella (Lepidoptera: Plutellidae) populations to Bacillus thuringiensis formulations. Pest Manag. Sci. 70, 488–495. doi:10.1002/ps.3600
Zhang H., Zhao M., Liu Y., Zhou Z., Guo J. (2018). Identification of cytochrome P450 monooxygenase genes and their expression in response to high temperature in the alligatorweed flea beetle Agasicles hygrophila (Coleoptera: Chrysomelidae). Sci. Rep. 8 (1), 1–13. doi:10.1038/s41598-018-35993-1
Zhang J., Ai Z., Liu H., Tang D. W. S., Yang X., Wang G., et al. (2022a). Short-term N addition in a Pinus tabuliformis plantation: Microbial community composition and interactions show different linkages with ecological stoichiometry. Appl. Soil Ecol. 174, 104422. doi:10.1016/j.apsoil.2022.104422
Zhang L., Gao X., Liang P. (2007). Beta-cypermethrin resistance associated with high carboxylesterase activities in a strain of house fly, Musca domestica (Diptera: Muscidae). Pestic. Biochem. Physiol. 89, 65–72. doi:10.1016/j.pestbp.2007.03.001
Zhang S., Zhou Y., Yu R., Xu X., Xu M., Li G., et al. (2022b). China’s biodiversity conservation in the process of implementing the sustainable development goals (SDGs). J. Clean. Prod. 338, 130595. doi:10.1016/j.jclepro.2022.130595
Zhang Z. l., Chen W. L., Wang J. (1980). Preliminary observation and prevention of the occurrence of Trialeurodes vaporariorum on the outskirts of Beijing. Insect Knowl. 04, 158–216. Jing jiao wen shi bai fen shi fa sheng de chu bu guan cha he fang zhi. 京郊温室白粉虱发生的初步观察和防治 kun chong zhi shi. 昆虫知识.
Zhao G., Liu W. E. I., Brown J. M., Knowles C. O. (1995). Insecticide resistance in field and laboratory strains of Western flower thrips (Thysanoptera: Thripidae). J. Econ. Entomol. 88, 1164–1170. doi:10.1093/jee/88.5.1164
Zhao M., Lin X., Guo X. (2022). The role of insect symbiotic bacteria in metabolizing phytochemicals and agrochemicals. Insects 13, 583. doi:10.3390/insects13070583
Zhou C. S., Lv H. H., Guo X. H., Cao Q., Zhang R. X., Ma D. Y. (2022). Transcriptional analysis of Bemisia tabaci MEAM1 cryptic species under the selection pressure of neonicotinoids imidacloprid, acetamiprid and thiamethoxam. BMC Genomics 23, 15–12. doi:10.1186/s12864-021-08241-6
Zhou X., Ma C., Li M., Sheng C., Liu H., Qiu X. (2010). CYP9A12 and CYP9A17 in the cotton bollworm, Helicoverpa armigera: Sequence similarity, expression profile and xenobiotic response. Pest Manag. Sci. Former. Pestic. Sci. 66, 65–73. doi:10.1002/ps.1832
Keywords: biological invasion, insecticide resistance, biodiversity risk, exotic species, resistance mechanisms
Citation: Siddiqui JA, Fan R, Naz H, Bamisile BS, Hafeez M, Ghani MI, Wei Y, Xu Y and Chen X (2023) Insights into insecticide-resistance mechanisms in invasive species: Challenges and control strategies. Front. Physiol. 13:1112278. doi: 10.3389/fphys.2022.1112278
Received: 30 November 2022; Accepted: 21 December 2022;
Published: 09 January 2023.
Edited by:
Xun Zhu, Institute of Plant Protection (CAAS), ChinaReviewed by:
Xinzheng Huang, China Agricultural University, ChinaCopyright © 2023 Siddiqui, Fan, Naz, Bamisile, Hafeez, Ghani, Wei, Xu and Chen. This is an open-access article distributed under the terms of the Creative Commons Attribution License (CC BY). The use, distribution or reproduction in other forums is permitted, provided the original author(s) and the copyright owner(s) are credited and that the original publication in this journal is cited, in accordance with accepted academic practice. No use, distribution or reproduction is permitted which does not comply with these terms.
*Correspondence: Xiaoyulong Chen, Y2hlbnhpYW95dWxvbmdAc2luYS5jbg==
Disclaimer: All claims expressed in this article are solely those of the authors and do not necessarily represent those of their affiliated organizations, or those of the publisher, the editors and the reviewers. Any product that may be evaluated in this article or claim that may be made by its manufacturer is not guaranteed or endorsed by the publisher.
Research integrity at Frontiers
Learn more about the work of our research integrity team to safeguard the quality of each article we publish.