- 1Department of Cardiology, Faculty of Medicine in Pilsen, Charles University, Prague, Czechia
- 2Department of Physiology, Faculty of Medicine in Pilsen, Charles University, Prague, Czechia
- 3Department of Internal Medicine I, Faculty of Medicine in Pilsen, Charles University, Prague, Czechia
- 4Biomedical Center, Faculty of Medicine in Pilsen, Charles University, Prague, Czechia
Infectious diseases, which often result in deadly sepsis or septic shock, represent a major global health problem. For understanding the pathophysiology of sepsis and developing new treatment strategies, reliable and clinically relevant animal models of the disease are necessary. In this review, two large animal (porcine) models of sepsis induced by either peritonitis or bacteremia are introduced and their strong and weak points are discussed in the context of clinical relevance and other animal models of sepsis, with a special focus on cardiovascular and immune systems, experimental design, and monitoring. Especially for testing new therapeutic strategies, the large animal (porcine) models represent a more clinically relevant alternative to small animal models, and the findings obtained in small animal (transgenic) models should be verified in these clinically relevant large animal models before translation to the clinical level.
1 Introduction
Infectious diseases and their associated sepsis and septic shock remain a global health problem, with a steadily growing yearly incidence of up to 20 million cases and 5 million sepsis-related deaths worldwide (Fleischmann et al., 2016). They are among the most severe life-threatening conditions in hospital ICUs, with a mortality rate of approximately 30% (Fleischmann et al., 2016). Epidemiological studies show sepsis as one of the most crucial healthcare problems, with a significant socioeconomic impact and high financial burden for public health systems—24 billion USD is spent annually in the USA, and the situation is similar in other developed countries (Torio and Moore, 2006; Liu et al., 2014; Mellhammar et al., 2016).
Sepsis is defined as life-threatening organ dysfunction caused by a dysregulated host response to infection (SEPSIS-3) (Singer et al., 2016), which corresponds to the stage of severe sepsis according to the earlier SEPSIS-1 and SEPSIS-2 definitions (Levy et al., 2003). Organ dysfunction can be identified as an acute change in the total Sequential Organ Failure Assessment (SOFA) score by >2 points. The SOFA score focuses on six vital organ systems (Figure 1): the respiratory system (PaO2/FiO2), hemostasis (number of thrombocytes), the liver (plasma levels of bilirubin), the kidneys (plasma levels of creatinine and urine volume), the central nervous system (Glasgow coma scale), and the cardiovascular system (mean arterial pressure, vasopressor support) (Singer et al., 2016; Napolitano, 2018). Septic shock is then defined as a subset of sepsis in which underlying circulatory and cellular/metabolic abnormalities are profound enough to substantially increase mortality. Patients with septic shock can be identified with a clinical construct of sepsis with persisting hypotension requiring vasopressors to maintain mean arterial pressure above 65 mmHg and having a serum lactate level above 2 mmol/L (Singer et al., 2016). For a quick bedside clinical judgement, the qSOFA (quick SOFA), which only incorporates three parameters (altered mentation, systolic blood pressure of 100 mm Hg or less, and respiratory rate of 22/min or greater) has been suggested. Although qSOFA is less robust than SOFA, it does not require laboratory tests and can be assessed quickly and repeatedly. The qSOFA criteria should be used to prompt clinicians to further investigate organ dysfunction, to initiate or escalate therapy as appropriate, and to consider referral to critical care or increase the frequency of monitoring (Singer et al., 2016; Raith et al., 2017).
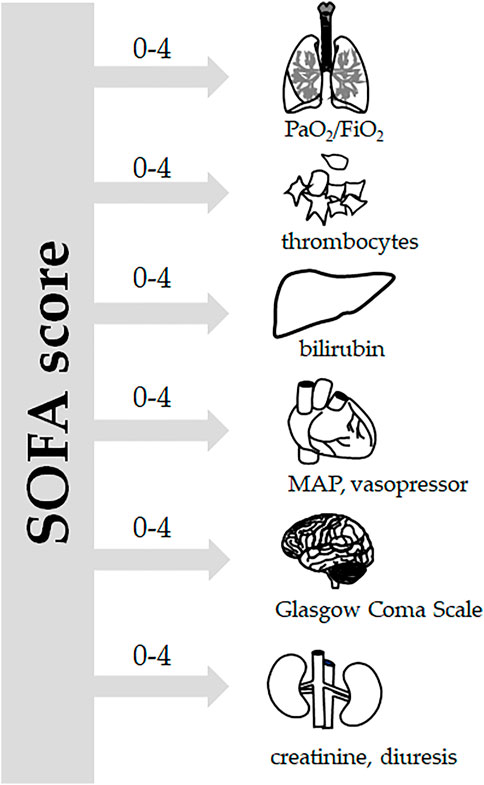
FIGURE 1. SOFA score. According to the Sepsis-3 definitions (Singer et al., 2016), sepsis is defined as life-threatening organ dysfunction caused by a dysregulated host response to infection. For clinical operationalization, organ dysfunction can be represented by an increase in the Sequential [Sepsis-related] Organ Failure Assessment (SOFA) score of two points or more. The SOFA score is based on grading six vital systems: respiration (PaO2/FiO2), coagulation (number of thrombocytes), the liver (bilirubin plasma levels), the cardiovascular system (mean arterial pressure and vasopressor support), the central nervous system (Glasgow Coma Scale), and the renal system (creatinine plasma levels, urine output), each of them scoring 0–4 points. In porcine experiments conducted under general anesthesia, the Glasgow coma scale-based neurologic component is excluded.
Animal models allow for a detailed investigation of the pathophysiological mechanisms of the disease; however, the translation of the findings to the clinical level is often complicated. Therefore, in this review, animal models of sepsis were analyzed with an emphasis on their clinical relevance. Small and large animal models of sepsis were compared to reveal their main advantages and disadvantages with special focus on cardiovascular and immune systems. As highly clinically relevant models, two porcine models of sepsis (peritonitis- and bacteremia-induced), which are used routinely in our lab, were scrutinized in detail, including methodological aspects of the experiments.
2 Large vs. small animal models
Despite significant progress in our understanding of this clinical syndrome, the pathophysiology of sepsis remains unclear and there is an increasing need to improve our understanding of sepsis and develop new effective treatments for this deadly condition. Obviously, reliable and clinically relevant animal models are necessary for understanding the complex pathophysiological concepts of sepsis as well as for developing new effective treatment options. Many preclinical studies have been conducted, with promising results; however, most of them could not be successfully transferred to clinical situations. Knowledge transfer is complicated by the heterogeneity and complexity of the animal models used (Table 1). Small preclinical models are often used for modeling specific clinical situations; however, their translational potential is limited. When compared to the human phenotype of sepsis, significant differences exist in terms of their inflammatory, immune, metabolic, and hemodynamic responses to infection insult (Reade and Young, 2003; Tao et al., 2004; Alverdy et al., 2020; Wang et al., 2022). Although there are specific advantages to using genetically engineered mouse and rat models, there is an urgent need for clinically relevant large animal models that allow for an easy and reliable clinical translation. Large porcine models show, similar to humans, a hyperdynamic circulation response to infectious insult, which is in contrast to small animal (mouse, rat) models with a hypodynamic response (low cardiac output and hypotension) (Fink and Heard, 1990; Park et al., 2019). Porcine anatomy and physiology are, in general, similar to those of humans, e.g., a similar heartrate range, force–frequency relationship, and cardiac action potential duration (Meurens et al., 2012; Swindle et al., 2012). Furthermore, the similar size allows for the experimental use of regular clinical tools (probes, catheters) without modifications. When compared to other large animal model species, pigs probably represent the most plausible clinically relevant option. Since experiments with apes and dogs are currently being minimized due to ethical reasons (Balls, 2022), pigs and sheep are the most commonly used large species in sepsis research (Guillon et al., 2019). In sheep as ruminant animals, however, important differences in anatomy and physiology of the gastrointestinal tract exist, compared to omnivorous species (pig, human), which have significant metabolic consequences (Wolffram et al., 1986; Bergman 1990). On the other hand, also pigs show some species differences that may complicate clinical translation such as susceptibility to pulmonary dysfunction and acute pulmonary hypertension (Guillon et al., 2019) (Table 2).
3 The cardiovascular system in sepsis
Cardiovascular system has been extensively studied in both small and large animal models, which allows for a fair comparison with humans and a proper assessment of the possibility of clinical translation. From a practical point of view, large animal models (e.g., pigs) have an obvious advantage in that their size and macroanatomy resemble those of the human cardiovascular system. That allows for the easy use of clinical devices and tools in large animal models, including their experimental testing. Also, microstructural studies show similar characteristics for the human and porcine myocardium (Milani-Nejad and Janssen, 2014), with dominant expression of slow β–MHC (myosin heavy chain): in the porcine ventricular myocardium, it approaches 100%, with some regional variations (Stelzer et al., 2008; Locher et al., 2011); in human ventricles, expression levels of β–MHC above 90% were reported (Miyata et al., 2000; Reiser et al., 2001; Jin et al., 2017). Similar to in humans, porcine hearts express significant levels of both stiff (small) N2B and compliant (large) N2BA titin isoforms, although probably with some quantitative differences: in the porcine myocardium, a higher relative expression of the titin N2BA isoform was reported compared to in humans (Cazorla et al., 2000; Makarenko et al., 2004; Chung et al., 2011). In contrast to human and large (porcine) animal models, in the rat ventricular myocardium the fast α-MHC is dominantly expressed (>90%) (Wang et al., 2002), which has significant consequences for tension cost and kinetics (Rundell et al., 2005). Also, the expression pattern of titin isoforms in mice and rats differs from that in humans and pigs: in mice and rats, the dominant expression of N2B titin isoform with very low expression of the N2BA isoform was seen (Cazorla et al., 2000).
In general, mouse and rat hearts are adapted for very high heartrates (>600 bpm in mice), which is associated with a faster kinetics of both cardiac contraction and excitation (Milani-Nejad and Janssen, 2014). Cardiac action potential duration in mouse and rat is much shorter, and the shape is triangular without the dominant plateau phase (Odening et al., 2021). The major ionic currents responsible for repolarization are different from those in the human heart: the repolarization is mainly due to the fast and slow components of the transient outward current (Ito,f and Ito,s), together with the rapidly activating, slowly inactivating delayed rectifier potassium currents (IK, slow1 and IK, slow2), whereas the main repolarization currents of human ventricular myocardium IKr (rapid delayed rectifier potassium current) and IKs (slow delayed rectifier potassium current) are functionally irrelevant (Odening et al., 2021). On the other hand, the porcine heartrate is similar (if slightly higher) to that in humans, and porcine ventricular action potentials resemble those of humans in many aspects, including the configuration with a dominant plateau phase and duration (Odening et al., 2021). Major contributing ionic currents correspond to those of humans with one exception: the transient outward current (voltage-dependent, 4-aminopyridine-sensitive Ito) that is responsible for early repolarization in human ventricular myocytes is missing in the porcine myocardium (Li et al., 2003).
High resting heartrates in mice and rats limit the extent of additional heartrate increase, i.e., heartrate reserve, which is significantly smaller in mice and rats (∼40%) than in humans or pigs (∼150%) (Milani-Nejad and Janssen, 2014). The limited heartrate reserve, together with the rather flat force–frequency relationship in mice and rats, results in a significantly lower ability to increase cardiac output as the product of heartrate and stroke volume. The porcine cardiac reserve, on the other hand, is similar to that of humans, since the high heartrate reserve is accompanied by a positive force–frequency relationship (Vogel et al., 2003; Jarkovska et al., 2018). These differences may contribute to the differential septic response in small and large animal models, where a hypodynamic response with low cardiac output and hypotension is typical of mice and rats, whereas hyperdynamic circulation with a high cardiac output and reduced systemic vascular resistance develops in the porcine model as well as in human patients.
4 Immune system and sepsis modeling
The immune system plays a critical role in combating infectious stimulus and the progression of sepsis. Interspecies differences in immune mechanisms, therefore, significantly influence the outcome of the disease and potential clinical translation. A detailed analysis of portions of the porcine, mouse, and human genome associated with the immune response revealed that the porcine immune system is significantly more similar to human on the level of non-protein-coding RNA/DNA and protein-coding genes as well as proteins (Dawson and Lunney, 2018). There is a growing consensus that pigs, as monogastric omnivorous animals with an organization of the immune system very similar to that of humans, represents a suitable model for immunology, although some particularities like the inverted lymph node structure or the ileal Peyer’s patches that have no obvious anatomical equivalent in humans will require further attention (Rothkötter, 2009; Pabst, 2020). The high level of similarity between the human and porcine immune systems can also be documented by the cluster of differentiation (CD) markers: in humans, 419 proteins have been designated as CD markers, and in pigs 359 corresponding CD proteins have been identified (Dawson and Lunney, 2018). The distribution pattern of white blood cell populations in pigs is similar to that of humans, which is in contrast to mice and rats, in which the neutrophil population represents only 10%–25% of white blood cells (Fairbairn et al., 2011). Striking interspecies differences in immune effector pathways have also been described. In mice, following macrophage activation, nitric oxide (NO) is produced by calcium-independent inducible NO synthase (iNOS). NO exerts antimicrobial actions and regulates metabolic remodeling and the production of cytokines in proinflammatory macrophage (Bailey et al., 2019). It seems that these pathways are not induced in human or porcine macrophages, although there is some inconsistency in the literature about the expression of iNOS and the production of NO in human macrophages (Fairbairn et al., 2011). On the other hand, both human and porcine macrophages respond to lipopolysaccharide challenge with induction of IDO (indoleamine 2,3-dioxygenase), involved in the pathway of tryptophan metabolism, which is not the case with mouse macrophages (Kapetanovic et al., 2012). A systemic comparison of the genomic response between human inflammatory diseases (trauma, burns, and endotoxemia) and mouse models demonstrated that, although the genomic responses were highly similar in humans, they were not reproduced in mouse models (Seok et al., 2013), indicating fundamental differences in the inflammatory responses of these two species. Mice are also much more resistant (by several orders of magnitude) than humans to endotoxin shock (Warren et al., 2010). This in vivo discrepancy, however, was not paralleled by the in vitro response of macrophages in cell culture. The studies of the response of mouse and human macrophages in the microenvironment of mouse and human serum indicated that proteins in mouse serum markedly suppress the induction of proinflammatory cytokines compared to human serum (Warren et al., 2010).
Dysregulated immune response in sepsis is associated with the cytokine storm with overproduction of proinflammatory cytokines and other signaling molecules, which induce widespread endothelial dysfunction, impaired coagulation and multiple organ dysfunction (Cavaillon, 2018; Kumar, 2020). The signaling pathway of toll-like receptor 4 (TLR4) and nuclear factor κB (NF-κB) are critical for this signaling cascade. The sequence and function of porcine TLR4 is probably closer to human than mouse TLR4. Consequently, humans are highly sensitive to LPS, pigs are moderately sensitive to LPS and mice are highly resistant to LPS challenge (Vaure and Liu, 2014). Effects of cytokines are complex and often difficult to interpret. Addition of interleukin 10 (IL-10) in patients with sepsis activated the adaptive immune system by improving T-cell IFN-γ production but diminished the activity of the innate immune system by decreasing TNF-α production as well as surface expression of HLA-DR. Furthermore, in IL-10–treated septic mice an increased IFN-γ production in splenocytes was found (Mazer et al., 2019). In various murine models of sepsis associated with cytokine storm, beneficial effects of interventions aimed at reducing inflammatory mediators were shown, nevertheless the clinical translation failed (Stortz et al., 2017). In porcine models of bacteremia- and peritonitis induced sepsis, differential cytokine profile was found in pigs with and without the acute kidney injury (AKI). Despite similar septic insult and systemic hemodynamic response, only pigs with AKI showed an early increase in the plasma level of TNF-α and IL-6 (Benes et al., 2011). Similarly, in patients with pneumonia AKI was associated with higher plasma levels of both IL6 and TNF-α (Murugan et al., 2010).
Taken together, there are multiple shortcomings of mice as models for studying sepsis and inflammation, and so pigs have emerged as a clinically relevant alternative, which could allow for a better and more reliable clinical translation. Various aspects of porcine immunology and physiology have, however, not yet been analyzed in as much detail as in mice and will require further attention.
5 Porcine model
5.1 General methodological aspects
An important advantage of large (porcine) animal models is that extensive monitoring of vital functions is plausible (Figures 2, 3). A standard experimental setting may include: a central venous catheter for drug and fluid infusion (inserted through the left jugular vein); a balloon-tipped thermodilution pulmonary artery catheter (placed via the right jugular vein); a femoral arterial catheter for blood pressure recording and blood sampling; a fiberoptic catheter for thermal-dye double-indicator dilution measurements; ultrasound transit time flow probes (e.g., around the portal vein, the common hepatic artery, and the left renal artery); catheters in the portal, renal, and hepatic veins; laser Doppler flowmetry for monitoring ileal mucosal and renal cortex microcirculation; and a cystostomy catheter for urine collection (percutaneous insertion under ultrasound guidance). In case hemofiltration techniques are investigated, a dialysis catheter (e.g., 14-French double-lumen) may be inserted into the right femoral vein and serve for hemofiltration access (Stengl et al., 2010b; Jarkovska et al., 2016; Jarkovska et al., 2018; Al-Obeidallah et al., 2021). Such extensive monitoring allows for a determination of the modified SOFA score according to the Third International Consensus definitions of sepsis and septic shock (Singer et al., 2016) with the exclusion of the Glasgow coma scale-based neurologic component due to the use of general anesthesia. In general, using the (modified) SOFA score in (large) animal studies allows for comparison and a better standardization of experimental conditions and results. In our opinion, a wide implementation of the SOFA score into animal sepsis research will facilitate the clinical translation of experimental results.
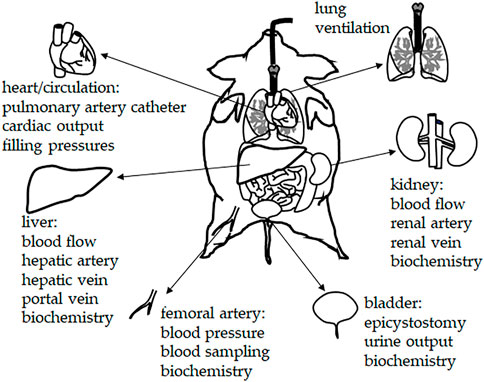
FIGURE 2. Chronic instrumentation and monitoring in porcine sepsis experiments. The experimental setting may include: central venous catheter for drug and fluid infusion (inserted through the left jugular vein); balloon-tipped thermodilution pulmonary artery catheter (placed via the right jugular vein); femoral arterial catheter for blood pressure recording and blood sampling; fiberoptic catheter for thermal-dye double-indicator dilution measurements; ultrasound transit time flow probes (e.g., around the portal vein, the common hepatic artery, and the left renal artery); catheters in the portal, renal, and hepatic veins; laser Doppler flowmetry for monitoring ileal mucosal and renal cortex microcirculation; cystostomy catheter for urine collection (percutaneous insertion under ultrasound guidance).
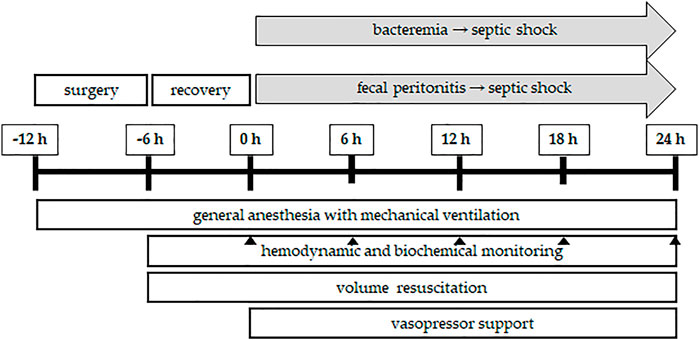
FIGURE 3. Flowchart of porcine sepsis experiments. After induction of general anesthesia and mechanical ventilation, the experiment starts with a surgical preparatory phase, during which access to various vital organ systems is secured and chronic instrumentation devices (see Figure 2) are installed. A postsurgical stabilization period of 6 h is allowed before baseline measurements are obtained (time point: 0 h). Afterwards, sepsis is induced either by bacteremia (continuous central venous infusion of the bacteria of interest, e.g., live P. aeruginosa) or fecal peritonitis (inoculating autologous feces suspended in saline into the abdominal cavity through the drainage tubes). Sepsis progression is followed for 24 h, during which, in a usual setting, irreversible septic shock develops. Throughout the experiment, general anesthesia and mechanical ventilation are maintained with proper volume resuscitation to maintain cardiac filling pressures and administration of vasopressor support, if needed, to maintain the mean blood pressure above 65 mmHg. Continuous hemodynamic monitoring is accompanied by blood and urine sampling for biochemical analyses at the time points of interest (usually 6, 12, 18, and 24 h from the induction of sepsis). At the end of the in vivo experiment, the animals are euthanized by anesthetic overdose and excision of the heart. The total duration of the in vivo porcine sepsis experiment is 36 h and it is followed by in vitro analysis of tissues, cells, and subcellular organelles.
Bacteremia can be induced by continuous central venous infusion of bacteria of interest. In our earlier study, live P. aeruginosa (strain O1, isolated from a patient with suppurative otitis, 1 x 109 colony-forming units/mL determined by serial dilution and colony counts) was used (Stengl et al., 2010a). The infusion rate was titrated throughout the experiment to result in moderate pulmonary hypertension (mean pulmonary artery pressure 35–40 mmHg). Peritonitis can be induced by inoculating autologous feces (0.5–2 g·kg-1, suspended in 200 mL saline) into the abdominal cavity through drainage tubes placed through the abdominal wall (Jarkovska et al., 2016). In our experimental setting, inoculation of high doses of feces (>1 g·kg-1) is usually necessary for reaching the septic shock stage within 24 h. On the other hand, inoculation of low doses of feces (0.5 g·kg-1) usually only results into development of sepsis but not septic shock (Al-Obeidallah et al., 2021). In peritonitis model, a significant variability in the host response is often encountered. As a potential source of this heterogeneity, fecal microbiome was analyzed and interestingly, a significant difference in bacterial composition was associated with the season (winter vs. spring/autumn). It seems, that the seasonal diversity of the microbiome composition could significantly influence outcomes of this experimental model of sepsis (Chalupova et al., 2022).
Regardless of the infectious insult, general anesthesia is maintained throughout the experiment through a combination of continuous intravenous thiopental and fentanyl infusions. Animals are mechanically ventilated and receive appropriate volume resuscitation. Continuous i.v. norepinephrine is administered if needed to maintain a mean blood pressure of 70–75 mmHg.
5.2 Peritonitis vs. bacteremia in a porcine model
With appropriate dosing, both peritonitis and bacteremia can lead to irreversible septic shock within 24 h, with similar general dynamics of sepsis progression (Figure 4A) and significant peripheral vasodilation (Figure 4B). Significant differences, however, developed in terms of the plasma levels of lactate, which were significantly increased in peritonitis but not bacteremia (Figure 4C). In contrast to the traditional view of hyperlactatemia as a product of oxygen debt and anaerobic metabolism, the current interpretation of lactate metabolism is more complex (Brooks, 2020). Hyperlactatemia develops during sepsis and septic shock, probably as a result of the dynamic balance between lactate production and clearance in various tissues and cells (Gibot, 2012). Although, in some studies, impaired lactate clearance was identified (Levraut et al., 1998), lactate overproduction is probably more important. Increased lactate formation was found in the skeletal muscles of patients with septic shock (Levy et al., 2005) as a result of exaggerated aerobic glycolysis through Na+/K + ATPase stimulation. In mild endotoxemia, however, skeletal muscle was unlikely to be a major contributor to increased lactate production (Michaeli et al., 2012). Increased glucose consumption and lactate production mediated through the MEK/ERK signaling cascade was also shown in LPS-activated mouse macrophages, suggesting a tight cross-talk between inflammatory signal transduction and metabolic networks (Través et al., 2012). Obviously, the precise origin of lactate in sepsis may differ in different clinical conditions; it is probably multifactorial and will require further elucidation.
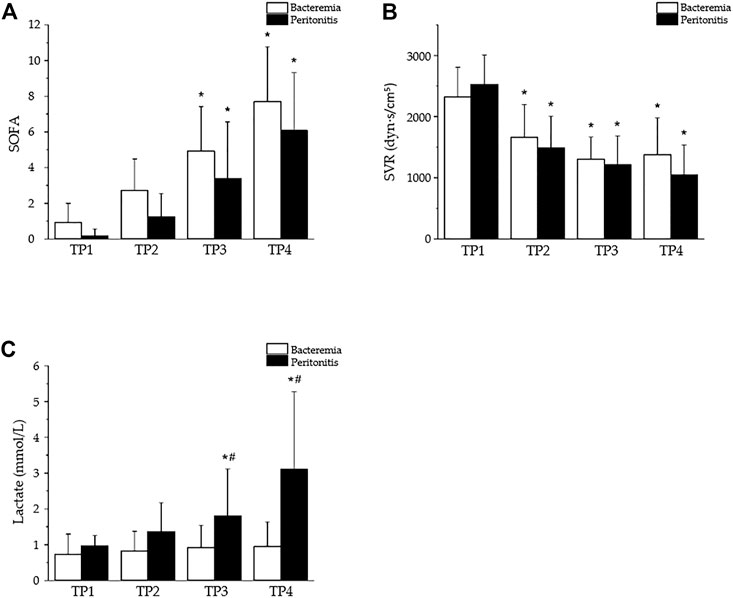
FIGURE 4. Selected parameters of sepsis progression in a porcine model of bacteremia- or peritonitis-induced sepsis. The bacteremia was induced by continuous central venous infusion of live P. aeruginosa (strain O1 isolated from a patient with suppurative otitis, 1 x 109 colony-forming units/mL) (Stengl et al., 2010a); the peritonitis was induced by inoculating autologous feces (0.5–2 g·kg-1, suspended in 200 mL saline) into the abdominal cavity (Jarkovska et al., 2016). Peritonitis, n = 13; bacteremia, n = 14; based on our previous publications (Stengl et al., 2008; 2010a; 2010b). TP1–4: time points 0, 12, 18, and 24 h after sepsis induction. Data are presented as the mean ± SD; *p < 0.05, vs. TP1; #p < 0.05, bacteremia vs. peritonitis. (A) Similar progression of sepsis in pigs with bacteremia and peritonitis documented by rising SOFA scores. (B) Similar hemodynamic alterations in both peritonitis- and bacteremia-induced sepsis documented by reduced systemic vascular resistance (SVR). (C) Plasma levels of lactate as example of differential response in peritonitis- and bacteremia-induced sepsis.
6 Concluding remarks
Three broad categories of experimental models of sepsis include locally induced sepsis (e.g., peritonitis), endotoxemia, and the intravenous administration of a viable pathogen (intermittent or continuous bacteremia). Each model has its strengths and limitations. Intravenous application of single live bacteria (e.g., Pseudomonas, E. coli) is technically simple and reproduces many pathophysiological features of human sepsis. This type of modeling allows for a tightly regulated load of bacterial exposure, and, therefore, severity of insult. Clinically, it replicates conditions such as acute endocarditis or overwhelming infections with Pneumococcus or Meningococcus. In addition, the specific host response to Gram-positive or Gram-negative bacterial challenge can be investigated. On the other hand, the intravenous application of live bacteria leads to a rapid immune-inflammatory and hemodynamic response, usually not seen in sepsis induced by an infectious focus. Hence, more complex, and perhaps more clinically relevant models include locally induced sepsis such as peritonitis. These models of polymicrobial sepsis allow for more protracted immune-inflammatory, metabolic, and hemodynamic alterations to be observed in a clinical setting. Autologous fecal material challenges the animals with its individual gut flora. However, marked intra- and interindividual variations in gut microbial diversity might predispose animals to significant heterogeneity in their host response. Another problem is the difficulty of controlling the quantity of bacteria introduced into the peritoneum.
In a wider context, there is a growing interest in a more tailored approach to sepsis treatment. This approach would require identification of subgroups of patients expressing phenotypic or even endotypic similarity that reflect unique pathophysiology. Indeed, many recent clinical studies described different sepsis phenopytes allowing to identify high-risk patients and those with high probability of responding well to targeted treatments (Seymour et al., 2019; Stanski and Wong, 2020; DeMerle et al., 2021). Unfortunately, to the best of our knowledge, there are no clearly defined large animal model of sepsis phenotypes, in which to explore underlying pathophysiology or to test novel therapeutic targets. Nevertheless, we have recently demonstrated the associations of outcome with clinically relevant phenotypes (Al-Obeidallah et al., 2021). In that study, SOFA score, hemodynamical parameters and body temperature were shown to significantly and early discriminate between sepsis and septic shock in a clinically relevant porcine model. This subgroup of animals nicely corresponds to a group A sub-phenotype characterized by hyperthermia, tachycardia, hypotension and significantly higher odds of mortality as shown in a recent large clinical trial (Bhavani et al., 2022). Our data suggest, that sepsis sub-phenotyping based on vital sign and pattern of organ dysfunction trajectory is feasible even in pre-clinical research of sepsis. Clearly, further studies exploring the correlation between these phenotypes and mechanism-based sepsis endotyping are necessary.
It may be concluded that large animal (porcine) models represent a more clinically relevant alternative to small animal (mouse, rat) models, especially for testing novel therapeutic strategies with not so clear cellular and molecular mechanisms. In a number of studies, porcine models of sepsis have shown a high level of correspondence with clinical situations and human disease progression, thus allowing for the easy and reliable translation of experimental findings to a clinical setting. On the other hand, small animal models, especially transgenic models, allow researchers to obtain mechanistic proof-of-principle insights into the molecular mechanisms of disease in a much faster and more cost-effective way. Obviously, the relationship between small and large models is not competitive but complementary, and the optimal translation should include both steps, with the verification of small animal model findings in large animal models.
Author contributions
Conceptualization, MŠ and MM; Methodology, JH, JC, LV, and LN; Literature search and analysis, PV, MA-O, JH, JC, LV, LN, and DJ; Writing—original draft preparation, PV, MA-O, and MŠ; Writing—review and editing, PV, MA-O, JH, JC, LV, LN, DJ, MM, and MŠ; Supervision and funding acquisition, MM and MŠ. All authors have read and agreed to the submitted version of the manuscript.
Funding
This research was funded by the project no. CZ.02.1.01/0.0/0.0/16_019/0000787 Fighting Infectious Diseases awarded by the MEYS CR, financed from EFRR, by the Ministry of Health of the Czech Republic, grant no. NU20-05-00165, and by the Charles University, the Cooperatio Program, research area IMMU.
Conflict of interest
The authors declare that the research was conducted in the absence of any commercial or financial relationships that could be construed as a potential conflict of interest.
Publisher’s note
All claims expressed in this article are solely those of the authors and do not necessarily represent those of their affiliated organizations, or those of the publisher, the editors and the reviewers. Any product that may be evaluated in this article, or claim that may be made by its manufacturer, is not guaranteed or endorsed by the publisher.
References
Al-Obeidallah M., Jarkovská D., Valešová L., Horák J., Jedlička J., Nalos L., et al. (2021). SOFA score, hemodynamics and body temperature allow early discrimination between porcine peritonitis-induced sepsis and peritonitis-induced septic shock. J. Pers. Med. 11, 164. doi:10.3390/jpm11030164
Alverdy J. C., Keskey R., Thewissen R. (2020). Can the cecal ligation and puncture model Be repurposed to better inform therapy in human sepsis? Infect. Immun. 88, 009422-19. doi:10.1128/IAI.00942-19
Bailey J. D., Diotallevi M., Nicol T., McNeill E., Shaw A., Chuaiphichai S., et al. (2019). Nitric oxide modulates metabolic remodeling in inflammatory macrophages through TCA cycle regulation and itaconate accumulation. Cell. Rep. 28, 218–230. e7. doi:10.1016/j.celrep.2019.06.018
Balls M. (2022). Alternatives to laboratory animals: Trends in replacement and the three rs. Altern. Lab. Anim. 50, 10–26. doi:10.1177/02611929221082250
Basas J., Palau M., Ratia C., Del Pozo J. L., Martín-Gómez M. T., Gomis X., et al. (2018). High-dose daptomycin is effective as an antibiotic lock therapy in a rabbit model of Staphylococcus epidermidis catheter-related infection. Antimicrob. Agents Chemother. 62, 017777-17. doi:10.1128/AAC.01777-17
Benes J., Chvojka J., Sykora R., Radej J., Krouzecky A., Novak I., et al. (2011). Searching for mechanisms that matter in early septic acute kidney injury: An experimental study. Crit. Care 15, R256. doi:10.1186/cc10517
Bergman E. N. (1990). Energy contributions of volatile fatty acids from the gastrointestinal tract in various species. Physiol. Rev. 70, 567–590. doi:10.1152/physrev.1990.70.2.567
Bhavani S. V., Semler M., Qian E. T., Verhoef P. A., Robichaux C., Churpek M. M., et al. (2022). Development and validation of novel sepsis subphenotypes using trajectories of vital signs. Intensive Care Med. 48, 1582–1592. doi:10.1007/s00134-022-06890-z
Brook B., Harbeson D., Amenyogbe N., Ben-Othman R., Kollmann T. R., Aniba R. (2019). Robust health-score based survival prediction for a neonatal mouse model of polymicrobial sepsis. PLoS One 14, e0218714. doi:10.1371/journal.pone.0218714
Brooks G. A. (2020). The tortuous path of lactate shuttle discovery: From cinders and boards to the lab and ICU. J. Sport Health Sci. 9, 446–460. doi:10.1016/j.jshs.2020.02.006
Cai S., Paudel S., Jin L., Ghimire L., Taylor C. M., Wakamatsu N., et al. (2021). NLRP6 modulates neutrophil homeostasis in bacterial pneumonia-derived sepsis. Mucosal Immunol. 14, 574–584. doi:10.1038/s41385-020-00357-4
Cavaillon J. M. (2018). Exotoxins and endotoxins: Inducers of inflammatory cytokines. Toxicon 149, 45–53. doi:10.1016/j.toxicon.2017.10.016
Cazorla O., Freiburg A., Helmes M., Centner T., McNabb M., Wu Y., et al. (2000). Differential expression of cardiac titin isoforms and modulation of cellular stiffness. Circ. Res. 86, 59–67. doi:10.1161/01.res.86.1.59
Chalupova M., Horak J., Kramna L., Nalos L., Stengl M., Chudejova K., et al. (2022). Gut microbiome diversity of porcine peritonitis model of sepsis. Sci. Rep. 12, 17430. doi:10.1038/s41598-022-21079-6
Chung C. S., Bogomolovas J., Gasch A., Hidalgo C. G., Labeit S., Granzier H. L. (2011). Titin-actin interaction: PEVK-actin-based viscosity in a large animal. J. Biomed. Biotechnol. 2011, 310791. doi:10.1155/2011/310791
Coutinho M. L., Matsunaga J., Wang L.-C., de la Peña Moctezuma A., Lewis M. S., Babbitt J. T., et al. (2014). Kinetics of leptospira interrogans infection in hamsters after intradermal and subcutaneous challenge. PLoS Negl. Trop. Dis. 8, e3307. doi:10.1371/journal.pntd.0003307
Dawson H. D., Lunney J. K. (2018). Porcine cluster of differentiation (CD) markers 2018 update. Res. Vet. Sci. 118, 199–246. doi:10.1016/j.rvsc.2018.02.007
DeMerle K. M., Angus D. C., Baillie J. K., Brant E., Calfee C. S., Carcillo J., et al. (2021). Sepsis subclasses: A framework for development and interpretation. Crit. Care Med. 49, 748–759. doi:10.1097/CCM.0000000000004842
Durosier L. D., Herry C. L., Cortes M., Cao M., Burns P., Desrochers A., et al. (2015). Does heart rate variability reflect the systemic inflammatory response in a fetal sheep model of lipopolysaccharide-induced sepsis? Physiol. Meas. 36, 2089–2102. doi:10.1088/0967-3334/36/10/2089
Fairbairn L., Kapetanovic R., Sester D. P., Hume D. A. (2011). The mononuclear phagocyte system of the pig as a model for understanding human innate immunity and disease. J. Leukoc. Biol. 89, 855–871. doi:10.1189/jlb.1110607
Fink M. P., Heard S. O. (1990). Laboratory models of sepsis and septic shock. J. Surg. Res. 49, 186–196. doi:10.1016/0022-4804(90)90260-9
Fleischmann C., Scherag A., Adhikari N. K. J., Hartog C. S., Tsaganos T., Schlattmann P., et al. (2016). Assessment of global incidence and mortality of hospital-treated sepsis. Current estimates and limitations. Am. J. Respir. Crit. Care Med. 193, 259–272. doi:10.1164/rccm.201504-0781OC
Ghanta S., Kwon M.-Y., Perrella M. A. (2021). Induction of sepsis via fibrin clot implantation. Methods Mol. Biol. 2321, 17–25. doi:10.1007/978-1-0716-1488-4_3
Granfeldt A., Letson H. L., Dobson G. P., Shi W., Vinten-Johansen J., Tønnesen E. (2014). Adenosine, lidocaine and Mg2+ improves cardiac and pulmonary function, induces reversible hypotension and exerts anti-inflammatory effects in an endotoxemic porcine model. Crit. Care 18, 682. doi:10.1186/s13054-014-0682-y
Guillon A., Preau S., Aboab J., Azabou E., Jung B., Silva S., et al. (2019). Preclinical septic shock research: Why we need an animal ICU. Ann. Intensive Care 9, 66. doi:10.1186/s13613-019-0543-6
Haake D. A. (2006). Hamster model of leptospirosis. Curr. Protoc. Microbiol. Chapter 12, Unit 12E.2, Chapter 12, Unit 12E.2. doi:10.1002/9780471729259.mc12e02s02
Hua T., Wu X., Wang W., Li H., Bradley J., Peberdy M. A., et al. (2018). Micro- and macrocirculatory changes during sepsis and septic shock in a rat model. Shock 49, 591–595. doi:10.1097/SHK.0000000000000954
Jarkovska D., Markova M., Horak J., Nalos L., Benes J., Al-Obeidallah M., et al. (2018). Cellular mechanisms of myocardial depression in porcine septic shock. Front. Physiol. 9, 726. doi:10.3389/fphys.2018.00726
Jarkovska D., Valesova L., Chvojka J., Benes J., Sviglerova J., Florova B., et al. (2016). Heart rate variability in porcine progressive peritonitis-induced sepsis. Front. Physiol. 6, 412. doi:10.3389/fphys.2015.00412
Jin Y., Wei L., Cai W., Lin Z., Wu Z., Peng Y., et al. (2017). Complete characterization of cardiac myosin heavy chain (223 kDa) enabled by size-exclusion chromatography and middle-down mass spectrometry. Anal. Chem. 89, 4922–4930. doi:10.1021/acs.analchem.7b00113
Judges D., Sharkey P., Cheung H., Craig I., Driedger A. A., Sibbald W. J., et al. (1986). Pulmonary microvascular fluid flux in a large animal model of sepsis: Evidence for increased pulmonary endothelial permeability accompanying surgically induced peritonitis in sheep. Surgery 99, 222–234.,
Kapetanovic R., Fairbairn L., Beraldi D., Sester D. P., Archibald A. L., Tuggle C. K., et al. (2012). Pig bone marrow-derived macrophages resemble human macrophages in their response to bacterial lipopolysaccharide. J. Immunol. 188, 3382–3394. doi:10.4049/jimmunol.1102649
Kastello M. D., Spertzel R. O. (1973). The rhesus monkey as a model for the study of infectious disease. Am. J. Phys. Anthropol. 38, 501–504. doi:10.1002/ajpa.1330380255
Kumar V. (2020). Toll-like receptors in sepsis-associated cytokine storm and their endogenous negative regulators as future immunomodulatory targets. Int. Immunopharmacol. 89, 107087. doi:10.1016/j.intimp.2020.107087
Kuypers E., Ophelders D., Jellema R. K., Kunzmann S., Gavilanes A. W., Kramer B. W. (2012). White matter injury following fetal inflammatory response syndrome induced by chorioamnionitis and fetal sepsis: Lessons from experimental ovine models. Early Hum. Dev. 88, 931–936. doi:10.1016/j.earlhumdev.2012.09.011
Lagoa C. E., de Figueiredo L. F. P., Cruz R. J., Silva E., Rocha e Silva M. (2004). Effects of volume resuscitation on splanchnic perfusion in canine model of severe sepsis induced by live Escherichia coli infusion. Crit. Care 8, R221–R228. doi:10.1186/cc2871
Levraut J., Ciebiera J. P., Chave S., Rabary O., Jambou P., Carles M., et al. (1998). Mild hyperlactatemia in stable septic patients is due to impaired lactate clearance rather than overproduction. Am. J. Respir. Crit. Care Med. 157, 1021–1026. doi:10.1164/ajrccm.157.4.9705037
Levy B., Gibot S., Franck P., Cravoisy A., Bollaert P.-E. (2005). Relation between muscle Na+K+ ATPase activity and raised lactate concentrations in septic shock: A prospective study. Lancet 365, 871–875. doi:10.1016/S0140-6736(05)71045-X
Levy M. M., Fink M. P., Marshall J. C., Abraham E., Angus D., Cook D., et al. (2003). 2001 SCCM/ESICM/ACCP/ATS/SIS international sepsis definitions conference. Intensive Care Med. 29, 530–538. doi:10.1007/s00134-003-1662-x
Li G.-R., Du X.-L., Siow Y. L., O K., Tse H.-F., Lau C.-P. (2003). Calcium-activated transient outward chloride current and phase 1 repolarization of swine ventricular action potential. Cardiovasc. Res. 58, 89–98. doi:10.1016/s0008-6363(02)00859-3
Li J.-L., Li G., Jing X.-Z., Li Y.-F., Ye Q.-Y., Jia H.-H., et al. (2018). Assessment of clinical sepsis-associated biomarkers in a septic mouse model. J. Int. Med. Res. 46, 2410–2422. doi:10.1177/0300060518764717
Liu V., Escobar G. J., Greene J. D., Soule J., Whippy A., Angus D. C., et al. (2014). Hospital deaths in patients with sepsis from 2 independent cohorts. JAMA 312, 90–92. doi:10.1001/jama.2014.5804
Locher M. R., Razumova M. V., Stelzer J. E., Norman H. S., Moss R. L. (2011). Effects of low-level α-myosin heavy chain expression on contractile kinetics in porcine myocardium. Am. J. Physiol. Heart Circ. Physiol. 300, H869–H878. doi:10.1152/ajpheart.00452.2010
Lu W.-H., Jin X.-J., Jiang X.-G., Wang Z., Wu J.-Y., Shen G.-G. (2015). Resuscitation with hydroxyethyl starch 130/0.4 attenuates intestinal injury in a rabbit model of sepsis. Indian J. Pharmacol. 47, 49–54. doi:10.4103/0253-7613.150333
Makarenko I., Opitz C. A., Leake M. C., Neagoe C., Kulke M., Gwathmey J. K., et al. (2004). Passive stiffness changes caused by upregulation of compliant titin isoforms in human dilated cardiomyopathy hearts. Circ. Res. 95, 708–716. doi:10.1161/01.RES.0000143901.37063.2f
Mazer M., Unsinger J., Drewry A., Walton A., Osborne D., Blood T., et al. (2019). IL-10 has differential effects on the innate and adaptive immune systems of septic patients. J. Immunol. 203, 2088–2099. doi:10.4049/jimmunol.1900637
Mellhammar L., Wullt S., Lindberg Å., Lanbeck P., Christensson B., Linder A. (2016). Sepsis incidence: A population-based study. Open Forum Infect. Dis. 3, ofw207. doi:10.1093/ofid/ofw207
Meurens F., Summerfield A., Nauwynck H., Saif L., Gerdts V. (2012). The pig: A model for human infectious diseases. Trends Microbiol. 20, 50–57. doi:10.1016/j.tim.2011.11.002
Michaeli B., Martinez A., Revelly J.-P., Cayeux M.-C., Chioléro R. L., Tappy L., et al. (2012). Effects of endotoxin on lactate metabolism in humans. Crit. Care 16, R139. doi:10.1186/cc11444
Milani-Nejad N., Janssen P. M. L. (2014). Small and large animal models in cardiac contraction research: Advantages and disadvantages. Pharmacol. Ther. 141, 235–249. doi:10.1016/j.pharmthera.2013.10.007
Minneci P. C., Deans K. J., Hansen B., Parent C., Romines C., Gonzales D. A., et al. (2007). A canine model of septic shock: Balancing animal welfare and scientific relevance. Am. J. Physiol. Heart Circ. Physiol. 293, H2487–H2500. doi:10.1152/ajpheart.00589.2007
Miranda M. L., Balarini M. M., Bouskela E. (2015). Dexmedetomidine attenuates the microcirculatory derangements evoked by experimental sepsis. Anesthesiology 122, 619–630. doi:10.1097/ALN.0000000000000491
Miyata S., Minobe W., Bristow M. R., Leinwand L. A. (2000). Myosin heavy chain isoform expression in the failing and nonfailing human heart. Circ. Res. 86, 386–390. doi:10.1161/01.res.86.4.386
Morimatsu H., Ishikawa K., May C. N., Bailey M., Bellomo R. (2012). The systemic and regional hemodynamic effects of phenylephrine in sheep under normal conditions and during early hyperdynamic sepsis. Anesth. Analg. 115, 330–342. doi:10.1213/ANE.0b013e31825681ab
Murakami K., Bjertnaes L. J., Schmalstieg F. C., McGuire R., Cox R. A., Hawkins H. K., et al. (2002). A novel animal model of sepsis after acute lung injury in sheep. Crit. Care Med. 30, 2083–2090. doi:10.1097/00003246-200209000-00022
Murugan R., Karajala-Subramanyam V., Lee M., Yende S., Kong L., Carter M., et al. (2010). Acute kidney injury in non-severe pneumonia is associated with an increased immune response and lower survival. Kidney Int. 77, 527–535. doi:10.1038/ki.2009.502
Mutlak H., Jennewein C., Tran N., Mehring M., Latsch K., Habeck K., et al. (2013). Cecum ligation and dissection: A novel modified mouse sepsis model. J. Surg. Res. 183, 321–329. doi:10.1016/j.jss.2012.11.046
Nagy S., Tárnoky K., Tutsek L., Boros M., Karácsony G. (1990). A canine model of hyperdynamic sepsis induced by intestinal ischemia. Acta Physiol. Hung 75, 303–320.
Napolitano L. M. (2018). Sepsis 2018: Definitions and guideline changes. Surg. Infect. (Larchmt) 19, 117–125. doi:10.1089/sur.2017.278
Natanson C., Danner R. L., Fink M. P., MacVittie T. J., Walker R. I., Conklin J. J., et al. (1988). Cardiovascular performance with E. Coli challenges in a canine model of human sepsis. Am. J. Physiol. 254, H558–H569. doi:10.1152/ajpheart.1988.254.3.H558
Odening K. E., Gomez A.-M., Dobrev D., Fabritz L., Heinzel F. R., Mangoni M. E., et al. (2021). ESC working group on cardiac cellular electrophysiology position paper: Relevance, opportunities, and limitations of experimental models for cardiac electrophysiology research. Europace 23, 1795–1814. doi:10.1093/europace/euab142
Okazaki N., Lankadeva Y. R., Peiris R. M., Birchall I. E., May C. N. (2021). Rapid and persistent decrease in brain tissue oxygenation in ovine gram-negative sepsis. Am. J. Physiol. Regul. Integr. Comp. Physiol. 321, R990–R996. –R996. doi:10.1152/ajpregu.00184.2021
Pabst R. (2020). The pig as a model for immunology research. Cell. Tissue Res. 380, 287–304. doi:10.1007/s00441-020-03206-9
Park I., Lee J. H., Jang D.-H., Kim D., Chang H., Kwon H., et al. (2019). Characterization of fecal peritonitis-induced sepsis in a porcine model. J. Surg. Res. 244, 492–501. doi:10.1016/j.jss.2019.06.094
Raith E. P., Udy A. A., Bailey M., McGloughlin S., MacIsaac C., Bellomo R., et al. (2017). Prognostic accuracy of the SOFA score, SIRS criteria, and qSOFA score for in-hospital mortality among adults with suspected infection admitted to the intensive care unit. JAMA 317, 290–300. doi:10.1001/jama.2016.20328
Reade M. C., Young J. D. (2003). Of mice and men (and rats): Implications of species and stimulus differences for the interpretation of studies of nitric oxide in sepsis. Br. J. Anaesth. 90, 115–118. doi:10.1093/bja/aeg033
Reiser P. J., Portman M. A., Ning X. H., Schomisch Moravec C. (2001). Human cardiac myosin heavy chain isoforms in fetal and failing adult atria and ventricles. Am. J. Physiol. Heart Circ. Physiol. 280, H1814–H1820. doi:10.1152/ajpheart.2001.280.4.H1814
Rothkötter H.-J. (2009). Anatomical particularities of the porcine immune system--a physician’s view. Dev. Comp. Immunol. 33, 267–272. doi:10.1016/j.dci.2008.06.016
Rundell V. L. M., Manaves V., Martin A. F., de Tombe P. P. (2005). Impact of beta-myosin heavy chain isoform expression on cross-bridge cycling kinetics. Am. J. Physiol. Heart Circ. Physiol. 288, H896–H903. doi:10.1152/ajpheart.00407.2004
Santos A. O. M. T., Furtado E. S., Villela N. R., Bouskela E. (2011). Microcirculatory effects of fluid therapy and dopamine, associated or not to fluid therapy, in endotoxemic hamsters. Clin. Hemorheol. Microcirc. 47, 1–13. doi:10.3233/CH-2010-1358
Scheiermann P., Hoegl S., Revermann M., Ahluwalia D., Zander J., Boost K. A., et al. (2009). Cecal ligation and incision: An acute onset model of severe sepsis in rats. J. Surg. Res. 151, 132–137. doi:10.1016/j.jss.2008.02.032
Seok J., Warren H. S., Cuenca A. G., Mindrinos M. N., Baker H. V., Xu W., et al. (2013). Genomic responses in mouse models poorly mimic human inflammatory diseases. Proc. Natl. Acad. Sci. U. S. A. 110, 3507–3512. doi:10.1073/pnas.1222878110
Seymour C. W., Kennedy J. N., Wang S., Chang C. H., Elliott C. F., Xu Z., et al. (2019). Derivation, validation, and potential treatment implications of novel clinical phenotypes for sepsis. JAMA 321, 2003–2017. –17. doi:10.1001/jama.2019.5791
Siddiqui A. Z., Bhatti U. F., Deng Q., Biesterveld B. E., Tian Y., Wu Z., et al. (2021). Cl-amidine improves survival and attenuates kidney injury in a rabbit model of endotoxic shock. Surg. Infect. (Larchmt) 22, 421–426. doi:10.1089/sur.2020.189
Singer M., Deutschman C. S., Seymour C. W., Shankar-Hari M., Annane D., Bauer M., et al. (2016). The Third international consensus definitions for sepsis and septic shock (Sepsis-3). JAMA 315, 801–810. doi:10.1001/jama.2016.0287
Solomon M. A., Correa R., Alexander H. R., Koev L. A., Cobb J. P., Kim D. K., et al. (1994). Myocardial energy metabolism and morphology in a canine model of sepsis. Am. J. Physiol. 266, H757–H768. doi:10.1152/ajpheart.1994.266.2.H757
Stanski N. L., Wong H. R. (2020). Prognostic and predictive enrichment in sepsis. Nat. Rev. Nephrol. 16, 20–31. doi:10.1038/s41581-019-0199-3
Stelzer J. E., Norman H. S., Chen P. P., Patel J. R., Moss R. L. (2008). Transmural variation in myosin heavy chain isoform expression modulates the timing of myocardial force generation in porcine left ventricle. J. Physiol. 586, 5203–5214. doi:10.1113/jphysiol.2008.160390
Stengl M., Bartak F., Sykora R., Chvojka J., Benes J., Krouzecky A., et al. (2010a). Reduced L-type calcium current in ventricular myocytes from pigs with hyperdynamic septic shock. Crit. Care Med. 38, 579–587. doi:10.1097/CCM.0b013e3181cb0f61
Stengl M., Sykora R., Chvojka J., Krouzecky A., Novak I., Varnerova V., et al. (2010b). Differential effects of hemofiltration and of coupled plasma filtration adsorption on cardiac repolarization in pigs with hyperdynamic septic shock. Shock 33, 101–105. doi:10.1097/SHK.0b013e3181ab6359
Stengl M., Sykora R., Krouzecky A., Chvojka J., Novak I., Varnerova V., et al. (2008). Continuous hemofiltration in pigs with hyperdynamic septic shock affects cardiac repolarization. Crit. Care Med. 36, 3198–3204. doi:10.1097/CCM.0b013e31818f9eda
Stortz J. A., Raymond S. L., Mira J. C., Moldawer L. L., Mohr A. M., Efron P. A. (2017). Murine models of sepsis and trauma: Can we bridge the gap? ILAR J. 58, 90–105. doi:10.1093/ilar/ilx007
Swindle M. M., Makin A., Herron A. J., Clubb F. J., Frazier K. S. (2012). Swine as models in biomedical research and toxicology testing. Vet. Pathol. 49, 344–356. doi:10.1177/0300985811402846
Tao W., Deyo D. J., Traber D. L., Johnston W. E., Sherwood E. R. (2004). Hemodynamic and cardiac contractile function during sepsis caused by cecal ligation and puncture in mice. Shock 21, 31–37. doi:10.1097/01.shk.0000101673.49265.5d
Tarkowski A., Collins L. V., Gjertsson I., Hultgren O. H., Jonsson I. M., Sakiniene E., et al. (2001). Model systems: Modeling human staphylococcal arthritis and sepsis in the mouse. Trends Microbiol. 9, 321–326. doi:10.1016/s0966-842x(01)02078-9
Torio C. M., Moore B. J. (2006). “National inpatient hospital costs: The most expensive conditions by payer, 2013,” in Healthcare Cost and utilization project (HCUP) statistical briefs (rockville (MD): Agency for healthcare research and quality (US)). Available at: http://www.ncbi.nlm.nih.gov/books/NBK368492/(Accessed August 5, 2022).
Traeger T., Koerner P., Kessler W., Cziupka K., Diedrich S., Busemann A., et al. (2010). Colon ascendens stent peritonitis (CASP)--a standardized model for polymicrobial abdominal sepsis. J. Vis. Exp. 2299, 2299. doi:10.3791/2299
Través P. G., de Atauri P., Marín S., Pimentel-Santillana M., Rodríguez-Prados J.-C., Marín de Mas I., et al. (2012). Relevance of the MEK/ERK signaling pathway in the metabolism of activated macrophages: A metabolomic approach. J. Immunol. 188, 1402–1410. doi:10.4049/jimmunol.1101781
Uranga-Murillo I., Tapia E., Garzón-Tituaña M., Ramirez-Labrada A., Santiago L., Pesini C., et al. (2021). Biological relevance of granzymes A and K during E. Coli sepsis. Theranostics 11, 9873–9883. doi:10.7150/thno.59418
Utiger J. M., Glas M., Levis A., Prazak J., Haenggi M. (2021). Description of a rat model of polymicrobial abdominal sepsis mimicking human colon perforation. BMC Res. Notes 14, 14. doi:10.1186/s13104-020-05438-y
Vaure C., Liu Y. (2014). A comparative review of toll-like receptor 4 expression and functionality in different animal species. Front. Immunol. 5, 316. doi:10.3389/fimmu.2014.00316
Vogel M., Cheung M. M. H., Li J., Kristiansen S. B., Schmidt M. R., White P. A., et al. (2003). Noninvasive assessment of left ventricular force-frequency relationships using tissue Doppler-derived isovolumic acceleration: Validation in an animal model. Circulation 107, 1647–1652. doi:10.1161/01.CIR.0000058171.62847.90
Wang J., Liu X., Ren B., Rupp H., Takeda N., Dhalla N. S. (2002). Modification of myosin gene expression by imidapril in failing heart due to myocardial infarction. J. Mol. Cell. Cardiol. 34, 847–857. doi:10.1006/jmcc.2002.2023
Wang N., Lu Y., Zheng J., Liu X. (2022). Of mice and men: Laboratory murine models for recapitulating the immunosuppression of human sepsis. Front. Immunol. 13, 956448. doi:10.3389/fimmu.2022.956448
Warren H. S., Fitting C., Hoff E., Adib-Conquy M., Beasley-Topliffe L., Tesini B., et al. (2010). Resilience to bacterial infection: Difference between species could be due to proteins in serum. J. Infect. Dis. 201, 223–232. doi:10.1086/649557
Whang K. T., Vath S. D., Becker K. L., Snider R. H., Nylen E. S., Muller B., et al. (2000). Procalcitonin and proinflammatory cytokine interactions in sepsis. Shock 14, 73–78. doi:10.1097/00024382-200014010-00013
Wolffram S., Eggenberger E., Scharrer E. (1986). Kinetics of D-glucose and L-leucine transport into sheep and pig intestinal brush border membrane vesicles. Comp. Biochem. Physiol. A Comp. Physiol. 84, 589–593. doi:10.1016/0300-9629(86)90370-1
Yaghouby F., Daluwatte C., Fukuda S., Nelson C., Salsbury J., Kinsky M., et al. (2017). Progression and variability of physiologic deterioration in an ovine model of lung infection sepsis. J. Appl. Physiol. 123, 172–181. doi:10.1152/japplphysiol.00122.2017
Zanetti G., Heumann D., Gérain J., Kohler J., Abbet P., Barras C., et al. (1992). Cytokine production after intravenous or peritoneal gram-negative bacterial challenge in mice. Comparative protective efficacy of antibodies to tumor necrosis factor-alpha and to lipopolysaccharide. J. Immunol. 148, 1890–1897.
Keywords: sepsis, large animal models, pig, peritonitis, bacteremia, SOFA score, cardiovascular system, immune system
Citation: Vintrych P, Al-Obeidallah M, Horák J, Chvojka J, Valešová L, Nalos L, Jarkovská D, Matějovič M and Štengl M (2023) Modeling sepsis, with a special focus on large animal models of porcine peritonitis and bacteremia. Front. Physiol. 13:1094199. doi: 10.3389/fphys.2022.1094199
Received: 09 November 2022; Accepted: 28 December 2022;
Published: 10 January 2023.
Edited by:
Chi-Wen Lung, Asia University, TaiwanReviewed by:
Fahni Haris, Muhammadiyah University of Yogyakarta, IndonesiaWei-Cheng Shen, Asia University, Taiwan
Wen-Tzu Wu, Asia University, Taiwan
Copyright © 2023 Vintrych, Al-Obeidallah, Horák, Chvojka, Valešová, Nalos, Jarkovská, Matějovič and Štengl. This is an open-access article distributed under the terms of the Creative Commons Attribution License (CC BY). The use, distribution or reproduction in other forums is permitted, provided the original author(s) and the copyright owner(s) are credited and that the original publication in this journal is cited, in accordance with accepted academic practice. No use, distribution or reproduction is permitted which does not comply with these terms.
*Correspondence: Milan Štengl, bWlsYW4uc3RlbmdsQGxmcC5jdW5pLmN6