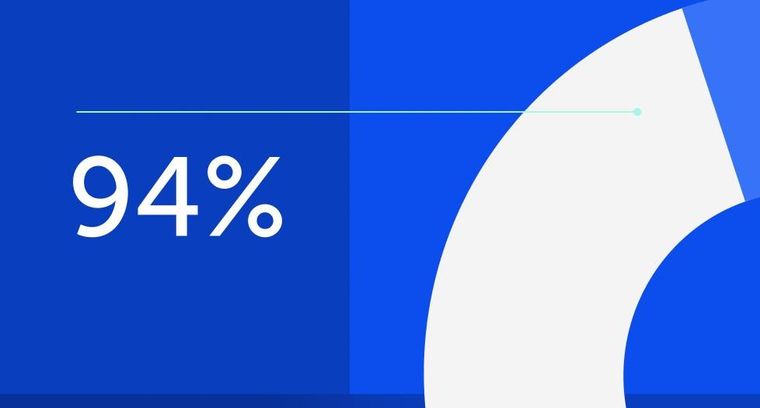
94% of researchers rate our articles as excellent or good
Learn more about the work of our research integrity team to safeguard the quality of each article we publish.
Find out more
ORIGINAL RESEARCH article
Front. Physiol., 05 January 2023
Sec. Aquatic Physiology
Volume 13 - 2022 | https://doi.org/10.3389/fphys.2022.1089164
Dissolved oxygen (DO) and water temperature vary in coastal environments. In tropical regions, the ability of aquatic ectotherms to cope with hypoxia and high-temperature interactive effects is fundamental for their survival. The mechanisms underlying both hypoxia and thermal tolerance are known to be interconnected, therefore, the idea of cross-tolerance between both environmental stressors has been put forward. We investigated the combined role of hypoxia and temperature changes on the physiological responses of blue crab Callinectes sapidus living in the southern Gulf of Mexico. We measured oxygen consumption, plasmatic biochemical indicators, total hemocyte count (THC), and antioxidant activity biomarkers in muscle and gill tissues of blue crab acclimated to moderate hypoxia or normoxia and exposed to a thermal fluctuation or a constant temperature, the former including a temperature beyond the optimum range. Animals recovered their routine metabolic rate (RMR) after experiencing thermal stress in normoxia, reflecting physiological plasticity to temperature changes. In hypoxia, the effect of increasing temperature was modulated as reflected in the RMR and plasmatic biochemical indicators concentration, and the THC did not suggest significant alterations in the health status. In both DO, the antioxidant defense system was active against oxidative (OX) damage to lipids and proteins. However, hypoxia was associated with an increase in the amelioration of OX damage. These results show that C. sapidus can modulate its thermal response in a stringent dependency with DO, supporting the idea of local acclimatization to tropical conditions, and providing insights into its potential as invasive species.
Hypoxia can originate in marine and freshwater environments, and its occurrence is constrained by hydrological processes, climate, and human activities (Zillén et al., 2008; Levin et al., 2009; Zhang et al., 2010). Episodic hypoxia can occur in estuarine and brackish shallow environments on a diel basis (diel-cycling hypoxia) since nighttime metabolic rates exceed oxygen resupply from the atmosphere (Smith and Able, 2003; Diaz and Rosenberg, 2008; Andersen et al., 2017; Borowiec et al., 2018). In shallow coastal areas, short-term hypoxia is provoked by upwelling events following seasonal patterns, and also by anthropogenic nutrient loading related to freshwater influx (Grantham et al., 2004).
Most benthic species living in fluctuating dissolved oxygen (DO) regimes possess different degrees of regulatory capacity (McMahon, 2001). In crustaceans, the primary response to hypoxia is increasing ventilation of the branchial chambers to maintain their metabolic rate—often measured as oxygen consumption rate (Cech and Brauner, 2011)—up to a critical oxygen level (Pc), that is, the concentration beneath which metabolic rate becomes oxygen dependent (Seibel et al., 2021). Below the Pc other mechanisms operate in response to hypoxic stress. Some of these responses include increasing hemocyanin concentration to improve hemolymph oxygen carrying capacity as seen in Cancer magister (Head, 2010), hypoxia-induced bradycardia to reduce overall cardiac work as reported in the grass shrimp Palaemonetes pugio (Guadagnoli et al., 2007; 2011), and metabolic rate depression (Sokolova et al., 2012). Overall, this suite of physiological adjustments aims at minimizing the disruption on the balance between energy supply and demand, defined as energy metabolism, until the organisms reach a lethal range of DO (Sokolova et al., 2012).
Based on the influence of temperature on energy metabolism, the oxygen-and capacity-limited thermal tolerance (OCLTT) hypothesis (Pörtner, 2002; 2010; Pörtner et al., 2006) highlights the interdependence of hypoxic and thermal stress responses. Organismal thermal tolerance limits are reached at temperatures where the cardiorespiratory system fails to meet tissue metabolic demands (Pörtner and Farrell, 2008). In the optimum temperature range, the aerobic capacity covers the energy demands of maintenance and major functions. In moderate thermal stress (the pejus range), the aerobic capacity is still positive but diminished due to an elevated routine metabolic rate (RMR)—the average metabolic rate of a postabsorptive ectotherm with free movement at a defined temperature—and the acclimation in protection capacity triggers key role mechanisms to maintain homeostasis (Pörtner, 2010; Sokolova et al., 2012). When thermal stress is extreme (the pessimum range), survival becomes time dependent, which implies a functional association between tolerance to temperature and hypoxia at all biological levels.
The effects of chronic hypoxia and thermal stress in crustaceans have been evaluated on physiological and biochemical traits. Previous studies have demonstrated that crustaceans may exploit glycolysis for energy production resulting in lactate accumulation (Aparicio-Simón et al., 2018), while an increment in reactive oxygen species (ROS) production is expected at mitochondrial level as a by-product of higher cell respiration when temperature increases (Romero et al., 2013) or DO decreases (Trasviña-Arenas et al., 2013). In gills, the activities of antioxidant enzymes increase after hypoxia exposure, returning to normal levels in normoxia (e.g., L. vannamei (García-Triana et al., 2010; Trasviña-Arenas et al., 2013)); there is evidence that elevated levels of antioxidant defense enzymes during hypoxia might occur as an adaptive strategy in fluctuating environments in preparation for oxidative stress during reoxygenation (Hermes-Lima et al., 2015). Furthermore, common pollution biomarkers, including the activities of cholinesterases and carboxylesterases, might provide insights on physiological condition at given temperature or DO regime. For instance, some authors report evident seasonal fluctuation in acetylcholinesterase (AChE) (e.g., Menezes et al., 2006), a key enzyme in cholinergic signal transmission in the sensory and neuromuscular systems that therefore appears to be likely related to animals’ locomotor activity (Anita et al., 2017). Moreover, carboxylesterases (CbE), a multifunctional group of enzymes relevant in lipid synthesis and decomposition and signal transmembrane transduction (Zang et al., 2020), are involved in the metabolism of endogenous compounds (Nos et al., 2021), and thermal stress can compromise their capability to face environmental chemical stressors (Saiz et al., 2022).
In terms of adaptation, the relationship between the mechanisms underlying thermal and hypoxia tolerance put forward the idea that acclimation to one stressor might enhance tolerance to another one (i.e. there is cross-tolerance) (Burleson and Silva, 2011; McBryan et al., 2016). Moreover, the ability of aquatic ectotherms to tolerate simultaneously hypoxia and elevated temperatures—likely to occur under climate change scenarios—together with all niche elements determine ecosystem stability (Masson-Delmotte et al., 2019). Nevertheless, scarce information exists about whether hypoxic acclimation improves thermal performance in crustaceans, and vice versa.
Among the biologically and commercially important decapod crustaceans, blue crab Callinectes sapidus—a keystone species (Glaspie et al., 2020)—has one of the broadest latitudinal native distribution in coastlines and adjacent wetlands on the western Atlantic where some of the species distribution spots coincide with hypoxic zones (Rabalais et al., 2007; Johnson, 2015; Seitz, 2020). The physiology of blue crab in tropical conditions remains relatively unexplored (Ángeles-González et al., 2020). However, García-Rueda et al. (2021) reported lower and upper thermal window limits of 11°C and 42°C, respectively; the highest thermal metabolic scope (TMS, a proxy for the aerobic window) at 32°C, and the highest hypoxia tolerance from 29°C to 34°C with a 50% reduction in RMR at ∼20% air saturation. Nonetheless, considering that blue crab in tropical conditions are close to their upper thermal limits, in warming scenarios where higher temperatures and more severe hypoxia are likely to occur (Glenn et al., 2015; Murray-Tortarolo, 2021), they are thought to be susceptible to future strong oxygen-temperature interactions.
We conducted lab-based experiments to evaluate the responses of oxygen consumption and biochemical indicators of blue crab from the southern Gulf of Mexico after being acclimated to different DO and thermal regimes to provide information about the interactive effects of both environmental stressors in animals from the tropics. For this purpose, we implemented two different thermal regimes (i.e. fluctuating and constant) under long-term moderate hypoxia and normoxia to test the hypothesis of cross-tolerance on routine metabolism.
Animal ethics approval was not required for the use of blue crab in research in Mexico, in accordance with national guidelines. However, for all experiments and animal husbandry, we used established criteria for good management practices for aquatic animals based on the norms of conduct of Universidad Nacional Autónoma de México (UNAM) and Centro de Investigación Científica y de Educación Superior de Ensenada.
Adult blue crab (n = 97; mean mass ± standard deviation = 189.35 ± 36.85 g) were collected from wild population close to Sisal, Yucatán, México (21 09’ 55’’ N and 90 01’ 50” W), using artisanal fishing traps. Females bearing eggs and individuals with conspicuous external lesions were excluded. Organisms were transported to the Laboratory of Applied Ecophysiology (Faculty of Sciences) at UNAM, Sisal, Yucatan. Blue crab were subjected to a conditioning period for 2 weeks to ensure that all had a similar recent thermal history (Vinagre et al., 2016) and allow them to fully recover from capture and handling in 3000 L tanks (maximum 30 organisms per tank) filled with flow-through natural filtered (20 µm) seawater (35–37 PSU) at 26°C ± 1°C and ≥80% air saturation, under a 12 h:12 h light/dark photoperiod. Organisms were fed small pieces of squid or fish twice a day, and feces and food residues were removed daily from the cages and tanks with a siphon. At the end of the laboratory conditioning period, epibionts from the crab carapace and abdomen were removed.
After the laboratory conditioning period, blue crab were transferred to Multi-stressor units (Aquabiotech INC, Coaticook, Quebec, Canada) that allow the control for several environmental variables. Animals were randomly distributed in the “habitat racks” and subjected to four acclimation conditions resulting from the combination of DO and thermal regime. The first DO was normoxia at ≥80% air saturation, and the second one was moderate hypoxia at 45%–50% air saturation. Thermal acclimation was either fluctuant or constant. Therefore, the resulting four acclimation conditions were: 1) thermal fluctuation in normoxia; 2) thermal fluctuation in moderate hypoxia; 3) constant temperature in normoxia; and 4) constant temperature in moderate hypoxia. The thermal fluctuation consisted of three consecutive 10-day (d) sections, each one followed by a sampling day: 1) initial section at 29°C (sampling at 10d); 2) high-temperature section at 34°C (sampling at 24d); and 3) return section at 29°C (sampling at 38d) (Figure 1A). The constant temperature lasted for 30d at 29°C (Figure 1B).
FIGURE 1. Schematic representation of the experimental design for the laboratory conditioning period and acclimation of Callinectes sapidus. (A) Fluctuating condition with an initial section at 29°C, followed by a high-temperature section at 34°C, and a return section at 29°C. (B) Constant condition at 29°C. Both thermal regimes were implemented in normoxia (≥80% air saturation) and moderated hypoxia (45%–50% air saturation). Black vertical arrows indicate the sampling time for respiratory and biochemical variables.
The required temperature changes were made at a rate of ∼1°C d−1 to reduce the risk of inducing additional thermal stress. Salinity, feeding, individualization cages cleaning, and photoperiod were maintained as described above for the laboratory conditioning period. Because seawater circulating through the Multi-stressor systems is partially recycled, we monitored nutrients (total ammonia, nitrite, and nitrate) periodically to verify that they were maintained under the safe range for the species. A group of blue crab was randomly selected at each sampling time to perform the measurements, and animals were fasted for 24 h to ensure they were in a post-absorptive condition.
Oxygen consumption rate was individually measured using flow-through respirometry, a method that allows to measure oxygen change from a controlled, continuous flow of water through the respirometer. This feature is especially convenient to maintain water inflow with a stable dissolved oxygen (DO) in the respirometric chambers during measurements in hypoxia. All the measurements were done in a semi-dark environment and in the absence of external stimuli. To record DO data, respirometric chambers were connected to a pair (entrance and exit) of flow-through oxygen sensors that were coupled by a fiber cable to a Witrox 4 instrument (Loligo Systems, Viborg, Denmark). Before measurements, all sensors were calibrated for each experimental temperature, using air saturated seawater and an oxygen-free solution with sodium sulfite (0.1 g in 10 ml−1 of seawater; 0% OD); the seawater flowrate in each chamber was standardized using a flowmeter. For measurements in hypoxia, once the system reached the testing temperature, DO was reduced using gaseous nitrogen injection directly in the seawater reservoir to 45%–50% air saturation, and animals were placed into the respirometric chambers only when experimental conditions were stable.
Respirometric trials lasted 36 h. An empty chamber (without organism) was used as control to estimate and correct background respiration in each trial. Blue crab were weighed at the end of the experiment, and oxygen consumption was calculated using the following equation:
Where
Plasmatic biochemical indicators, total hemocyte count (THC), and oxidative stress indicators were quantified from each experimental treatment after RMR measurements. Plasmatic biochemical indicators included total proteins (PRO), cholesterol (CHO), acylglycerides (ACY), glucose (GLU) and lactate (LAC). Antioxidant defense system (ADS) markers–catalase (CAT), glutathione S-transferase (GST), superoxide dismutase (SOD), and total glutathione levels (T.GSH)—and physiological condition biomarkers–activities of acetylcholinesterase (AChE) and carboxylesterase (CbE) –, as well as oxidative indicators (OX)—protein carbonylation (PO) and lipid peroxidation (LPO) –, were analyzed in muscle and gill tissue.
We obtained blue crab hemolymph from the swimming leg coxa with a precooled disposable insulin syringe impregnated internally with anticoagulant solution SIC-EDTA (450 mM NaCl; 10 mM KCl; 10 mM HEPES; 10 mM EDTA). A sample of 10 µl of hemolymph from each organism was processed immediately after extraction to determine the THC using TC10 counting slides with dual chambers. Readings were performed with a TC10™ automated cell counter (Bio-Rad INC, Hercules, California, United States). For plasmatic biochemical indicators quantification, 100 µl of hemolymph from each organism was homogenized with SIC-EDTA (1:2 ratio) in individual Eppendorf tubes and refrigerated until analysis within the next two hours. The samples were centrifuged (800 g, 4°C for 5 min, Bio-Rad INC, Hercules, California, United States) to obtain plasma as supernatant. CHO, ACY, GLU, and LAC were quantified by colorimetric analysis in microplate using 10 µl of plasma and 200 µl of enzyme chromogen reagent in commercial kits (ELITech Group, Puteaux, Paris, France) following the manufacturer’s instructions. PRO were determined following the Bradford method (1976). Concentration (mg ml−1) was estimated using the absorbance value of each sample, values obtained from the linear regression analysis of the standard absorbance curve (intercept and slope), and the value of the dilution factor:
Blue crab were sacrificed immediately after taking hemolymph samples. After that, a portion of locomotor muscle and gill tissue were separated on ice, snap-frozen in liquid nitrogen, and then stored at −80°C until further analysis. Samples were homogenized with TRIS 0.05 M pH 7.4 at 100 mg tissue ml−1 using a Potter-Elvehjem homogenizer. The sample homogenate was divided in Eppendorf tubes, each containing enough volume for duplicate assays of AChE (Ellman et al., 1961 adapted by Rodríguez-Fuentes et al., 2008), CbE (Hosokawa and Satoh, 2002), CAT (Góth, 1991 modified by Hadwan and Abed, 2016), GST (Habig and Jakoby, 1981), SOD (Sigma-Aldrich assay kit 19160), PO (Mesquita et al., 2014), LPO (Sigma-Aldrich PeroxiDetect Kit), and T.GSH (Sigma-Aldrich Glutathione Assay Kit CS0260). Prior quantifications of AChE, CbE, SOD, CAT, and GST, muscle and gill homogenates were centrifuged (10,000 g, 4°C for 5 min, Bio-Rad INC, Hercules, California, United States) and the supernatant was separated for analysis. Proteins were analyzed in the supernatant following Bradford (1976) to standardize all enzyme activities in activity unit (U) mg−1 protein.
We used two fixed explanatory factors. The first factor was dissolved oxygen (DO) with two levels: normoxia and hypoxia. The second factor was sampling time, which represented the combination of thermal regime (fluctuant or constant) and time, consisting of four different levels: 1) 10d (i.e., 10d at 29°C); 2) 24d (i.e., 10d at 29°C + 10d at 34°C); and 3) 38d (i.e., 10d at 29°C + 10d at 34°C + 10 days at 29°C) along the thermal fluctuation and 4) constant temperature at 29°C lasting 30d (Figure 1).
We analyzed the differences between routine metabolic rate (RMR) using a Linear Mixed-effects Model and tested the significance of including replicates in the experimental setup as a random factor and variance structure that accounts for changes in error-variance due to one or both fixed factors using the likelihood ratio and the Akaike Information Criterion (AIC). We visually inspected model standardized residuals (i.e., residuals versus fitted values and quantile-quantile plots) for outliers, normality, and variance homogeneity, and we applied pairwise post hoc comparisons between sampling times for each DO based on the model estimated parameters.
We performed a two-way ANOVA to assess the effects of DO and sampling time on total hemocyte count (THC). Here again, residuals were visually inspected. We also performed normality (Shapiro-Wilk test) and variance homogeneity (Levene’s test) tests on the model residuals. A Fisher Least Significant Difference (LSD) post hoc test was conducted.
The response of energetic and stress indicators was evaluated separately for 1) plasmatic biochemical indicators concentrations, 2) antioxidant defense system (ADS) and oxidative (OX) damage biomarkers, and 3) physiological condition biomarkers. All variables were Log(x+1) transformed and normalized (centered on their means) previous to analysis. A permutational analysis of variance (PERMANOVA) was used to test the hypothesis of statistical differences between experimental groups. The homogeneity of the multivariate dispersion in the Euclidean space was previously tested with a multivariate analogue to Levene’s test (PERMDISP), using distances to centroids. Similarity percentages (SIMPER) analysis was also used to determine which dependent variables contributed most to the observed pattern of separation between experimental groups. All procedures were based on 9999 permutations under the reduced models (Anderson, 2006). Plasmatic biochemical indicators concentrations and ADS and OX damage biomarkers were visualized by means of a Principal Coordinate Analysis (PCoA) applied on the resemble matrixes of Euclidean distances. Furthermore, linear models using Generalized Least Squares (GLS) were used to analyze the patterns of univariate responses. We performed a procedure through restricted maximum likelihood to test different weights or within-group heteroscedasticity structure terms (allow errors to have unequal variances), and we selected the most informative model based on visual inspection of Pearson’s residuals and AIC (Montgomery and Peck, 1992; Zuur et al., 2007). We conducted two-way ANOVA analyses and Tukey post hoc comparisons for statistically significant ANOVA results.
We conducted statistical analyses using R Studio and PRIMER 7. R package nlme (Phineiro et al., 2022) was used to adjust Linear Mixed-effects Model and GLS models; package vegan (Oksanen et al., 2022) was used to conduct PCoA analyses; and ggplot2 (Wickham, 2016) was used to generate graphic visualizations. Data are shown as mean value ± standard deviation.
The most informative model included the random factor associated to the replication of the acclimation conditions (AIC = −360, L. Ratio = 15.11, p < 0.001 compared to the reduced model without the random factor), a variance structure accounting for changes in error-variance between regimes (AIC = −362, L. Ratio = 8.90, p < 0.05 compared to the reduced model without the variance structure), and the interaction between dissolved oxygen (DO) and sampling time (AIC = −423, L. Ratio = 11.75, p < 0.01 compared to the reduced model lacking the interaction term). Our results showed that the thermal fluctuation provoked changes across sampling times in the RMR of blue crab acclimated to normoxia (Figure 2). The highest RMR was observed in blue crab after the first 10d at 29°C, followed by progressive reductions after they experienced 34°C (24d, p < 0.01) and then returned to 29°C (38d, p < 0.001). Similar values were reached at the end of the thermal fluctuation compared to the constant temperature (0.11 ± 0.03 mgO2 g−1 h−1 and 0.11 ± 0.02 mgO2 g−1 h−1, respectively; p = 0.223). In blue crab acclimated to hypoxia, the RMR did not change across sampling times in the thermal fluctuation (Figure 2; p > 0.05 for all comparisons), and no significant differences were observed in the RMR of blue crab maintained in constant temperature (0.1 ± 0.02 mgO2 g−1 h−1) compared to those evaluated at each sampling time during fluctuation (p > 0.05 for all comparisons) (Figure 2).
FIGURE 2. Routine metabolic rate (RMR) of Callinectes sapidus acclimated to a thermal fluctuation or a constant temperature in normoxia (≥80% air saturation) and moderate hypoxia (∼45% air saturation). Sampling times along the fluctuation are 10d at 29°C (n = 7 in normoxia and 11 in hypoxia), 24d at 34°C (n = 11 in normoxia and 12 in hypoxia), and 38d at 29°C (n = 18 in normoxia and 15 in hypoxia). The constant temperature lasted for 30d (n = 11 in normoxia and 12 in hypoxia). The asterisks symbols refer to statistically significant difference at p ≤ 0.05.
TCH was significantly affected by DO (ANOVA: F-value = 4.4; p < 0.05). Sampling time and its interaction with DO were unsignificant (F-value = 2.1; p = 0.122, and F-value = 1.3; p = 0.284, respectively). However, significant differences were only detected for the first 10 d in the thermal fluctuation (p < 0.05) (Supplementary Table S1).
The interaction between dissolved oxygen (DO) and sampling time was significant (Table 1). This result indicates that the alteration in plasmatic biochemical indicators caused by temperature changes depended on DO. This is supported by differential distribution of sampling times centroids by DO in the PCoA for the distance matrix (Figure 3A). Cholesterol (CHO) and lactate (LAC) were the most correlated variables with PCO1 and PCO2, respectively, being the most influential ones in groups ordination (Figure 3B). Pairwise multivariate comparisons among sampling times at each DO were significant for all contrasts only in normoxia (Table 2), and the SIMPER analysis revealed that peaks in LAC, glucose (GLU) and lipids (acylglycerides (ACY) and CHO) levels at 10d, 24d and 38d in the fluctuation, respectively, provoked these differences (Supplementary Figure S1, Table 2).
TABLE 1. Permutational analysis of variance (PERMANOVA) of the effect of DO and sampling time on blue crab. A = plasmatic biochemical indicators, B = ADS and OX damage biomarkers in muscle, C = ADS and OX damage biomarkers in gill, D = physiological condition biomarkers in muscle, and E = physiological condition biomarkers in gill. The probabilities associated at each F-ratio were obtained with 9999 permutations of residuals under a reduced model.
FIGURE 3. Plasmatic biochemical indicators in blue crab hemolymph. (A) Principal coordinate analysis (PCoA) of centroids and individual points per DO (N = normoxia; H = hypoxia) and sampling time (10d, 24d, and 38d in the thermal fluctuation, and the constant temperature). The lines follow the centroid trajectories (solid line = normoxia; dashed line = hypoxia) along the fluctuation. DO and sampling time combinations are referred as N10d (n = 6), N24d (n = 4), N38d (n = 6), NCons (n = 6), H10d (n = 4), H24d (n = 5), H38d (n = 9), and HCons (n = 5). (B) Biplot of biochemical indicators. Vectors in red and Pearson correlation values correspond to the most correlated variables with PCO1 and PCO2. LAC = lactate, GLU = glucose, PRO = total proteins, CHO = cholesterol, ACY = acylglycerides.
TABLE 2. Results of pair-wise comparisons among sampling times at each DO based on the Euclidean distance matrix. Results of the SIMPER analysis are included for significant comparisons. A = plasmatic biochemical indicators, B = ADS and OX damage biomarkers in muscle, C = ADS and OX damage biomarkers in gill, D = physiological condition biomarkers in muscle, E = physiological condition biomarkers in gill, Var. = variable, and %Cont. = contribution of individual variables to the overall Euclidean dissimilarity (cut-off of 70%). The probabilities associated at each F-ratio were obtained with 9999 permutations of residuals under a reduced model.
No statistical differences were detected in terms of multivariate dispersion of the groups data (combinations of DO and sampling time) from the centroids (PERMDISP, p = 0.066). Therefore, the dispersion effect is not driving the existing differences among groups in normoxia nor the lack of differences among them in hypoxia.
A significant effect of sampling time and DO on the antioxidant defense system (ADS) and oxidative (OX) damage in muscle of blue crab was observed, while the interaction between both factors was not significant (Table 1). Therefore, temperature modulated the ADS and the OX damage in muscle of blue crab acclimated to normoxia and hypoxia following a similar pattern (Figure 4A). Superoxide dismutase (SOD) and protein carbonylation (PO) were the most correlated variables with PCO1 and PCO2, respectively, being the most influential ones in groups ordination (Figure 4C). Significant pairwise comparisons among sampling times occurred only between 10d and the rest of sampling times in normoxia, which is associated to higher levels of catalase (CAT) and SOD, and lower levels of PO according to the SIMPER analysis (Supplementary Figures S2, S3, Table 2). However, significant differences in dispersion were observed in terms of multivariate dispersion of the groups data (combinations of DO and sampling time; PERMDISP, p = 0.024), indicating that the lack of differences between sampling times was associated with the variability in concentrations.
FIGURE 4. Antioxidant defense system and oxidative damage indicators in tissues. Principal coordinate analysis (PCoA) of centroids and individual points per DO (N = normoxia; H = hypoxia) and sampling time (10d, 24d, and 38d in the thermal fluctuation, and the constant temperature) for muscle (A) and gill (B). The lines follow the centroid trajectories (solid line = normoxia; dashed line = hypoxia) along the fluctuation. DO and sampling time combinations are referred as N10d (n = 6), N24d (n = 4), N38d (n = 6), NCons (n = 6), H10d (n = 4), H24d (n = 5), H38d (n = 9), and HCons (n = 5). Biplot of biochemical indicators for muscle (C) and gills (D). Vectors in red and Pearson correlation values correspond to the most correlated variables with PCO1 and PCO2. LPO = lipid peroxidation, PO = protein carbonylation, CAT = catalase, SOD = superoxide dismutase, T.GSH = total glutathione, and GST = glutathione S-transferase.
Differences in the activity of carboxylesterase (CbE) and acetylcholinesterase (AChE) in muscle of blue crab were attributed to sampling time, DO, and the interaction between both factors (Table 1). Significant pairwise comparisons among sampling times were found only in normoxia between 38d and the rest of sampling times, as well as between 24d and the constant temperature (Table 2). The SIMPER analysis revealed that lower and higher levels of AChE at 38d and 24d, respectively, were responsible for these differences (Supplementary Figure S4, Table 2).
No statistical differences were detected in the physiological condition indicators data (PERMDISP, p = 0.145). Therefore, the dispersion effect is not driving the existing differences among groups in normoxia nor the lack of differences among them in hypoxia.
A significant effect of sampling time, DO, and the interaction between these factors was observed on the ADS and the OX damage in gill of blue crab (Table 1). This result indicates that the alteration in these indicators caused by temperature changes depended on DO (Figure 4B). SOD and glutathione S-transferase (GST) were the most correlated variables with PCO1, while LPO was the most correlated variable with PCO2, being the most influential ones in groups ordination (Figure 4D). The PCoA of centroids for the distance matrix showed that the differences among sampling times increased in normoxia compared to hypoxia, particularly between 24d (34°C) and 38d (29°C) in the thermal fluctuation (Figure 4B). Pairwise test showed that statistically significant differences existed only between 38d (29°C) and the rest of sampling times in normoxia due to higher levels of PO and lower levels of lipid peroxidation (LPO) and CAT (Supplementary Figures S2, S3, Table 2).
No statistical differences were detected in terms of multivariate dispersion of the groups data (combinations of DO and sampling time) from the centroids for ADS and OX damage in gill (PERMDISP, p = 0.113). Therefore, the dispersion effect is not driving the existing differences among groups in normoxia nor the lack of differences among them in hypoxia.
Statistical differences were found between the activity of CbE and AChE in gills of blue crab of different sampling times and DO, while the interaction term was not significant (Table 1). Significant pairwise comparisons among sampling times were detected between 10d and 24d in hypoxia. In normoxia significant differences occurred between 10d and 38d, as well as between 38d and the constant temperature (Table 2). The SIMPER analysis showed that higher levels of CbE at 10d contributed drove the differences in both DO, whereas lower levels of AChE at 38d were responsible for the differences only in normoxia (Supplemenetary Figure S4, Table 2). However, significant differences in dispersion were observed in the physiological condition indicators data (PERMDISP, p = 0.028), indicating that the lack of differences between sampling times was associated with the variability in concentrations.
The results in this study provide a novel insight into the energetic and stress response at organismal and cellular levels in blue crab in tropical conditions subjected to the interaction of two key environmental variables: dissolved oxygen (DO) and temperature.
DO modulated the temperature effect on energy demands of blue crab. In normoxia, changes in temperature provoked alterations in the routine metabolic rate (RMR), whereas chronic moderate hypoxia reduced oxygen delivery, neutralizing the passive effects of temperature fluctuation on RMR. Reducing metabolic rate may allow the blue crab to conserve energy and extend their survival time under hypoxia (Hochachka and Somero, 2002; King et al., 2022).
Despite the alterations in RMR under normoxia along the thermal fluctuation, blue crab had the ability to recover their physiological condition after experiencing a pejus temperature (34°C; García-Rueda et al., 2021). Animals that returned to 29°C showed a similar RMR to those maintained at constant 29°C. This result agrees with Jayasundara and Somero (2013), who found that the longjaw mudsucker Gillichthys mirabilis showed metabolic rate recovery when temperature returned to a value within the preferred range after experiencing suboptimum high temperatures, reflecting physiological plasticity to temperature changes.
The idea that the reduced oxygen delivery to tissues helped blue crab to cope with thermal stress under hypoxia is supported by attenuation in shifts in dominant hemolymph fuel molecules in hypoxia. Crustaceans use glycogen, proteins, and several lipids as the main metabolic fuels, which are transported to tissues in hemolymph and respond to seasonal changes in temperature (Vinagre et al., 2007; Buckup et al., 2008; Pellegrino et al., 2008). In animals acclimated to normoxia, a hyperglycemic response was observed when they were exposed to 34°C in the thermal fluctuation, indicating that glycogenolysis (glycogen catabolism) was turned ON to release glucose (GLU) that presumably satisfied the metabolic requirements provoked by high temperature. Similar results were obtained in many crustacean species (e.g., Vasudevan and Rajendran, 2021), in which the glycogenolysis pathway is the first mechanism to fuel the physiological process involved to maintain homeostasis under stress conditions. Moreover, significantly higher lipid levels were detected when blue crab returned to 29°C at the end of the thermal fluctuation, which suggested that lipolysis (triglycerides catabolism) was used as a second source of energy after the glycogen-glucose pathway. The primary metabolic reserve in most crustaceans are acylglycerides (ACY), mainly triglycerides (Alava et al., 2007), while and cholesterol (CHO) is a key structural component in cell membrane structure and it serves as a precursor of physiological components including sex and molting hormones (Wouters et al., 2001; Kumar et al., 2018). Therefore, a possible hypothesis is that the peaks of these lipids in crab hemolymph were due to metabolic debt, membrane repair mechanism, and molting processes triggered by 34°C exposure and still evident at the end of the thermal fluctuation (38d), even when exposed to an optimum temperature value (29°C). In the constant temperature, the dominant plasmatic biochemical indicator were total proteins (PRO), reflecting an adequate nutritional and physiological status that can be inferred considering the dependence of many crustaceans on PRO as an energetic substrate (Rosas et al., 2001a; Rosas et al., 2001b). Previous studies in several shrimp species found that PRO concentrations in hemolymph function as organic reserves, serving as the basis of glycogen synthesis, the main glucose reserve (Rosas et al., 2002).
In wetlands and coastal lagoons along the northern portion of the Yucatan Peninsula, DO can temporarily reach <2 mgO2 L−1, in combination with elevated temperatures around 35°C (Herrera-Silveira et al., 2004). In adjacent marine coastal waters, DO are above 4.5 mgO2 L−1 and temperature oscillates between 24°C and 28°C at 4 m deep (Enriquez et al., 2013; Okolodkov et al., 2014). For that reason, the regulation of major fuel molecules concentration in hemolymph and metabolic pathways might reflect the ability of blue crab in tropical conditions to maintain energy expenditure across environmental gradients when they alternate between coastal waters and their adjacent ecosystems for reproduction purposes (Epifanio, 2019).
The ability of blue crab to tolerate environmental gradients may be further supported by the results obtained for total hemocyte count (THC). In this study, THC did not change significantly between DO at high temperature or across temperatures for the same DO in the thermal fluctuation. These results are different from those of Xie et al. (2021) who found that diel-cycling hypoxia can both lead to the THC reduction in the oyster Crassostrea hongkongensis and reported it as evidence that hypoxia may impair health of this species.
Overall, the antioxidant defense system (ADS) was active in muscles and gills of the blue crab exposed to both (fluctuating and constant) thermal regimes to contend oxidative (OX) damage in lipids and proteins in hypoxia and normoxia. The differences detected in the patterns observed in each tissue can be explained by different functions and location within the body that make them more or less exposed to the environment (Vinagre et al., 2014).
Neither did normoxia nor hypoxia combined with high temperature significantly alter the stress response in muscle and gills compared to the constant temperature, which can be considered as the baseline scenario for each DO and tissue. This result agrees with previous findings about blue crab being well adapted to tolerate—at least temporarily—34°C, which is considered a pejus temperature (García-Rueda et al., 2021). The detection of some lipid peroxidation (LPO) and protein carbonylation (PO) levels in the constant temperature may seem counterintuitive with the idea of an optimum environmental regime. However, as with the concentration of free radicals in cells, low LPO and PO levels can be beneficial in cellular signaling because of their role in key processes that can improve physiological performance, like apoptosis (Wong et al., 2010; Dhabhar, 2014).
Some interesting trends were found in the OX damage indicators and the ADS enzymes in muscles of blue crab at the end of the thermal fluctuation. In animals acclimated to normoxia, LPO was neutralized when they returned to 38d (29°C), but PO was still detected at this final sampling time. PO is considered a not chemically-reversible (Dalle-Donne et al., 2006) reactive oxygen species (ROS) effect; thus, it could have accumulated through time (in organisms that returned to 29°C it doubled the value measured when they were exposed to 34°C). However, the lack of significant differences in the stress response between organisms at the end of the thermal fluctuation and those maintained at constant 29°C reflects, once again, the physiological plasticity of blue crab for environmental temperature. In this scenario, hypoxia was associated to an increase in ADS activity compared to normoxia; crabs acclimated to hypoxia presented higher levels of superoxide dismutase (SOD), the most influential variable in PCO1. This result agrees with previous findings that demonstrated how crustaceans generally maintain a high basal level of the ADS enzymes during hypoxia (Hermes-Lima and Zenteno-Savín, 2002; Welker et al., 2013). Actually, this fact is supported by the hypothesis that the increase in levels and activities of the antioxidant components during periods of hypoxia occurs in “preparation for oxidative stress” to prevent increased ROS levels during reoxygenation (Hermes-Lima et al., 2015).
In the gills of blue crab acclimated to normoxia a delayed effect of thermal stress (34°C at 24d) on OX damage associated with ROS production was observed. As in muscles, after experiencing thermal stress and decreasing temperature back to 29°C at 38d in the thermal fluctuation, the ADS was also able to neutralize LPO, but it failed to contend PO. However, the gills, significant differences were found between the stress response in blue crab that returned to 29°C in the thermal fluctuation and the rest of sampling times, including the constant temperature. The generation of ROS is presumed to be high in the gill in response to oxygen flux and diffusion because of respiration, acid-base balance, and osmotic and ionic regulation that occur in this organ (Paital and Chainy, 2010). Moreover, the gill provides the first line of antioxidant defense due to direct exposure to environmental stressors (Maciel et al., 2004), which supports a pronounced effect of thermal stress that should be contended by the ADS to maintain the functionality of this key organ. Under hypoxia, the effect of temperature was mitigated because of an increase in ADS, as previously described for muscles.
Whilst hypoxia is commonly associated with a burst in ROS (Welker et al., 2013) the increase would occur during the first hours of exposure to hypoxia and a prolonged exposure time may allow the remaining O2 to continue being partially reduced by complex II and III (Hermes-Lima et al., 2015). This situation could be the case of blue crab, considering that organisms were subjected to moderate hypoxia for weeks, which has been observed in other decapod species like the crab Neohelice granulate. After 10 h of hypoxia, LPO was successfully controlled thanks to the activity of the antioxidant defense system present in tissue (Geihs et al., 2014).
Carboxylesterase (CbE) and acetylcholinesterase (AChE) concentrations in muscle and gills provide more insight about the effects of fluctuating temperature between optimum and pejus thermal conditions for blue crab in tropical environments. CbEs are responsible for hydrolysis of a broad range of endogenous esters and play a significant role during molting (Homola and Chang, 1997). AChE is measured as a neurotoxicity biomarker (Antó et al., 2009). In muscle, when blue crab were exposed to 34°C under normoxia, thermal stress provoked an increment in AChE compared to the constant temperature, followed by a drastic decrease when blue crab returned to 29°C. Conversely, CbE levels were maintained relatively stable. Since AChE is involved in the synapse between nerve cells and muscle cells and it appears to be likely related to animals’ locomotor activity (Anita et al., 2017), higher levels at 34°C suggest an increase in neurotransmission in muscle during the escape response, which was reduced after going back to an optimum temperature of 29°C. Moderate hypoxia seemed to modulate this thermal effect. This result supports the findings for the ADS and OX damage as chronic exposure to hypoxia mitigated the effects of temperature change in muscle tissue. In the gills of blue crab acclimated to normoxia and hypoxia, differences among sampling times were mainly due to CbE, which was lower at high temperature indicating that membrane lipids in the gill could be altered by thermal stress. In contrast, AChE was maintained relatively stable among sampling times at both DO, suggesting that the nervous system linked with the gill function was maintained independently of the thermal and DO regime. However, it is worth mentioning that more detailed studies should be done in attempt to broaden the knowledge about the relationship between those enzymes, temperature, DO, and metabolism in blue crab.
Overall, it has been shown that temperatures that promote high values of aerobic scope (i.e., thermal metabolic scope) are associated with optimal thermal environments. Within the optimum, it has been postulated that reductions in DO decrease the energy available to meet the energy demands of routine metabolism, impairing the performance of ectotherms. In this conceptual framework, hypoxia reduces the thermal window as a consequence of the limitations posed by low DO on the cardiorespiratory system’s capacity to sustain the elevated cost of maintenance, threatening homeostasis, especially outside the optimal range (Pörtner, 2010; Pórtner et al., 2017; Sokolova et al., 2012). Contrary to this proposal, the results obtained in the present study show that hypoxia, far from reducing the performance of crabs, has a beneficial effect, motivating adaptive responses which allow a reduction in the production of free radicals, preparation of the ADS for possible re-oxygenation, and stability of the nervous system. To improve the OCLTT hypothesis and its relationship with hypoxia, it might be necessary to consider that a decrease in the metabolic rate associated with oxygen reduction does not necessarily provoke adverse effects on the life of ectotherm species like C. sapidus that possess the physiological mechanisms necessary to perform adequately in conditions that could be perceived as limiting.
Given the results, we conclude that in blue crab in tropical conditions, a cross-tolerance exists between temperature and DO. Moderate hypoxia attenuated the effects of high temperature on the RMR and metabolic pathways. Moreover, in blue crab exposed to temperature changes through the thermal fluctuation, when they return to an optimum temperature after experiencing thermal stress in moderate hypoxia, the degree of OX damage in muscles and gills is reduced. This general pattern attributed to DO is associated with the reduction in oxygen delivery provoked by low oxygen availability. In such circumstances, OX stress is reduced due to the role of the ADS and the decrease in ROS production associated to prolonged exposure to hypoxia.
The effect of DO on thermal tolerance of aquatic ectotherms in the literature is currently mixed (Brijs et al., 2015; Verberk et al., 2016; Jung et al., 2020).We hypothesize that in blue crab from the Yucatan Peninsula, the reduction of oxygen delivery, the modulation of energetic pathways derived from lower energy demands, and the increase in ADS activity under moderate and chronic hypoxia act as mechanisms to cope with temperature increases. This reflects the local acclimatization of blue crab to the environmental conditions that characterizes the ecological niche in the tropics. Moreover, these findings provide insights on how blue crab contend the constraints posed by abiotic variables that might be their physiological filters in aquatic environments as an invasive species.
The original contributions presented in the study are included in the Supplementary Material, further inquiries can be directed to the corresponding author.
Conceptualization and investigation: AG-R, FD, KP, and CR; Methodology: AG-R and CC-M; Data curation and formal analysis: AG-R and MM; Writing—original draft: AG-R; Writing—review and editing: AG-R, CR, MM, and GR-F; Project administration: CR and CC-M.
This study was partially financed by PAPIIT-UNAM program (IN203022) given to CR and the CONACYT project No. 61503. A doctoral scholarship from the Consejo Nacional de Ciencia y Tecnologia (CONACYT) was awarded to AG-R (number CVU: 746562).
This research is the result of a doctoral degree thesis being carried out in Posgrado en Ciencias del Mar y Limnologia at Universidad Nacional Autonoma de Mexico (UNAM). The authors are grateful for the support granted to student AG-R by CONACYT.
The authors declare that the research was conducted in the absence of any commercial or financial relationships that could be construed as a potential conflict of interest.
All claims expressed in this article are solely those of the authors and do not necessarily represent those of their affiliated organizations, or those of the publisher, the editors and the reviewers. Any product that may be evaluated in this article, or claim that may be made by its manufacturer, is not guaranteed or endorsed by the publisher.
The Supplementary Material for this article can be found online at: https://www.frontiersin.org/articles/10.3389/fphys.2022.1089164/full#supplementary-material
Alava V. R., Quinitio E. T., De Pedro J. B., Orosco Z. G. A., Wille M. (2007). Reproductive performance, lipids and fatty acids of mud crab Scylla serrata (Forsskål) fed dietary lipid levels. Aqua. Res. 38, 1442–1451. doi:10.1111/j.1365-2109.2007.01722.x
Andersen M. R., Kragh T., Sand-Jensen K. (2017). Extreme diel dissolved oxygen and carbon cycles in shallow vegetated lakes. Proc. R. Soc. B Biol. Sci. 284, 20171427. doi:10.1098/rspb.2017.1427
Anderson M. J. (2006). Distance-based tests for homogeneity of multivariate dispersions. Biometrics 62, 245–253. doi:10.1111/J.1541-0420.2005.00440.X
Ángeles-González L., Martínez-Meyer E., Yañez-Arenas C., Velázquez-Abunader I., Garcia-Rueda A., Díaz F., et al. (2020). Using realized thermal niche to validate thermal preferences from laboratory studies. How do they stand? Ecol. Indic. 118, 106741. doi:10.1016/j.ecolind.2020.106741
Anita J., David Š., Simona P., Žiga F., Primož Z. (2017). Comparative study of acetylcholinesterase and glutathione S-transferase activities of closely related cave and surface asellus aquaticus (isopoda: Crustacea). PLoS One 12, e0176746. doi:10.1371/JOURNAL.PONE.0176746
Antó M., Arnau S., Buti E., Cortijo V., Gutiérrez E., Solé M. (2009). Characterisation of integrated stress biomarkers in two deep-sea crustaceans, Aristeus antennatus and Nephrops norvegicus, from the NW fishing grounds of the Mediterranean sea. Ecotoxicol. Environ. Saf. 72, 1455–1462. doi:10.1016/J.ECOENV.2009.02.007
Aparicio-Simón B., Piñón M., Racotta R., Racotta I. S. (2018). Neuroendocrine and metabolic responses of Pacific whiteleg shrimp Penaeus vannamei exposed to hypoxia stress. Lat. Am. J. Aquat. Res. 46, 364–376. doi:10.3856/vol46-issue2-fulltext-12
Borowiec B. G., McClelland G. B., Rees B. B., Scott G. R. (2018). Distinct metabolic adjustments arise from acclimation to constant hypoxia and intermittent hypoxia in estuarine killifish (Fundulus heteroclitus). J. Exp. Biol. 221, jeb190900. doi:10.1242/jeb.190900
Bradford M. M. (1976). A rapid and sensitive method for the quantitation of microgram quantities of protein utilizing the principle of protein-dye binding. Anal. Biochem. 72, 248–254. doi:10.1006/abio.1976.9999
Brijs J., Jutfelt F., Clark T. D., Gräns A., Ekström A., Sandblom E. (2015). OF-TISSUE-OXYGEN-SUPPLY, 218, 2448–2454. doi:10.1242/JEB.121889/262056/AM/EXPERIMENTAL-MANIPULATIONS-Experimental manipulations of tissue oxygen supply do not affect warming tolerance of European perchJ. Exp. Biol.
Buckup L., Dutra B. K., Ribarcki F. P., Fernandes F. A., Noro C. K., Oliveira G. T., et al. (2008). Seasonal variations in the biochemical composition of the crayfish Parastacus defossus (Crustacea, Decapoda) in its natural environment. Comp. Biochem. Physiol. Part A Mol. Integr. Physiol. 149, 59–67. doi:10.1016/J.CBPA.2007.10.008
Burleson M. L., Silva P. E. (2011). Cross tolerance to environmental stressors: Effects of hypoxic acclimation on cardiovascular responses of channel catfish (Ictalurus punctatus) to a thermal challenge. J. Therm. Biol. 36, 250–254. doi:10.1016/j.jtherbio.2011.03.009
Cech J. J., Brauner C. J. (2011). VENTILATION and animal respiration | techniques in whole animal respiratory physiology. Encycl. Fish. Physiol. 2, 846–853. doi:10.1016/B978-0-12-374553-8.00128-3
Dalle-Donne I., Aldini G., Carini M., Colombo R., Rossi R., Milzani A. (2006). Protein carbonylation, cellular dysfunction, and disease progression. J. Cell Mol. Med. 10 (2), 389–406. doi:10.1111/j.1582-4934.2006.tb00407.x
Dhabhar F. S. (2014). Effects of stress on immune function: The good, the bad, and the beautiful. Immunol. Res. 582 58, 193–210. doi:10.1007/S12026-014-8517-0
Diaz R. J., Rosenberg R. (2008). Spreading dead zones and consequences for marine ecosystems. Sci. (80-. ) 321, 926–929. doi:10.1126/science.1156401
Ellman G. L., Courtney K. D., Andres V., Featherstone R. M. (1961). A new and rapid colorimetric determination of acetylcholinesterase activity. Biochem. Pharmacol. 7, 88–95. doi:10.1016/0006-2952(61)90145-9
Enriquez C., Mariño-Tapia I., Jeronimo G., Capurro-Filograsso L. (2013). Thermohaline processes in a tropical coastal zone. Cont. Shelf Res. 69, 101–109. doi:10.1016/j.csr.2013.08.018
Epifanio C. E. (2019). Early life history of the blue crab Callinectes sapidus: A review. J. Shellfish Res. 38, 1. doi:10.2983/035.038.0101
García-Rueda A., Tremblay N., Mascaró M., Díaz F., Paschke K., Caamal-Monsreal C., et al. (2021). The thermal tolerance of a tropical population of blue crab (Callinectes sapidus) modulates aerobic metabolism during hypoxia. J. Therm. Biol. 102, 103078. doi:10.1016/J.JTHERBIO.2021.103078
García-Triana A., Zenteno-Savín T., Peregrino-Uriarte A. B., Yepiz-Plascencia G. (2010). Hypoxia, reoxygenation and cytosolic manganese superoxide dismutase (cMnSOD) silencing in Litopenaeus vannamei: Effects on cMnSOD transcripts, superoxide dismutase activity and superoxide anion production capacity. Dev. Comp. Immunol. 34, 1230–1235. doi:10.1016/J.DCI.2010.06.018
Geihs M. A., Vargas M. A., Nery L. E. M. (2014). Damage caused during hypoxia and reoxygenation in the locomotor muscle of the crab Neohelice granulata (Decapoda: Varunidae). Comp. Biochem. Physiol. Part A Mol. Integr. Physiol. 172, 1–9. doi:10.1016/J.CBPA.2014.02.010
Glaspie C. N., Seitz R. D., Lipcius R. N. (2020). Are predator-prey model predictions supported by empirical data? Evidence for a storm-driven shift to an alternative stable state in a crab-clam system. Mar. Ecol. Prog. Ser. 645, 83–90. doi:10.3354/MEPS13368
Glenn E., Comarazamy D., González J. E., Smith T. (2015). Detection of recent regional sea surface temperature warming in the Caribbean and surrounding region. Geophys. Res. Lett. 42, 6785–6792. doi:10.1002/2015GL065002
Góth L. (1991). A simple method for determination of serum catalase activity and revision of reference range. Clin. Chim. Acta. 196, 143–151. doi:10.1016/0009-8981(91)90067-M
Grantham B. A., Chan F., Nielsen K. J., Fox D. S., Barth J. A., Huyer A., et al. (2004). Upwelling-driven nearshore hypoxia signals ecosystem and oceanographic changes in the northeast Pacific. Nature 429, 749–754. doi:10.1038/NATURE02605
Guadagnoli J. A., Tobita K., Reiber C. L. (2007). Assessment of the pressure-volume relationship of the single ventricle of the grass shrimp, Palaemonetes pugio. J. Exp. Biol. 210, 2192–2198. doi:10.1242/jeb.02784
Guadagnoli J. A., Tobita K., Reiber C. L. (2011). Changes in cardiac performance during hypoxic exposure in the grass shrimp Palaemonetes pugio. J. Exp. Biol. 214, 3906–3914. doi:10.1242/jeb.060442
Habig W. H., Jakoby W. B. (1981). Glutathione S-transferases (rat and human). Methods Enzymol. 77, 218–231. doi:10.1016/S0076-6879(81)77029-0
Hadwan M. H., Abed H. N. (2016). Data supporting the spectrophotometric method for the estimation of catalase activity. Data Br. 6, 194–199. doi:10.1016/J.DIB.2015.12.012
Head J. M. (2010). The effects of hypoxia on hemocyanin regulation in Cancer magister: Possible role of Hypoxia-Inducible Factor-1. J. Exp. Mar. Bio. Ecol. 386, 77–85. doi:10.1016/j.jembe.2010.02.010
Hermes-Lima M., Moreira D. C., Rivera-Ingraham G. A., Giraud-Billoud M., Genaro-Mattos T. C., Campos É. G. (2015). Preparation for oxidative stress under hypoxia and metabolic depression: Revisiting the proposal two decades later. Free Radic. Biol. Med. 89, 1122–1143. doi:10.1016/J.FREERADBIOMED.2015.07.156
Hermes-Lima M., Zenteno-Savín T. (2002). Animal response to drastic changes in oxygen availability and physiological oxidative stress. Comp. Biochem. Physiol. Part C Toxicol. Pharmacol. 133, 537–556. doi:10.1016/S1532-0456(02)00080-7
Herrera-Silveira J. A., Comin F. A., Aranda-Cirerol N., Troccoli L., Capurro L. (2004). Coastal water quality assessment in the Yucatan Peninsula: Management implications. Ocean. Coast. Manag. 47, 625–639. doi:10.1016/j.ocecoaman.2004.12.005
Hochachka P. W., Somero G. N. (2002). Biochemical adaptation : Mechanism and process in physiological evolution. Oxford University Press. Available at:https://global.oup.com/academic/product/biochemical-adaptation-9780195117035?cc=mx&lang=en& (Accessed April 20, 2017).
Homola E., Chang E. S. (1997). Distribution and regulation of esterases that hydrolyze methyl farnesoate inHomarus americanusand other Crustaceans. Gen. Comp. Endocrinol. 106, 62–72. doi:10.1006/GCEN.1996.6850
Hosokawa M., Satoh T. (2002). Measurement of carboxylesterase (CES) activities. Curr. Protoc. Toxicol. Chapter 4, Unit4.7. doi:10.1002/0471140856.TX0407S10
Jayasundara N., Somero G. N. (2013). Physiological plasticity of cardiorespiratory function in a eurythermal marine teleost, the longjaw mudsucker, Gillichthys mirabilis. J. Exp. Biol. 216, 2111–2121. doi:10.1242/jeb.083873
Johnson D. S. (2015). The savory swimmer swims north: A northern range extension of the blue crab callinectes sapidus? J. Crustac. Biol. 35, 105–110. doi:10.1163/1937240X-00002293
Jung E. H., Brix K. V., Richards J. G., Val A. L., Brauner C. J. (2020). Reduced hypoxia tolerance and survival at elevated temperatures may limit the ability of Amazonian fishes to survive in a warming world. Sci. Total Environ. 748, 141349. doi:10.1016/j.scitotenv.2020.141349
King E. E., Williams C. M., Stillman J. H. (2022). Hypoxia decreases thermal sensitivity and increases thermal breadth of locomotion in the invasive freshwater snail potamopyrgus antipodarum, 251–264. doi:10.1086/719899.95
Kumar V., Sinha A. K., Romano N., Allen K. M., Bowman B. A., Thompson K. R., et al. (2018). Metabolism and nutritive role of cholesterol in the growth, gonadal development, and reproduction of Crustaceans. Rev. Fish. Sci. Aquac. 26, 254–273. doi:10.1080/23308249.2018.1429384
Levin L. A., Ekau W., Gooday A. J., Jorissen F., Middelburg J. J., Naqvi S. W. A., et al. (2009). Effects of natural and human-induced hypoxia on coastal benthos. Biogeosciences 6, 2063–2098. doi:10.5194/bg-6-2063-2009
Maciel F. E., Rosa C. E., Santos E. A., Monserrat J. M., Nery L. E. M. (2004). Daily variations in oxygen consumption, antioxidant defenses, and lipid peroxidation in the gills and hepatopancreas of an estuarine crab. Can. J. Zool. 82, 1871–1877. doi:10.1139/Z04-182
Masson-Delmotte V., Zhai P., Pörtner H.-O., Roberts D., Skea J., Shukla P. R., et al. (2019). Global warming of 1.5°C an IPCC Special Report on the impacts of global warming of 1.5°C above pre-industrial levels and related global greenhouse gas emission pathways, in the context of strengthening the global response to the threat of climate change, sustainable development, and efforts to eradicate poverty Edited by Science Officer Science Assistant Graphics Officer Working Group I Technical Support Unit. Available at: (Accessed May 3, 2022).
McBryan T. L., Healy T. M., Haakons K. L., Schulte P. M. (2016). Warm acclimation improves hypoxia tolerance in Fundulus heteroclitus. J. Exp. Biol. 219, 474–484. doi:10.1242/jeb.133413
McMahon B. R. (2001). Respiratory and circulatory compensation to hypoxia in crustaceans. Respir. Physiol. 128, 349–364. doi:10.1016/S0034-5687(01)00311-5
Menezes S., Soares A. M. V. M., Guilhermino L., Peck R. M. (2006). Biomarker responses of the estuarine Brown shrimp Crangon crangon L. to non-toxic stressors: Temperature, salinity and handling stress effects. J. Exp. Mar. Bio. Ecol. 335, 114–122. doi:10.1016/J.JEMBE.2006.03.009
Mesquita C. S., Oliveira R., Bento F., Geraldo D., Rodrigues J. V., Marcos J. C. (2014). Simplified 2, 4-dinitrophenylhydrazine spectrophotometric assay for quantification of carbonyls in oxidized proteins. Anal. Biochem. 458, 69–71. doi:10.1016/J.AB.2014.04.034
Murray-Tortarolo G. N. (2021). Seven decades of climate change across Mexico. Atmósfera 34, 217–226. doi:10.20937/atm.52803
Nos D., Navarro J., Macías D., Solé M. (2021). Carboxylesterase activities as potential biomarkers of pollution in marine pelagic predators. Ecol. Indic. 122, 107217. doi:10.1016/J.ECOLIND.2020.107217
Okolodkov Y., Merino-Virgilio F. D. C., Aké-Castillo J. A., Aguilar-Trujillo A., Espinosa-Matías S., Herrera-Silveira J. (2014). Seasonal changes in epiphytic dinoflagellate assemblages near the northern coast of the Yucatan Peninsula, Gulf of Mexico. Acta Bot. Mex. 107, 121–151.
Oksanen J., Blanchet F. G., Friendly M., Kindt R., Legendre P., McGlinn D., et al. (2022). vegan: Community ecology package. R. package version 2, 4–284.
Paital B., Chainy G. B. N. (2010). Antioxidant defenses and oxidative stress parameters in tissues of mud crab (Scylla serrata) with reference to changing salinity. Comp. Biochem. Physiol. - C Toxicol. Pharmacol. 151, 142–151. doi:10.1016/j.cbpc.2009.09.007
Pellegrino R., Kucharski L. C., Da Silva R. S. M. (2008). Effect of fasting and refeeding on gluconeogenesis and glyconeogenesis in the muscle of the crab Chasmagnathus granulatus previously fed a protein- or carbohydrate-rich diet. J. Exp. Mar. Bio. Ecol. 358, 144–150. doi:10.1016/J.JEMBE.2008.02.005
Pörtner H. (2002). Climate variations and the physiological basis of temperature dependent biogeography: Systemic to molecular hierarchy of thermal tolerance in animals. Comp. Biochem. Physiol. Part A Mol. Integr. Physiol. 132, 739–761. doi:10.1016/S1095-6433(02)00045-4
Pörtner H. O., Bennett A. F., Bozinovic F., Clarke A., Lardies M. A., Lucassen M., et al. (2006). Trade-offs in thermal adaptation: The need for a molecular to ecological integration. Physiol. Biochem. Zool. 79, 295–313. doi:10.1086/499986
Pörtner H. O., Farrell A. P. (2008). Physiology and climate change. Science (80-. ). 322. Available at:http://science.sciencemag.org/content/322/5902/690.
Pörtner H. O. (2010). Oxygen- and capacity-limitation of thermal tolerance: A matrix for integrating climate-related stressor effects in marine ecosystems. J. Exp. Biol. 213, 881–893. doi:10.1242/JEB.037523
Rabalais N. N., Turner R. E., Sen Gupta B. K., Boesch D. F., Chapman P., Murrell M. C. (2007). Hypoxia in the northern Gulf of Mexico: Does the science support the plan to reduce, mitigate, and control hypoxia? Estuaries Coasts 30, 753–772. doi:10.1007/BF02841332
Rodríguez-Fuentes G., Armstrong J., Schlenk D. (2008). Characterization of muscle cholinesterases from two demersal flatfish collected near a municipal wastewater outfall in Southern California. Ecotoxicol. Environ. Saf. 69, 466–471. doi:10.1016/J.ECOENV.2007.06.008
Romero M. C., Schvezov N., Sotelano M. P., Diez M. J., Florentin O., Tapella F., et al. (2013). Antioxidant defenses and lipid peroxidation in two Lithodes species from South Atlantic during summer. Rev. Biol. Mar. Oceanogr. 48, 227–234. doi:10.4067/S0718-19572013000200003
Rosas C., Cuzon G., Gaxiola G., Le Priol Y., Pascual C., Rossignyol J., et al. (2001a). Metabolism and growth of juveniles of Litopenaeus vannamei: Effect of salinity and dietary carbohydrate levels. J. Exp. Mar. Bio. Ecol. 259, 1–22. doi:10.1016/S0022-0981(01)00222-2
Rosas C., Cuzon G., Gaxiola G., Pascual C., Taboada G., Arena L., et al. (2002). An energetic and conceptual model of the physiological role of dietary carbohydrates and salinity on Litopenaeus vannamei juveniles. J. Exp. Mar. Bio. Ecol. 268, 47–67. doi:10.1016/S0022-0981(01)00370-7
Rosas C., Cuzon G., Taboada G., Pascual C., Gaxiola G., Van Wormhoudt A. (2001b). Effect of dietary protein and energy levels on growth, oxygen consumption, haemolymph and digestive gland carbohydrates, nitrogen excretion and osmotic pressure of Litopenaeus vannamei (Boone) and L. setiferus (Linne) juveniles (Crustacea, Decapoda; Penae. Aquac. Res. 32, 531–547. doi:10.1046/J.1365-2109.2001.00573.X
Saiz E., Griffell K., Olivares M., Solé M., Theodorou I., Calbet A. (2022). Reduction in thermal stress of marine copepods after physiological acclimation. J. Plankton Res. 44, 427–442. doi:10.1093/PLANKT/FBAC017.--
Seibel B. A., Andres A., Birk M. A., Burns A. L., Shaw C. T., Timpe A. W., et al. (2021). Oxygen supply capacity breathes new life into critical oxygen partial pressure (Pcrit). J. Exp. Biol. 224, jeb242210. doi:10.1242/JEB.242210
Seitz R. D. (2020). Blue Crabs: Beautiful Savory Swimmers of the Chesapeake Bay, 107–125. doi:10.1007/978-3-030-45481-4_7
Smith K. J., Able K. W. (2003). Dissolved oxygen dynamics in salt marsh pools and its potential impacts on fish assemblages. Mar. Ecol. Prog. Ser. 258, 223–232. doi:10.3354/meps258223
Sokolova I. M., Frederich M., Bagwe R., Lannig G., Sukhotin A. A. (2012). Energy homeostasis as an integrative tool for assessing limits of environmental stress tolerance in aquatic invertebrates. Mar. Environ. Res. 79, 1–15. doi:10.1016/j.marenvres.2012.04.003
Trasviña-Arenas C. H., Garcia-Triana A., Peregrino-Uriarte A. B., Yepiz-Plascencia G. (2013). White shrimp Litopenaeus vannamei catalase: Gene structure, expression and activity under hypoxia and reoxygenation. Comp. Biochem. Physiol. B. Biochem. Mol. Biol. 164, 44–52. doi:10.1016/J.CBPB.2012.10.004
Vasudevan S., Rajendran S. (2021). Thermal stress induced hyperglycemia in the blue swimmer crab, Portunus pelagicus. J. Therm. Biol. 100, 103076. doi:10.1016/j.jtherbio.2021.103076
Verberk W. C. E. P., Overgaard J., Ern R., Bayley M., Wang T., Boardman L., et al. (2016). Does oxygen limit thermal tolerance in arthropods? A critical review of current evidence. Comp. Biochem. Physiol. -Part A Mol. Integr. Physiol. 192, 64–78. doi:10.1016/j.cbpa.2015.10.020
Vinagre A. S., Nunes do Amaral A. P., Ribarcki F. P., Fraga da Silveira E., Périco E. (2007). Seasonal variation of energy metabolism in ghost crab Ocypode quadrata at Siriú Beach (Brazil). Comp. Biochem. Physiol. Part A Mol. Integr. Physiol. 146, 514–519. doi:10.1016/J.CBPA.2006.02.004
Vinagre C., Leal I., Mendonça V., Madeira D., Narciso L., Diniz M. S., et al. (2016). Vulnerability to climate warming and acclimation capacity of tropical and temperate coastal organisms. Ecol. Indic. 62, 317–327. doi:10.1016/j.ecolind.2015.11.010
Vinagre C., Madeira D., Mendonça V., Dias M., Roma J., Diniz M. S. (2014). Effect of increasing temperature in the differential activity of oxidative stress biomarkers in various tissues of the Rock goby, Gobius paganellus. Mar. Environ. Res. 97, 10–14. doi:10.1016/J.MARENVRES.2014.01.007
Welker A. F., Moreira D. C., Campos É. G., Hermes-Lima M. (2013). Role of redox metabolism for adaptation of aquatic animals to drastic changes in oxygen availability. Comp. Biochem. Physiol. A. Mol. Integr. Physiol. 165, 384–404. doi:10.1016/J.CBPA.2013.04.003
Wong C. M., Marcocci L., Liu L., Suzuki Y. J. (2010). Cell signaling by protein carbonylation and decarbonylation. Antioxid. Redox Signal. 12, 393–404. doi:10.1089/ARS.2009.2805
Wouters R., Lavens P., Nieto J., Sorgeloos P. (2001). Penaeid shrimp broodstock nutrition: An updated review on research and development. Aquaculture 202, 1–21. doi:10.1016/S0044-8486(01)00570-1
Xie Z., Wei S., Dong H., Chen H., Zhang Q., Liu W., et al. (2021). Hemocyte responses of the oyster Crassostrea hongkongensis exposed to diel-cycling hypoxia and salinity change. Front. Mar. Sci. 8, 1280. doi:10.3389/fmars.2021.749623
Zang Y., Zhang X., Huang P., Ame K. H., Shen H. (2020). Cloning and expression of two carboxylesterases, and their activity modulation in Chinese mitten crab eriocheir sinensis under pesticide exposer. Turk. J. Fish. Aquat. Sci. 20, 521–529. doi:10.4194/1303-2712-V20_7_02
Zhang J., Gilbert D., Gooday A. J., Levin L., Naqvi S. W. A., Middelburg J. J., et al. (2010). Natural and human-induced hypoxia and consequences for coastal areas: Synthesis and future development. Biogeosciences 7, 1443–1467. doi:10.5194/bg-7-1443-2010
Zillén L., Conley D. J., Andrén T., Andrén E., Björck S. (2008). Past occurrences of hypoxia in the Baltic Sea and the role of climate variability, environmental change and human impact. Earth-Science Rev. 91, 77–92. doi:10.1016/j.earscirev.2008.10.001
Keywords: Callinectes sapidus, dissolved oxygen, thermal changes, routine metabolism, physiological indicators
Citation: Garcia-Rueda AL, Mascaro M, Rodriguez-Fuentes G, Caamal-Monsreal CP, Diaz F, Paschke K and Rosas C (2023) Moderate hypoxia mitigates the physiological effects of high temperature on the tropical blue crab Callinectes sapidus. Front. Physiol. 13:1089164. doi: 10.3389/fphys.2022.1089164
Received: 04 November 2022; Accepted: 15 December 2022;
Published: 05 January 2023.
Edited by:
Alaa El-Din Hamid Sayed, Assiut University, EgyptReviewed by:
Daniel Carneiro Moreira, University of Brasilia, BrazilCopyright © 2023 Garcia-Rueda, Mascaro, Rodriguez-Fuentes, Caamal-Monsreal, Diaz, Paschke and Rosas. This is an open-access article distributed under the terms of the Creative Commons Attribution License (CC BY). The use, distribution or reproduction in other forums is permitted, provided the original author(s) and the copyright owner(s) are credited and that the original publication in this journal is cited, in accordance with accepted academic practice. No use, distribution or reproduction is permitted which does not comply with these terms.
*Correspondence: Carlos Rosas, Y3J2QGNpZW5jaWFzLnVuYW0ubXg=
Disclaimer: All claims expressed in this article are solely those of the authors and do not necessarily represent those of their affiliated organizations, or those of the publisher, the editors and the reviewers. Any product that may be evaluated in this article or claim that may be made by its manufacturer is not guaranteed or endorsed by the publisher.
Research integrity at Frontiers
Learn more about the work of our research integrity team to safeguard the quality of each article we publish.