- 1College of Human Kinesiology, Shenyang Sport University, Shenyang, Liaoning, China
- 2College of Rehabilitation Medicine, Fujian University of Traditional Chinese Medicine, Fuzhou, Fujian, China
- 3Department of Rehabilitation Medicine, Nanfang Hospital, Southern Medical University, Guangzhou, Guangdong, China
Objective:Previous behavioral studies have reported the potential of transcranial alternating current stimulation in analyzing the causal relationship between neural activity and behavior. However, the efficacy of tACS on motor performance and learning in healthy individuals remains unclear. This systematic reviewexamines the effectiveness of tACS on motor performance and motor learning in healthy individuals.
Methods: Literature was systematically searched through the Cochrane Library, PubMed, EMBASE, and Web of Science until 16 October 2022. Studies were eligible for review if they were randomized, parallel, or crossover experimental designs and reported the efficacy of tACS on motor performance and motor learning in healthy adults. Review Manager 5.3 was used to evaluate the methodological quality and analyze the combined effect.
Results: Ten studies (270 participants) met all the inclusion criteria. The results showed that motor performance was not significantly greater than that with sham tACS stimulation [I2 = 44%, 95% CI (–0.01, 0.35), p = 0.06, standardized mean difference = 0.17], whereas motor learning ability improved significantly [I2 = 33%, 95% CI (−1.03, −0.31), p = 0.0002, SMD = −0.67]. Subgroup analysis found that gamma bend tACS could affect the changes in motor performance (I2 = 6%, 95% CI (0.05, 0.51), p = 0.02, SMD = 0.28), and online tACS did as well [I2 = 54%, 95% CI (0.12, 0.56), p = 0.002, SMD = 0.34].
Conclusion: The results showed that tACS effectively improves motor performance (gamma band and online mode) and motor learning in healthy individuals, which indicates that tACS may be a potential therapeutic tool to improve motor behavioral outcomes. However, further evidence is needed to support these promising results.
Systematic Review Registration: PROSPERO, identifier CRD42022342884
1 Introduction
Motor performance and motor learning play a leading role in improving the quality of daily life in healthy adults, including physiological aspects, psychological health, and cognitive performance (Li et al., 2022; Orssatto et al., 2022; Wiegel et al., 2022; Zhou et al., 2022). Studies in the field of sports sciences have indicated that transcranial alternating current stimulation (tACS) can be a promising noninvasive brain stimulation tool to improve motor performance and motor learning, slowing declines in physical function (Urrútia and Bonfill, 2010; Antal and Herrmann, 2016).
TACS is a unique noninvasive form of brain stimulation that modulates the internal nerve oscillation by delivering a low-intensity sinusoidal alternating current to the scalp (Elyamany et al., 2021), forcing the membrane potential oscillation away from its resting potential to slightly increased depolarization or hyperpolarization (Wu et al., 2021). In the depolarization state, neurons are more likely to respond to other neurons; this reaction is called stochastic resonance (Fertonani and Miniussi, 2017). The final effect of this reaction is that the firing time of neurons is locked to the increased stimulation frequency (Del Felice et al., 2019). Therefore, tACS plays a regulatory rather than dominant role and has great potential in the analysis of the causal relationship between neural activity and behavior (Herrmann et al., 2013).
In terms of motor performance and motor learning, there are further limitations and gaps in the existing research related to the effects of tACS. For motor performance, Rumpf et al. found no change in motor performance after applying 10 or 20 Hz tACS with an intensity of 1 mA to stimulate the left primary motor cortex (M1) during the motion sequence task (Rumpf et al., 2019). In contrast, Miyaguchi et al. used different amplitudes for tACS stimulation but found significant differences in motor performance between groups and significantly reduced task errors (Miyaguchi et al., 2019). The results were in accordance with Del Felice et al.‘s study, even in patients with Parkinson’s disease (Del Felice et al., 2019).
For motor learning, Krause et al. found that 10 Hz tACS stimulation can significantly reduce the reaction time and enhance motor learning ability after applying 1 mA, 10 Hz, or 20 Hz tACS stimulation to the left M1 (Krause et al., 2016). Antal et al. applied 1, 10, 15, 30, and 45 Hz tACS stimulation to the left M1. Transcranial magnetic stimulation (TMS) was used to detect the changes in spinal cord excitability before and after the reaction time task (RTT). Excitability significantly increased with only 10 Hz tACS stimulation, which indicated that 10 Hz tACS stimulation could improve motor learning (Antal et al., 2008). These results may be associated with stimulus parameters.
In view of the above, the efficacy of tACS on motor performance and motor learning skill needs to be explored. The present study systematically evaluated the effectiveness of tACS on motor performance and motor learning to determine the optimal treatment parameters of tACS for healthy adults, with the expectation of enriching the application of tACS in sports science.
2 Methods
Consistent with the preferred reporting items for systematic reviews and meta-analyses (PRISMA) statement (Moher et al., 2009), we conducted a systematic review and meta-analysis, which were registered with PROSPERO (registration number: CRD42022342884).
2.1 Inclusion criteria
The inclusion criteria strictly followed participants, interventions, controls, outcomes, and study design (PICOS) principles: 1) Participants: healthy adults; 2) Intervention: tACS; 3) Control: sham stimulation of tACS; 4) Outcomes: related outcome indicators of motor function [outcomes related to motor performance: task error, number of parts, accuracy, error rate (ER), time on target, seated chest pass throw (SCPT), seated backward overhead medicine ball throw (SBOMBT), squat jump (SJ), counter-movement jump (CMJ), counter-movement jump arm-swing (CMJ-AS); outcome related to motor learning: reaction time (RT)]; 5) Study design: random experiment, parallel, or cross-experimental design. Review articles, case studies, and animal studies were all excluded.
2.2 Source of information
As of 16 October 2022, the Cochrane Library, PubMed, EMBASE, and Web of Science were searched without using filters.
2.3 Search strategy
We searched using the following keywords: “transcranial alternating current stimulation” OR “tACS” OR “HD-tACS” and “motor behavior” OR “motor performance” OR “resistance” OR “strength” OR “weight” OR “power” OR “training” OR “training” OR “motor learning” OR “sequence learning” OR “motor skill” OR “motor acquisition”.
2.4 Study selection
Kun Hu and Ruihan Wan oversaw the initial screening via titles and abstracts from the electronic databases, independently and jointly excluding unrelated studies by reading titles and abstracts. For articles with unclear relevance, their full texts were retrieved to further evaluate their relevance. Then, all included articles were divided into relevant, possible, or irrelevant. The two authors determined the extent to which related research was subject to the PICOS principle. Finally, they carefully judged possible related studies and excluded unrelated studies. The places with different opinions were finally decided by the third author (Feng Guo).
2.5 Data extraction
The form on extracted information was jointly designed by two authors (Kun Hu and Ruihan Wan). The main extracted contents were as follows: characteristics of the participants (sample size, age, gender and group), tACS intervention program (electrode size, electrode position, intensity, and stimulus mode), exercise program (isometric force task, physical task, and so on), and main results (task error, accuracy, and so on). All the contents were extracted by the two authors. If the relevance was unclear, then the two authors would have a discussion to further reduce the risk of data extraction bias.
2.6 Quality assessment
Risk of bias was assessed in accordance with the criteria set out in the Cochrane Guidelines (Higgins and Green, 2011) (a) random sequence generation; (b) allocation concealment; (c) blinding of participants and personnel; (d) blinding of the outcome assessments; (e) incomplete outcome data; (f) selective reporting; and (g) other biases. The two evaluators (Kun Hu and Ruihan Wan) evaluated the eligible studies independently, and divided these into low, high, and unclear risk prejudices on the basis of the criteria (Higgins and Green, 2011). Any disagreement was discussed by the two evaluators and finally decided by the third author (Feng Guo).
2.7 Quantitative analysis
Review Manager software (RevMan 5.3; Cochrance Collaboration) was used for quantitative analysis. The main contents were as follows: subject characteristics, eligibility criteria, intervention programs, and main outcomes. Then, the heterogeneity of the study was evaluated to determine whether it was suitable for comprehensive analysis. To make the content of studies with large differences more comparable, the random-effects model was applied. The standardized mean difference (SMD) and 95% confidence interval (95% CI) were used to avoid the different measurement units of the data in the extracted research. Heterogeneity was evaluated by using chi-squared statistics (Chi2) and the heterogeneity index (I2). Significant heterogeneity exists when I2 is greater than 50% (Higgins and Green, 2011). The sources of heterogeneity were identified through subgroup analysis. Finally, sensitivity analysis was applied to exclude low-quality studies. In addition to evaluating the heterogeneity between studies, all values were analyzed by two-tailed analysis with a significance level of 5% (The p-value of the final result was calculated by RevMan 5.3. If p˂0.05, it indicated that tDCS had a significant effect on motor performance and motor learning.).
3 Results
A total of 1780 articles were initially screened from the electronic databases. After removing duplicate articles (n = 189) and relevant articles (n = 1,591), 19 articles were retained based on titles and abstracts. Articles that did not meet the inclusion criteria were excluded by browsing the full text (n = 6), including for qualitative analysis (n = 13). Ten eligible articles were analyzed quantitatively, as shown in Figure 1.
3.1 Research characteristics
Table 1 provides a detailed description of the participants’ characteristics of the effects of tACS on motor performance and motor learning. A total of 270 participants were involved in this analysis (170 males [63%]; 100 females [37%]), and the average age was between 20.7 ± 0.75 (Miyaguchi et al., 2019) and 66.8 ± 5.7 (Rumpf et al., 2019) years old. The control group of all studies was the sham group, and the experimental group of six studies (60%) was divided into two groups—alpha (a) (10 Hz), beta (ß) (20 Hz) group (Krause et al., 2016; Rumpf et al., 2019), or β (20 Hz), gamma (?) (>30 Hz) group (Miyaguchi et al., 2018; Miyaguchi et al., 2020; Giustiniani et al., 2021; Ma et al., 2021)—according to stimulus frequencies; three studies (30%) had one experimental group (γ group) (Miyaguchi et al., 2019; Miyaguchi et al., 2019; Miyaguchi et al., 2022); one (10%) study also had one experimental group (β group) (Yamaguchi et al., 2020).
Table 2 describes the research characteristics of the impact of tACS on motor performance and motor learning in detail. All the included studies were randomized, among which 6 articles (60%) were crossover studies (Miyaguchi et al., 2018; Miyaguchi et al., 2019; Miyaguchi et al., 2019; Miyaguchi et al., 2020; Ma et al., 2021; Miyaguchi et al., 2022) and four articles (40%) were parallel studies (Krause et al., 2016; Rumpf et al., 2019; Yamaguchi et al., 2020; Giustiniani et al., 2021). Eight studies (80%) were related to motor performance (Miyaguchi et al., 2018; Miyaguchi et al., 2019; Miyaguchi et al., 2019; Rumpf et al., 2019; Yamaguchi et al., 2020; Giustiniani et al., 2021; Miyaguchi et al., 2020; Miyaguchi et al., 2022), and 2 (20%) studies were related to motor learning (Krause et al., 2016; Ma et al., 2021). With regard to the exercise program included in the study, the exercise tasks of all studies in motor performance were inconsistent, including visuomotor control task (VCT), visuomotor tacking (VMT), Purdue Pegboard Test (PPT), motor sequence learning task (MSLT), physical test (PT), and isometric force task (IFT); in motor learning, all the research tasks were RTTs. The stimuli in the seven studies were located at the left M1 (Krause et al., 2016; Miyaguchi et al., 2018; Miyaguchi et al., 2019; Miyaguchi et al., 2019; Rumpf et al., 2019; Yamaguchi et al., 2020; Ma et al., 2021); one study simultaneously stimulated the left and right M1 (Giustiniani et al., 2021); the stimulation position of two studies was the supplementary motor area (SMA) (Miyaguchi et al., 2020; Miyaguchi et al., 2022). The stimulation intensity was 1 mA (Krause et al., 2016; Miyaguchi et al., 2018; Miyaguchi et al., 2019; Miyaguchi et al., 2019; Rumpf et al., 2019; Miyaguchi et al., 2020; Miyaguchi et al., 2022), 1.5 mA (Giustiniani et al., 2021) and 2 mA (Yamaguchi et al., 2020), and the area of the stimulation electrode was 25 cm2 (Miyaguchi et al., 2018; Miyaguchi et al., 2019; Miyaguchi et al., 2019; Miyaguchi et al., 2020; Giustiniani et al., 2021; Miyaguchi et al., 2022) and 35 cm2 (Krause et al., 2016; Rumpf et al., 2019; Yamaguchi et al., 2020). We could not determine the intensity and area of stimulation in Ma’s study (Ma et al., 2021). The stimuli in the six studies (60%) were online stimuli (Miyaguchi et al., 2018; Miyaguchi et al., 2019; Miyaguchi et al., 2019; Miyaguchi et al., 2020; Ma et al., 2021; Miyaguchi et al., 2022); the stimuli in the four studies (40%) were offline stimuli (Krause et al., 2016; Rumpf et al., 2019; Yamaguchi et al., 2020; Giustiniani et al., 2021).
3.2 Main results and quantitative synthesis
3.2.1 Effect of tACS on motor performance
In terms of the impact of tACS on motor performance, eight studies were included in this analysis (Miyaguchi et al., 2018; Miyaguchi et al., 2019; Miyaguchi et al., 2019; Rumpf et al., 2019; Miyaguchi et al., 2020; Yamaguchi et al., 2020; Giustiniani et al., 2021; Miyaguchi et al., 2022). The outcomes of each study were inconsistent. Giustiniani et al. included five outcomes (Giustiniani et al., 2021) from seated chest pass throw (SCPT), seated backward overhead medicine ball throw (SBOMBT), squat jump (SJ), counter-movement jump (CMJ), and counter-movement jump arm-swing (CMJ-AS). The outcome indicators of the other seven studies are task error, number of parts, accuracy, time of target and error rate (Miyaguchi et al., 2018; Miyaguchi et al., 2019; Miyaguchi et al., 2019; Rumpf et al., 2019; Miyaguchi et al., 2020; Yamaguchi et al., 2020; Miyaguchi et al., 2022). The experimental groups in most studies included two groups, namely, the α, β, or β, γ groups (Miyaguchi et al., 2019; Rumpf et al., 2019; Miyaguchi et al., 2020; Giustiniani et al., 2021); three experimental groups were γ groups (Miyaguchi et al., 2018; Miyaguchi et al., 2019; Miyaguchi et al., 2022); and only one experimental group was used by Yamaguchi, which is the β group (Yamaguchi et al., 2020). Therefore, a total of 15 datasets were included in this synthesis. Compared with the sham group, no significant effect was found on the improvement of exercise performance for application of tACS (Z = 1.85, p = 0.06). Nevertheless, moderate heterogeneity (Chi2 = 24.81, p = 0.04, I2 = 44%) was observed. Thus, quantitative synthesis could be included (Figure 2).
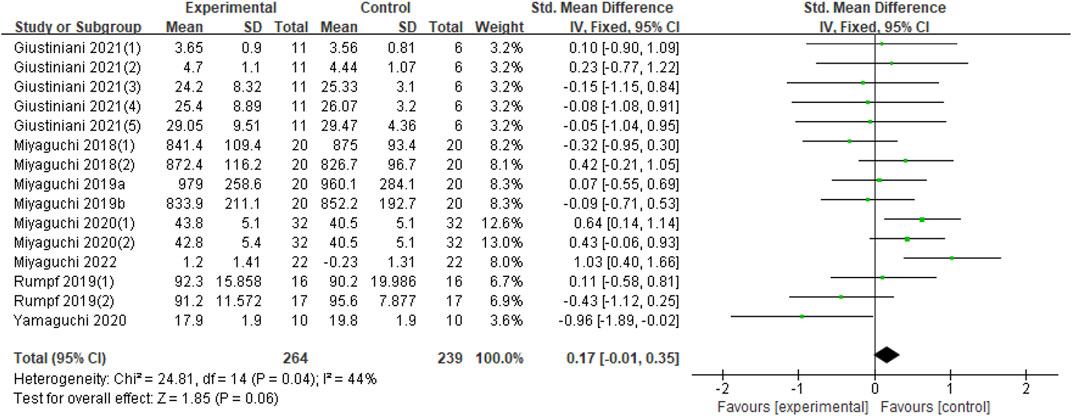
FIGURE 2. Forest plot for the effect of tACS on improving motor performance. Notes: Giustiniani (1) (2) (3) (4) (5)-The five outcome measures in Giustiniani’s study were SCPT, SBOMBT, SJ, CMJ, CMJ-AS; Miyaguchi 2018 (1) (2)- The 20 Hz and 70 Hz stimuli in this study, respectively; Miyaguchi 2020 (1) (1)- The 20 Hz and 80 Hz stimuli in this study, respectively; Rumpf (1) (2)- The 10 Hz and 20 Hz stimuli in this study, respectively; Miyaguchi et al. published two studies in 2019 distinguished by a and (B)
3.2.2 Influence of tACS with different frequencies on motor performance
The tACS studies included in this meta-analysis were mainly divided into three different stimulus frequencies (alpha, beta, and gamma bands). Among the 15 datasets, only one dataset was the alpha (10 Hz) band (one dataset could not be included in the synthetic analysis), and the other datasets were the beta (20 Hz) and gamma (>30 Hz) bands. Therefore, we tried to incorporate tACS research at beta and gamma frequencies into quantitative synthesis, with a total of 14 datasets.
3.2.2.1 β-tACS (20 Hz)
Four datasets (28.6%) were included in this study. Compared with the sham group, no significant effect was observed on the improvement of motor performance for β-tACS (Z = 0.21, p = 0.84) with more significant heterogeneity (Chi2 = 12.76, p = 0.005, I2 = 76%) (Figure 3).
3.2.2.2 γ-tACS(>30 Hz)
Ten datasets (71.4%) were included in this study. Compared with the sham group, γ-tACS had significant effect on improving motor performance (Z = 2.40, p = 0.02), and had good heterogeneity (Chi2 = 9.58, p = 0.39, I2 = 6%) (Figure 4).
3.2.3 Effects of different tACS stimulation modes on motor performance
The studies included in this meta-analysis were mainly divided into two types of stimuli (online and offline). Fifteen datasets were included in the quantitative synthesis.
3.2.3.1 Online
Seven datasets were included in the synthetic analysis. Compared with the sham group, online stimulation significantly improved motor performance (Z = 3.02, p = 0.002) but with moderate heterogeneity (Chi2 = 13.06, p = 0.04, I2 = 54%) (Figure 5).
3.2.3.2 Offline
For meta-analysis, eight datasets were included in the quantitative synthesis. Compared with the sham group, offline stimulation did not improve motor performance (Z = 1.06, p = 0.29) and had no significant heterogeneity (Chi2 = 4.90, p = 0.67, I2 = 0%) (Figure 6).
3.2.4 Influence of tACS on motor learning
With regard to the effect of tACS on motor learning, this analysis included two studies. The experimental groups in both studies had two groups; thus, the experimental group in Krause’s study was α and β-tACS stimulation (Krause et al., 2016); in the study of Ma (Ma et al., 2021), β and γ stimuli were used in the experimental group. Four datasets are therefore included in this synthesis. Compared with the sham group, tACS significantly improved motor learning ability (Z = 3.68, p = 0.0002) but had moderate heterogeneity (Chi2 = 4.48, p = 0.21, I2 = 33%) (Figure 7).

FIGURE 7. Forest plot for the effect of tACS on improving motor learning. Notes: Krause (1) (2)- The 10 Hz and 20 Hz stimuli in this study, respectively; Ma (1) (2)- The 20 Hz and 70 Hz stimuli in this study, respectively.
3.2.4.1 Risk of bias in included studies
As shown in Figure 8, reporting bias (selective report results), detection bias (blinding of the outcome assessments), performance bias (blinding of participants and personnel), and other biases were all low-risk biases. For random sequence generation and allocation concealment, Miyaguchi (2018), Miyaguchi (2019)a, Miyaguchi (2019)b, Miyaguchi (2020) and Miyaguchi (2022) did not report these biases (Miyaguchi et al. published two studies in 2019, which we distinguished by a and b) (Miyaguchi et al., 2018; Miyaguchi et al., 2019; Miyaguchi et al., 2019; Miyaguchi et al., 2020; Miyaguchi et al., 2022); thus, they were unclear risk biases. In addition, in the attrition bias (incomplete outcome data), one subject was dropped from the experiment in Rumpf’s study, resulting in high-risk bias being reported (Rumpf et al., 2019).
4 Discussion
To our knowledge, the present study was the first to systematically evaluate the efficacy of tACS on motor performance and motor learning in healthy individuals. Significant conclusions can be shown that tACS can effectively improve motor performance (beta band and online mode) and motor learning in healthy individuals compared with the sham group.
Although the mechanism of tACS action remains unclear, previous studies have shown that entrainment of stimulation frequencies by brain oscillations and coupling or decoupling of distant oscillatory connections between distant brain regions may be involved (Weinrich et al., 2017; Schwab et al., 2019; Elyamany et al., 2021). Therefore, the effectiveness of tACS on motor performance in the current study was divided into two main categories, including online effects (during the stimulation period) and offline effects (outlast the stimulation period); Considering the difference in stimulation frequency, the current study classified it into three categories—α (10 Hz), β (20 Hz), and γ-tACS (>30 Hz) —to analyze on the premise of roughly the same stimulation position and intensity.
Although the synthesis results showed low heterogeneity (Figure 2), the uncertainty of the synthesis of the study can be diminished. In the 15 datasets of eight studies, only one dataset was α-tACS; β-tACS and γ-tACSs are mainstream, consistent with the current research status. The current belief is that β-tACS and γ-tACS stimuli affect motor performance and motor learning (Pogosyan et al., 2009; Joundi et al., 2012; Pollok et al., 2015; Moisa et al., 2016; Santarnecchi et al., 2017). Therefore, we mainly discussed β-tACS and γ-tACSs.
In terms of motor performance, as shown in Figure 3, β-tACS did not improve motor performance in healthy individuals and presented a significant heterogeneity, which may be linked to the differences in exercise program, tACS stimulation location, and stimulation mode. Notably, Miyaguchi et al. (2020) found that β-tACS was positively correlated with exercise performance and γ-tACS was negatively correlated with motor performance. These results were in line with Muthukumaraswamy’s study (Muthukumaraswamy et al., 2011). β-band activity was also deemed anti-dynamic activity, and γ-band activity was essentially pro-dynamic activity (Miyaguchi et al., 2018). These findings indicate that different stimulus frequencies played a major role in motor performance (Miyaguchi et al., 2020). In contrast, γ-tACS resulted in improved motor performance, and the results were not significantly heterogeneous (Figure 4). Interestingly, ten datasets of six studies were included, and five datasets were from the same study. The other five datasets come from five studies by the same team: Miyaguchi (2018), Miyaguchi (2019) a, Miyaguchi (2019) b Miyaguchi (2020) and Miyaguchi (2022) (Miyaguchi et al. published two studies in 2019, which we distinguished by a and b) (Miyaguchi et al., 2018; Miyaguchi et al., 2019; Miyaguchi et al., 2019; Miyaguchi et al., 2020; Miyaguchi et al., 2022). The effect of tACS stimulation at different frequencies (70 Hz or 80 Hz) on motor performance was significantly different, which was not reflected by Giustiniani et al., who applied a frequency of 50 Hz (Giustiniani et al., 2021). This result may have occurred because 50 Hz tACS was thought to increase the speed of visual motion (Moisa et al., 2016) and time-dependent modulation of γ-aminobutyric acid (Nowak et al., 2017), indicating that tACS stimuli in the γ-band have great frequency specificity. Further attention should be given to the detailed frequency study of γ-tACS.
In relation to the stimulation of tACS on motor performance, online tACS can significantly improve the motor performance of healthy individuals (Figure 5). No significant effect was observed on offline tACS for motor performance, which indicated that the stimulation mode was the main influencing factor in the study of tACS on motor performance.
At present, it remains controversial whether tACS plays a role during or after stimulation (post-effect) (Fertonani et al., 2017; Samaei et al., 2017; Galli et al., 2019). TMS-induced motor-evoked potential (MEP) measurement of tACS online and offline effects has been widely recognized (Pozdniakov et al., 2021). Growing evidence has confirmed the frequency-specific online effect of tACS through TMS-induced MEP (Feurra et al., 2011; Feurra et al., 2013; Shpektor et al., 2017; Feurra et al., 2019). For example, Feurra et al. explored the effect of online tACS stimulation at different frequencies on the spinal cord excitability of M1 via TMS and found that 20 Hz tACS stimulation increased the excitability of the cortical spinal cord, while 5, 10, and 40 Hz had no effect on MEP (Feurra et al., 2011), which was also verified in Feurra et al.‘s two studies (Feurra et al., 2013; Feurra et al., 2019). In addition, no consistent conclusion was derived on offline tACS. Antal et al. explored the changes in spinal cord excitability of the motor cortex through TMS-induced MEP, and no significant change was found in spinal cord excitability when stimulated by tACS at 1, 10, 15, 30, and 45 Hz after intergroup analysis (Antal et al., 2008). Heise et al. evaluated spinal cord excitability before, during, and after stimulation at 20 Hz tACS and found that spinal cord excitability increased significantly during immediately after stimulation (Heise et al., 2016). These results show that tACS stimulation seems to have a certain timeliness. Hence, although online tACS achieves a significant improvement in motor performance, future research still needs to focus on the timeliness of tACS, which will have far-reaching significance for the standardization of clinical application.
In terms of motor learning, as shown in Figure 7, a significant improvement was found in healthy individuals. The analysis included only four datasets of two studies; thus, the lack of sample size was a key problem. In addition, inconsistent with a previous study, only one of the four datasets was γ-tACS, which made determining the role of tACS in motor learning ability impossible. Sugata et al. and Giustiniani showed the potential of the high-frequency band γ-tACS on sequential learning tasks (Sugata et al., 2018; Giustiniani et al., 2019). Transcranial electrical stimulation (TES) has a good regulating effect on neuroplasticity (Galea et al., 2009; Zuchowski et al., 2014; Wessel et al., 2016), and tACS can exert a more selective effect on target neurons, so that the “characteristic frequency” of neurons tends to the stimulation frequency (Antal et al., 2016; Naro et al., 2017). In addition, previous studies have found that oscillatory activity in gamma and beta bands plays an important role in motor learning (Pollok et al., 2014; Pollok et al., 2015; Naro et al., 2016; Naro et al., 2017; Nowak et al., 2017; Wessel et al., 2020). These oscillatory activities are thought to be induced by the activation of excitatory glutamatergic cells and inhibitory GABAergic interneurons in M1 (Buzsáki et al., 2012; Guerra et al., 2016). Among them, Naro and Wessel found that a 50 Hz stimulation frequency can promote motor learning ability (Naro et al., 2016; Naro et al., 2017; Wessel et al., 2020). In accordance with a previous study, α and β-tACSs could significantly improve motor learning ability (Pollok et al., 2014; Sugata et al., 2014; Pollok et al., 2015; Krause et al., 2016). Among them, alpha oscillations affect vision and sensorimotor activity (Sugata et al., 2014); beta oscillations affect motor performance and motor learning (Houweling et al., 2008; Sugata et al., 2014). In brief, γ-tACS should receive more attention for motor learning, especially the impact of frequency specificity.
5 Limitations
This systematic review and meta-analysis has several limitations. First, some methodological variables of this study were not standardized and unified, particularly the stimulation frequency and stimulation mode (online and offline), which may directly affect the accuracy and reliability of the results of this study. There were no significant findings in this area; thus, a more precise experimental design should be conducted to systematically standardize this problem in the future. Second, few quantitative analyses were included, which increased the incidence of false negative or false positive synthesis results. Therefore, the authors are cautious about the results of these tACS analyses on motor learning. More research evidence is needed to support our results in the future. Third, this study did not report the safety issues of tACS (skin sensation, phosphenes, other sensation, etc.). Thus, caution should be taken in the application of tACS in healthy people and even special populations. In a previous meta-analysis of tACS on cortical spinal cord excitability, the authors reported that tACS had certain effects on skin sensation and perceiving phosphenes in healthy adults (Wischnewski et al., 2019). Therefore, safe application of tACS in healthy people and even special populations is needed clinically. The physiological mechanism by which tDCS improves motor performance and motor learning is still unknown. At present, the most widely used technology to observe spinal cord excitability during stimulation is TMS-induced MEP, but this technology still has certain limitations. The authors suggest that future research should combine tACS technology with neuroimaging technology. For example, simultaneous use of electroencephalogram (EEG) with high temporal resolution can immediately explore brain changes during tACS stimulation and reveal the physiological mechanism of tACS. Combined with functional near-infrared spectroscopy (fNIRS), which has low movement requirements and small movement artifacts, it can meet more task designs and reveal the physiological mechanism of tACS application in certain groups (such as high-level athletes, dyskinesia patients, hyperkinesia patients, etc.).
6 Conclusion
This systematic review and meta-analysis found that online tACS and gamma band tACS can significantly improve motor performance. In addition, tACS has a certain effect on motor learning, but the authors remain cautious about this conclusion. In the future, more research evidence is needed to verify the efficacy of tACS on motor learning.
Abr7 Abbreviations: tACS-transcranial alternating current stimulation, M1-primary motor cortex, TMS-transcranial magnetic stimulation, RTT-reaction time task, SRTT-Serial reaction time task, SMD-standardized mean difference, SMA-supplementary motor area, SCPT-seated chest pass throw, SBOMBT-seated backward overhead medicine ball throw, SJ-squat jump, CMJ-counter-movement jump, CMJ-AS-counter-movement jump arm-swing, MEP-motor-evoked potential, TES-transcranial electrical stimulation, EEG-electroencephalogram, fNIRS-functional near-infrared spectroscopy, RCT-Randomized controlled trial, VCT-Visuomotor control task, PPT-Purdue Pegboard Test, MSLT-Motor sequence learning task, PT-Physical test, IFT-Isometric force task, VMT-Visuomotor tracking, ER-Error rate, RT-Reaction time.
Data availability statement
The original contributions presented in the study are included in the article/supplementary materials, further inquiries can be directed to the corresponding author.
Author contributions
This study was designed by KH, and RW, MN performed the data extraction. JG made the tables and figures. YL polished the language. The manuscript was written by KH and FG. All authors commented on the manuscript.
Funding
This work was supported by the Natural Science Foundation of Liaoning Province (2022-MS-415), Scientific Research Project of Liaoning Education Department (LQN2019ST02) and Key Project of Liaoning Province Social Science Planning Fund (L21ZD045).
Acknowledgments
I would like to appreciate and thank my dear supervisor FG for his time. This manuscript could not have been completed without his efforts.
Conflict of interest
The authors declare that the research was conducted in the absence of any commercial or financial relationships that could be construed as a potential conflict of interest.
Publisher’s note
All claims expressed in this article are solely those of the authors and do not necessarily represent those of their affiliated organizations, or those of the publisher, the editors and the reviewers. Any product that may be evaluated in this article, or claim that may be made by its manufacturer, is not guaranteed or endorsed by the publisher.
References
Antal A., Boros K., Poreisz C., Chaieb L., Terney D., Paulus W. (2008). Comparatively weak after-effects of transcranial alternating current stimulation (tACS) on cortical excitability in humans. Brain Stimul. 1 (2), 97–105. doi:10.1016/j.brs.2007.10.001
Antal A., Herrmann C. S. (2016). Transcranial alternating current and random noise stimulation: Possible mechanisms. Neural Plast. 2016, 3616807. doi:10.1155/2016/3616807
Buzsáki G., Wang X. J. (2012). Mechanisms of gamma oscillations. Annu. Rev. Neurosci. 35, 203–225. doi:10.1146/annurev-neuro-062111-150444
Del Felice A., Castiglia L., Formaggio E., Cattelan M., Scarpa B., Manganotti P., et al. (2019). Personalized transcranial alternating current stimulation (tACS) and physical therapy to treat motor and cognitive symptoms in Parkinson's disease: A randomized cross-over trial. Neuroimage. Clin. 22, 101768. doi:10.1016/j.nicl.2019.101768
Elyamany O., Leicht G., Herrmann C. S., Mulert C. (2021). Transcranial alternating current stimulation (tACS): From basic mechanisms towards first applications in psychiatry. Eur. Arch. Psychiatry Clin. Neurosci. 271 (1), 135–156. doi:10.1007/s00406-020-01209-9
Fertonani A., Miniussi C. (2017). Transcranial electrical stimulation: What we know and do not know about mechanisms. Neuroscientist 23 (2), 109–123. doi:10.1177/1073858416631966
Feurra M., Bianco G., Santarnecchi E., Del Testa M., Rossi A., Rossi S. (2011). Frequency-dependent tuning of the human motor system induced by transcranial oscillatory potentials. J. Neurosci. 31 (34), 12165–12170. doi:10.1523/JNEUROSCI.0978-11.2011
Feurra M., Blagovechtchenski E., Nikulin V. V., Nazarova M., Lebedeva A., Pozdeeva D., et al. (2019). State-dependent effects of transcranial oscillatory currents on the motor system during action observation. Sci. Rep. 9 (1), 12858. doi:10.1038/s41598-019-49166-1
Feurra M., Pasqualetti P., Bianco G., Santarnecchi E., Rossi A., Rossi S. (2013). State-dependent effects of transcranial oscillatory currents on the motor system: What you think matters. J. Neurosci. 33 (44), 17483–17489. doi:10.1523/JNEUROSCI.1414-13.2013
Galea J. M., Jayaram G., Ajagbe L., Celnik P. (2009). Modulation of cerebellar excitability by polarity-specific noninvasive direct current stimulation. J. Neurosci. 29 (28), 9115–9122. doi:10.1523/JNEUROSCI.2184-09.2009
Galli G., Vadillo M. A., Sirota M., Feurra M., Medvedeva A. (2019). A systematic review and meta-analysis of the effects of transcranial direct current stimulation (tDCS) on episodic memory. Brain Stimul. 12 (2), 231–241. doi:10.1016/j.brs.2018.11.008
Giustiniani A., Battaglia G., Messina G., Morello H., Guastella S., Iovane A., et al. (2021). Transcranial alternating current stimulation (tACS) does not affect sports people's explosive power: A pilot study. Front. Hum. Neurosci. 15, 640609. doi:10.3389/fnhum.2021.640609
Giustiniani A., Tarantino V., Bonaventura R. E., Smirni D., Turriziani P., Oliveri M. (2019). Effects of low-gamma tACS on primary motor cortex in implicit motor learning. Behav. Brain Res. 376, 112170. doi:10.1016/j.bbr.2019.112170
Guerra A., Pogosyan A., Nowak M., Tan H., Ferreri F., Di Lazzaro V., et al. (2016). Phase dependency of the human primary motor cortex and cholinergic inhibition cancelation during beta tACS. Cereb. Cortex 26 (10), 3977–3990. doi:10.1093/cercor/bhw245
Heise K. F., Kortzorg N., Saturnino G. B., Fujiyama H., Cuypers K., Thielscher A., et al. (2016). Evaluation of a modified high-definition electrode montage for transcranial alternating current stimulation (tACS) of pre-central areas. Brain Stimul. 9 (5), 700–704. doi:10.1016/j.brs.2016.04.009
Herrmann C. S., Rach S., Neuling T., Strüber D. (2013). Transcranial alternating current stimulation: A review of the underlying mechanisms and modulation of cognitive processes. Front. Hum. Neurosci. 7, 279. doi:10.3389/fnhum.2013.00279
Higgins J., Green S. R. (2011). Cochrane handbook for systematic reviews of interventions. Chichester, England.Cochrane collaboration
Houweling S., Daffertshofer A., van Dijk B. W., Beek P. J. (2008). Neural changes induced by learning a challenging perceptual-motor task. Neuroimage 41 (4), 1395–1407. doi:10.1016/j.neuroimage.2008.03.023
Joundi R. A., Jenkinson N., Brittain J. S., Aziz T. Z., Brown P. (2012). Driving oscillatory activity in the human cortex enhances motor performance. Curr. Biol. 22 (5), 403–407. doi:10.1016/j.cub.2012.01.024
Krause V., Meier A., Dinkelbach L., Pollok B. (2016). Beta band transcranial alternating (tACS) and direct current stimulation (tDCS) applied after initial learning facilitate retrieval of a motor sequence. Front. Behav. Neurosci. 10, 4. doi:10.3389/fnbeh.2016.00004
Li R., Du J., Yang K., Wang X., Wang W. (2022). Effectiveness of motor imagery for improving functional performance after total knee arthroplasty: A systematic review with meta-analysis. J. Orthop. Surg. Res. 17 (1), 65. doi:10.1186/s13018-022-02946-4
Ma R., Xia X., Zhang W., Lu Z., Wu Q., Cui J., et al. (2021). High gamma and beta temporal interference stimulation in the human motor cortex improves motor functions. Front. Neurosci. 15, 800436. doi:10.3389/fnins.2021.800436
Miyaguchi S., Inukai Y., Mitsumoto S., Otsuru N., Onishi H. (2022). Gamma-transcranial alternating current stimulation on the cerebellum and supplementary motor area improves bimanual motor skill. Behav. Brain Res. 424, 113805. doi:10.1016/j.bbr.2022.113805
Miyaguchi S., Otsuru N., Kojima S., Saito K., Inukai Y., Masaki M., et al. (2018). Transcranial alternating current stimulation with gamma oscillations over the primary motor cortex and cerebellar hemisphere improved visuomotor performance. Front. Behav. Neurosci. 12, 132. doi:10.3389/fnbeh.2018.00132
Miyaguchi S., Otsuru N., Kojima S., Yokota H., Saito K., Inukai Y., et al. (2019a). Gamma tACS over M1 and cerebellar hemisphere improves motor performance in a phase-specific manner. Neurosci. Lett. 694, 64–68. doi:10.1016/j.neulet.2018.11.015
Miyaguchi S., Otsuru N., Kojima S., Yokota H., Saito K., Inukai Y., et al. (2019b). The effect of gamma tACS over the M1 region and cerebellar hemisphere does not depend on current intensityEffects of stimulating the supplementary motor area with a transcranial alternating current for bimanual movement performance. Behav. Brain Res.Behav Brain Res. 65393, 54112801–54112858. doi:10.1016/j.jocn.2019.03.045Miyaguchi10.1016/j.bbr.2020.112801
Moher D., Liberati A., Tetzlaff J., Altman D. G. (2009). Preferred reporting items for systematic reviews and meta-analyses: The PRISMA statement. PLoS Med. 6 (7), e1000097. doi:10.1371/journal.pmed.1000097
Moisa M., Polania R., Grueschow M., Ruff C. C. (2016). Brain network mechanisms underlying motor enhancement by transcranial entrainment of gamma oscillations. J. Neurosci. 36 (47), 12053–12065. doi:10.1523/JNEUROSCI.2044-16.2016
Muthukumaraswamy S. D. (2011). Temporal dynamics of primary motor cortex γ oscillation amplitude and piper corticomuscular coherence changes during motor control. Exp. Brain Res. 212 (4), 623–633. doi:10.1007/s00221-011-2775-z
Naro A., Bramanti A., Leo A., Manuli A., Sciarrone F., Russo M., et al. (2017). Effects of cerebellar transcranial alternating current stimulation on motor cortex excitability and motor function. Brain Struct. Funct. 222 (6), 2891–2906. doi:10.1007/s00429-016-1355-1
Naro A., Leo A., Russo M., Cannavò A., Milardi D., Bramanti P., et al. (2016). Does transcranial alternating current stimulation induce cerebellum plasticity? Feasibility, safety and efficacy of a novel electrophysiological approach. Brain Stimul. 9 (3), 388–395. doi:10.1016/j.brs.2016.02.005
Nowak M., Hinson E., van Ede F., Pogosyan A., Guerra A., Quinn A., et al. (2017). Driving human motor cortical oscillations leads to behaviorally relevant changes in local GABA(A) inhibition: A tACS-TMS study. J. Neurosci. 37 (17), 4481–4492. doi:10.1523/JNEUROSCI.0098-17.2017
Orssatto L., Borg D. N., Pendrith L., Blazevich A. J., Shield A. J., Trajano G. S. (2022). Do motoneuron discharge rates slow with aging? A systematic review and meta-analysis. Mech. Ageing Dev. 203, 111647. doi:10.1016/j.mad.2022.111647
Pogosyan A., Gaynor L. D., Eusebio A., Brown P. (2009). Boosting cortical activity at Beta-band frequencies slows movement in humans. Curr. Biol. 19 (19), 1637–1641. doi:10.1016/j.cub.2009.07.074
Pollok B., Boysen A. C., Krause V. (2015). The effect of transcranial alternating current stimulation (tACS) at alpha and beta frequency on motor learning. Behav. Brain Res. 293, 234–240. doi:10.1016/j.bbr.2015.07.049
Pollok B., Latz D., Krause V., Butz M., Schnitzler A. (2014). Changes of motor-cortical oscillations associated with motor learning. Neuroscience 275, 47–53. doi:10.1016/j.neuroscience.2014.06.008
Pozdniakov I., Vorobiova A. N., Galli G., Rossi S., Feurra M. (2021). Online and offline effects of transcranial alternating current stimulation of the primary motor cortex. Sci. Rep. 11 (1), 3854. doi:10.1038/s41598-021-83449-w
Rumpf J. J., Barbu A., Fricke C., Wegscheider M., Classen J. (2019). Posttraining alpha transcranial alternating current stimulation impairs motor consolidation in elderly people. Neural Plast. 2019, 2689790. doi:10.1155/2019/2689790
Samaei A., Ehsani F., Zoghi M., Hafez Yosephi M., Jaberzadeh S. (2017). Online and offline effects of cerebellar transcranial direct current stimulation on motor learning in healthy older adults: A randomized double-blind sham-controlled study. Eur. J. Neurosci. 45 (9), 1177–1185. doi:10.1111/ejn.13559
Santarnecchi E., Biasella A., Tatti E., Rossi A., Prattichizzo D., Rossi S., et al. (2017). High-gamma oscillations in the motor cortex during visuo-motor coordination: A tACS interferential study. Brain Res. Bull. 131, 47–54. doi:10.1016/j.brainresbull.2017.03.006
Schwab B. C., Misselhorn J., Engel A. K. (2019). Modulation of large-scale cortical coupling by transcranial alternating current stimulation. Brain Stimul. 12 (5), 1187–1196. doi:10.1016/j.brs.2019.04.013
Shpektor A., Nazarova M., Feurra M. (2017). Effects of transcranial alternating current stimulation on the primary motor cortex by online combined approach with transcranial magnetic stimulation. J. Vis. Exp. 127. doi:10.3791/55839
Sugata H., Hirata M., Yanagisawa T., Shayne M., Matsushita K., Goto T., et al. (2014). Alpha band functional connectivity correlates with the performance of brain-machine interfaces to decode real and imagined movements. Front. Hum. Neurosci. 8, 620. doi:10.3389/fnhum.2014.00620
Sugata H., Yagi K., Yazawa S., Nagase Y., Tsuruta K., Ikeda T., et al. (2018). Modulation of motor learning capacity by transcranial alternating current stimulation. Neuroscience 391, 131–139. doi:10.1016/j.neuroscience.2018.09.013
Urrútia G., Bonfill X. (2010). PRISMA declaration: A proposal to improve the publication of systematic reviews and meta-analyses. Med. Clin. 135 (11), 507–511. doi:10.1016/j.medcli.2010.01.015
Weinrich C. A., Brittain J. S., Nowak M., Salimi-Khorshidi R., Brown P., Stagg C. J. (2017). Modulation of long-range connectivity patterns via frequency-specific stimulation of human cortex. Curr. Biol. 27 (19), 3061–3068. e3. doi:10.1016/j.cub.2017.08.075
Wessel M. J., Draaisma L. R., de Boer A. F. W., Park C. H., Maceira-Elvira P., Durand-Ruel M., et al. (2020). Cerebellar transcranial alternating current stimulation in the gamma range applied during the acquisition of a novel motor skill. Sci. Rep. 10 (1), 11217. doi:10.1038/s41598-020-68028-9
Wessel M. J., Zimerman M., Timmermann J. E., Heise K. F., Gerloff C., Hummel F. C. (2016). Enhancing consolidation of a new temporal motor skill by cerebellar noninvasive stimulation. Cereb. Cortex 26 (4), 1660–1667. doi:10.1093/cercor/bhu335
Wiegel P., Elizabeth Spedden M., Ramsenthaler C., Malling Beck M., Lundbye-Jensen J. (2022). Trial-to-trial variability and cortical processing depend on recent outcomes during human reinforcement motor learning. Neuroscience 501, 85–102. doi:10.1016/j.neuroscience.2022.08.012
Wischnewski M., Schutter D., Nitsche M. A. (2019). Effects of beta-tACS on corticospinal excitability: A meta-analysis. Brain Stimul. 12 (6), 1381–1389. doi:10.1016/j.brs.2019.07.023
Wu L., Liu T., Wang J. (2021). Improving the effect of transcranial alternating current stimulation (tACS): A systematic review. Front. Hum. Neurosci. 15, 652393. doi:10.3389/fnhum.2021.652393
Yamaguchi T., Svane C., Forman C. R., Beck M. M., Geertsen S. S., Lundbye-Jensen J., et al. (2020). Transcranial alternating current stimulation of the primary motor cortex after skill acquisition improves motor memory retention in humans: A double-blinded sham-controlled study. Cereb. Cortex Commun. 1 (1), tgaa047. doi:10.1093/texcom/tgaa047
Zhou W., Kruse E. A., Brower R., North R., Joiner W. M. (2022). Motion state-dependent motor learning based on explicit visual feedback is quickly recalled, but is less stable than adaptation to physical perturbations. J. Neurophysiol. 128, 854–871. doi:10.1152/jn.00520.2021
Keywords: transcranial alternating current stimulation, motor performance, motor learning, healthy individuals, frequency specificity
Citation: Hu K, Wan R, Liu Y, Niu M, Guo J and Guo F (2022) Effects of transcranial alternating current stimulation on motor performance and motor learning for healthy individuals: A systematic review and meta-analysis. Front. Physiol. 13:1064584. doi: 10.3389/fphys.2022.1064584
Received: 08 October 2022; Accepted: 07 November 2022;
Published: 18 November 2022.
Edited by:
Gianpiero Greco, University of Bari Aldo Moro, ItalyReviewed by:
Junhong Zhou, Harvard Medical School, United StatesJozef Simenko, University of Hertfordshire, United Kingdom
Copyright © 2022 Hu, Wan, Liu, Niu, Guo and Guo. This is an open-access article distributed under the terms of the Creative Commons Attribution License (CC BY). The use, distribution or reproduction in other forums is permitted, provided the original author(s) and the copyright owner(s) are credited and that the original publication in this journal is cited, in accordance with accepted academic practice. No use, distribution or reproduction is permitted which does not comply with these terms.
*Correspondence: Feng Guo, Z3VvZmVuZ19maXJzdEAxNjMuY29t