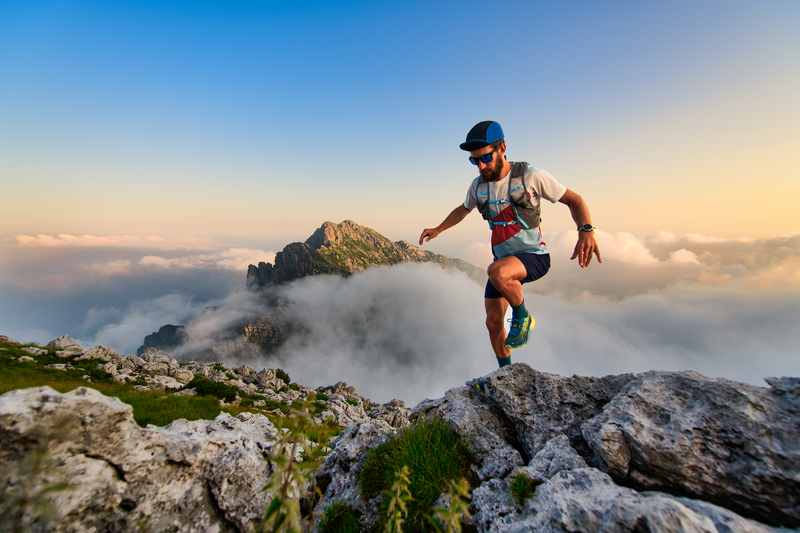
95% of researchers rate our articles as excellent or good
Learn more about the work of our research integrity team to safeguard the quality of each article we publish.
Find out more
ORIGINAL RESEARCH article
Front. Physiol. , 04 January 2023
Sec. Developmental Physiology
Volume 13 - 2022 | https://doi.org/10.3389/fphys.2022.1055234
This article is part of the Research Topic Women in Developmental Physiology: 2022 View all 7 articles
Clinically, fetal growth restriction (FGR) is only detectable in later gestation, despite pathophysiological establishment likely earlier in pregnancy. Additionally, there are no effective in utero treatment options for FGR. We have developed a nanoparticle to deliver human insulin-like 1 growth factor (hIGF-1) in a trophoblast-specific manner which results in increased expression of hIGF-1. IGF-1 signaling in the placenta regulates multiple developmental processes including trophoblast invasion and maternal vascular remodeling, both of which can be diminished in the FGR placenta. We aimed to determine the effects of short-term hIGF-1 nanoparticle treatment on sub-placenta/decidua trophoblast signaling mechanisms in FGR and under normal growth conditions. Using the guinea pig maternal nutrient restriction (MNR) model of FGR, ultrasound-guided, intra-placenta injections of hIGF-1 nanoparticle were performed at gestational day 30–33, and dams sacrificed 5 days later. Sub-placenta/decidua tissue was separated from placenta for further analyses. Western blot was used to analyze protein expression of ERK/AKT/mTOR signaling proteins (phospho-Erk (pERK), phospho-Akt (pAKT), raptor, rictor and deptor). qPCR was used to analyze gene expression of vascular/remodeling factors [vascular endothelial growth factor (Vegf), placenta growth factor (Pgf), platelet-derived growth factor (Pdgf)) and tight junction/adhesion proteins (claudin 5 (Cldn5), p-glycoprotein (Abcb1), occludin (Ocln) and tight junction protein 1 (Zo1)]. MNR reduced expression of pERK, PdgfB and Cldn5, and increased expression of Ocln and Zo1 in the sub-placenta/decidua. In MNR + hIGF1 nanoparticle sub-placenta/decidua, expression of PdgfB, Ocln and Zo1 was normalized, whilst pAkt, VegfB, Vegf receptor 1 and PdgfB receptor were increased compared to MNR. In contrast, hIGF-1 nanoparticle treatment of normal placentas reduced expression of pERK, raptor and increased expression of the mTOR inhibitor deptor. This was associated with reduced expression of VegfA, Plgf, and PdgfB. Here we have shown that the impact of hIGF-1 nanoparticle treatment is dependent on pregnancy environment. Under MNR/FGR, hIGF-1 nanoparticle treatment triggers increased expression of growth factors and normalization of EMT factors. However, under normal conditions, the response of the placenta is to decrease AKT/mTOR signaling and growth factor expression to achieve homeostasis.
Fetal growth restriction (FGR; estimated fetal weight <10th percentile), is a significant risk factor for stillbirth and contributes significantly to infant morbidity and mortality (Bernstein, Horbar et al., 2000). Currently, there is no effective in utero treatment for FGR, which in part, is due to the fact that whilst the pathophysiology of FGR is established in the first half of pregnancy, clinically FGR is only detectable in later gestation (Sun, Groom et al., 2020).
Fetal growth increases exponentially throughout the latter half of pregnancy (Reynolds and Redmer 1995), and increased uterine blood flow is essential to meet metabolic demands from the growing fetus, as well as uterus and placenta (Bulmer, Burton et al., 2012). Increased uterine blood flow is supported in part in humans, by properly remodeled maternal spiral arteries coordinated by invasion of extravillous trophoblasts (EVTs) (Burton and Fowden 2015). Aberrations in invasion can limit remodeling and hence, blood flow from the mother to the maternal-fetal interface, restricting nutrient and oxygen delivery and contributing to FGR (Brosens, Dixon et al., 1977; Kaufmann, Black et al., 2003). The guinea pig model offers several advantages including similar developmental milestones through gestation, a haemomonochorial placenta, and deep trophoblast invasion-all similar to humans. They also have a longer gestation (∼65 days) than rodent and rabbit models (Carter, Enders et al., 2006; Mess 2007). The guinea pig maternal nutrient restriction (MNR) model is an established model to study the effects of FGR on fetal development, pregnancy outcome and the developmental origins of health and disease (Kunkele and Trillmich 1997; Morrison, Botting et al., 2018). Moderate food restriction prior to and throughout pregnancy results in reduced fetal and placental weight from mid gestation (Sohlstrom, Katsman et al., 1998) without significantly affecting fertility (Elias, Ghaly et al., 2016). Most importantly, placental development in the guinea pig includes trophoblast invasion, which is not present in other small animal models (Mess 2007). A defining characteristic of the guinea pig, and other caviamorphs’, placenta is the sub-placenta (Carter, Enders et al., 2006). This region, distinct from the chorioallantoic placenta, is comprised of trophoblasts arranged in folded layers encircled by syncytial trophoblast, and the source of invasive trophoblasts (Verkeste, Slangen et al., 1998). These invasive trophoblasts are the source of interstitial invasion into the decidua, and into the arterial walls similar to human placentation (Carter, Enders et al., 2006), making guinea pigs ideal for identifying mechanisms involved in trophoblast invasion.
Some of the central signaling cascades that regulate trophoblast proliferation and invasion are the ERK and AKT signaling pathways (Knöfler and Pollheimer 2012). These are well studied kinase pathways involved in cell survival, proliferation, and invasion that are activated through phosphorylation after a ligand, such as IGF-1, binds to its receptor and activates its kinase domain(Rieger and O’Connor 2021). ERK and AKT signaling activate extracellular matrix breakdown, adhesion molecule reduction for epithelial-mesenchymal transition (EMT) and invasion, and mTOR/raptor/rictor activation for proliferation through regulation of AKT. Raptor and Rictor both positively regulate AKT, while deptor negatively regulates(Chakraborty, Gleeson et al., 2002; Xu, Yang et al., 2015; Crespo et al., 2016). Each of these pathways are critical for EVTs to invade into the maternal tissue. Moreover, trophoblast invasion can be intrinsically and extrinsically influenced by growth factors, adhesion and tight junction molecules as well as extracellular matrix components (Lunghi, Ferretti et al., 2007). Most studied of these growth factors are the vascular endothelial growth factor (VEGF) proteins which include placental growth factor (PGF), and regulate placental development through binding with their specific transmembrane receptors, including VEGFR-1 (Flt-1) and VEGFR-2 (KDR) (Shore, Wang et al., 1997). These growth factors can stimulate these same pathways, like that of IGF-1 binding, as well as they themselves being correlated with changes in IGF-1 expression, in particular, IGF-1 being known to induce VEGF expression (Reinmuth, Fan et al., 2002; Slomiany and Rosenzweig 2004).
Tight junction and adhesion proteins are critical components in determining epithelial cell polarity. These tight junction proteins, such as claudin5, occludin, and tight junction protein 1 (ZO-1), must be repressed for cells to go through EMT to reduce cell adhesion and increase cell mobility (migration/invasion) (Ikenouchi, Matsuda et al., 2003). Claudin protein expression has been shown to increase cell proliferation, invasion, and migration of EVTs in vitro. Through activation of the ERK1/2 pathway, claudin proteins upregulate matrix-metalloproteinases to help break down the extracellular matrix (ECM) as they invade into the maternal tissue and control permeability. mRNA levels of these claudin proteins have also been shown to be down in cases of hypertension and/or preeclampsia, suggesting a role in placental dysfunction (Zhao, Qi et al., 2020). During normal placenta development ZO-1 and occludin are expressed in the proliferating trophoblasts and down regulation of these proteins is associated with the epithelial cell transformation that is necessary for proper invasion of EVTs (Marzioni, Banita et al., 2001). P-glycoprotein is also down regulated in cases of preeclampsia and functionally important for EVT invasion (Ceckova-Novotna, Pavek et al., 2006; Dunk, Pappas et al., 2018).
Insulin-like 1 growth factor (IGF-1) is a known activator of the ERK and AKT signaling cascades in the maternal fetal interface (Dumolt, Powell et al., 2021). Expressed in placental and fetal tissue from the pre-implantation period through to birth (Fowden 2003), IGF-1 is known to regulate trophoblast invasion through binding with the IGF-1 receptor (IGF-1R). Fetal growth positively correlates with maternal IGF-1 concentrations (Chellakooty, Vangsgaard et al., 2004), and both maternal serum levels of IGF-1 and placenta expression of IGF-1R are decreased in FGR (Holmes, Montemagno et al., 1997; Laviola, Perrini et al., 2005). Additionally, we have previously shown that treatment of the placenta with a non-viral, polymer based, nanoparticle gene therapy that specifically increases human IGF-1 (hIGF-1) in trophoblast, maintains fetal growth in a surgically-ligated FGR mouse model, and increases nutrient transporter expression (Abd Ellah, Taylor et al., 2015; Wilson, Owens et al., 2020; Wilson, Lampe et al., 2022). Placenta treatment with the hIGF-1 nanoparticle does not adversely impact maternal or fetal outcomes and cannot cross the placenta into fetal circulation (Wilson, Lampe et al., 2022; Wilson, Stephens et al., 2021). We have previously demonstrated that short-term treatment with the hIGF-1 nanoparticle results in increased hIGF-1 expression in trophoblasts of the guinea pig sub-placenta/decidua (Wilson, Lampe et al., 2022).
In the present study, we determined the effects of short-term hIGF-1 nanoparticle treatment on trophoblast ERK and AKT signaling, and downstream effectors that regulate trophoblast function and survival in both normal and FGR conditions. We hypothesize that maternal nutrient restriction (MNR) alters trophoblast proliferation and invasion through changes in ERK and AKT activation. Increased hIGF-1 expression with placental nanoparticle gene therapy is hypothesized to increase activation of ERK and AKT signaling resulting in downstream changes relating to ECM breakdown, and tight junction and ECM protein expression that will improve trophoblast function.
All experiments were approved by the Cincinnati Children’s Hospital Medical Center and University of Florida Institutional Animal Care and Use Committee (Protocol numbers 2017-0065 and 202011236, respectively). Female Dunkin-Hartley guinea pigs were purchased (Charles River Laboratories, Wilmington, MA) at 500–550 g and housed in an environmentally controlled room (22°C/72°F and 50% humidity) under a 12 h light-dark cycle. Upon arrival, food (LabDiet diet 5025: 27% protein, 13.5% fat, and 60% carbohydrate as % of energy) and water were provided ad libitum. After a 2-week acclimatization period, females were weighed and assigned to either an ad libitum fed diet (termed Control: n = 11) or maternal nutrient restriction (MNR) diet (n = 12), as previously described (Sohlstrom, Katsman et al., 1998; Elias, Maki et al., 2017). Time mating, pregnancy confirmation ultrasounds, and ultrasound-guided, transcutaneous, intra-placental nanoparticle treatment were performed as previously described (Wilson, Lampe et al., 2022). Briefly, nanoparticles were formed by complexing plasmids containing the hIGF-1 gene under the control of the placental trophoblast specific promotor CYP19A1 with a non-viral PHPMA115-b-PDMEAMA115 co-polymer (50 µg plasmid in a 200 µL injection volume) (Wilson, Lampe et al., 2022). At GD30-33, females underwent a nanoparticle injection of plasmid containing hIGF-1 (Control + hIGF-1 n = 4 or MNR + hIGF-1 n = 7), or PBS sham injection (Control n = 7 or MNR n = 5), and were then sacrificed 5 days after injection (GD35-38). We have previously shown in mice placentas in vivo, robust mRNA expression of plasmid-specific hIGF-1 at 96 h after injection (Abd Ellah, Taylor et al., 2015). Therefore, we chose to sacrifice all dams 5 days after injection (GD35-38) to ensure that transient expression of plasmid hIGF-1 was still present within the placenta. At time of sacrifice, major maternal and fetal organs were collected and weighed; fetal sex was also determined by visual inspection and confirmed using PCR as previously described (Depreux, Czech et al., 2018; Wilson, Stephens et al., 2021). Placentas were halved and either fixed 4% w/v paraformaldehyde (PFA) or the labyrinth and sub-placenta/decidua tissue separated, and snap-frozen in liquid nitrogen and stored at −80°C.
Approximately 150–200 mg of snap frozen placenta labyrinth and sub-placenta/decidua tissue was lysed and homogenized in RLT-lysis buffer (Qiagen) using a tissue homogenizer. RNA was extracted and DNase treated using the RNeasy Midi Kit (Qiagen) following standard manufacturers protocols. The High-capacity cDNA Reverse Transcription kit (Applied Biosystems) was used to convert 1 µg RNA to cDNA, and qPCR reactions were set up and performed on the StepOne-Plus Real-Time PCR System (Applied Biosystems) as previously described (Wilson, Lampe et al., 2022). Commercially available KiCqStart (Sigma) primers were used to measure mRNA expression of growth factors, their binding partners, and their receptors: VegfA, Pgf, PdgfB, VegfB, VegfR1, and PdgfBR. Tight junction and ECM mRNAs were also measured including: claudin5, p-glycoprotein/abcb1, occludin, and zo-1. Relative mRNA expression was calculated using the comparative CT method with the StepOne Software v2.3 (Applied Biosystems).
Following fixation in 4% PFA, placenta tissue was processed, and paraffin embedded following standard protocols. 5 µm thick tissue sections were obtained using a microtome (Leica), de-waxed and rehydrated as standard. Targeted antigen retrieval was performed by boiling sections in 1x IHC antigen retrieval solution (Invitrogen) for 15–20 min, followed by a 20 min cooling period at room temperature. Incubation in 3% hydrogen peroxidase solution for 10 min was used to suppress endogenous peroxidase activity, and a protein block of 10% goat serum with 1% bovine serum albumin (BSA; Jackson ImmunoResearch) was then applied for 1 h at room temperature. Primary antibodies, Claudin5, P-glycoprotein, Occludin, Vimentin, and Cytokeratin-7, were diluted in 10% goat serum with 1% BSA and applied to sections overnight a 4°C (antibody manufacturer and concentration listed in Supplementary Table S1). Sections were then incubated with a biotinylated secondary antibody for 1 h at room temperature, amplified using the Vector ABC kit (Vector), and detected using DAB (Vector). Hematoxylin was used to counterstain nuclei. Details on antibodies and dilutions are supplied in Supplementary Table S1. Stained sections were visualized using the Axioscan scanning microscope (Zeiss), and representative images obtained using the Zen imaging software (Zeiss).
Sub-placenta/decidua tissue was homogenized in ice-cold RIPA buffer containing protease and phosphatase inhibitors and protein concentrations determined using the Pierce™ Coomassie Blue Assay kit (Thermo Scientific) following standard protocol. 40 µg of protein was then mixed with Nupage SDS Loading Buffer (Invitrogen) and Reducing Agent (Invitrogen) and denatured by heating at 95°C for 10 min. The lysates were then run on a 4%–12% Tris-Bis precast gel (Invitrogen) following manufacturers protocols and transferred onto nitrocellulose membranes using the Bolt Mini Electrophoresis unit (Invitrogen). Both a Prestain Protein ladder (PageRuler, Thermo Scientific) and Biotinylated Protein ladder (Cell Signaling) were included on the gels. Membranes were placed into 5% skim-milk in Tris-buffered Saline containing Tween 20 (TBS-T) and incubated overnight at 4°C. Primary antibodies for p-ERK, p-AKT, Raptor, Rictor, Deptor, Claudin5, p-glycoprotein/abcb1, and occludin (Supplementary Table S1) were then applied for 1–2 h at room temperature, the membranes washed 3 times in fresh TBS-T, and then further incubated with a HRP conjugated secondary (Cell Signaling) for 1 h at room temperature. During the secondary incubation, an anti-biotin antibody (Cell Signaling) was also applied to visualize the biotinylated protein ladder. Protein bands were visualized by chemiluminescence using SuperSignal West Femto Maximum Sensitivity Substrate (Thermo Scientific) on the Universal Imager (Biorad). Signal intensity of the protein bands were calculated using Image Lab software (version 6.1, Biorad) and normalized to β-actin.
All statistical analyses were performed using SPSS Statistics 27 software. Generalized estimating equations were used to determine differences between diet and nanoparticle treatment, with maternal environment treated as a random effect and gestational age as a covariate. Fetal sex was also included as a main effect but removed if no statistical significance (p ≤ .05) was determined. For statistically significant results, a Bonferroni post hoc analysis was performed. Results are reported as estimated marginal means ± standard error.
We have shown that sub-placenta/decidua expression of endogenous Igf1 is not altered by either diet or hIGF-1 nanoparticle treatment (Wilson, Lampe et al., 2022). Sub-placenta/decidua expression of Igf1 Receptor (Igf-1R), IGF Binding Protein 3 (IgfBP3) and Igf-2 were also not impacted by either diet or hIGF-1 nanoparticle treatment (all p > 0.05; Supplemental Figure S1).
IGF-1 binding to IGF-1R modifies gene expression and cellular function through the ERK/AKT signaling pathways. Both MNR and control + hIGF-1 sub-placenta/decidua demonstrated reduced expression of pERK compared to Control (p = 0.025 and p < 0.001, respectively; Figure 1A). When comparing MNR groups, there was no difference in pERK expression between MNR and MNR + hIGF-1. pAKT expression was not changed in Control + hIGF-1 sub-placenta/decidua, however expression increased in the MNR + hIGF-1 sub-placenta/decidua when compared to Control and MNR (p = 0.038; Figure 1B). Compared to Control, there was decreased protein expression of the mTORC1 component Raptor in Control + hIGF-1 sub-placenta/decidua (p < 0.01; Figure 1C). However, there was no difference in Raptor expression in the MNR and MNR + hIGF-1 when compared to Control (p > 0.05; Figure 1C), nor was there a change in the mTORC2 component Rictor (p > 0.05; Figure 1D). Protein expression of the mTOR inhibitor Deptor was increased in the Control + hIGF-1 and MNR + hIGF-1 sub-placenta/decidua compared to Control (p < .05 for both, respectively; Figure 1E).
FIGURE 1. Effect of maternal nutrient restriction (MNR) diet and hIGF-1 nanoparticle treatment on expression of Erk/Akt signaling proteins. (A) Protein expression of phospho-Erk (pErk) was reduced in Control + hIGF-1, MNR and MNR + hIGF-1 sub-placenta/decidua when compared to Control. (B) In MNR + hIGF-1 nanoparticle treatment there was increased expression of phospho-Akt (pAkt) compared to MNR, but there was no difference between Control + hIGF-1 and Control sub-placenta/decidua. (C) Compared to Control, there was decreased expression of Raptor in Control + hIGF-1 nanoparticle treatment, but no difference with MNR or MNR + hIGF-1 nanoparticle treatment. (D) There was no difference in the expression of Rictor across any groups. (E) hIGF-1 nanoparticle treatment increased expression of Deptor in both Control + hIGF-1 and MNR + hIGF-1 sub-placenta decidua when compared to Control. Representative western blots for each protein are shown below the graphs with the beta-actin loading control (45 kDa). Western blots for Raptor, Rictor and Deptor were performed on the same membrane, therefore the beta-actin loading control is the same. Samples loaded left to right: Control, Control + hIGF-1, MNR, MNR + hIGF-1. n = 4 Control dams (8 sub-placenta/decidua), 4 Control + hIGF-1 dams (8 sub-placenta/decidua) 4 MNR dams (8 sub-placenta/decidua) and 4 MNR + hIGF-1 dams (8 sub-placenta/decidua). Data are estimated marginal means ±95% confidence interval. p values calculated using generalized estimating equations with Bonferroni post hoc analysis. Different letters denote a significant difference of p ≤ .05.
Angiogenic growth factor mRNA in the sub-placenta/decidua was differentially regulated by hIGF-1 depending on maternal diet group (VegfA: p = 0.049, Pgf: p < 0.01, PdgfB: p < 0.01, VegfB: p < 0.001, PdgfBR: p = 0.035; Figure 2). VegfA, Pgf and PdgfB mRNA expression was decreased in Control + hIGF-1 sub-placenta/decidua (p < 0.05; Figures 2A–C) compared to Control. However, there was no difference in VegfA, Pgf or PdgfB expression in the MNR + hIGF sub-placenta-decidua compared to MNR. Conversely, expression of VegfB, VegfR1 and PdgfBR was increased in MNR + hIGF-1 sub-placenta/decidua compared to MNR.
FIGURE 2. Effect of maternal nutrient restriction (MNR) diet and hIGF-1 nanoparticle treatment on expression of angiogenic growth factors. Compared to Control, hIGF-1 nanoparticle treatment reduced mRNA expression of Vascular endothelial growth factor A (VegfA; (A) and Placenta growth factor (Pgf; (B) in control + hIGF-1 sub-placenta/decidua but was not different in MNR and MNR + hIGF-1 nanoparticle treatment sub-placenta/decidua. (C). Expression of Platelet-derived growth factor B (PdgfB) was decreased in Control + hIGF-1 and MNR sub-placenta/decidua compared to Control, and increased in MNR + hIGF-1 to normal. In MNR + hIGF-1 nanoparticle treatment, there was increased expression of VegfB (D), Vegf Receptor 1 (VegfR1; (E) and PdgfB Receptor (PdgfBR; (F) compared to all other groups. n = 6 Control dams (12 sub-placenta/decidua), 4 Control + hIGF-1 dams (12 sub-placenta/decidua) 5 MNR dams (10 sub-placenta/decidua) and 7 MNR + hIGF-1 dams (13 sub-placenta/decidua). Data are estimated marginal means ±95% confidence interval. p values calculated using generalized estimating equations with Bonferroni post hoc analysis. Different letters denote a significant difference of p ≤ .05.
IHC confirmed expression of Cldn5 in trophoblasts lining maternal spiral arteries within the sub-placenta/decidua (Figure 3A), Abcb1 in the cytotrophoblasts within the cell columns (Figure 3B), and Ocln in the trophoblast progenitor cells of the cell columns (Figure 3C). Cytokeratin 7 was used to confirm expression of Cldn5, Abcb1 and Ocln was localized to trophoblasts (Figures 3D,E). mRNA expression of Cldn5 was reduced in the MNR sub-placenta/decidua (p = 0.001; Figure 4A), but was not regulated by hIGF-1 treatment. In MNR + hIGF-1 gene expression of Abcb1 was increased when compared to all groups (p < 0.05; Figure 4B). Increased expression of Ocln and Zo1 was evident in MNR compared to Control but was normalized by hIGF-1 treatment (p = 0.01 and p = 0.036; Figures 4C, D, respectively). At the protein level, there was no difference in expression of Cldn5 with diet or hIGF-1 nanoparticle treatment (Figure 4E). hIGF-1 nanoparticle treatment increased protein expression of Abcb1 in Control + hIGF-1 and MNR sub-placenta/decidua compared to Control (p = 0.047 and p = 0.046, respectively; Figure 4F). There was no difference in protein levels of Ocln with either diet or hIGF-1 nanoparticle treatment.
FIGURE 3. Protein localization of epithelial-mesenchymal transition (EMT) factors in the sub-placenta/decidua. (A) Representative image of immunohistochemistry (IHC) staining of Claudin 5 in the sub-placenta/decidua trophoblasts (closed arrow head) that line maternal spiral arteries. (B) Representative image of IHC staining of Abcb1 (P-glycoprotein) in the sub-placenta/decidua cytotrophoblasts (closed arrow head) of the cell column. (C) Representative image of IHC staining of Occludin in the trophoblast progenitor cells in the cell columns (open arrow head). (D) Representative image of double label IHC staining for Cytokeratin (brown; closed arrow head), indicating trophoblast cells, and Vimentin (black: red asterisk), indicating blood vessels, of a maternal spiral artery. (E) Representative image of double label IHC staining for Cytokeratin (brown; closed arrow head), indicating cytotrophoblast cells, and Vimentin (black: red asterisk), indicating fetal blood vessels, of the sub-placenta cell column. Trophoblast progenitor cells in the cell column stained weakly for cytokeratin (open arrow head). Scale bar = 10 μm n = 3–5 control placentas.
FIGURE 4. Effect of maternal nutrient restriction (MNR) diet and hIGF-1 nanoparticle treatment on expression of epithelial-mesenchymal transition factors. (A). There was decreased expression of Claudin 5 (Cldn5) in MNR and MNR + hIGF-1 sub-placenta/decidua compared to Control and Control + hIGF-1 nanoparticle treatment (B). There was increased expression of P-glycoprotein (Abcb1) in MNR + hIGF-1 compared to Control, Control + hIGF-1 and MNR. (C) In MNR sub-placenta/decidua, there was increased expression of Occludin (Ocln; (C) and Tight junction protein 1 (Zo1; (D) compared to Control, but expression was decreased in MNR + hIGF-1 nanoparticle treatment to normal. (E) Neither diet or hIGF-1 nanoparticle treatment affected protein expression of Cldn5. (F) Protein expression of Abcb1 was increased in Control + hIGF-1 and MNR + hIGF-1 nanoparticle compared to control. (G) Neither diet or hIGF-1 nanoparticle treatment affected protein expression of Ocln. Representative western blots for each protein are shown below the graphs with the beta-actin loading control (45 kDa). Western blots for Cldn5 and Abcb1 were performed on the same membrane, therefore the beta-actin loading control is the same. Samples loaded, from left-right: Control, Control + hIGF-1, MNR, MNR + hIGF1. qPCR analysis: n = 6 Control dams (12 sub-placenta/decidua), 4 Control + hIGF-1 dams (12 sub-placenta/decidua) 5 MNR dams (10 sub-placenta/decidua) and 7 MNR + hIGF-1 dams (13 sub-placenta/decidua). Western blot: n = 4 Control dams (8 sub-placenta/decidua), 4 Control + hIGF-1 dams (8 sub-placenta/decidua) 4 MNR dams (8 sub-placenta/decidua) and 4 MNR + hIGF-1 dams (8 sub-placenta/decidua). Data are estimated marginal means ±95% confidence interval. p values calculated using generalized estimating equations with Bonferroni post hoc analysis. Different letters denote a significant difference of p ≤ .05.
The etiology of fetal growth restriction (FGR) is often associated with aberrant extravillous trophoblast (EVT) invasion which limits maternal blood flow and thus nutrient/oxygen availability (Abbas, Turco et al., 2020). The aim of the present study was to determine the effects of maternal nutrient restriction (MNR) on sub-placenta trophoblast signaling, and whether increasing hIGF-1 expression, with nanoparticle-mediated gene therapy impacted ERK/AKT signaling pathways and expression of factors involved in EMT and vascular remodeling. We show that MNR results in reduced levels of pERK in the sub-placenta/decidua and was associated with reduced gene expression of PdgfB, and increased expression of Ocln and Zo1. In MNR placentas treated with hIGF-1 nanoparticle, expression of PdgfB, Ocln and Zo1 was normalized, whilst expression of VegfB, Vegf-R1 and PdgfB-R was increased.
The ERK/AKT signaling pathways are complex, involving multiple protein families, and can be activated or inactivated through different phosphorylation mechanisms (Bhaskar and Hay 2007; Fremin, Saba-El-Leil et al., 2015). In the present study, MNR reduced the expression of pERK in the sub-placenta/decidua compared to Control but had no effect on pAKT expression. Homozygous knockout of ERK isoform 1 (Erk1−/−), results in little disruption to embryonic development, with fetuses born viable (Pages, Guerin et al., 1999), however, homozygous knockout of isoform 2 (Erk2−/−) results in embryonic lethality at E6.5 despite expression of ERK1 in the embryos (Hatano, Mori et al., 2003). Loss of a single Erk2 allele results in decreased placental weight, which is further exacerbated with disruption to Erk1 (Fremin, Saba-El-Leil et al., 2015), and emphasizes the role of placental ERK1/2 activity during gestation. pERK expression remained reduced compared to control in the MNR + hIGF-1 sub-placenta/decidua, however there was increased expression of pAKT with hIGF-1 nanoparticle treatment. This indicates the ability to manipulate ERK/AKT signaling in the dysfunctional placenta which is necessary for the changes in proliferation and invasion needed for proper placenta function.
In normal human pregnancies, EVT invasion of the maternal vasculature result in remodeling of the maternal spiral arteries into low resistance vessels which allows for delivery of high volumes of nutrient and oxygen rich maternal blood to enter the placenta and support adequate fetal growth (Boyd and Hamilton 1970). However, in pregnancies complicated by FGR, insufficient spiral artery remodeling is often a common feature of placental pathology (Burton, Woods et al., 2009). We have previously shown that hIGF-1 nanoparticle treatment of the placenta increases hIGF-1 in trophoblasts residing in the sub-placenta/decidua (Wilson, Lampe et al., 2022). These trophoblasts in the guinea pig placenta are responsible for communication with the maternal environment and remodeling of the maternal vasculature. Utero-Placental blood flow in the MNR guinea pig placenta remains to be determined, however, MNR decreased placental blood flow has been shown in rat MNR pregnancies (Ahokas, Anderson et al., 1983). In MNR + hIGF-1 sub-placenta/decidua, there was increased expression of pAKT which was associated with increased expression of VegfB, Vegf-R1 PdgfB, and PdgfB-R. VEGF and PDGFB are produced by the placenta and secreted into maternal circulation where they aid maternal adaptations to pregnancy like inducing vasodilation of resistance vessels (Chen, Liu et al., 2015; Cuffe, Holland et al., 2017). VEGF in particular, has been shown to act as a vasodilator for myometrial resistance arteries (Szukiewicz, Szewczyk et al., 2005) and is decreased in maternal serum of FGR pregnancies (Nadar, Karalis et al., 2005). Furthermore, VEGF and PDGFB play an important role in regulating the uterine immune response involved in regulating trophoblast invasion (Kalkunte, Mselle et al., 2009; Wang, Nian et al., 2021). Increased expression of VegfB, PdgfB and their receptors did not result in noticeable changes to the gross morphology of the sub-placenta/decidua examined by 2D histology (Wilson, Lampe et al., 2022). This may be due to the short time period between hIGF-1 nanoparticle treatment and sample collection, and with longer treatment may result in noticeable changes to trophoblast invasion and thus maternal spiral artery remodeling.
Tight junction and adhesion proteins, including claudins, occludins, and ZO-1, are critical components in determining epithelial cell polarity and are repressed in trophoblasts undergoing EMT prior to trophoblast invasion (Marzioni, Banita et al., 2001; Ikenouchi, Matsuda et al., 2003). In the guinea pig sub-placenta/decidua, Cldn5 was expressed specifically in trophoblasts lining the maternal spiral arteries, Abcb1 was expressed in the trophoblasts of the sub-placenta cell columns, and Ocln to the trophoblast progenitor cells. The cell columns of the guinea pig sub-placenta contain the progenitor trophoblast that become invasive trophoblast responsible for maternal vascular remodeling (Verkeste, Slangen et al., 1998). Under MNR conditions we demonstrated reduced Cldn5 expression, unsurprisingly since this has been shown to be down in cases of hypertension and preeclampia(Zhao, Qi et al., 2020; Adu-Gyamfi, Czika et al., 2021). Increased Ocln and Zo1 expression in the sub-placenta/decidua were seen suggesting strong cell adhesion and tight junctions which would not be conducive to EMT for proper invasion. However, hIGF-1 expression in the MNR sub-placenta/decidua resulted in normalization of Ocln and Zo1 levels to that of Control, and expression of Abcb1 was increased compared to MNR at the transcript but not protein level. This may be due to the short time period of treatment or the use of whole sub-placenta/decidua samples, although single cell analysis is beyond the scope of this manuscript. These changes in Ocln, Zo1, and Abcb1 demonstrate that our IGF-1 treatment is contributing to the proper ECM changes needed to create an environment suitable for proper trophoblast invasion. Currently, more than 50% of FGR cases remain undiagnosed prior to birth due, in part, to a lack of definitive ways to predict and detect FGR (Sun, Groom et al., 2020). The placenta is an ideal target for treatment of FGR as it contributes significantly to the etiology of FGR, and is discarded after birth, thus mitigating any potential long-term consequences for maternal health. We have previously shown that treatment of the placenta with hIGF-1 nanoparticle does not result in maternal or fetal toxicity (Wilson, Lampe et al., 2022). Furthermore, in the present study we show that treatment of control placentas with the hIGF-1 nanoparticle results in reduced expression of pERK, VegfA and Pgf, and increased expression of the mTOR inhibitor Deptor indicating the ability of the sub-placenta/decidua under control conditions to maintain homeostasis when excess IGF-1 is present.
We have previously shown our hIGF-1 nanoparticle treatment has the ability to increase glucose and amino acid transporters within the placenta (Wilson, Lampe et al., 2022). In addition to this, we have now shown that under MNR, hIGF-1 nanoparticle treatment is able to increase expression of growth factors and normalize EMT factors through ERK and AKT activation in the sub-placenta for improved trophoblast function. In conclusion, this further indicates potential for improving growth and cell function in pregnancies complicated by FGR and indicates further investigation into long-term placental changes are warranted.
The original contributions presented in the study are included in the article/Supplementary Materials, further inquiries can be directed to the corresponding author.
The animal study was reviewed and approved by Animal care and usage was approved by the Institutional Animal Care and Use Committees at Cincinnati Children’s Hospital and Medical Center (Protocol number 2017-0065).
BD performed experiments, analyzed data and wrote manuscript. HJ obtained funding, conceived the study and edited manuscript. RW conceived the study, performed experiments, analyzed data and wrote manuscript. All authors approve final version of manuscript.
This study was funded by Eunice Kennedy Shriver National Institute of Child Health and Human Development (NICHD) award R01HD090657 (HJ).
We would like to thank Drs Craig Duvall and Mukesh Gupta for providing the co-polymer, and Kristin Lampe for her assistance with the animal experiments.
The authors declare that the research was conducted in the absence of any commercial or financial relationships that could be construed as a potential conflict of interest.
All claims expressed in this article are solely those of the authors and do not necessarily represent those of their affiliated organizations, or those of the publisher, the editors and the reviewers. Any product that may be evaluated in this article, or claim that may be made by its manufacturer, is not guaranteed or endorsed by the publisher.
The Supplementary Material for this article can be found online at: https://www.frontiersin.org/articles/10.3389/fphys.2022.1055234/full#supplementary-material
Abbas Y., Turco M. Y., Burton G. J., Moffett A. (2020). Investigation of human trophoblast invasion in vitro. Hum. Reprod. Update 26 (4), 501–513. doi:10.1093/humupd/dmaa017
Abd Ellah N., Taylor L., Troja W., Owens K., Ayres N., Pauletti G. (2015). Development of non-viral, trophoblast-specific gene delivery for placental therapy. PLoS One 10 (10), e0140879. doi:10.1371/journal.pone.0140879
Adu-Gyamfi E. A., Czika A., Gorleku P. N., Ullah A., Panhwar Z., Ruan L. L. (2021). The involvement of cell adhesion molecules, tight junctions, and gap junctions in human placentation. Reprod. Sci. 28 (2), 305–320. doi:10.1007/s43032-020-00364-7
Ahokas R. A., Anderson G. D., Lipshitz J. (1983). Cardiac output and uteroplacental blood flow in diet-restricted and diet-repleted pregnant rats. Am. J. Obstet. Gynecol. 146 (1), 6–13. doi:10.1016/0002-9378(83)90918-3
Bernstein I. M., Horbar J. D., Badger G. J., Ohlsson A., Golan A. (2000). Morbidity and mortality among very-low-birth-weight neonates with intrauterine growth restriction. The Vermont Oxford Network. Am. J. Obstet. Gynecol. 182 (1 Pt 1), 198–206. doi:10.1016/s0002-9378(00)70513-8
Bhaskar P. T., Hay N. (2007). The two TORCs and Akt. Dev. Cell. 12 (4), 487–502. doi:10.1016/j.devcel.2007.03.020
Brosens I., Dixon H. G., Robertson W. B. (1977). Fetal growth retardation and the arteries of the placental bed. Br. J. Obstet. Gynaecol. 84 (9), 656–663. doi:10.1111/j.1471-0528.1977.tb12676.x
Bulmer J. N., Burton G. J., Collins S., Cotechini T., Crocker I. P., Croy B. A. (2012). IFPA Meeting 2011 workshop report II: Angiogenic signaling and regulation of fetal endothelial function; placental and fetal circulation and growth; spiral artery remodeling. Placenta 33 (Suppl. l), S9–S14. doi:10.1016/j.placenta.2011.11.014
Burton G. J., Fowden A. L. (2015). The placenta: A multifaceted, transient organ. Philos. Trans. R. Soc. Lond B Biol. Sci. 370 (1663), 20140066. doi:10.1098/rstb.2014.0066
Burton G. J., Woods A. W., Jauniaux E., Kingdom J. C. (2009). Rheological and physiological consequences of conversion of the maternal spiral arteries for uteroplacental blood flow during human pregnancy. Placenta 30 (6), 473–482. doi:10.1016/j.placenta.2009.02.009
Carter A. M., Enders A. C., Jones C. J., Mess A., Pfarrer C., Pijnenborg R. (2006). Comparative placentation and animal models: Patterns of trophoblast invasion - a workshop report. Placenta 27 (Suppl. l), S30–S33. doi:10.1016/j.placenta.2006.01.008
Ceckova-Novotna M., Pavek P., Staud F. (2006). P-Glycoprotein in the placenta: Expression, localization, regulation and function. Reprod. Toxicol. 22 (3), 400–410. doi:10.1016/j.reprotox.2006.01.007
Chakraborty C., Gleeson L. M., McKinnon T., Lala P. K. (2002). Regulation of human trophoblast migration and invasiveness. Can. J. Physiol. Pharmacol. 80 (2), 116–124. doi:10.1139/y02-016
Chellakooty M., Vangsgaard K., Larsen T., Scheike T., Falck-Larsen J., Legarth J. (2004). A longitudinal study of intrauterine growth and the placental growth hormone (GH)-insulin-like growth factor I axis in maternal circulation: Association between placental GH and fetal growth. J. Clin. Endocrinol. Metab. 89 (1), 384–391. doi:10.1210/jc.2003-030282
Chen C. Y., Liu S. H., Chen C. Y., Chen P. C., Chen C. P. (2015). Human placenta-derived multipotent mesenchymal stromal cells involved in placental angiogenesis via the PDGF-BB and STAT3 pathways. Biol. Reprod. 93 (4), 103. doi:10.1095/biolreprod.115.131250
Crespo S., Kind* M., Arcaro A. (2016). The role of the PI3K/AKT/mTOR pathway in brain tumor metastasis. J. Cancer Metastasis Treat. 2, 80–89. doi:10.20517/2394-4722.2015.72
Cuffe J. S. M., Holland O., Salomon C., Rice G. E., Perkins A. V. (2017). Review: Placental derived biomarkers of pregnancy disorders. Placenta 54, 104–110. doi:10.1016/j.placenta.2017.01.119
Depreux F. F., Czech L., Whitlon D. S. (2018). Sex genotyping of archival fixed and immunolabeled Guinea pig cochleas. Sci. Rep. 8 (1), 5156. doi:10.1038/s41598-018-23491-3
Dumolt J. H., Powell T. L., Jansson T. (2021). Placental function and the development of fetal overgrowth and fetal growth restriction. Obstetrics Gynecol. Clin. 48 (2), 247–266. doi:10.1016/j.ogc.2021.02.001
Dunk C. E., Pappas J. J., Lye P., Kibschull M., Javam M., Bloise E. (2018). P-Glycoprotein (P-gp)/ABCB1 plays a functional role in extravillous trophoblast (EVT) invasion and is decreased in the pre-eclamptic placenta. J. Cell. Mol. Med. 22 (11), 5378–5393. doi:10.1111/jcmm.13810
Elias A. A., Ghaly A., Matushewski B., Regnault T. R., Richardson B. S. (2016). Maternal nutrient restriction in Guinea pigs as an animal model for inducing fetal growth restriction. Reprod. Sci. 23 (2), 219–227. doi:10.1177/1933719115602773
Elias A. A., Maki Y., Matushewski B., Nygard K., Regnault T. R. H., Richardson B. S. (2017). Maternal nutrient restriction in Guinea pigs leads to fetal growth restriction with evidence for chronic hypoxia. Pediatr. Res. 82 (1), 141–147. doi:10.1038/pr.2017.92
Fowden A. L. (2003). The insulin-like growth factors and feto-placental growth. Placenta 24 (8-9), 803–812. doi:10.1016/s0143-4004(03)00080-8
Fremin C., Saba-El-Leil M. K., Levesque K., Ang S. L., Meloche S. (2015). Functional redundancy of ERK1 and ERK2 MAP kinases during development. Cell. Rep. 12 (6), 913–921. doi:10.1016/j.celrep.2015.07.011
Hatano N., Mori Y., Oh-hora M., Kosugi A., Fujikawa T., Nakai N. (2003). Essential role for ERK2 mitogen-activated protein kinase in placental development. Genes cells. 8 (11), 847–856. doi:10.1046/j.1365-2443.2003.00680.x
Holmes R., Montemagno R., Jones J., Preece M., Rodeck C., Soothill P. (1997). Fetal and maternal plasma insulin-like growth factors and binding proteins in pregnancies with appropriate or retarded fetal growth. Early Hum. Dev. 49 (1), 7–17. doi:10.1016/s0378-3782(97)01867-7
Ikenouchi J., Matsuda M., Furuse M., Tsukita S. (2003). Regulation of tight junctions during the epithelium-mesenchyme transition: Direct repression of the gene expression of claudins/occludin by snail. J. Cell. Sci. 116 (Pt10), 1959–1967. doi:10.1242/jcs.00389
Kalkunte S. S., Mselle T. F., Norris W. E., Wira C. R., Sentman C. L., Sharma S. (2009). Vascular endothelial growth factor C facilitates immune tolerance and endovascular activity of human uterine NK cells at the maternal-fetal interface. J. Immunol. 182 (7), 4085–4092. doi:10.4049/jimmunol.0803769
Kaufmann P., Black S., Huppertz B. (2003). Endovascular trophoblast invasion: Implications for the pathogenesis of intrauterine growth retardation and preeclampsia. Biol. Reprod. 69 (1), 1–7. doi:10.1095/biolreprod.102.014977
Knöfler M., Pollheimer J. (2012). IFPA award in placentology lecture: Molecular regulation of human trophoblast invasion. Placenta 33, S55–S62. doi:10.1016/j.placenta.2011.09.019
Kunkele J., Trillmich F. (1997). Are precocial young cheaper? Lactation energetics in the Guinea pig. Physiol. Zool. 70 (5), 589–596. doi:10.1086/515863
Laviola L., Perrini S., Belsanti G., Natalicchio A., Montrone C., Leonardini A. (2005). Intrauterine growth restriction in humans is associated with abnormalities in placental insulin-like growth factor signaling. Endocrinology 146 (3), 1498–1505. doi:10.1210/en.2004-1332
Lunghi L., Ferretti M. E., Medici S., Biondi C., Vesce F. (2007). Control of human trophoblast function. Reprod. Biol. Endocrinol. 5, 6. doi:10.1186/1477-7827-5-6
Marzioni D., Banita M., Felici A., Paradinas F. J., Newlands E., De Nictolis M. (2001). Expression of ZO-1 and occludin in normal human placenta and in hydatidiform moles. Mol. Hum. Reprod. 7 (3), 279–285. doi:10.1093/molehr/7.3.279
Mess A. (2007). The Guinea pig placenta: Model of placental growth dynamics. Placenta 28 (8-9), 812–815. doi:10.1016/j.placenta.2007.02.005
Morrison J. L., Botting K. J., Darby J. R. T., David A. L., Dyson R. M., Gatford K. L. (2018). Guinea pig models for translation of the developmental origins of health and disease hypothesis into the clinic. J. Physiol. 596 (23), 5535–5569. doi:10.1113/JP274948
Nadar S. K., Karalis I., Al Yemeni E., Blann A. D., Lip G. Y. (2005). Plasma markers of angiogenesis in pregnancy induced hypertension. Thromb. Haemost. 94 (5), 1071–1076. doi:10.1160/TH05-03-0167
Pages G., Guerin S., Grall D., Bonino F., Smith A., Anjuere F. (1999). Defective thymocyte maturation in p44 MAP kinase (Erk 1) knockout mice. Science 286 (5443), 1374–1377. doi:10.1126/science.286.5443.1374
Reinmuth N., Fan F., Liu W., Parikh A. A., Stoeltzing O., Jung Y. D. (2002). Impact of insulin-like growth factor receptor-I function on angiogenesis, growth, and metastasis of colon cancer. Lab. Invest. 82 (10), 1377–1389. doi:10.1097/01.lab.0000032411.41603.c2
Reynolds L. P., Redmer D. A. (1995). Utero-placental vascular development and placental function. J. Anim. Sci. 73 (6), 1839–1851. doi:10.2527/1995.7361839x
Rieger L., O’Connor R. (2021). Controlled signaling—insulin-like growth factor receptor endocytosis and presence at intracellular compartments. Front. Endocrinol. 11, 620013. doi:10.3389/fendo.2020.620013
Shore V. H., Wang T. H., Wang C. L., Torry R. J., Caudle M. R., Torry D. S. (1997). Vascular endothelial growth factor, placenta growth factor and their receptors in isolated human trophoblast. Placenta 18 (8), 657–665. doi:10.1016/s0143-4004(97)90007-2
Slomiany M. G., Rosenzweig S. A. (2004). IGF-1-induced VEGF and IGFBP-3 secretion correlates with increased HIF-1 alpha expression and activity in retinal pigment epithelial cell line D407. Invest. Ophthalmol. Vis. Sci. 45 (8), 2838–2847. doi:10.1167/iovs.03-0565
Sohlstrom A., Katsman A., Kind K. L., Roberts C. T., Owens P. C., Robinson J. S. (1998). Food restriction alters pregnancy-associated changes in IGF and IGFBP in the Guinea pig. Am. J. Physiol. 274 (3), E410–E416. doi:10.1152/ajpendo.1998.274.3.E410
Sun C., Groom K. M., Oyston C., Chamley L. W., Clark A. R., James J. L. (2020). The placenta in fetal growth restriction: What is going wrong? Placenta 96, 10–18. doi:10.1016/j.placenta.2020.05.003
Szukiewicz D., Szewczyk G., Watroba M., Kurowska E., Maslinski S. (2005). Isolated placental vessel response to vascular endothelial growth factor and placenta growth factor in normal and growth-restricted pregnancy. Gynecol. Obstet. Invest. 59 (2), 102–107. doi:10.1159/000082622
Verkeste C. M., Slangen B. F., Daemen M., van Straaten H., Kohnen G., Kaufmann P. (1998). The extent of trophoblast invasion in the preplacental vasculature of the Guinea-pig. Placenta 19 (1), 49–54. doi:10.1016/s0143-4004(98)90098-4
Wang H., Nian L., Li Z., Lu C. (2021). Inhibiting PDGF-D alleviates the symptoms of HELLP by suppressing NF-κB activation. J. Mol. Endocrinol. 66 (3), 233–243. doi:10.1530/JME-20-0308
Wilson R. L., Lampe K., Gupta M. K., Duvall C. L., Jones H. N. (2022). Nanoparticle-mediated transgene expression of insulin-like growth factor 1 in the growth restricted Guinea pig placenta increases placenta nutrient transporter expression and fetal glucose concentrations. Mol. Reprod. Dev. 89, 540–553. doi:10.1002/mrd.23644
Wilson R. L., Owens K., Sumser E. K., Fry M. V., Stephens K. K., Chuecos M. (2020). Nanoparticle mediated increased insulin-like growth factor 1 expression enhances human placenta syncytium function. Placenta 93, 1–7. doi:10.1016/j.placenta.2020.02.006
Wilson R. L., Stephens K. K., Lampe K., Jones H. N. (2021). Sexual dimorphisms in brain gene expression in the growth-restricted Guinea pig can be modulated with intra-placental therapy. Pediatr. Res. 89 (7), 1673–1680. doi:10.1038/s41390-021-01362-4
Xu W., Yang Z., Lu N. (2015). A new role for the PI3K/Akt signaling pathway in the epithelial-mesenchymal transition. Cell. Adh Migr. 9 (4), 317–324. doi:10.1080/19336918.2015.1016686
Keywords: placenta, pregnancy, therapeutic, fetal growth restriction, insulin-like growth factor 1
Citation: Davenport BN, Jones HN and Wilson RL (2023) Placental treatment with insulin-like growth factor 1 via nanoparticle differentially impacts vascular remodeling factors in guinea pig sub-placenta/decidua. Front. Physiol. 13:1055234. doi: 10.3389/fphys.2022.1055234
Received: 27 September 2022; Accepted: 13 December 2022;
Published: 04 January 2023.
Edited by:
Amanda Sferruzzi-Perri, University of Cambridge, United KingdomReviewed by:
Claudio Gustavo Barbeito, National University of La Plata, ArgentinaCopyright © 2023 Davenport, Jones and Wilson. This is an open-access article distributed under the terms of the Creative Commons Attribution License (CC BY). The use, distribution or reproduction in other forums is permitted, provided the original author(s) and the copyright owner(s) are credited and that the original publication in this journal is cited, in accordance with accepted academic practice. No use, distribution or reproduction is permitted which does not comply with these terms.
*Correspondence: Rebecca L. Wilson, cmViZWNjYS53aWxzb25AdWZsLmVkdQ==
Disclaimer: All claims expressed in this article are solely those of the authors and do not necessarily represent those of their affiliated organizations, or those of the publisher, the editors and the reviewers. Any product that may be evaluated in this article or claim that may be made by its manufacturer is not guaranteed or endorsed by the publisher.
Research integrity at Frontiers
Learn more about the work of our research integrity team to safeguard the quality of each article we publish.