- 1Faculty of Kinesiology and Physical Education, University of Toronto, Toronto, ON, Canada
- 2The Tannenbaum Institute for Science in Sport, University of Toronto, Toronto, ON, Canada
Ischemic preconditioning (IPC) has been reported to augment exercise performance, but there is considerable heterogeneity in the magnitude and frequency of performance improvements. Despite a burgeoning interest in IPC as an ergogenic aid, much is still unknown about the physiological mechanisms that mediate the observed performance enhancing effects. This narrative review collates those physiological responses to IPC reported in the IPC literature and discusses how these responses may contribute to the ergogenic effects of IPC. Specifically, this review discusses documented central and peripheral cardiovascular responses, as well as selected metabolic, neurological, and perceptual effects of IPC that have been reported in the literature.
Introduction
Ischemic preconditioning (IPC) is a potential ergogenic technique that involves administering brief repeated episodes of non-lethal ischemia and reperfusion to the limb(s) prior to engaging in exercise. IPC is performed by temporarily occluding the flow of blood through the limb(s) via pressurized tourniquets for a specific duration (most commonly 5 min) followed by a period of reperfusion. This tourniquet-induced ischemia and reperfusion is then repeated for a prespecified number of intervals (most commonly 3–4 times) before commencing exercise (O’Brien and Jacobs, 2021). Although IPC is similar to the increasingly popular modality of blood flow restricted (BFR) exercise (Patterson et al., 2019)—which typically refers to low intensity aerobic or resistance exercise while inflated cuffs are applied to the exercising limb(s) to restrict blood flow during exercise–IPC differs in its application as it is employed passively prior to exercise and is assumed to require complete occlusion of the arteries supplying blood to the limb(s) for prespecified durations and intervals (O’Brien and Jacobs, 2021).
Previously, IPC has been demonstrated to have protective properties against the injurious effects of ischemia-reperfusion injury in mammalian animal models (Pérez-Pinzón, 2004; Konstantinov et al., 2005; Kristiansen et al., 2005; Wang et al., 2016) and has been shown in humans to improve various clinical outcomes such as protection against post-ischemia-reperfusion endothelial dysfunction, and post-surgical acute kidney injury, among others (Luca et al., 2013; Khaliulin et al., 2019; Gu et al., 2021; Liu et al., 2021). In sport and exercise research literature, IPC has been repeatedly reported to improve athletic performance using a variety of maximal exercise intensities and modalities such as running, swimming, or cycling lasting from about 30 s to 20 min (Jean-St-Michel et al., 2011; Bailey et al., 2012b; Cruz et al., 2015, 2016; Kraus et al., 2015; Cocking et al., 2018b; Paradis-Deschênes et al., 2018). However, the consistency and magnitude of such ergogenic responses is highly variable throughout the literature and merits further attention (For review of the ergogenic responses to IPC see: Marocolo et al., 2015a; Marocolo et al., 2019; Incognito et al., 2016; Salvador et al., 2016; Caru et al., 2019).
It has previously been suggested that the training status or sex of the participant may influence the probability of IPC ergogenicity (Gibson et al., 2013; Tocco et al., 2015; Paradis-Deschênes et al., 2017), although there is conflicting evidence (Jean-St-Michel et al., 2011). The possibility has also been raised that there are IPC non-responders (Slysz and Burr, 2018; Slysz et al., 2019). Others have suggested that, due to a paucity of well-blinded investigations, IPC-mediated performance enhancement may be a placebo effect (Marocolo et al., 2015b; Marocolo et al., 2016; Sabino-Carvalho et al., 2017; de Souza et al., 2021). However, both placebo- and nocebo-controlled studies have still found ergogenic benefits of IPC which suggests that a placebo effect alone is unlikely to explain the entirety of observed ergogenic responses (Ferreira et al., 2016; Cheung et al., 2020). We recently reviewed the burgeoning literature on IPC and exercise performance and identified various methodological considerations that may further contribute to heterogenous IPC response rates including differences between or lack of controls of the specific IPC protocol, the tourniquet pressures used, the location of the IPC stimulus, and the time between IPC administration and exercise (O’Brien and Jacobs, 2021).
There is no doubt that there has been growing interest from sport and exercise researchers and practitioners in IPC as a promising ergogenic aid, and there is also no doubt that the mechanistic explanations for the observed performance enhancing effects of the treatment are incompletely understood. Thus, a comprehensive understanding of the physiology of IPC and exercise is imperative to maximizing the likelihood of a positive ergogenic response occurring. While several published reviews have summarized the observed ergogenic effects or methodological variations in IPC exercise studies, at the time of preparing this paper we could find no review that has collated the myriad physiological mediators purported to be the mechanisms that explain the observed ergogenic effects attributed to IPC. Therefore, this narrative review aims to summarize the current evidence of the effects of an acute bout of IPC on the physiological responses to exercise, regardless of whether an ergogenic effect was elicited. Specifically, this report discusses the documented cardiovascular, metabolic, neural, and perceptual responses that occur via IPC and how they may contribute to enhancing exercise performance outside of the laboratory. The primary objective of this review is to provide the reader with an up-to-date report on the physiology of IPC-exercise that will help to inform future IPC practitioners when using IPC for the purposes of performance enhancement during exercise.
Potential mechanisms for IPC-induced ergogenicity
Figure 1 has been constructed to provide a schematic depiction of many of the triggers and subsequent physiological responses raised in the literature that have been purported as contributing to IPC-induced ergogenicity. The reader should be aware that each of the relationships shown in Figure 1 are accompanied by varying degrees of evidence, which is a main focus of this manuscript.
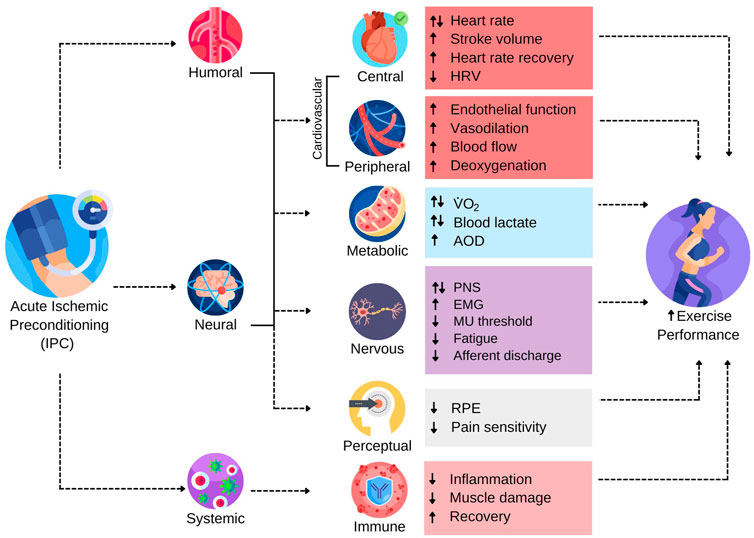
FIGURE 1. The Range of Reported Potential Triggers and Subsequent Responses Contributing to Ergogenic Effects of IPC. AOD, accumulated oxygen deficit; EMG, electromyography; HRV, heart rate variability; MU, motor unit; PNS, peripheral nervous system; RPE, rating of perceived exertion;
Much of the foundational work on underlying IPC mechanisms arose from clinical and animal models that investigated the role of remote IPC (RIPC) in preventing or remediating ischemia-reperfusion injury in various tissue types (Murry et al., 1986; Dickson et al., 1999, 2000; Kristiansen et al., 2005). The protective effects of IPC are reputed to involve humoral, neural, and systemic triggers that initiate a cascade of physiological responses that render tissues more tolerant to subsequent ischemia (Hausenloy and Yellon, 2008). The downstream biochemical pathways purportedly responsible for the protective effects of IPC are complex and have been reviewed previously (Heusch et al., 2015; Kleinbongard et al., 2017; Lang et al., 2022). Presently, it is not known how or if these protective biochemical pathways interact with exercise to promote performance enhancement, or whether the ergogenic effects involve distinctly different physiological responses. Nevertheless, humoral, neural, and systemic responses are putative triggers to the ergogenic effects of IPC (Figure 1). Autacoids released from the ischemic tissue appear to stimulate cardiovascular and neural responses that may affect the physiological response to exercise (Amann et al., 2011; Bouffard et al., 2021). Moreover, IPC has been shown to have systemic effects that modulate the inflammatory response to exercise and attenuate muscle damage, which may indicate the utility of IPC for facilitating training adaptations by reducing cellular damage and augmenting recovery potential from an exercise bout (Arriel et al., 2018; Mieszkowski et al., 2020; Patterson et al., 2021).
Effects of IPC on central and peripheral cardiovascular responses
Central cardiovascular responses
It is important to consider if the well-documented cardioprotective effects of RIPC (Serejo et al., 2007; Rassaf et al., 2014; Singh et al., 2017) mean that RIPC also has beneficial cardiovascular effects on exercise performance. Supplementary Table S1 summarizes the central and peripheral cardiovascular and hemodynamic responses documented following an acute bout of IPC. Changes in central cardiovascular responses following IPC have been observed previously but are not a typical response. For example, in two studies, resting heart rate (HR) was shown to decrease following IPC (Enko et al., 2011; Morley et al., 2021). At the same submaximal exercise intensity, IPC was reported in one study to be associated with a decreased HR (Arriel et al., 2020), whereas another study found an increase in exercising HR at 30% of maximal oxygen consumption (
Similarly, when examining the effects on blood pressure responses, a minority of studies listed in Supplementary Table S1 reported an effect of IPC. Foster et al. (2011) reported that lower limb IPC attenuated the typical increase in systolic blood pressure during hypoxic exercise. Using a protocol where single arm IPC occurred before metaboreflex activation elicited via 3 min of post exercise muscle ischemia, Mulliri et al. (2016) found that the mean arterial pressure response was reduced compared to post-exercise muscle ischemia without preceding IPC. During isometric plantar flexion exercise to exhaustion, Pereira et al. (2020) reported a sex-specific response of IPC, with increased mean arterial pressure responses occurring only in male participants. One investigation reported reduced post-exercise blood pressure following resistance exercise preceded by IPC compared to a control condition (Panza et al., 2020), which may constitute safety concerns for using IPC in populations prone to post-exercise syncope. Furthermore, Barbosa et al. (2015) found increased mean blood pressure at peak exercise intensity during handgrip exercise preceded by IPC, which contrasts with reports finding no changes in blood pressure during static or dynamic handgrip exercise (Horiuchi et al., 2015; Incognito et al., 2017).
Collectively, even with the sound theoretical basis for speculating that IPC before exercise should influence central cardiovascular responses during exercise, there is very limited supporting empirical evidence that such is the case. Thus, it is likely that physiological mechanisms other than central cardiovascular effects of IPC are primarily responsible for the ergogenic effects of IPC, while central cardiovascular responses may contribute modestly in some cases.
Peripheral cardiovascular responses
Compared to central cardiovascular responses more robust evidence exists to postulate that peripheral cardiovascular responses contribute to IPC-induced performance enhancement. IPC has been shown to acutely affect peripheral vascular responses such as enhanced conduit artery vasodilation, endothelial function, convective O2 delivery, and deoxygenation kinetics at the muscle capillary interface (Loukogeorgakis et al., 2005; Enko et al., 2011; Paradis-Deschênes et al., 2016; Cocking et al., 2018a). The reader is directed to the following references for associated details of each of these phenomena (Pagliaro et al., 2003; Luca et al., 2013; Paradis-Deschênes et al., 2016, 2017; Cocking et al., 2018a; Gu et al., 2021).
Changes in blood vessel function appear to involve both local and neural mechanisms depending on the location of the IPC stimulus relative to the tissue of interest (i.e., RIPC versus local IPC). External compression of the peripheral blood vessels in the limb via IPC may augment local feedforward and feedback responses at the site of ischemia that may persist for some time following the cessation of the treatment (Tschakovsky and Pyke, 2010; Joyner and Casey, 2015; Roseguini and Laughlin, 2018). During ischemia, local vasodilators such as adenosine, bradykinin, or nitric oxide (NO) are phosphorylated in the ischemic tissue and released into systemic circulation where they promote vasodilatory responses and upregulate parasympathetic activity (Gho et al., 1996; Liem et al., 2002; Kimura et al., 2007; Enko et al., 2011). During reperfusion, the sudden and rapid restoration of blood flow to the limb may be expected to increase shear stress in the conduit arteries, promoting flow mediated dilation (FMD) in the target limb(s) (Bailey et al., 2012a). This may further stimulate NO synthase or adenosine mediated actions at the ischemic site that further contribute to changes in local vessel diameter (Rosenberry and Nelson, 2020). Together, when IPC is applied to the exercising limb(s), upstream vasoactive responses such as FMD may theoretically contribute to enhancing vascular conductance and promote greater downstream convective O2 delivery to the site of the active skeletal muscle, thereby enriching the pool of available O2 for extraction and utilization during exercise. This assumes that there is a proportional metabolic demand for O2 during exercise preceded by IPC, which may not be the case if ischemic protection is involved in altering metabolic efficiency (Pang et al., 1995; Addison et al., 2003; Andreas et al., 2011).
Indeed, evidence supports the above theory of local vasodilatory responses to IPC. A recent systematic review and meta-analysis by Gu et al. (2021) investigated the role of arm IPC on resting vascular endothelial function and the relationship between IPC, FMD, and brachial artery diameter. That review concluded that there are beneficial effects of IPC on endothelial function of the blood vessels by enhancing FMD. However, despite improved endothelial function and FMD, there was no effect found of IPC on brachial artery diameter. Although conducted in non-exercise studies, these findings lend support to the hypothesis of acute improvements in vascular endothelial function and our speculation that such improvements have the potential to contribute to enhanced exercise capacity. Further, one exercise study did find enlarged brachial artery diameter during rhythmic handgrip exercise preceded by IPC to the arms, which may suggest an effect of IPC on conduit artery diameter when IPC is combined with exercise (Cocking et al., 2018a). Several other investigations have demonstrated positive effects of IPC techniques on endothelial function but more research is necessary to elucidate the role of IPC in modulating endothelial responses during exercise (Loukogeorgakis et al., 2005; Enko et al., 2011; Manchurov et al., 2014; el Dabagh et al., 2017).
Vasoactive responses have also been observed in blood vessels surrounding tissues located remotely to the IPC stimulus; such observations cannot be plausibly be explained by local feedforward or feedback responses (Tschakovsky and Pyke, 2010; Crecelius et al., 2012). However, these observations may be indirect evidence suggestive of the existence of a neural component to altered peripheral vascular responses following RIPC, although the precise mechanisms for this response are equivocal. Demonstrating this phenomenon, Manchurov et al. (2014) reported augmented brachial artery FMD after acute RIPC to the contralateral limb with the effects persisting up to 7 d after exposure. Another study reported a larger brachial artery diameter after RIPC was applied to the contralateral limb and this response was associated with increased parasympathetic activity (Enko et al., 2011). This finding is consistent with other reports highlighting the ability of IPC techniques to blunt sympathetic nervous system activity which will be discussed later in this review (Horiuchi et al., 2015). RIPC has also been reported to sustain FMD in the contralateral limb for at least 48 h after ischemia-reperfusion injury, and these effects are purportedly neuronally mediated (Loukogeorgakis et al., 2005). Similar remote vasoactive effects have been observed in tissues more distal to the RIPC stimulus as well. For example, Bailey et al. (2012a) found that IPC applied to the lower extremities prior to 5 km running time-trials prevented the typically observed post-exercise reductions in brachial artery endothelial function during FMD tests. Another report demonstrated increased FMD in young people as well as healthy or hypertensive elderly patients following both local and remote IPC, which lends further support to local and neuronally mediated vasoactive response to IPC and RIPC (Moro et al., 2011).
The above responses provide a theoretical basis for speculating that local and neurally mediated vasoactive responses to IPC could lead to improved exercise performance. Moreover, studies assessing blood volume and deoxygenation kinetics during exercise provide additional support for this theory by demonstrating the expected downstream effects of these responses at the exercising muscle. For example, acute local IPC is often accompanied by near infrared spectroscopy-measured (NIRS) marked increases in total haemoglobin [tHb] which is an indicator of total blood volume (Paradis-Deschênes et al., 2016, 2017), and deoxygenated haemoglobin [HHb] which is an indicator of muscle oxygen extraction (Paradis-Deschênes et al., 2016, 2017, 2020; Halley et al., 2018) in active muscle during intense exercise, although this is not always the case (Supplementary Table S1). The tissue saturation index (TSI)—which represents the equilibrium between O2 supply and consumption during exercise–has also been shown to be reduced along with concomitant reductions in oxygenated haemoglobin [O2Hb], further highlighting this deoxygenation response (Turnes et al., 2018). It is not clear for how long after the treatment protocol that enhanced muscle perfusion and deoxygenation kinetics persist, but Cunniffe et al. (2017) reported increased change in oxygenated haemoglobin [ΔO2Hb] in the vastus lateralis for at least 15 min after and da Mota et al. (2019) found augmented deoxygenation responses when performing exercise 2 h after IPC, which suggests some latency in the effect. Despite these examples demonstrating evidence of enhanced blood volume and deoxygenation during exercise following IPC, other studies have reported contrasting results (Seeger et al., 2017; Griffin et al., 2018; Beek et al., 2020; Cocking et al., 2020; Mota et al., 2020; Tanaka et al., 2020). One study reported greater TSI maintenance during repeated sprint running bouts, suggesting attenuated muscle deoxygenation kinetics (Patterson et al., 2015). However, these authors did not report values for [tHb], [HHb], or [O2Hb], which presents challenges with determining the mediator of enhanced TSI maintenance.
The peripheral hemodynamic response may be less robust during RIPC compared to local IPC, although the limited available evidence should be acknowledged here. One study found modest changes to hemodynamic variables following RIPC (Richard and Billaut, 2018), whereas another found no effect of RIPC on hemodynamic responses during rhythmic handgrip exercise (Barbosa et al., 2015). With such limited evidence on the hemodynamic responses of RIPC in exercising muscle, it would not be appropriate to propose that an effect exists. Similarly, more research is necessary to improve the understanding of the potential neural contribution to RIPC vasodilation and whether this leads to downstream enhancements in muscle perfusion and deoxygenation responses.
Taken together, a growing body of evidence summarized in Supplementary Table S1 suggests that peripheral vascular and hemodynamic responses may contribute to the ergogenic responses observed following local and remote IPC. These responses are likely mediated by several known vasoactive mechanisms such as shear mediated dilation (Gu et al., 2021), neuronally mediated vasodilation (Manchurov et al., 2014), reactive hyperemia (Rosenberry and Nelson, 2020), and increased extraction of O2 at the muscle-capillary interface (Paradis-Deschênes et al., 2016). Curiously, these effects are not always observed, which may be partly attributable to methodological variations between study protocols or may infer the existence of IPC non-responders (O’Brien and Jacobs, 2021).
Effect of IPC on metabolic responses to exercise
One of the central motivations for the initial study of IPC as an ergogenic aid was its promising potential to promote enhanced metabolic efficiency in active tissues. In theory, this could be useful during exercise at terminal intensities where the magnitude of the mismatch between O2 supply and demand can be reduced with improved metabolic efficiency resulting in a greater endurance capacity (Joyner and Casey, 2015). Early IPC studies provided evidence that IPC modulates metabolic activity in tissues as reflected by attenuated rates of muscle lactate accumulation, as well as adenosine triphosphate and glycogen depletion during prolonged ischemic episodes in resting animal muscle (Pang et al., 1995; Addison et al., 2003). This suggests that IPC could alter oxidative efficiency or substrate utilization in active muscle during exercise. In humans, functional MRI has been used to demonstrate that IPC positively influences resting skeletal muscle metabolism by promoting increased phosphocreatine production and O2 consumption (Andreas et al., 2011). However, more recent evidence suggests that altered muscle energy metabolism during exercise preceded by IPC does not appear to be as promising of a potential mediator of ergogencity as was once hypothesized (Supplementary Table S2).
In exercise interventions, the metabolic responses to IPC maneuvers have been extensively measured with varying results (Supplementary Table S2). Most commonly, exercising metabolic activity has been characterized using indirect calorimetry and blood lactate measurements, although some studies have used other methods to assess additional metabolic variables. For example, blood chemistry profiles have consistently demonstrated no effect of IPC on blood glucose, pH, bicarbonate, carbon dioxide, or O2 saturation values after exercise (Williams et al., 2018; Halley et al., 2020; Paradis-Deschênes et al., 2020; Chen et al., 2022). Sixty-seven percent of the studies listed in Supplementary Table S2 report no effect of IPC techniques on key indices of aerobic or anaerobic metabolism, regardless of outcomes of IPC on athletic/exercise performance. Still, those studies that have reported acute alterations in aerobic and/or anaerobic exercise metabolism indicate the topic merits further interrogation.
Acute modifications to aerobic metabolism can be observed in studies assessing respiratory variables such as oxygen consumption (
The effect of IPC on anaerobic metabolic activity has also been extensively examined indirectly through measuring changes in blood lactate concentrations and respiratory gases. Nine of the studies listed in Supplementary Table S2 reported altered blood lactate responses following IPC whereas 34 studies found no alterations to blood lactate concentrations. Baseline blood lactate concentrations were found to be lowered via IPC in two studies (Paull and van Guilder, 2019; Mota et al., 2020). Bailey et al. (2012b) reported attenuated increases in submaximal blood lactate concentrations after IPC compared to a low-pressure sham which could suggest enhanced submaximal oxidative metabolism. However, no differences in submaximal gas exchange parameters were found in this same report. Other reports found post-exercise blood lactate concentrations to be significantly higher in IPC conditions after intense cycling (Cruz et al., 2015, 2016; Patterson et al., 2015) and swimming exercise (Lisbôa et al., 2017). Conversely, Gibson et al. (2015) found significantly lower blood lactate concentrations in female, but not male, participants after repeated sprint cycling. Finally, Ceylan and Franchini (2022) reported significantly lower post-exercise blood lactate concentrations after judo-specific fitness tests preceded by IPC. The accumulated oxygen deficit (AOD)—which is used as a proxy of anaerobic capacity during exercise (Noordhof et al., 2010)—has also been demonstrated to be influenced by IPC administration (Cruz et al., 2016; Paull and van Guilder, 2019; Chen et al., 2022). Cruz et al. (2016) reported increased AOD following IPC administration during sprint cycling exercise whereas Paull and Van Guilder (2019) observed RIPC to increase AOD during supramaximal running time-to-exhaustion tests. More recently, Chen et al. (2022) found both IPC and RIPC techniques to increase AOD during supramaximal running in trained runners. In contrast, one study did not find alterations to AOD following IPC administration during simulated 1,000 m kayak ergometry races (Halley et al., 2020).
Taken together, the effects of IPC on metabolic responses to exercise are widely ranging and equivocal (see Supplementary Table S2). Although early IPC studies showed promise for IPC to potentially modulate metabolic activity during exercise, most studies do not demonstrate an acute effect of IPC on indices of aerobic or anerobic metabolic activity. However, there are a notable subset of studies that have demonstrated differential metabolic responses to exercise after IPC such as changes in
Effect of IPC on neurological and perceptual responses to exercise
Seeing as there is a known neural trigger from RIPC in mediating ischemic protection (Costa et al., 2001; Weinbrenner et al., 2002; Khaliulin et al., 2019), it is tempting to speculate that the nervous system contributes to the ergogenic benefit of IPC. Yet, comparatively fewer IPC-exercise investigations have directly examined the effects of IPC techniques on modulating neurological responses during exercise (Supplementary Table S3). Growing research efforts have begun to reveal the potential beneficial effects of IPC on modulating cardiac autonomic responses to exercise. These reports have shown IPC to increase heart rate recovery, and reduce cardiac parasympathetic activity and heart rate variability responses (Lopes et al., 2018; Sabino-Carvalho et al., 2019; Morley et al., 2021; da Silvas Telles et al., 2021; Telles et al., 2022). Reduced heart rate variability can be used as an indirect marker of reduced cardiac parasympathetic nervous activity (Morely et al., 2021), which could possibly attribute to increased cardiac output during IPC exercise (Crisafulli et al., 2011). Conversely, other reports have found no effect of IPC maneuvers on heart rate variability responses which indicates that more work is necessary to elucidate the neurocardiovascular response to IPC (Lopes et al., 2018; Sabino-Carvalho et al., 2019). Furthermore, IPC has been shown to augment functional sympatholysis which may contribute to enhanced peripheral vascular function during exercise (Horiuchi et al., 2015).
Based on the available literature, most studies that measure electromyographical (EMG) activity during IPC-exercise have found no effect on neuromuscular function, regardless of whether performance enhancement was found (Supplementary Table S3). Conversely, acute IPC has been shown to enhance EMG activity and delay neuromuscular fatigue in other reports (Cruz et al., 2015, 2016; Hyngstrom et al., 2018; Pethick et al., 2021). In some investigations, increases in maximal strength following IPC have been purported to involve increased neuromuscular activity and reduced motor unit recruitment thresholds (Hyngstrom et al., 2018; Durand et al., 2019). It is not clear why some reports find altered EMG activity during exercise preceded by IPC while others do not, and the interpretation of such conflicting findings is challenging. Nevertheless, the minority of studies suggesting modulated neuromuscular responses should not be overlooked. It is possible that there exists a dose-response relationship between IPC and neuromuscular responses to exercise. Recently, Morley et al. (2021) demonstrated greater perturbations on cardiac autonomic responses when combining RIPC with concurrent low intensity cycling exercise compared to traditional RIPC. Another report found the ergogenic effects of IPC were augmented by enhancing metabolic stress by walking or electrical muscle stimulation in conjunction with IPC (Slysz and Burr, 2018). Further research efforts should aim to elucidate the required metabolic stress threshold to elicit effects of IPC on physiological responses to exercise.
One neural mechanism that has been previously hypothesized to contribute to the ergogenic effect of IPC involves the desensitization of metabolically sensitive metabo-nociceptive neurons known as group III/IV muscle afferents (Crisafulli et al., 2011; Cruz et al., 2015, 2016; Salvador et al., 2016; Incognito et al., 2017). Briefly, group III/IV muscle afferents are free nerve endings that respond to perturbations in the mechanical and metabolic states of muscle, respectively (Amann et al., 2011). Feedback mechanisms via group III/IV muscle afferents have been shown to initiate the exercise pressor reflex, which is an essential response governing the cardiovascular response to exercise (Rowell and O’Leary, 1990; Amann et al., 2011; Nobrega et al., 2014). The exercise pressor reflex may account for some of the central and peripheral cardiovascular responses discussed earlier in this review. Group III/IV muscle afferents contribute to various essential processes of the typical physiological response to exercise, including inducing central fatigue (Sidhu et al., 2013), inhibiting α-motor neuron activation (Gandevia, 2001; Amann et al., 2011), and modulating ascending pain signalling in response to noxious metabolic stimuli produced as biproducts of muscle contraction (O’Connor and Cook, 1999; Crisafulli et al., 2006, 2008; Kaufman, 2012). Theoretically, if IPC were to reduce stimulation of these muscle afferents, exercise performance could be prolonged through attenuating central nervous system fatigue, delaying the inhibition of α-motor neurons, ameliorating the perception of pain, and/or augmenting the neuro-cardiovascular response to intense exercise.
Few studies have sought to directly investigate the relationship between IPC on discharge from group III/IV muscle afferents. One study found an attenuated hemodynamic response to metaboreflex activation during dynamic handgrip exercise preceded by IPC (Mulliri et al., 2016), whereas another report did not show IPC to reduce hemodynamic responses or central muscle sympathetic nervous activity during activation of the metaboreflex (Incognito et al., 2017). More recently, Angius et al. (2022) corroborated the previously observed reduction in hemodynamic responses during metaboreflex activation using dynamic leg extension exercise. However, these authors also reported reduced end diastolic volumes following IPC which seems to indicate that altered hemodynamic responses during metaboreflex activation were due to blunted cardiac preload rather than discharge from group III/IV muscle afferents. To the best of our knowledge, no published studies have investigated the effect of IPC on the mechanoreflex. Thus, the current evidence on the effect of IPC on group III/IV afferent discharge is insufficient to draw a conclusion about the magnitude of the potential contribution to IPC-induced ergogenicity at this time.
Perhaps lending further support to the muscle afferent hypothesis is the evidence of IPC inducing hypoalgesic effects in humans. Indeed, previous investigations have demonstrated IPC techniques to reduce pressure pain ratings, pain under ischemia, perceived pain following eccentric exercise induced muscle damage, and time spent under pain during cold-water immersion tests (Ferreira et al., 2016; Pereira et al., 2020; Slysz and Burr, 2021; Angius et al., 2022). IPC-induced hypoalgesia may be governed by endogenous substances that have antinociceptive effects on pain pathways. Metabolic mediators including adenosine, bradykinin, opioids, and endocannabinoids–all of which are involved in pain signalling during exercise (Sgherza et al., 2002; Murase et al., 2010; Aguiar et al., 2020; Hughes and Patterson, 2020; Hughes et al., 2021)—have been purported to contribute to the protective response to RIPC (Lee et al., 1996; Tomai et al., 1999; Cohen et al., 2000; Schoemaker and van Heijningen, 2000; Dickson et al., 2002; Patel et al., 2002; Hajrasouliha et al., 2008). Furthermore, the endogenous release of some of these autacoids is thought to modulate the stimulation and signalling of group III/IV afferents (Amann et al., 2009, Amann et al., 2011; Bouffard et al., 2021).
Indeed, activation of opioid and endocannabinoid receptors in the central and peripheral sites of the pain pathway such as the spine and peripheral nerves can modulate ascending and descending pain signalling (Sgherza et al., 2002; Hughes and Patterson, 2020; Hughes et al., 2021). Specifically, plasma concentrations of an opioid neuropeptide known as beta-endorphin (BE) and the endocannabinoid endogenous ligand agonist 2-arachidonoylglycerol (2-AG) have recently been shown to be elevated following BFR exercise in humans (Hughes and Patterson, 2020; Hughes et al., 2021). Although there is a distinction between BFR and IPC, the similarities between these techniques involves overlapping mechanisms (Jessee et al., 2018; Patterson et al., 2019), which may implicate the involvement of these substances in the IPC response. Briefly, BE is thought to be released by the pituitary in response to stimulation of group III/IV afferents, thereby activating the opioid system and descending pain inhibitory pathways (Thoren et al., 1990; Hughes and Patterson, 2020). 2-AG appears to involve activation of endocannabinoid receptors on A-delta and C-delta primary afferents (Koltyn et al., 2014), which, to the best of our knowledge have not been explored in IPC research. Future research efforts should aim to elucidate the potential role between the endogenous opioid and endocannabinoid systems in modulating perceived pain or discomfort during exercise, and whether this contributes to the ergogenic response.
Pain perception serves an important function in protecting the body from deleterious stimuli through promoting avoidance behaviours (Philips and Jahanshahi, 1986). Similarly, during intense exercise, perceptual markers such as rating of perceived exertion (RPE) are known to initiate exercise intolerance and similar avoidance behaviours (Noble et al., 1983; Tucker, 2009). Thus, when considering the potential hypoalgesic properties of IPC, it is tempting to speculate that IPC may also attenuate perceptual markers of exertional discomfort during exercise. If IPC indeed attenuates RPE, it may be hypothesized that exercise capacity could be enhanced by this effect. That is, by attenuating RPE at a specific absolute exercise intensity, the associated relative exercise intensity is also reduced which may be significant for prolonging endurance through elevating the threshold at which exercise intolerance occurs (Tucker, 2009). Despite similar avoidance behaviours, pain and RPE are distinct subjective perceptions (Marcora, 2009). Moreover, reduced subjective pain ratings following IPC were not shown to influence RPE during fatiguing plantar flexion exercise and did not correlate with the ergogenic effects during exercise (Pereira et al., 2020; Slysz and Burr, 2021). This suggests that altered pain sensitivity does not govern the ergogenic response which is consistent with previous assertions that afferent feedback is independent from effort perception (Marcora, 2009). Further supporting the divergence between afferent feedback and perceived effort, the available evidence suggests that IPC has menial effects on RPE. Five of the studies found in Supplementary Table S4 showed attenuated RPE during exercise preceded by IPC (Bailey et al., 2012b; Cruz et al., 2015; Paradis-Deschênes et al., 2018; Beek et al., 2020; Behrens et al., 2020), whereas 25 studies found no influence of IPC on RPE during various exercise interventions. Some of this disagreement may be due to variability in exercise protocols across interventions. For example, RPE may be more likely to be attenuated during endurance efforts where an individual is exercising near maximal aerobic capacity versus supramaximal intensities where perceived effort should already be maximized. Nevertheless, most research efforts show no effect of IPC on RPE.
The effects of IPC on neural and perceptual responses to exercise are still being probed. Currently, there is no consistently reported effect of IPC on neural responses, however, a notable body of evidence suggests some relationship. Moreover, the majority of studies that have examined IPC and RPE have found no evidence to postulate that IPC modulates the perception of discomfort during exercise. Future research efforts should aim to generate more data on the neural effects of IPC to better characterize the potential contribution to IPC ergogenicity.
Conclusion
The present review aimed to summarize the myriad physiological responses measured during acute IPC exercise interventions and to contemplate how such responses may contribute to enhancing exercise performance. Acute bouts of IPC have been demonstrated to modulate cardiovascular, hemodynamic, metabolic, neurological, and perceptual responses during subsequent acute exercise. However, the reproducibility and synchroneity among these responses to IPC are equivocal for reasons that remain unclear. O’Brien and Jacobs (2021) previously reviewed the widely varying methodologies extant in the IPC exercise literature, and discussed how that methodological heterogeneity likely explains some the equivocal nature of the related research findings. Thus the present review does not address the methodological differences in IPC among the studies included in the review, but instead focused on reviewing the purported physiological mediators of the effects of IPC on exercise performance.
Based on the widely varying mechanisms reported in this review as primarily responsible for mediating IPC-induced ergogenic effects, it is tempting to speculate that an “entourage” effect, i.e., a combined effect of several mediators is the explanation for observed performance enhancements. Future efforts should aim to assess the relative importance and contributions of the identified physiological and biochemical mediators as well as the necessary methodological strategies for optimizing the conditions for the most important mediators to maximize the efficacy of the treatment on performance outcomes.
Author contributions
Both authors, LO’B and IJ contributed to the writing and reading of this manuscript. The collection and interpretation of the literature review was undertaken by LO’B. Drafting and formatting of the article was undertaken by LO’B and IJ. Both LO’B and IJ contributed to the critical revisions of the manuscript. Both authors have reviewed and approved the final version of this manuscript and agree to be accountable for the accuracy of the information presented.
Funding
LO’B is supported by the University of Toronto, Faculty of Kinesiology and Physical Education.
Conflict of interest
The authors declare that the research was conducted in the absence of any commercial or financial relationships that could be construed as a potential conflict of interest.
Publisher’s note
All claims expressed in this article are solely those of the authors and do not necessarily represent those of their affiliated organizations, or those of the publisher, the editors and the reviewers. Any product that may be evaluated in this article, or claim that may be made by its manufacturer, is not guaranteed or endorsed by the publisher.
Supplementary material
The Supplementary Material for this article can be found online at: https://www.frontiersin.org/articles/10.3389/fphys.2022.1051529/full#supplementary-material
References
Addison P. D., Neligan P. C., Ashrafpour H., Khan A., Zhong A., Moses M., et al. (2003). Noninvasive remote ischemic preconditioning for global protection of skeletal muscle against infarction. Am. J. Physiol. Heart Circ. Physiol. 285, 1435–1443. doi:10.1152/ajpheart.00106.2003
Aguiar A. S., Speck A. E., Canas P. M., Cunha R. A. (2020). Neuronal adenosine A2A receptors signal ergogenic effects of caffeine. Sci. Rep. 10, 13414. doi:10.1038/s41598-020-69660-1
Amann M., Proctor L. T., Sebranek J. J., Pegelow D. F., Dempsey J. A. (2009). Opioid-mediated muscle afferents inhibit central motor drive and limit peripheral muscle fatigue development in humans. J. Physiol. 587, 271–283. doi:10.1113/jphysiol.2008.163303
Amann M., Runnels S., Morgan D. E., Trinity J. D., Fjeldstad A. S., Wray D. W., et al. (2011). On the contribution of group III and IV muscle afferents to the circulatory response to rhythmic exercise in humans. J. Physiol. 589, 3855–3866. doi:10.1113/jphysiol.2011.209353
Andreas M., Schmid A. I., Keilani M., Doberer D., Bartko J., Crevenna R., et al. (2011). Effect of ischemic preconditioning in skeletal muscle measured by functional magnetic resonance imaging and spectroscopy: A randomized crossover trial. J. Cardiovasc. Magn. Reson. 13, 32–10. doi:10.1186/1532-429X-13-32
Angius L., Pageaux B., Crisafulli A., Hopker J., Marcora S. M. (2022). Ischemic preconditioning of the muscle reduces the metaboreflex response of the knee extensors. Eur. J. Appl. Physiol. 122, 141–155. doi:10.1007/s00421-021-04815-0
Arriel R. A., de Souza H. L. R., da Mota G. R., Marocolo M. (2018). Declines in exercise performance are prevented 24 hours after post-exercise ischemic conditioning in amateur cyclists. PLoS One 13, e0207053. doi:10.1371/journal.pone.0207053
Arriel R. A., Meireles A., Hohl R., Marocolo M. (2020). Ischemic preconditioning improves performance and accelerates the heart rate recovery. J. Sports Med. Phys. Fit. 60, 1209–1215. doi:10.23736/S0022-4707.20.10822-3
Bailey T. G., Birk G. K., Timothy Cable N., Atkinson G., Green D. J., Jones H., et al. (2012a). Remote ischemic preconditioning prevents reduction in brachial artery flow-mediated dilation after strenuous exercise. Am. J. Physiol. Heart Circ. Physiol. 303, 533–538. doi:10.1152/ajpheart.00272.2012
Bailey T. G., Jones H., Gregson W., Atkinson G., Cable N. T., Thijssen D. H. J. (2012b). Effect of ischemic preconditioning on lactate accumulation and running performance. Med. Sci. Sports Exerc. 44, 2084–2089. doi:10.1249/MSS.0b013e318262cb17
Barbosa T. C., Machado A. C., Braz I. D., Fernandes I. A., Vianna L. C., Nobrega A. C. L., et al. (2015). Remote ischemic preconditioning delays fatigue development during handgrip exercise. Scand. J. Med. Sci. Sports 25, 356–364. doi:10.1111/sms.12229
Beek T. F., Jokumsen P. S., Sloth B. N., Stevenson A. J. T., Larsen R. G. (2020). Ischemic preconditioning attenuates rating of perceived exertion but does not improve maximal oxygen consumption or maximal power output. J. Strength Cond. Res. 36 (9), 2479–2485. doi:10.1519/JSC.0000000000003625
Behrens M., Zschorlich V., Mittlmeier T., Bruhn S., Husmann F. (2020). Ischemic preconditioning did not affect central and peripheral factors of performance fatigability after submaximal isometric exercise. Front. Physiol. 11, 371–414. doi:10.3389/fphys.2020.00371
Bouffard S., Paradis-Deschênes P., Billaut F. (2021). Neuromuscular adjustments following sprint training with ischemic preconditioning in endurance athletes: Preliminary data. Sports 9, 124. doi:10.3390/sports9090124
Caru M., Levesque A., Lalonde F., Curnier D. (2019). An overview of ischemic preconditioning in exercise performance: A systematic review. J. Sport Health Sci. 8, 355–369. doi:10.1016/j.jshs.2019.01.008
Ceylan B., Franchini E. (2022). Ischemic preconditioning does not improve judo-specific performance but leads to better recovery in elite judo athletes. Sci. Sports 37, 322.e1–322.e7. doi:10.1016/j.scispo.2021.08.004
Chen Y., Yang J., Muradov O., Li X., Lee J. K. W., Qiu J. (2022). Effect of ischemic preconditioning on maximum accumulated oxygen deficit in 400-meter runners. Eur. J. Sport Sci., 1–8. doi:10.1080/17461391.2022.2064769
Cheung C. P., Slysz J. T., Burr J. F. (2020). Ischemic preconditioning: Improved cycling performance despite nocebo expectation. Int. J. Sports Physiol. Perform. 15, 354–360. doi:10.1123/ijspp.2019-0290
Clevidence M. W., Mowery R. E., Kushnick M. R. (2012). The effects of ischemic preconditioning on aerobic and anaerobic variables associated with submaximal cycling performance. Eur. J. Appl. Physiol. 112, 3649–3654. doi:10.1007/s00421-012-2345-5
Cocking S., Cable N. T., Wilson M. G., Green D. J., Thijssen D. H. J., Jones H. (2018a). Conduit artery diameter during exercise is enhanced after local, but not remote, ischemic preconditioning. Front. Physiol. 9, 435. doi:10.3389/fphys.2018.00435
Cocking S., Ihsan M., Jones H., Hansen C., Cable N. T., Thijssen D. H. J., et al. (2020). Repeated sprint cycling performance is not enhanced by ischaemic preconditioning or muscle heating strategies. Eur. J. Sport Sci. 21, 166–175. doi:10.1080/17461391.2020.1749312
Cocking S., Wilson M. G., Nichols D., Cable N. T., Green D. J., Thijssen D. H. J., et al. (2018b). Is there an optimal ischemic-preconditioning dose to improve cycling performance? Int. J. Sports Physiol. Perform. 13, 274–282. doi:10.1123/ijspp.2017-0114
Cohen M. v., Baines C. P., Downey J. M. (2000). Ischemic preconditioning: From adenosine receptor to K ATP channel. Annu. Rev. Physiol. 62, 79–109. doi:10.1146/annurev.physiol.62.1.79
Costa F., Christensen N. J., Farley G., Biaggioni I. (2001). NO modulates norepinephrine release in human skeletal muscle: Implications for neural preconditioning. Am. J. Physiol. Regul. Integr. Comp. Physiol. 280, 1494–1498. doi:10.1152/ajpregu.2001.280.5.r1494
Crecelius A. R., Kirby B. S., Luckasen G. J., Larson D. G., Dinenno F. A. (2012). ATP-mediated vasodilatation occurs via activation of inwardly rectifying potassium channels in humans. J. Physiol. 590, 5349–5359. doi:10.1113/jphysiol.2012.234245
Crisafulli A., Milia R., Lobina A., Caddeo M., Tocco F., Concu A., et al. (2008). Haemodynamic effect of metaboreflex activation in men after running above and below the velocity of the anaerobic threshold. Exp. Physiol. 93, 447–457. doi:10.1113/expphysiol.2007.041863
Crisafulli A., Salis E., Pittau G., Lorrai L., Tocco F., Melis F., et al. (2006). Modulation of cardiac contractility by muscle metaboreflex following efforts of different intensities in humans. Am. J. Physiol. Heart Circ. Physiol. 291, 3035–3042. doi:10.1152/ajpheart.00221.2006
Crisafulli A., Tangianu F., Tocco F., Concu A., Mameli O., Mulliri G., et al. (2011). Ischemic preconditioning of the muscle improves maximal exercise performance but not maximal oxygen uptake in humans. J. Appl. Physiol. 111, 530–536. doi:10.1152/japplphysiol.00266.2011
Cruz R. S., Alves de Aguiar R., Turnes T., Leonardo Pereira K., Caputo F., Rso C., et al. (2015). Effects of ischemic preconditioning on maximal constant-load cycling performance. J. Appl. Physiol. 119, 961–967. doi:10.1152/japplphysiol.00498.2015
Cruz R. S., de Aguiar R. A., Turnes T., Salvador A. F., Caputo F. (2016). Effects of ischemic preconditioning on short-duration cycling performance. Appl. Physiology, Nutr. Metabolism 41, 825–831. doi:10.1139/apnm-2015-0646
Cunniffe B., Sharma V., Cardinale M., Yellon D. (2017). Characterization of muscle oxygenation response to vascular occlusion: Implications for remote ischaemic preconditioning and physical performance. Clin. Physiol. Funct. Imaging 37, 785–793. doi:10.1111/cpf.12353
da Mota G. R., Willis S. J., Sobral N. D. S., Borrani F., Billaut F., Millet G. P. (2019). Ischemic preconditioning maintains performance on two 5-km time trials in hypoxia. Med. Sci. Sports Exerc. 51, 2309–2317. doi:10.1249/MSS.0000000000002049
de Groot P. C. E., Thijssen D. H. J., Sanchez M., Ellenkamp R., Hopman M. T. E. (2010). Ischemic preconditioning improves maximal performance in humans. Eur. J. Appl. Physiol. 108, 141–146. doi:10.1007/s00421-009-1195-2
de Souza H. L. R., Arriel R. A., Mota G. R., Hohl R., Marocolo M. (2021). Does ischemic preconditioning really improve performance or it is just a placebo effect? PLoS One 16, e0250572. doi:10.1371/journal.pone.0250572
Dickson E., Porcaro W., Fenton R., Heard S., Reindhardt C., Renzi F., et al. (2000). Preconditioning at a distance’ in the isolated rabbit heart. Acad. Emerg. Med. 7, 311–317. doi:10.1111/j.1553-2712.2000.tb02228.x
Dickson E. W., Lorbar M., Porcaro W. A., Fenton R. A., Reinhardt C. P., Gysembergh A., et al. (1999). Rabbit heart can be “preconditioned” via transfer of coronary effluent. Am. J. Physiol. 277, 2451–2457. doi:10.1152/ajpheart.1999.277.6.h2451
Dickson E. W., Tubbs R. J., Porcaro W. A., Lee W. J., Blehar D. J., Carraway R. E., et al. (2002). Myocardial preconditioning factors evoke mesenteric ischemic tolerance via opioid receptors and KATP channels. Am. J. Physiol. Heart Circ. Physiol. 283, 22–28. doi:10.1152/ajpheart.01055.2001
Durand M. J., Boerger T. F., Nguyen J. N., Alqahtani S. Z., Wright M. T., Schmit B. D., et al. (2019). Two weeks of ischemic conditioning improves walking speed and reduces neuromuscular fatigability in chronic stroke survivors. Invest. Ophthalmol. Vis. Sci. 126, 755–763. doi:10.1152/jappl
el Dabagh Y., Petersen L., Pedersen M., Bek T. (2017). The diameter of retinal vessels is affected by transient ischemia of the arm in normal persons. Invest. Ophthalmol. Vis. Sci. 58, 5263–5269. doi:10.1167/iovs.17-22461
Enko K., Nakamura K., Yunoki K., Miyoshi T., Akagi S., Yoshida M., et al. (2011). Intermittent arm ischemia induces vasodilatation of the contralateral upper limb. J. Physiol. Sci. 61, 507–513. doi:10.1007/s12576-011-0172-9
Ferreira T. N., Sabino-Carvalho J. L. C., Lopes T. R., Ribeiro I. C., Succi J. E., da Silva A. C., et al. (2016). Ischemic preconditioning and repeated sprint swimming: A placebo and nocebo study. Med. Sci. Sports Exerc. 48, 1967–1975. doi:10.1249/MSS.0000000000000977
Foster G. P., Westerdahl D. E., Foster L. A., Hsu J. v., Anholm J. D. (2011). Ischemic preconditioning of the lower extremity attenuates the normal hypoxic increase in pulmonary artery systolic pressure. Respir. Physiol. Neurobiol. 179, 248–253. doi:10.1016/j.resp.2011.09.001
Gandevia S. C. (2001). Spinal and supraspinal factors in human muscle fatigue. Physiol. Rev. 81 (4), 1725–1789. doi:10.1152/physrev.2001.81.4.1725
Gho B. C. G., Schoemaker R. G., van den Doel M. A., Duncker D. J., Verdouw P. D. (1996). Myocardial protection by brief ischemia in noncardiac tissue. Circulation 94, 2193–2200. doi:10.1161/01.CIR.94.9.2193
Gibson N., Mahony B., Tracey C., Fawkner S., Murray A. (2015). Effect of ischemic preconditioning on repeated sprint ability in team sport athletes. J. Sports Sci. 33, 1182–1188. doi:10.1080/02640414.2014.988741
Gibson N., White J., Neish M., Murray A. (2013). Effect of ischemic preconditioning on land-based sprinting in team-sport athletes. Int. J. Sports Physiol. Perform. 8, 671–676. doi:10.1123/ijspp.8.6.671
Griffin P. J., Ferguson R. A., Gissane C., Bailey S. J., Patterson S. D. (2018). Ischemic preconditioning enhances critical power during a 3 minute all-out cycling test. J. Sports Sci. 36, 1038–1043. doi:10.1080/02640414.2017.1349923
Gu X., Liu Z., Gao S., Ma L., Chen J., Wang Z., et al. (2021). The effects of ischemic preconditioning supplementation on endothelial function: A systematic review and meta-analysis. Evid. Based. Complement. Altern. Med. 2021, 6690691. doi:10.1155/2021/6690691
Hajrasouliha A. R., Tavakoli S., Ghasemi M., Jabehdar-Maralani P., Sadeghipour H., Ebrahimi F., et al. (2008). Endogenous cannabinoids contribute to remote ischemic preconditioning via cannabinoid CB2 receptors in the rat heart. Eur. J. Pharmacol. 579, 246–252. doi:10.1016/j.ejphar.2007.09.034
Halley S. L., Marshall P., Siegler J. C. (2018). The effect of ischaemic preconditioning on central and peripheral fatiguing mechanisms in humans following sustained maximal isometric exercise. Exp. Physiol. 103, 976–984. doi:10.1113/EP086981
Halley S. L., Peeling P., Brown H., Sim M., Mallabone J., Dawson B., et al. (2020). Repeat application of ischemic preconditioning improves maximal 1,000- m kayak ergometer performance in a simulated competition format. J. Strength Cond. Res. doi:10.1519/JSC.0000000000003748
Hausenloy D. J., Yellon D. M. (2008). Remote ischaemic preconditioning: Underlying mechanisms and clinical application. Cardiovasc. Res. 79, 377–386. doi:10.1093/cvr/cvn114
Heusch G., Bøtker H. E., Przyklenk K., Redington A., Yellon D. (2015). Remote ischemic conditioning. J. Am. Coll. Cardiol. 65, 177–195. doi:10.1016/j.jacc.2014.10.031
Horiuchi M., Endo J., Thijssen D. H. J. (2015). Impact of ischemic preconditioning on functional sympatholysis during handgrip exercise in humans. Physiol. Rep. 3, e12304–e12309. doi:10.14814/phy2.12304
Hughes L., Grant I., Patterson S. D. (2021). Aerobic exercise with blood flow restriction causes local and systemic hypoalgesia and increases circulating opioid and endocannabinoid levels. J. Appl. Physiol. 131, 1460–1468. doi:10.1152/japplphysiol.00543.2021
Hughes L., Patterson S. D. (2020). The effect of blood flow restriction exercise on exercise-induced hypoalgesia and endogenous opioid and endocannabinoid mechanisms of pain modulation. J. Appl. Physiol. 128, 914–924. doi:10.1152/japplphysiol.00768.2019
Hyngstrom A. S., Murphy S. A., Nguyen J., Schmit B. D., Negro F., David X., et al. (2018). Ischemic conditioning increases strength and volitional activation of paretic muscle in chronic stroke: A pilot study. J. Appl. Physiol. 124, 1140–1147. doi:10.1152/japplphysiol.01072.2017
Incognito A. V., Burr J. F., Millar P. J. (2016). The effects of ischemic preconditioning on human exercise performance. Sports Med. 46, 531–544. doi:10.1007/s40279-015-0433-5
Incognito A. V., Doherty C. J., Lee J. B., Burns M. J., Millar P. J. (2017). Ischemic preconditioning does not alter muscle sympathetic responses to static handgrip and metaboreflex activation in young healthy men. Physiol. Rep. 5, e13342. doi:10.14814/phy2.13342
Jean-St-Michel E., Manlhiot C., Li J., Tropak M., Michelsen M. M., Schmidt M. R., et al. (2011). Remote preconditioning improves maximal performance in highly trained athletes. Med. Sci. Sports Exerc. 43, 1280–1286. doi:10.1249/MSS.0b013e318206845d
Jessee M. B., Mattocks K. T., Buckner S. L., Dankel S. J., Mouser J. G., Abe T., et al. (2018). “Mechanisms of blood flow restriction: The new testament,” in Techniques in orthopaedics (Philadelphia, Pennsylvania, United States: Lippincott Williams and Wilkins), 72–79. doi:10.1097/BTO.0000000000000252
Joyner M. J., Casey D. P. (2015). Regulation of increased blood flow (hyperemia) to muscles during exercise: A hierarchy of competing physiological needs. Physiol. Rev. 95, 549–601. doi:10.1152/physrev.00035.2013
Kaufman M. P. (2012). “The exercise pressor reflex in animals,” in Experimental physiology (Hoboken, New Jersey, United States: Blackwell Publishing Ltd), 51–58. doi:10.1113/expphysiol.2011.057539
Khaliulin I., Fleishman A. N., Shumeiko N. I., Korablina T. v., Petrovskiy S. A., Ascione R., et al. (2019). Neuro-autonomic changes induced by remote ischemic preconditioning (RIPC) in healthy young adults: Implications for stress. Neurobiol. Stress 11, 100189. doi:10.1016/j.ynstr.2019.100189
Kido K., Suga T., Tanaka D., Honjo T., Homma T., Fujita S., et al. (2015). Ischemic preconditioning accelerates muscle deoxygenation dynamics and enhances exercise endurance during the work-to-work test. Physiol. Rep. 3, e12395. doi:10.14814/phy2.12395
Kilding A. E., Sequeira · G. M., Wood · M. R. (2018). Effects of ischemic preconditioning on economy, VO 2 kinetics and cycling performance in endurance athletes. Eur. J. Appl. Physiol. 118, 2541–2549. doi:10.1007/s00421-018-3979-8
Kimura M., Ueda K., Goto C., Jitsuiki D., Nishioka K., Umemura T., et al. (2007). Repetition of ischemic preconditioning augments endothelium-dependent vasodilation in humans: Role of endothelium-derived nitric oxide and endothelial progenitor cells. Arterioscler. Thromb. Vasc. Biol. 27, 1403–1410. doi:10.1161/ATVBAHA.107.143578
Kleinbongard P., Skyschally A., Heusch G. (2017). Cardioprotection by remote ischemic conditioning and its signal transduction. Pflugers Arch. 469, 159–181. doi:10.1007/s00424-016-1922-6
Koltyn K. F., Brellenthin A. G., Cook D. B., Sehgal N., Hillard C. (2014). Mechanisms of exercise-induced hypoalgesia. J. Pain 15, 1294–1304. doi:10.1016/j.jpain.2014.09.006
Konstantinov I. E., Li J., Cheung M. M., Shimizu M., Stokoe J., Kharbanda R. K., et al. (2005). Remote ischemic preconditioning of the recipient reduces myocardial ischemia-reperfusion injury of the denervated donor heart via a Katp channel-dependent mechanism. Transplantation 79, 1691–1695. doi:10.1097/01.TP.0000159137.76400.5D
Kraus A. S., Pasha E. P., Machin D. R., Alkatan M., Kloner R. A., Tanaka H. (2015). Bilateral upper limb remote ischemic preconditioning improves anaerobic power. Open Sports Med. J. 9, 1–6. doi:10.2174/1874387001509010001
Kristiansen S. B., Henning O., Kharbanda R. K., Nielsen-Kudsk J. E., Schmidt M. R., Redington A. N., et al. (2005). Remote preconditioning reduces ischemic injury in the explanted heart by a KATP channel-dependent mechanism. Am. J. Physiol. Heart Circ. Physiol. 288, 1252–1256. doi:10.1152/ajpheart.00207.2004
Lang J. A., Kim J., Lang J. A., Kim J. (2022). Remote ischaemic preconditioning-translating cardiovascular benefits to humans. J. Physiology 600, 3053–3067. doi:10.1113/jp282568
Lee H. T., Schroeder C. A., Shah P. M., Babu S. C., Thompson C. I., Belloni F. L. (1996). Preconditioning with ischemia or adenosine protects skeletal muscle from ischemic tissue reperfusion injury. J. Surg. Res. 63, 29–34. doi:10.1006/jsre.1996.0217
Liem D. A., Verdouw P. D., Ploeg H., Kazim S., Duncker D. J. (2002). Sites of action of adenosine in interorgan preconditioning of the heart. Am. J. Physiol. Heart Circ. Physiol. 283, 29–37. doi:10.1152/ajpheart.01031.2001
Lisbôa F. D., Turnes T., Cruz R. S. O., Raimundo J. A. G., Pereira G. S., Caputo F. (2017). The time dependence of the effect of ischemic preconditioning on successive sprint swimming performance. J. Sci. Med. Sport 20, 507–511. doi:10.1016/j.jsams.2016.09.008
Liu Z., Zhao Y., Lei M., Zhao G., Li D., Sun R., et al. (2021). Remote ischemic preconditioning to prevent acute kidney injury after cardiac surgery: A meta-analysis of randomized controlled trials. Front. Cardiovasc. Med. 8, 601470. doi:10.3389/fcvm.2021.601470
Lopes T. R., Sabino-Carvalho J. L., Ferreira T. H. N., Succi J. E., Silva A. C., Silva B. M. (2018). Effect of ischemic preconditioning on the recovery of cardiac autonomic control from repeated sprint exercise. Front. Physiol. 9, 1465. doi:10.3389/fphys.2018.01465
Loukogeorgakis S. P., Panagiotidou A. T., Broadhead M. W., Donald A., Deanfield J. E., MacAllister R. J. (2005). Remote ischemic preconditioning provides early and late protection against endothelial ischemia-reperfusion injury in humans: Role of the autonomic nervous system. J. Am. Coll. Cardiol. 46, 450–456. doi:10.1016/j.jacc.2005.04.044
Luca M. C., Liuni A., McLaughlin K., Gori T., Parker J. D. (2013). Daily ischemic preconditioning provides sustained protection from ischemia–reperfusion induced endothelial dysfunction: A human study. J. Am. Heart Assoc. 2, e000075. doi:10.1161/JAHA.112.000075
Manchurov V., Ryazankina N., Khmara T., Skrypnik D., Reztsov R., Vasilieva E., et al. (2014). Remote ischemic preconditioning and endothelial function in patients with acute myocardial infarction and primary PCI. Am. J. Med. 127, 670–673. doi:10.1016/j.amjmed.2014.02.012
Marcora S. (2009). Perception of effort during exercise is independent of afferent feedback from skeletal muscles, heart, and lungs. J. Appl. Physiol. 106, 2060–2062. doi:10.1152/japplphysiol.90378.2008
Marocolo M., da Mota G., Pelegrini V., Appell Coriolano H.-J. (2015a). Are the beneficial effects of ischemic preconditioning on performance partly a placebo effect? Int. J. Sports Med. 36, 822–825. doi:10.1055/s-0035-1549857
Marocolo M., da Mota G. R., Simim M. A. M., Appell Coriolano H. J. (2015b). Myths and facts about the effects of ischemic preconditioning on performance. Int. J. Sports Med. 37, 87–96. doi:10.1055/s-0035-1564253
Marocolo M., Simim M. A. M., Bernardino A., Monteiro I. R., Patterson S. D., da Mota G. R. (2019). Ischemic preconditioning and exercise performance: Shedding light through smallest worthwhile change. Eur. J. Appl. Physiol. 119, 2123–2149. doi:10.1007/s00421-019-04214-6
Marocolo M., Willardson J. M., Marocolo I. C., Ribeiro Da Mota G., Simão R., Maior A. S. (2016). Ischemic preconditioning and placebo intervention improves resistance exercise performance. J. Strength Cond. Res. 30, 1462–1469. doi:10.1519/JSC.0000000000001232
Mieszkowski J., Stankiewicz B., Kochanowicz A., Niespodziński B., Borkowska A., Antosiewicz J. (2020). Effect of ischemic preconditioning on marathon-induced changes in serum exerkine levels and inflammation. Front. Physiol. 11, 571220. doi:10.3389/fphys.2020.571220
Morley W. N., Coates A. M., Burr J. F. (2021). Cardiac autonomic recovery following traditional and augmented remote ischemic preconditioning. Eur. J. Appl. Physiol. 121, 265–277. doi:10.1007/s00421-020-04526-y
Moro L., Pedone C., Mondì A., Nunziata E., Antonelli Incalzi R., Mondi A. (2011). Effect of local and remote ischemic preconditioning on endothelial function in young people and healthy or hypertensive elderly people. Atherosclerosis 219, 750–752. doi:10.1016/j.atherosclerosis.2011.08.046
Mota G. R., Rightmire Z. B., Martin J. S., McDonald J. R., Kavazis A. N., Pascoe D. D., et al. (2020). Ischemic preconditioning has no effect on maximal arm cycling exercise in women. Eur. J. Appl. Physiol. 120, 369–380. doi:10.1007/s00421-019-04281-9
Mulliri G., Sainas G., Magnani S., Palazzolo G., Milia N., Orrù A., et al. (2016). Ischemic preconditioning reduces hemodynamic response during metaboreflex activation. Am. J. Physiol. Regul. Integr. Comp. Physiol. 310, R777–R787. doi:10.1152/ajpregu.00429.2015
Murase S., Terazawa E., Queme F., Ota H., Matsuda T., Hirate K., et al. (2010). Bradykinin and nerve growth factor play pivotal roles in muscular mechanical hyperalgesia after exercise (Delayed-onset muscle soreness). J. Neurosci. 30, 3752–3761. doi:10.1523/JNEUROSCI.3803-09.2010
Murry C. E., Jennings R. B., Reimer K. A. (1986). Preconditioning with ischemia: A delay of lethal cell injury in ischemic myocardium. Circulation 74, 1124–1136. doi:10.1161/01.CIR.74.5.1124
Noble B. J., Borg G. A. V., Jacobs I., Ceci R., Kaiser P. (1983). A category-ratio perceived exertion scale: Relationship to blood and muscle lactates and heart rate. Med. Sci. Sports Exerc. 15, 523–528. doi:10.1249/00005768-198315060-00015
Nobrega A. C. L., O’Leary D., Silva B. M., Marongiu E., Piepoli M. F., Crisafulli A. (2014). Neural regulation of cardiovascular response to exercise: Role of central command and peripheral afferents. Biomed. Res. Int. 2014, 478965. doi:10.1155/2014/478965
Noordhof D. A., de Koning J. J., Foster C. (2010). The maximal accumulated oxygen deficit method: A valid and reliable measure of anaerobic capacity? Sports Med. 40, 285–302. doi:10.2165/11530390-000000000-00000
O’Brien L., Jacobs I. (2021). Methodological variations contributing to heterogenous ergogenic responses to ischemic preconditioning. Front. Physiol. 12, 656980. doi:10.3389/fphys.2021.656980
O’Connor P. J., Cook D. B. (1999). Exercise and pain the neurobiology, measurement, and laboratory study of pain in relation to exercise in humans. Exerc. Sport Sci. Rev. 27, 119–166.
Pagliaro P., Chiribiri A., Mancardi D., Rastaldo R., Gattullo D., Losano G. (2003). Coronary endothelial dysfunction after ischemia and reperfusion and its prevention by ischemic preconditioning. Ital. Heart J. 4, 383–394. Available at: http://www.ncbi.nlm.nih.gov/pubmed/12898803 (Accessed March 19, 2020).
Pang C. Y., Yang R. Z., Zhong A., Xu N., Boyd B., Forrest C. R. (1995). Acute ischaemic preconditioning protects against skeletal muscle infarction in the pig. Cardiovasc. Res. 29, 782–788. doi:10.1016/0008-6363(96)88613-5
Panza P., Novaes J., Telles L. G., Campos Y., Araújo G., Neto N., et al. (2020). Ischemic preconditioning promotes post-exercise hypotension in a session of resistance exercise in normotensive trained individuals. Int. J. Environ. Res. Public Health 17, E78. doi:10.3390/ijerph17010078
Paradis-Deschênes P., Joanisse D. R., Billaut F. (2018). Ischemic preconditioning improves time trial performance at moderate altitude. Med. Sci. Sports Exerc. 50, 533–541. doi:10.1249/MSS.0000000000001473
Paradis-Deschênes P., Joanisse D. R., Billaut F. (2016). Ischemic preconditioning increases muscle perfusion, oxygen uptake, and force in strength-trained athletes. Appl. physiology, Nutr. metabolism 41, 938–944. doi:10.1139/apnm-2015-0561
Paradis-Deschênes P., Joanisse D. R., Billaut F. (2017). Sex-specific impact of ischemic preconditioning on tissue oxygenation and maximal concentric force. Front. Physiol. 7, 674–679. doi:10.3389/fphys.2016.00674
Paradis-Deschênes P., Lapointe J., Joanisse D. R., Billaut F. (2020). Similar recovery of maximal cycling performance after ischemic preconditioning, neuromuscular electrical stimulation or active recovery in endurance athletes. J. Sports Sci. Med. 19, 761–771.
Patel H. H., Moore J., Hsu A. K., Gross G. J. (2002). Cardioprotection at a distance: Mesenteric artery occlusion protects the myocardium via an opioid sensitive mechanism. J. Mol. Cell. Cardiol. 34, 1317–1323. doi:10.1006/jmcc.2002.2072
Patterson S. D., Bezodis N. E., Glaister M., Pattison J. R. (2015). The effect of ischemic preconditioning on repeated sprint cycling performance. Med. Sci. Sports Exerc. 47, 1652–1658. doi:10.1249/MSS.0000000000000576
Patterson S. D., Hughes L., Warmington S., Burr J., Scott B. R., Owens J., et al. (2019). Blood flow restriction exercise: Considerations of methodology, application, and safety. Front. Physiol. 10, 533. doi:10.3389/fphys.2019.00533
Patterson S. D., Swan R., Page W., Marocolo M., Jeffries O., Waldron M. (2021). The effect of acute and repeated ischemic preconditioning on recovery following exercise-induced muscle damage. J. Sci. Med. Sport 24, 709–714. doi:10.1016/j.jsams.2021.02.012
Paull E. J., van Guilder G. P. (2019). Remote ischemic preconditioning increases accumulated oxygen deficit in middle-distance runners. J. Appl. Physiol. 126, 1193–1203. doi:10.1152/japplphysiol.00585.2018
Pereira H. M., de Lima F. F., Silva B. M., Kohn A. F. (2020). Sex differences in fatigability after ischemic preconditioning of non-exercising limbs. Biol. Sex. Differ. 11, 59. doi:10.1186/s13293-020-00338-z
Pérez-Pinzón M. A. (2004). Neuroprotective effects of ischemic preconditioning in brain mitochondria following cerebral ischemia. J. Bioenerg. Biomembr. 36, 323–327. doi:10.1023/B:JOBB.0000041762.47544.ff
Pethick J., Casselton C., Winter S. L., Burnley M. (2021). Ischemic preconditioning blunts loss of knee extensor torque complexity with fatigue. Med. Sci. Sports Exerc. 53, 306–315. doi:10.1249/MSS.0000000000002475
Philips H. C., Jahanshahi M. (1986). The components of pain behaviour report. Behav. Res. Ther. 24, 117–125. doi:10.1016/0005-7967(86)90082-3
Rassaf T., Totzeck M., Hendgen-Cotta U. B., Shiva S., Heusch G., Kelm M. (2014). Circulating nitrite contributes to cardioprotection by remote ischemic preconditioning. Circ. Res. 114, 1601–1610. doi:10.1161/CIRCRESAHA.114.303822
Richard P., Billaut F. (2018). Time-trial performance in elite speed skaters after remote ischemic preconditioning. Int. J. Sports Physiol. Perform. 13, 1308–1316. doi:10.1123/ijspp.2018-0111
Roseguini B. T., Laughlin M. H. (2018). “Muscle blood flow and vascularization in response to exercise and training,” in Muscle and exercise physiology (Amsterdam, Netherlands: Elsevier), 379–389. doi:10.1016/B978-0-12-814593-7.00017-7
Rosenberry R., Nelson M. D. (2020). Reactive hyperemia: A review of methods, mechanisms, and considerations. Am. J. Physiol. Regul. Integr. Comp. Physiol. 318, R605–R618. doi:10.1152/AJPREGU.00339.2019
Rowell L. B., O’Leary D. S. (1990). Reflex control of the circulation during exercise: Chemoreflexes and mechanoreflexes. J. Appl. Physiol. 69, 407–418. doi:10.1152/jappl.1990.69.2.407
Sabino-Carvalho J. L., Lopes T. R., Obeid-Freitas T., Ferreira T. N., Succi J. E., Silva A. C., et al. (2017). Effect of ischemic preconditioning on endurance performance does not surpass placebo. Med. Sci. Sports Exerc. 49, 124–132. doi:10.1249/MSS.0000000000001088
Sabino-Carvalho J. L., Obeid-Freitas T., Paula-Ribeiro M., Lopes T. R., Ferreira T. H. N., Succi J. E., et al. (2019). Ischemic preconditioning boosts post-exercise but not resting cardiac vagal control in endurance runners. Eur. J. Appl. Physiol. 119, 621–632. doi:10.1007/s00421-018-4052-3
Salvador A. F., de Aguiar R. A., Lisbôa F. D., Pereira K. L., de Cruz R. S. O., Caputo F. (2016). Ischemic preconditioning and exercise performance: A systematic review and meta-analysis. Int. J. Sports Physiol. Perform. 11, 4–14. doi:10.1123/ijspp.2015-0204
Schoemaker R. G., van Heijningen C. L. (2000). Bradykinin mediates cardiac preconditioning at a distance. Am. J. Physiol. Heart Circ. Physiol. 278 (5), 1571–1576. doi:10.1152/ajpheart.2000.278.5.H1571
Seeger J. P. H., Timmers S., Ploegmakers D. J. M., Cable N. T., Hopman M. T. E., Thijssen D. H. J. (2017). Is delayed ischemic preconditioning as effective on running performance during a 5 km time trial as acute IPC? J. Sci. Med. Sport 20, 208–212. doi:10.1016/j.jsams.2016.03.010
Serejo F. C., Rodrigues L. F., da Silva Tavares K. C., de Carvalho A. C. C., Nascimento J. H. M. (2007). Cardioprotective properties of humoral factors released from rat hearts subject to ischemic preconditioning. J. Cardiovasc. Pharmacol. 49, 214–220. doi:10.1097/FJC.0b013e3180325ad9
Sgherza A. L., Axen K., Fain R., Hoffman R. S., Dunbar C. C., Ois Haas F. (2002). Effect of naloxone on perceived exertion and exercise capacity during maximal cycle ergometry. J. Appl. Physiol. 93, 2023–2028. doi:10.1152/japplphysiol.00521.2002
Sidhu S. K., Cresswell A. G., Carroll T. J. (2013). Corticospinal responses to sustained locomotor exercises: Moving beyond single-joint studies of central fatigue. Sports Med. 43, 437–449. doi:10.1007/s40279-013-0020-6
Singh L., Randhawa P. K., Singh N., Jaggi A. S. (2017). Redox signaling in remote ischemic preconditioning-induced cardioprotection: Evidences and mechanisms. Eur. J. Pharmacol. 809, 151–155. doi:10.1016/j.ejphar.2017.05.033
Slysz J. T., Burr J. F. (2018). Enhanced metabolic stress augments ischemic preconditioning for exercise performance. Front. Physiol. 9, 1621–1628. doi:10.3389/fphys.2018.01621
Slysz J. T., Burr J. F. (2021). Ischemic preconditioning: Modulating pain sensitivity and exercise performance. Front. Physiol. 12, 1621. doi:10.3389/fphys.2018.01621
Slysz J. T., Petrick H. L., Marrow J. P., Burr J. F. (2019). An examination of individual responses to ischemic preconditioning and the effect of repeated ischemic preconditioning on cycling performance. Eur. J. Sport Sci. 0, 633–640. doi:10.1080/17461391.2019.1651401
Tanaka D., Suga T., Kido K., Honjo T., Hamaoka T., Isaka T. (2020). Acute remote ischemic preconditioning has no effect on quadriceps muscle endurance. Transl. Sports Med. 3, 314–320. doi:10.1002/tsm2.149
Telles L. G., Billaut F., de Souza Ribeiro A., Junqueira C. G., Leitão L., Barreto A. C., et al. (2022). Ischemic preconditioning with high and low pressure enhances maximum strength and modulates heart rate variability. Int. J. Environ. Res. Public Health 19, 7655. doi:10.3390/ijerph19137655
da Silva Telles L. G., Vianna J. M., Kingsley J. D., Araújo G., de Souza Ribeiro A. A., Monteiro E. R., et al. (2021). Ischemic preconditioning improves autonomic modulation after session of resistance exercise. bjdv. 7, 85451–85470. doi:10.34117/bjdv7n8-652
Thoren P., Floras J. S., Hoffmann P., Seals D. R. (1990). Endorphins and exercise: Physiological mechanisms and clinical implications. Med. Sci. Sports Exerc. 22, 417–428. doi:10.1249/00005768-199008000-00001
Tocco F., Marongiu E., Ghiani G., Sanna I., Palazzolo G., Olla S., et al. (2015). Muscle ischemic preconditioning does not improve performance during self-paced exercise. Int. J. Sports Med. 36, 9–15. doi:10.1055/s-0034-1384546
Tomai F., Crea F., Chiariello L., Gioffrè P. A. (1999). Ischemic preconditioning in humans: Models, mediators, and clinical relevance. Circulation 100 (5), 559–563. doi:10.1161/01.cir.100.5.559
Tschakovsky M. E., Pyke K. E. (2010). Control of skeletal muscle microcirculation in exercise. Editor S. P. Philippe Connes (Amsterdam, Netherlands: Olivier Hue IOS Press). doi:10.3233/978-1-60750-497-9-192
Tucker R. (2009). The anticipatory regulation of performance: The physiological basis for pacing strategies and the development of a perception-based model for exercise performance. Br. J. Sports Med. 43, 392–400. doi:10.1136/bjsm.2008.050799
Turnes T., de Aguiar R. A., de Oliveira Cruz R. S., Salvador A. F., Lisbôa F. D., Pereira K. L., et al. (2018). Impact of ischaemia–reperfusion cycles during ischaemic preconditioning on 2000-m rowing ergometer performance. Eur. J. Appl. Physiol. 118, 1599–1607. doi:10.1007/s00421-018-3891-2
Wang M., Qi D. S., Zhou C., Han D., Li P. P., Zhang F., et al. (2016). Ischemic preconditioning protects the brain against injury via inhibiting CaMKII-nNOS signaling pathway. Brain Res. 1634, 140–149. doi:10.1016/j.brainres.2016.01.008
Weinbrenner C., Nelles M., Herzog N., Sárváry L., Strasser R. H. (2002). Remote preconditioning by infrarenal occlusion of the aorta protects the heart from infarction: A newly identified non-neuronal but PKC-dependent pathway. Cardiovasc. Res. 55, 590–601. doi:10.1016/S0008-6363(02)00446-7
Keywords: ischemic preconditioning, exercise, mechanism, IPC exercise, athletic performance, ergogenic aid, physiology, sport science
Citation: O’Brien L and Jacobs I (2022) Potential physiological responses contributing to the ergogenic effects of acute ischemic preconditioning during exercise: A narrative review. Front. Physiol. 13:1051529. doi: 10.3389/fphys.2022.1051529
Received: 22 September 2022; Accepted: 15 November 2022;
Published: 28 November 2022.
Edited by:
Hun-Young Park, Konkuk University, South KoreaReviewed by:
Wonil Park, Chung-Ang University, South KoreaHyoseong Yeo, Seoul National University Bundang Hospital, South Korea
Copyright © 2022 O’Brien and Jacobs. This is an open-access article distributed under the terms of the Creative Commons Attribution License (CC BY). The use, distribution or reproduction in other forums is permitted, provided the original author(s) and the copyright owner(s) are credited and that the original publication in this journal is cited, in accordance with accepted academic practice. No use, distribution or reproduction is permitted which does not comply with these terms.
*Correspondence: Ira Jacobs, SXJhLmphY29ic0B1dG9yb250by5jYQ==