- 1Department of Physiology, Faculty of Medicine, Hunan University of Chinese Medicine, Changsha, Hunan, China
- 2Hunan Key Laboratory of Translational Research in Formulas and Zheng of Traditional Chinese Medicine, Hunan University of Chinese Medicine, Changsha, Hunan, China
- 3Department of Internal Medicine, College of Integrated Chinese and Western Medicine, Hunan University of Chinese Medicine, Changsha, Hunan, China
- 4State Key Laboratory of Quality Research in Chinese Medicine, Macau Institute for Applied Research in Medicine and Health, Macau University of Science and Technology, Macau, Macau SAR, China
- 5Department of Liver Diseases, The First Hospital of Hunan University of Chinese Medicine, Changsha, Hunan, China
- 6Department of Andrology, The First Hospital of Hunan University of Chinese Medicine, Changsha, Hunan, China
Gastrointestinal cancer may be associated with dysbiosis, which is characterized by an alteration of the gut microbiota. Understanding the role of gut microbiota in the development of gastrointestinal cancer is useful for cancer prevention and gut microbiota-based therapy. However, the potential role of dysbiosis in the onset of tumorigenesis is not fully understood. While accumulating evidence has demonstrated the presence of dysbiosis in the intestinal microbiota of both healthy individuals and patients with various digestive system diseases, severe dysbiosis is often present in patients with digestive system cancer. Importantly, specific bacteria have been isolated from the fecal samples of these patients. Thus, the association between dysbiosis and the development of digestive system cancer cannot be ignored. A new model describing this relationship must be established. In this review, we postulate that dysbiosis serves as the first hit for the development of digestive system cancer. Dysbiosis-induced alterations, including inflammation, aberrant immune response, bacteria-produced genotoxins, and cellular stress response associated with genetic, epigenetic, and/or neoplastic changes, are second hits that speed carcinogenesis. This review explains the mechanisms for these four pathways and discusses gut microbiota-based therapies. The content included in this review will shed light on gut microbiota-based strategies for cancer prevention and therapy.
Introduction
Dysbiosis, defined as perturbations in the quality or quantity of gut microbiota, has recently emerged as a crucial pathophysiological factor for cancer onset and progression, especially digestive system cancer (Ponziani et al., 2019; Sobhani et al., 2019; Yachida et al., 2019; Huang et al., 2020; Jia et al., 2021; Yang et al., 2021; Kartal et al., 2022). Unlike gastric cancer and cervical cancer, which are induced by single pathogenic agents, the pattern of gut microbiota-associated carcinogenesis remains elusive. Helicobacter pylori (Hatakeyama, 2014; Amieva and Peek, 2016) and human papillomavirus (HPV) (Marx, 1986; Clifford et al., 2017) are widely accepted as the etiologic agents of gastric cancer and cervical cancer, respectively. Long-term infection by H. pylori is the strongest risk factor for both intestinal and diffuse gastric non-cardia carcinomas. In addition, H. pylori eradication is the standard therapy for gastric cancer prevention (Yan et al., 2022). Similarly, persistent infection with oncogenic HPV types is strongly associated with the development of cervical cancer. HPV vaccination is an effective method of cervical cancer prevention (Wang et al., 2020a; Printz, 2021) to significantly reduce morbidity and mortality due to cervical cancer. Therefore, the causality of microorganisms demonstrated in gastric cancer and cervical cancer leads us to consider the possible roles of gut microbiota in digestive system cancer.
In the clinic, only a few bacterial species have been associated with increased cancer morbidity, including Fusobacterium nucleatum (Yu et al., 2017; Kong et al., 2021; Chen et al., 2022). Monitoring GI microbiota is also not included in therapeutic guidelines for digestive system cancer, although it is recommended as the standard therapy for Clostridioides difficile infection in adults (Johnson et al., 2021; van Prehn et al., 2021). Despite this, a strong correlation exists between dysbiosis and digestive system cancer carcinogenesis, as confirmed by numerous preclinical and clinical studies. Composition shifts of gut microbiota have been reported in patients with colorectal cancer (CRC) (Castellarin et al., 2012; Yachida et al., 2019; Jia et al., 2021; Yang et al., 2021) and hepatocellular carcinoma (HCC) (Jia et al., 2021; Ray, 2021; Schneider et al., 2022). Fecal transplantation from CRC model mice to control mice identified cancer-related biochemical or behavioral changes in the recipient (Wong et al., 2017; Li et al., 2019; Chang et al., 2020). These findings lead to questions regarding the potential role of dysbiosis on the onset of digestive system cancer. Based on collective evidence, we propose that dysbiosis works as the first hit on the development of digestive system cancer. The presence of dysbiosis (the first hit) will not guarantee carcinogenesis unless dysbiosis-associated alterations (second hits) also occur. This review discusses the presence of dysbiosis in digestive system cancer and how dysbiosis-associated alterations can potentiate carcinogenesis. Microbiota-based cancer therapies are also described.
Normal gut microbiota
Trillions of microbes have been identified in the normal adult digestive system tract, including facultative anaerobes (e.g., Lactobacilli and Enterobacteria) and strict anaerobes (e.g., Bacteroides and Bifidobacterium) (Lloyd-Price et al., 2016). Firmicutes and Bacteroidetes are major phyla in a healthy gut (>90%). Other phyla include Proteobacteria (<5%), Actinobacteria (<2%), Verrucomicrobia, and Fusobacteria (<1%), and others. The gut microbiota is dynamic and varies among individuals. Furthermore, gut microbiota can be influenced by environmental factors, diet, and medications (Zmora et al., 2019; Lindell et al., 2022). Recent research has demonstrated the important role gut microbiota play in several physiological processes, including immune system development and maturation (Rooks and Garrett, 2016; Song et al., 2020; Paik et al., 2022), bile acid metabolism (Funabashi et al., 2020; Poland and Flynn, 2021), energy consumption (Coelho et al., 2019; Miyamoto et al., 2019), and neurotransmitter biosynthesis (Yano et al., 2015; Choi et al., 2020). Therefore, dysbiosis may contribute to various diseases, including digestive system cancer. As aforementioned, while no single genus has been associated with intestinal cancer, dysbiosis of gut microbiota has been observed and may be the first hit for gastrointestinal cancer. Thus, this dysbiosis may be a better diagnostic marker or a potential therapeutic target.
The first hit: Perturbance of gut microbiota in digestive system cancer
Dysbiosis of gut microbiota in CRC
CRC is a heterogeneous group of cancers that mostly develop from polyps (neoplastic precursor lesions). CRC accounted for approximately 10% of all diagnosed cancers and cancer-related deaths worldwide in 2020 (Sung et al., 2021). Hereditary (e.g., hereditary colorectal cancer syndromes and APC mutations) and environmental risk factors (e.g., low intake of vegetables and fruits, high body fat, and obesity) play roles in CRC development. Male sex and increasing age are also positively associated with CRC incidence.
Recently, microbiome profiling via 16S rRNA or shotgun metagenomics of stool samples confirmed dysbiosis in patients with CRC (Dai et al., 2018; Thomas et al., 2019; Coker et al., 2022). The representation of three bacterial species is consistently increased in patients with CRC, including F. nucleatum, Enterotoxigenic bacteroides fragilis (ETBF), and Escherichia coli with the pks genetic island (pks + E. coli) (Table 1). Other non-specific genera include at least 27 genera, including Porphyromonas, Peptostreptococcus, and Prevotella, and the order Clostridiales (Wirbel et al., 2019). In addition, dysbiosis also relates to certain precursor lesions in CRC, including the adenocarcinoma (70–90%) and serrated neoplasia (10–20%) pathways. Clinical tests have shown significant decreases in microbial diversity, overall composition, and normalized taxon abundance in these two precursors, especially in patients with advanced traditional adenoma-carcinoma (Peters et al., 2016). Clostridia operational taxonomic units are depleted in these patients, whereas classes Gammaproteobacteria and Bacilli, order Enterobacteriales, and genera Streptococcus and Actinomyces were enriched. Therefore, some intestinal bacteria may be biomarkers for CRC. Multi-omics analysis from a CRC cohort showed a significantly increased abundance of F. nucleatum spp. from intramucosal carcinoma to more advanced stages, whereas Atopobium parvulum and Actinomyces odontolyticus were only dominant in multiple polypoid adenomas and/or intramucosal carcinomas (Yachida et al., 2019).
Gut microbiota is also correlated with CRC therapy. In neoadjuvant chemoradiotherapy (nCRT) for locally advanced rectal cancer (LARC), the gut microbiota in patients with effective response differed significantly from those in non-responders (Yi et al., 2021). Some butyrate-producing bacteria, including Roseburia, Dorea, and Anaerostipes, were dominant in responders, whereas non-responders showed increased Coriobacteriaceae and Fusobacterium. Gut microbiota can metabolize some of the chemotherapeutic drugs, thus regulating the response to chemotherapy (Chattopadhyay et al., 2021). Bacterial species, such as F. nucleatum, are also associated with CRC recurrence (Yi et al., 2021). F. nucleatum-positive patients after nCRT treatment showed a depletion of CD8+ T cells and may be at a higher risk of recurrence (Serna et al., 2020).
In contrast, some bacterial species may play anti-tumorigenic roles in CRC. Faecalibaculum rodentium (Holdemanella biformis in humans) reduced tumor growth in a mouse intestinal tumor model by producing short-chain fatty acids (SCFAs) (Zagato et al., 2020). SCFAs control protein acetylation and tumor cell proliferation by suppressing calcineurin/NFATc3 activity. Similarly, oral gavage of Streptococcus thermophilus significantly prevented tumor formation in two mouse models of intestinal tumors by activating oxidative phosphorylation and downregulating Hippo pathway kinases (Li et al., 2021). CRC cells co-incubated with Streptococcus thermophilus or its conditioned medium showed decreased proliferation rate via produced β-galactosidase in vitro. The anti-tumor effect of S. thermophilus was also attributed to the increased abundance of commensal bacteria such as Bifidobacterium and Lactobacillus. Other bacterial species showing a protective effect on CRC include Akkermansia muciniphila (Fan et al., 2021), Clostridiales (Montalban-Arques et al., 2021), Lactobacillus reuteri (Bell et al., 2022), and Bacillus toyonensis (Chen et al., 2020).
Dysbiosis of gut microbiota in HCC
Liver cancer is another global health challenge that includes hepatocellular carcinoma (HCC) and intrahepatic cholangiocarcinoma (iCCA). HCC comprises the majority of primary liver cancer cases (90%) and is the third leading cause of cancer-related deaths worldwide (Sung et al., 2021). It usually develops from a series of risk factors, such as susceptibility genes (TERT mutation), viral risk factors (HBV and HCV infection), alcohol-induced liver disease (alcoholic cirrhosis), or non-alcoholic disease (non-alcoholic fatty liver disease, NAFLD).
Accumulating evidence indicates the association of dysbiosis in HCC development (Table 2). A recent long-term, large-scale study identified gut microbiota instability mostly related to factors contributing to metabolic syndrome, such as fatty liver disease (FLD) and diabetes mellitus (Frost et al., 2021). A total increase of facultative pathogens (e.g., Enterobacteriaceae, Escherichia, and Shigella) was observed in patients with FLD and was more evident in newly developed cases. FLD is a well-known precursor disease for HCC. The gut microbiota also involve in NAFLD development and progression (Borrelli et al., 2018; Lang and Schnabl, 2020; Behary et al., 2021); thus, they are related to NAFLD-induced HCC. One mechanism of NAFLD is closely linked to endogenous alcohol production (autobrewery syndrome or gut fermentation syndrome), which leads to nonalcoholic steatohepatitis (NASH). Intestinal bacteria isolated from patients with NASH showed an increase in Klebsiella pneumoniae strains (Yuan et al., 2019) with varied alcohol-producing activities. The close relationship between dysbiosis and HCC precursor diseases (e.g., FLD, cirrhosis, alcohol dependence syndrome, and alcoholic liver cirrhosis) indicates that the tumor-inducible role of dysbiosis is precursor-dependent. In addition, the composition of gut microbiota varies in patients with different types of precursors. In patients with HCC-cirrhosis, the predominant bacteria are Clostridium and CF231 (a member of the Paraprevotellaceae family) (Lapidot et al., 2020), compared to Clostridiales and Bacteroidales in alcohol dependence syndrome and alcoholic liver cirrhosis (Dubinkina et al., 2017).
Preclinical models have also reported the correlation between dysbiosis and HCC. Generally, HCC with icterus was induced by the consumption of soluble fibers in a series of dysbiotic mice. However, germ-free (GF) mice (without gut microbiota) or antibiotics-treated mice (with decreased gut microbiota) were resistant to such diets and HCC was not stimulated (Singh et al., 2018). Depletion of fermenting bacteria by antibiotics or inhibiting fermentation by plant-derived β-acids prevented HCC progression. In another study, antibiotic treatment decreased liver tumor growth in the primary liver and liver metastasis models by recruiting CXCL16, a regulator of natural killer T cell (NKT) accumulation (Ma et al., 2018).
Dysbiosis of gut microbiota in other digestive system cancers
Limited evidence exists regarding dysbiosis and other digestive system cancers, i.e., esophageal cancer, gastric cancer, and pancreatic cancer (PC). In esophageal cancer, oral or esophageal microbiota, instead of gut microbiota, have been recognized as cancer-related microbial factors. Similarly, the effect of gut microbiota on gastric cancer is negligible. However, gut microbiota may crosstalk with H. pylori or gastric microbiota, which are carcinogens for gastric cancer. However, the correlation between gut microbiota and PC is a new research area that emerged in 2017. A recent study reported a fecal microbiota signature in pancreatic cancer (Kartal et al., 2022). Thus, unique microbiota may be used as non-invasive biomarkers for the early detection of pancreatic ductal adenocarcinoma (PDAC). However, further studies are needed to confirm this role.
The information above suggests that the order prevalence of dysbiosis and various types of digestive cancer is CRC > HCC > esophageal cancer > gastric cancer > PC, consistent with PubMed search results. Searches for studies on “gut microbiota and CRC” and “gut microbiota and HCC” indexed in PubMed in the last 5 years revealed 1,458 and 262 articles, respectively. These comprise the first and second most common cancer types among digestive system cancer. Therefore, the following sections mainly focus on CRC and HCC.
The second hit: Dysbiosis-associated alterations potentiate carcinogenesis in the digestive system
To potentiate carcinogenesis in the digestive system, dysbiosis (the first hit) usually works with other dysbiosis-induced alterations (second hits), including inflammation, immune response, bacteria-produced genotoxins, and cellular stress response associated with genetic, epigenetic and/or neoplastic changes. These alterations together with dysbiosis serve as the sequential hits for the digestive system and speed carcinogenesis in the intestine and liver.
Dysbiosis drives inflammation
Inflammation is an evolutionarily conserved process involving the activation, recruitment, and action of the innate and adaptive immune systems. Initially, inflammation is an essential host defense against pathogens and the regulation of tissue homeostasis (repair, regeneration, and remodeling). In recent past decades, increased attention has been paid to the contribution of inflammation to cancer development and progression. In the intestine and liver, inflammation can be induced by bacteria-derived metabolites, bile acids, and bacterial components.
Bacteria-derived metabolites
The major inflammation-related metabolites produced by gut microbes are trimethylamine N-oxide (TMAO) and SCFAs. TMAO is a converted trimethylamine byproduct from the metabolism of dietary phosphatidylcholine, choline, and carnitine. An elevated serum TMAO level is highly related to cancer, especially CRC. In a nested case–control study (Guertin et al., 2017), men with higher serum choline, the precursor of TMAO, had an approximately three-fold higher risk of developing CRC over the ensuing 14 ± 10 years. In an obesity-associated CRC cohort, the composition of gut microbiota confirmed by 16S rRNA gene sequences differs from that of the CRC cohort without obesity (Sánchez-Alcoholado et al., 2020). A higher abundance of opportunistic pathogens was observed in the obesity cohort with an increased TMAO and proinflammatory cytokine IL-1. The dysbiotic bacteria included Fusobacterium, Clostridium, Prevotella, Desulfovibrio, and Enterococcus. SCFAs were the major products fermented by intestinal bacteria from indigestible dietary components. Acetate, propionate, and butyrate are three major SCFAs. The Bacteroidetes phylum is mainly responsible for acetate and propionate production, whereas the Firmicutes phylum produces butyrate. Generally, SCFAs have a protective effect on cancer. Decreased SCFA production is associated with increased CRC risks in healthy individuals or patients with CRC-related diseases (Ou et al., 2013). SCFAs help to maintain intestinal integrity by suppressing histone deacetylases (HDACs) in colonic epithelial and immune cells, resulting in decreased pro-inflammatory cytokine release (Chang et al., 2014) and increased apoptosis in CRC cells (Buda et al., 2003). Several preclinical studies have confirmed the anti-tumor role of SCFAs in CRC and HCC (Sheng et al., 2017; Tian et al., 2018). The oral gavage of acetate, butyrate, and propionate in a colitis-associated CRC mouse model significantly decreased tumor size. This protective effect depended on the suppression of pro-inflammatory cytokines, including IL-6, TNF-α, and IL-17. Analysis of the disease activity index further confirmed decreased CRC activity in the group administered SCFAs. Similarly, the administration of mixed SCFAs to HBx (an HBV-encoded oncoprotein) transgenic mice prevented HCC development. The model mice with SCFAs showed fewer tumor nodules and increased expression of disabled homolog 2 (DAB2), a tumor suppressor (McBrearty et al., 2021).
Bile acids
Bile acids (BAs), especially secondary BAs, are another gut microbiota-produced product associated with CRC and HCC. Generally, primary BAs are synthesized in the liver from cholesterol. BAs are then secreted into the intestinal lumen to undergo further biotransformation by gut microbiota. In the intestine, BAs are unconjugated by intestinal bacteria-produced bile salt hydrolase (BSH) and biotransformed into secondary BAs via bacteria-mediated 7α-dehydroxylation or epimerization. Therefore, the gut microbiota is directly involved in BA biosynthesis and dysbiosis-related alterations in BA composition are related to intestinal and hepatic inflammation. CRC and HCC cohorts both showed close relationships between circulating BAs and carcinogenesis. In one CRC cohort (569 CRC cases and 569 matched controls) (Kühn et al., 2020), a higher CRC risk was associated with increased serum levels of conjugated BA metabolites, including glycocholic acid (GCA), taurochenodeoxycholic acid (TCDCA), taurocholic acid (TCA), glycohyocholic acid (GHCA), glycochenodeoxycholic acid (GCDCA), glycodeoxycholic acid (GDCA), and taurodeoxycholic acid (TDCA). In an HCC cohort (233 pairs of HCC cases and controls), a positive correlation was observed between HCC and overall serum BAs and taurine- or choline-conjugated BAs (Stepien et al., 2021). Increased deoxycholic acid (DCA) has a deleterious effect on intestinal cancer cells by promoting the production of pro-inflammatory cytokines (Liu et al., 2018). Moreover, oral gavage of DCA in a mouse model of intestinal tumors resulted in significant increases in adenoma number and size. Elevated levels of pro-inflammatory cytokines (e.g., IL-1β, IL-6, and TNF-α) and NLRP3 inflammasome-associated proteins (e.g., NOD-like receptor family) were also observed in the DCA-treated group.
The gut microbiota also regulates BA metabolism through the nuclear farnesoid X receptor (FXR) (Jia et al., 2018; Sun et al., 2021) (Figure 1). FXR is a crucial BA receptor (the other is membrane G protein-coupled receptor 5, TGR5) and is closely related to CRC and HCC. Unconjugated BAs (e.g., chenodeoxycholic acid, CDCA; deoxycholic acid, DCA; lithocholic acid, LCA; and cholic acid, CA) are bacteria-produced high-affinity agonists of FXR. The rank order for the ability of BAs to activate FXR is CDCA > DCA > LCA > CA. FXR levels are closely correlated with CRC and HCC. Several FXR disruption studies have confirmed the role of FXR in the initiation of colon or hepatocellular carcinogenesis. In a CRC mouse model, whole-body FXR depletion resulted in increased expression of inflammation-related genes, lymphoid nodule numbers, and intestinal crypt heights, as well as fewer differentiated goblet cells. These morphological and genetic changes reveal an increased susceptibility for CRC in the mouse model (Modica et al., 2008). Similarly, Fxr-null mice spontaneously developed hepatocellular adenomas and carcinomas with increased circulating and hepatic BA levels, pro-inflammatory cytokines, and myelocytomatosis oncogene (Yang et al., 2007). In contrast, overexpression of FXR by adenovirus injection inhibited xenograft growth in nude mice. Colon cancer cells co-cultured with FXR agonists showed suppressed cell proliferation (Peng et al., 2012).
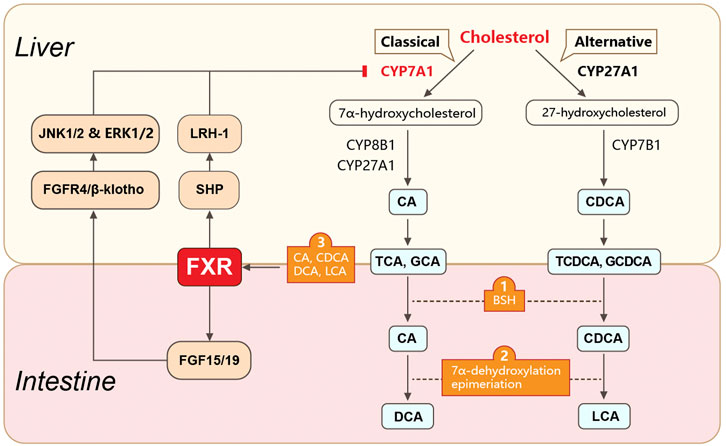
FIGURE 1. Gut microbiota-involved biosynthesis and metabolism of bile acids. Gut microbiota play roles in BA biosynthesis and metabolism. Primary BAs are synthesized in hepatocytes from cholesterol via classical or alternative pathways. They are further conjugated with taurine (in mice) or glycine (in humans) and transformed into conjugated BAs (e.g., TCA, GCA, TCDCA, and GCDCA). The conjugated primary BAs are secreted from the liver into the bile and subsequently into the intestinal lumen, where they are unconjugated by bacteria-produced BSH. The unconjugated primary BAs are biotransformed into secondary BAs (e.g., DCA, UDCA, and LCA) via 7α-dehydroxylation or epimerization. The gut microbiota also mediates the enterohepatic circulation of BAs via FXR. FXR is activated by unconjugated BAs (e.g., CDCA, DCA, LCA, and CA) and regulates BA synthesis via molecules in the liver and intestine. In the liver, FXR suppresses the expression of CYP7A1 (the rate-limiting enzyme for the classical pathway of BA synthesis) by activating SHP. SHP further binds to LRH1. In the intestine, FXR suppresses CYP7A1 expression by upregulating FGF15 (FGF19 in humans) expression. FGF15 further binds to the FGFR4/β-klotho complex and induces JNK1/2 and ERK1/2 signaling. BAs, bile acids; TCA, taurocholic acid; GCA, glycocholic acid; TCDCA, taurochenodeoxycholic acid; GCDCA, glycochenodeoxycholic acid; BSH, bile salt hydrolase; DCA, deoxycholic acid; UDCA, ursodeoxycholic acid; LCA, lithocholic acid; FXR, farnesoid X receptor; SHP, small heterodimer partner; LRH1, liver receptor homolog 1; FGF15, fibroblast growth factor 15; FGFR4, FGF receptor 4.
The mechanism of FXR-regulated intestinal carcinogenesis has not been clearly defined but may involve interactions with matrix metallopeptidase 7 (MMP7) (Peng et al., 2019) or innate immunity-related molecules (Vavassori et al., 2009). The mechanism of FXR in HCC initiation depends on the FGF15/19–FGFR4–β-klotho axis. Abnormal activation of this axis directly stimulates the epithelial–mesenchymal transition of HCC cells, resulting in invasion and metastasis in an HCC mouse model (He et al., 2015). In particular, increased hepatocellular FGF19 levels were positively correlated with cirrhosis in patients with HCC, whereas depletion of FGF15 inhibited hepatocellular proliferation via tumor suppressors such as Ndrg2 (Deuschle et al., 2012) and miR-122 (He et al., 2015).
Bacterial components
Inflammation also can be induced by bacterial components, especially lipopolysaccharide (LPS). LPS is a cell wall component of Gram-negative bacteria that induces inflammation through interactions with pattern recognition receptors (PRRs). PRRs are expressed on the surface of most innate immune response-related cells. Toll-like receptors 4 (TLR4) are PRRs that recognize LPS and activate NF-κB through myeloid differentiation factor 88 (MyD88)-dependent or MyD88 adaptor-like (MAL) pathways. A cross-region cohort study analyzing 526 metagenomic fecal samples from CRC patients and healthy controls identified several LPS-related signaling pathways (Dai et al., 2018). Seven dominant bacterial species may contribute to those pathways, including Bacteroides fragilis, F. nucleatum, Porphyromonas asaccharolytica, Parvimonas micra, Prevotella intermedia, Alistipes finegoldii, and Thermanaerovibrio acidaminovorans. Similarly, in a case–control study with 139 HCC patients and matched controls, levels of serum anti-LPS antibody and anti-flagellin Ig A and IgG were significantly higher in the HCC group (Fedirko et al., 2017). This tendency was positively correlated with an increased risk of HCC.
Dysbiosis alters the immune response
The immune system helps identify and destroy nascent tumor cells and plays an important role in cancer defense. Dysfunction of the innate or adaptive immune systems induced by the bacteria-derived metabolites and bacterial components promotes carcinogenesis in the intestine and liver.
Evidence of gut microbiota in regulating immune response in CRC or HCC
GF mice without bacteria showed immature immune systems with poor gut-associated lymphoid tissue, lower levels of tissue-resident macrophages, smaller spleens, and decreased serum immunoglobulin levels. This incomplete immune system can be corrected by introducing intestinal bacteria, bacterial metabolites (e.g., SCFAs), or bacterial components (e.g., polysaccharide A, PSA) (Allen-Vercoe et al., 2011; Malik et al., 2018). Gut microbiota depletion by antibiotics alleviated the tumor burden in mice with CRC. However, this protective effect was not observed in mice with immune gene knockouts (Tao et al., 2007), indicating it depends on an intact immune system. In addition, exposure to gut microbiota stimulated the expression of CRC-associated chemokine genes (e.g., CCL5, CXCL9, CXCL10, CXCL1, and CCL20) (Cremonesi et al., 2018). Increased levels of CRC infiltrating-related chemokines helped inhibit CRC development by recruiting tumor-infiltrating lymphocytes (TILs). Therefore, patients with specific chemokine-expressing bacteria show improved survival rates. Similarly, treatment with Akkermansia muciniphila or Amuc_1100 (an outer membrane protein produced by Akkermansia muciniphila) improved colitis in mice with CRC by reducing levels of infiltrating macrophages and CD8+ cytotoxic T lymphocytes in the colon (Wang et al., 2020b).
Bacterial metabolite-based intestinal immune system
The gut microbiota influences the normal functions of innate and adaptive immune response through its metabolites, including SCFAs, aryl hydrocarbon receptor (AhR) ligands, and polyamines.
SCFAs affect nearly every process of the intestinal immune response. Thus, they play important roles in maintaining the intestinal immune system (Figure 2). First, SCFAs serve as signaling molecules in the innate immune system by inhibiting HDACs (Rooks and Garrett, 2016). This inhibitory effect is NF-κB-dependent (Kendrick et al., 2010). HDAC suppression also facilitates the inhibitory activity of FOXP3+ regulatory T (Treg) cells (Tao et al., 2007). Signaling molecule GPCRs, including GPR43, GPR41, and GPR109A, are also involved in the SCFA-mediated immune response. Third, SCFAs help maintain intestinal barrier integrity. The commensal bacteria in the lumen (bacterial barrier), immunoglobin A (IgA) secreted by intestinal immune cells (immunological barrier), and the mucus layer and epithelial elements (physical barrier) help maintain intestinal integrity and reduce intestinal permeability. This protection wall efficiently prevents lumen colonization by pathogens and the translocation of bacteria or their products and components, such as LPS. Increased gut permeability or the so-called leaky gut is closely related to the onset of CRC and HCC. Exposure to LPS in the liver activates Kupffer cells via binding to TLR4, resulting in increased TNF-α, IL-6, and IL-8 levels. The increased production of proinflammatory cytokines and chemokines leads to HCC, as discussed previously and elsewhere (Yu et al., 2010; Dapito et al., 2012; Sanduzzi Zamparelli et al., 2017). Similarly, increased barrier function is helpful for CRC treatment (Bhutiani et al., 2018). Exposure to SCFAs increased the expression of mucin genes in epithelial goblet cells and strengthened the immunological barrier via mucosal immunity (Willemsen et al., 2003; Gaudier et al., 2004). SCFAs also modulate the permeability of tight junctions in intestinal epithelial cells, which is an important element in the physical barrier. A compact tight junction inhibits the translocation of enteropathogenic toxins. Colonization with SCFAs-producing bacteria relieved mice from infection by E. coli (Fukuda et al., 2011).
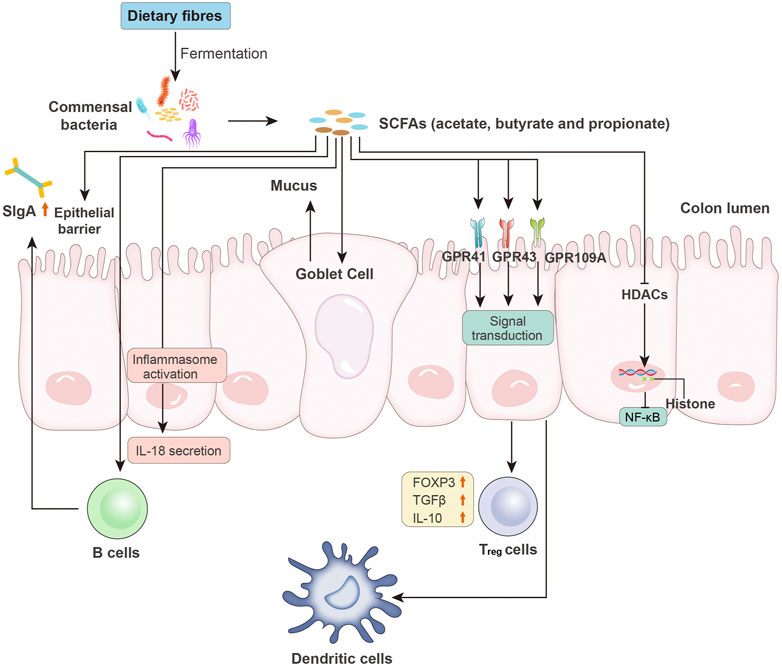
FIGURE 2. Microbiota-derived SCFAs and intestinal immunity. SCFAs, such as acetate, butyrate, and propionate, are produced by intestinal bacteria via the fermentation of undigested dietary fibers. SCFAs affect the host immune system through the following pathways: increased epithelial barrier function; increased B cell-based sIgA secretion; activation of inflammasomes and induced production of IL-18; enhanced goblet cell-based mucus production; binding to epithelial surface GPCRs (e.g., GPR41, GPR43, and GPR109A) to reduce expression of T-cell-activating molecules on antigen-presenting cells (e.g., dendritic cells) and increased levels of FOXP3 and anti-inflammatory cytokines (e.g., TGF-β and IL-10) in Treg cells; and inhibition of HDAC activity, followed by NF-κB inhibition. sIgA, secretory IgA; GPCRs, G protein-coupled receptors; FOXP3, forkhead box P3; Treg cells, regulatory T cells; HDACs, histone deacetylases.
Aryl hydrocarbon receptor (AHR) is expressed by immune cells, epithelial cells, and some tumor cells. It is a member of the periodic circadian protein (PER)-AHR nuclear translocator (ARNT)-single-minded protein (SIM) superfamily of transcription factors. AHR is activated by both endogenous (e.g., kynurenine, a tryptophan derivative) and exogenous factors (e.g., diindolylmethane and indolocarbazole derived from the diet) (McIntosh et al., 2010). These factors are known as AHR ligands. The binding of AHR to microbial metabolites, such as the indole derivatives of tryptophan (Cervantes-Barragan et al., 2017; Carambia and Schuran, 2021), plays an essential role in the regulation of innate and adaptive immune responses. Deficiency of AHR or AHR ligands in mice led to increased Bacteroides spp., decreased antimicrobial peptide (AMP) production, and reduction of intestinal intraepithelial lymphocytes (IELs) and turnover of intestinal epithelial cells (IECs) (Li et al., 2011). Injection of wild-type IELs to Ahr−/− mice with a maintained IEC barrier function and normalized bacterial composition. In wild-type mice, deficiency of AHR ligands resulted in increased severity of dextran sodium sulfate-induced colonic inflammation. The inflammation was improved when the mice were administered AHR ligand-containing diets. Together, these studies provided solid evidence for the AHR-mediated immune response. In general, indoleamine 2,3-dioxygenase 1–tryptophan and 2,3-dioxygenase 2–kynurenine–AHR (IDO1/TDO2–KYN–AHR) are important axes in AHR-involved immune regulation. IDO1 and TDO2 are two intracellular heme-containing metalloproteins that convert tryptophan into a series of biologically active molecules, such as KYN and several indole-containing substrates (e.g., neurotransmitter melatonin). The KYN pathway is the major route of tryptophan intake. KYN and its metabolic product kynurenic acid are endogenous AHR ligands (Gaitanis et al., 2008; Lamas et al., 2016). KYN-activated AHR is an important regulator of both innate and adaptive immune cells. Its activation can lead to the generation of immune-tolerant dendritic cells (DCs) and Treg cells, resulting in a host-friendly tumor microenvironment (TME). This action is essential for the eradication of cancer cells (Cervantes-Barragan and Colonna, 2018; Cheong and Sun, 2018).
Polyamines such as spermidine, spermine, and putrescine are polycationic molecules produced by almost all living cells, including bacteria. The human intestinal lumen contains high levels of polyamines, which are derived from the diet and metabolism by the host and bacteria. Gut bacteria can use amino acid decarboxylase to produce polyamines that differ from the mammalian versions (using the arginase 1 and ornithine decarboxylase). Polyamines can affect the virulence of bacterial pathogens and also strengthen the integrity of the IEC barrier. Polyamines can induce the production of intercellular junction proteins (Rao et al., 2020), including zonula occludens 1 (ZO1), occludin, and E-cadherin, which are vital for maintaining intestinal permeability and enhancing epithelial barrier function. Moreover, polyamine metabolism plays an important role in regulating both innate and adaptive immune responses. Spermine can suppress the classic (M1) macrophage activation by inhibiting the expression of ornithine decarboxylase and the synthesis of pro-inflammatory cytokines (Kaczmarek et al., 1992; Zhang et al., 2000). The administration of Bifidobacterium animalis subsp. lactis LKM512 with an arginine-containing diet led to decreased levels of colonic TNF and IL-6 (Kibe et al., 2014). Pups supplied with polyamine showed elevated maturation of lamina propria CD4+ T cells and intraepithelial CD8+ T cells, accompanied by an earlier appearance of splenic B cells (Pérez-Cano et al., 2010). Higher polyamine concentrations are generally associated with carcinogenesis (Casero et al., 2018). Patients with cancer have increased polyamine levels in their blood and urine compared to healthy individuals (Kassi and Kaltsas, 2022). The dysregulation of polyamine metabolism by the host or gut microbiota may contribute to CRC (Yang et al., 2019). Furthermore, polyamines can suppress anti-tumor immune responses. Polyamine depletion via inhibition of ornithine decarboxylase activity attenuated tumor growth in a T-cell-dependent manner (Hayes et al., 2014), supporting the hypothesis that reducing intra-tumor polyamines may reverse immunosuppression in the TME.
Bacterial components-based immune response
The gut microbiota also modulates the immune response through bacterial components, including formyl peptides, PSA, and peptidoglycan. Formyl peptides are conserved N-formyl peptide motifs secreted by bacteria including Staphylococcus aureus. High concentrations of formyl peptides activate formyl peptide receptor 2 (FPR2) (Bloes et al., 2015) and induce neutrophil diapedesis at infection sites. PSA is a well-known polysaccharide produced by ETBF. It works with TLRs on DCs and is presented to T cells by CD11c+ DCs. The anti-inflammation effect of PSA depends on IL-10-producing CD4+ T cells and IL-10-producing CD25+ FOXP3+ Treg cells (Round and Mazmanian, 2010). Peptidoglycan has a harmful effect on nucleotide-binding oligomerization domain-containing protein 1 (NOD1). NOD1 signaling is related to the innate immune system, which increases the number of ileal γδ T cells and stimulates the release of pro-inflammatory cytokine IL-17A (Hergott et al., 2016).
Dysbiosis-induced DNA damage via genotoxins
Gut microbiota-produced genotoxins also work as second hits that accelerate CRC and HCC carcinogenesis. Cytolethal distending toxin (CDT) and colibactin are two genotoxins that induce DNA double-strand breaks. CDT and CDTB (its active subunit) are mainly produced by Campylobacter jejuni. CDT can lead to genetic instability by inducing replicative stress in several human cells (e.g., HeLa, U2OS, and RKO) and human colorectal organoids (Tremblay et al., 2021). This replicative stress leads to chromosomic aberrations by slowing DNA replication and expressing fragile sites. In addition, mice treated with a CDTB mutant Campylobacter jejuni (C. jejuni) showed less DNA damage and a lower risk of CRC (He et al., 2019). Interestingly, this CDT-mediated carcinogenesis was autophagy-dependent (Seiwert et al., 2017), indicating that gut microbiota-produced genotoxins may interact with the cancer-related cellular stress response. Similarly, E. coli-produced colibactin induced CRC in both mice and humans. A recent study identified a DNA-damage signature in bacteria-infected human colorectal cells (Dziubańska-Kusibab et al., 2020), which suggested the etiological role of colibactin in CRC.
Dysbiosis induces cellular stress response associated with genetic, epigenetic, and/or neoplastic changes
Dysbiosis induces genetic and epigenetic alterations
Human intestinal organoids exposed to pks + E. coli show distinct mutational signatures, including increased numbers of single base substitutions (SBSs) and induced insertion–deletion (ID) (e.g., single T deletions at T homopolymers) (Cremonesi et al., 2018). These characteristic mutations have also been observed in human cancer genomes, especially in patients with CRC. Studies have proposed the underlying mechanisms for dysbiosis-involved carcinogenesis, i.e., the effects on gene mutation. A study on mutant p53 further discussed the role of gut microbiota on gene mutation (Kadosh et al., 2020). In general, somatic mutations in p53 inactivate its tumor-suppressor function and confer oncogenic gain-of-function. Model mice with mutant p53 showed contrasting effects in different intestinal segments. An oncogenic effect was observed in the distal gut (colon and ileum) through activated WNT signaling, whereas a tumor-suppressive effect was observed in the proximal gut and tumor organoids. The administration of an antibiotic cocktail reversed the oncogenic effect of mutant p53 in the distal part, with shorter crypts, better-organized villi, and decreased WNT signaling. These data suggested that microbiota in the distal part are crucial for the oncogenic effect of mutant p53. Gut microbiota help switch mutant p53 from tumor-suppressive to oncogenic. Gut microbiota also can change the function of long non-coding RNA (lncRNA). F. nucleatum, the predominant bacteria in patients with CRC, stimulates glucose metabolism in CRC cells by activating the transcription of lncRNA enolase 1-intronic transcript 1 (ENO1-IT1) (Hong et al., 2021). This increased transcription further regulates the activation of KAT7 histone acetyltransferase, consequently altering the CRC biological function.
The gut microbiota is also associated with epigenetic events such as DNA methylation (Sobhani et al., 2020). DNA methylation is a crucial process in carcinogenesis. Exposure to gut microbiota induces methylome and transcriptome changes in intestinal epithelial cells and is beneficial for mild host inflammation within the mucosa. Thus, dysbiotic microbiota drives aberrant epigenetic events, leading to CRC or HCC. DNMT1, DNMT3B, and EZH2 histone (H3K27) methyltransferases are upregulated in microsatellite instability tumors in CRC (Joensuu et al., 2015).
Dysbiosis modulates cancer-related cellular stress responses
Autophagy (Onorati et al., 2018; Li et al., 2020) and oxidative stress (Greenwood and Witney, 2021; Kuo et al., 2022) are cancer-related cellular stress responses. Autophagy refers to a series of mechanisms that transport superfluous or potentially dangerous cytoplasmic entities to the lysosome for degradation. The inhibition of autophagy sensitizes cells to regulated cell death. Evidence supporting the involvement of gut microbiota in autophagy is as follows: first, several bacteria-produced proteins are mediated by autophagy receptors such as SQSTM1/p62 and CALCOCO2/NDP52 (Sudhakar et al., 2019). Second, autophagy-mediated inhibition of type I interferon is gut microbiota-dependent (Martin et al., 2018). Third, the administration of gut microbiota-produced metabolites (butyrate) increases the expression of LC3 (a marker for autophagy) and the formation of autolysosomes in human colorectal cells (Luo et al., 2019). Recent studies showed that exposure to pathogen-associated molecular patterns (PAMPs) in dysbiosis activated hepatic NOD2, a general intracellular PRR, via bacterial muramyl dipeptide (MDP). The NOD2 activation was receptor-interacting protein 2 (RIP2)-dependent and upregulated NF-κB, JAK2/STAT3, and MAPK signaling in hepatocytes to promote a carcinogenesis-related pro-inflammatory response in the liver. Moreover, RIP2-dependent NOD2 activation induced a novel nuclear autophagy pathway. After transport to the nucleus, NOD2 binds to lamin A/C, a component of nuclear laminae, resulting in its protein degradation, ultimately leading to impaired DNA damage repair and increased genomic instability (Zhou et al., 2021). Oxidative stress is another gut microbiota-associated cellular stress response. Microbiota-produced metabolites such as butyrate can induce the production of reactive oxygen species (ROS) in HCC cells (Pant et al., 2017a; Pant et al., 2017b). The gut microbiota also produces endogenous ethanol, which is metabolized into acetaldehyde and acetate. Ethanol and its derivates induce ROS formation (Zhu et al., 2018; Sha’fie et al., 2020) by hepatic stellate cells (HSCs) and Kupffer cells, resulting in increased TLR4 expression (Hubbard et al., 2015). The resulting production of inflammatory cytokines leads to liver injury, including HCC.
Gut microbiota-based therapy in CRC and HCC
As discussed earlier, dysbiosis is the first hit for the digestive system. Therefore, reshaping the composition of commensal bacteria is an important method for cancer prevention and anti-tumor therapy in the digestive system, especially in CRC and HCC.
The role of gut microbiota in the treatment of CRC and HCC
Most data on gut microbiota-based therapy has been reported in CRC, whereas data in HCC are limited. The gut microbiota was associated with the recruitment of T follicular helper cells (TFH) in both mouse models and patients with CRC (Roberti et al., 2020). TFH cells are subtypes of tumor-infiltrating lymphocyte (TIL), the presence of which indicates a better prognosis. Increased family Fusobacteriaceae and decreased family Erysipelotrichaceae have been reported in patients with CRC. Increased Fusobacteriaceae harms TIL recruitment, thereby promoting colonic tumorigenesis. In contrast, some bacteria show beneficial effects on cancer therapy. In CRC-related immunotherapy, patients with efficient responses showed increased Bifidobacterium (Sivan et al., 2015; Matson et al., 2018) and Faecalibacterium (Gopalakrishnan et al., 2018). In addition, a lower risk of relapse is associated with decreased F. nucleatum in patients receiving post-neoadjuvant chemoradiotherapy (nCRT) (Serna et al., 2020). Similarly, mice gavage with Bifidobacterium showed an efficient response to anti-CD47-based immunotherapy (Shi et al., 2020). Ileac residence of Bacteroides fragilis and Erysipelotrichaceae was associated with an immunosurveillance-based protective effect in a mouse model of CRC (Roberti et al., 2020).
Gut microbiota-based therapeutic approaches in CRC and HCC
The first approach involves the supplementation of indigestible fermentable dietary fibers (i.e., prebiotics) or living microorganisms (i.e., probiotics) to increase beneficial intestinal bacteria. Some studies mixed prebiotics and probiotics to form synbiotics. Oligosaccharides such as fructooligosaccharides (FOS) and galactooligosaccharides (GOS) are the most common materials used as prebiotics. The commonly used probiotics include Bifidobacteria, Lactobacilli, Streptococcus, and VSL#3. VSL#3 is a mixture of probiotics, which can increase beneficial bacteria such as Lachnospiraceae, Faecalibacterium, and Ruminococcus (Jena et al., 2020).
Fecal microbiota transplantation (FMT) is the second approach. It directly reverses the overall bacterial composition and is a clinic-favored strategy for dysbiosis-dependent cancer therapy. The potential effect of FMT on CRC and HCC is still under investigation, although it is a useful treatment for recurrent Clostridium difficile infection (CDI) (Hvas et al., 2019; Khoruts et al., 2021).
Regarding bacteria-produced metabolites, the administration of postbiotics (e.g., SCFAs) has been accepted as a third approach for indirectly managing gut microbiota-involved carcinogenesis. A recent consensus statement defined postbiotics as “the preparation of inanimate microorganisms and/or their components that confers a health benefit on the host” (Salminen et al., 2021). Different materials and components can be used as postbiotics, including cell-free supernatants from bacterial culture medium, bacterial exopolysaccharides, enzymes or cell wall fragments, bacterial lysates, and microbiota-produced metabolites (Żółkiewicz et al., 2020).
Similarly, paraprobiotics (or ghost probiotics) have also been introduced to amend dysbiotic microflora. Paraprobiotics refers to viable microbial cells or crude cell extracts that confer a benefit to the receiver (either human or animal) when orally or topically administrated (Taverniti and Guglielmetti, 2011). Several methods can be utilized to prepare inactive probiotics, including heat, gamma or ultraviolet rays, chemicals (e.g., formalin), and sonication.
In addition, other microbiota-targeted manipulation, such as supplementation of TLR antagonists (e.g., polymyxin B), FXR agonists (e.g., obeticholic acid), or prokinetics (e.g., cisapride), also serve as accessary approaches for HCC. The use of antibiotics is controversial. Antibiotics aim to suppress the overall growth of bacteria in the intestine and have demonstrated protective effects on tumor cell proliferation and invasion (Bullman et al., 2017; Han et al., 2020). However, antibiotics can cause further dysbiosis and are associated with CRC onset (Zhang et al., 2019).
Two recently updated reviews compared the advantages and disadvantages of each approach (Kaźmierczak-Siedlecka et al., 2020; Pothuraju et al., 2021). Some unsolved must be addressed for better clinical application. First, the use of probiotics may cause bacterial translocation and the transfer of resistant genes through horizontal gene transfer, especially in patients with underlying medical conditions. Similarly, FMT may transfer unrecognized pathogens from the donor to the recipient. However, aside from the safety issue, the potential benefits of FMT as a therapeutic strategy are much higher than those of probiotics. FMT improves the overall intestinal microbial diversity, whereas probiotics have limited bacterial input. The third inconclusive issue is postbiotics, since isolating metabolites synthesized by bacteria remains challenging. However, recently developed technology and analysis techniques, such as air-flow-assisted desorption electrospray ionization mass spectrometry imaging analysis (AFAI-MSI) (Chen et al., 2021), may help solve these problems.
Conclusion and perspectives
In closing, evidence from preclinical models and clinical data support the “two-hit hypothesis” for dysbiosis and its associated alterations in the development of digestive system cancer. Dysbiosis alone is not powerful enough to potentiate carcinogenesis until the development of dysbiosis-induced alterations, including inflammation, aberrant immune response, gut microbiota-produced genotoxins, and cellular stress response associated with genetic, epigenetic, and/or neoplastic changes. These four mechanisms serve as second hits to speed up carcinogenesis in gastrointestinal cancer, especially CRC and HCC. Finally, three application-related questions remain to be addressed:
• Inter-individual differences in gut microbiota may complicate the differentiation of cancer patients and healthy controls. Therefore, is it possible to screen gut microbiota from healthy individuals and develop a list of “beneficial bacteria”? Moreover, can we build continent- or race-based lists of beneficial bacteria to distinguish healthy individuals from potential patients?
• Since each type of digestive system cancer harbors a unique gut microbiota, can we screen them to build a cancer type-based bacteria list? This category may help identify the “gut microbiota-based susceptible factors” in digestive system cancer and may be a novel measurement for cancer prevention.
• Dietary soluble fibers are fermented by gut bacteria into SCFAs, which have diverse health-promoting effects. Increasing numbers of studies have reported the beneficial role of SCFAs in cancer prevention and anti-tumor therapy. If possible, nutrition-based strategies should be incorporated into standard care for cancer therapy, as dietary interventions are both cost-effective and patient-friendly compared to other pharmacological interventions. In the future, standard criteria or guidelines for nutrient selection are needed and may improve treatment outcomes and tolerance of cancer therapy.
Author contributions
All authors listed have made a substantial, direct, and intellectual contribution to the work and approved it for publication.
Funding
This research was funded by the National Natural Science Foundation of China (Nos U20A20408 and 82074450), the Natural Science Foundation of Hunan Province (No. 2020JJ4066), the Education Department of Hunan Province (No. 21B0374), the Hunan Administration of Traditional Chinese Medicine (D2022070), Project in Hunan university of Chinese Medicine (21YS003), and the Integrated Traditional Chinese and Western Medicine Open Fund Project (Nos 2020ZXYJH20 and 2020ZXYJH35).
Conflict of interest
The authors declare that the research was conducted in the absence of any commercial or financial relationships that could be construed as a potential conflict of interest.
Publisher’s note
All claims expressed in this article are solely those of the authors and do not necessarily represent those of their affiliated organizations, or those of the publisher, the editors, and the reviewers. Any product that may be evaluated in this article, or claim that may be made by its manufacturer, is not guaranteed or endorsed by the publisher.
References
Allen-Vercoe E., Strauss J., Chadee K. (2011). Fusobacterium nucleatum: An emerging gut pathogen? Gut microbes 2 (5), 294–298. doi:10.4161/gmic.2.5.18603
Amieva M., Peek R. M. (2016). Pathobiology of Helicobacter pylori-induced gastric cancer. Gastroenterology 150 (1), 64–78. doi:10.1053/j.gastro.2015.09.004
Arthur J. C., Perez-Chanona E., Muhlbauer M., Tomkovich S., Uronis J. M., Fan T. J., et al. (2012). Intestinal inflammation targets cancer-inducing activity of the microbiota. Sci. (New York, N.Y.) 338 (6103), 120–123. doi:10.1126/science.1224820
Bajaj J. S., Betrapally N. S., Hylemon P. B., Heuman D. M., Daita K., White M. B., et al. (2015). Salivary microbiota reflects changes in gut microbiota in cirrhosis with hepatic encephalopathy. Hepatol. Baltim. Md.) 62 (4), 1260–1271. doi:10.1002/hep.27819
Behary J., Amorim N., Jiang X. T., Raposo A., Gong L., McGovern E., et al. (2021). Gut microbiota impact on the peripheral immune response in non-alcoholic fatty liver disease related hepatocellular carcinoma. Nat. Commun. 12 (1), 187. doi:10.1038/s41467-020-20422-7
Bell H. N., Rebernick R. J., Goyert J., Singhal R., Kuljanin M., Kerk S. A., et al. (2022). Reuterin in the healthy gut microbiome suppresses colorectal cancer growth through altering redox balance. Cancer Cell 40 (2), 185–200.e6. doi:10.1016/j.ccell.2021.12.001
Bhutiani N., Li Q., Anderson C. D., Gallagher H. C., De Jesus M., Singh R., et al. (2018). Enhanced gut barrier integrity sensitizes colon cancer to immune therapy. Oncoimmunology 7 (11), e1498438. doi:10.1080/2162402X.2018.1498438
Bloes D. A., Kretschmer D., Peschel A. (2015). Enemy attraction: Bacterial agonists for leukocyte chemotaxis receptors. Nat. Rev. Microbiol. 13 (2), 95–104. doi:10.1038/nrmicro3390
Borrelli A., Bonelli P., Tuccillo F. M., Goldfine I. D., Evans J. L., Buonaguro F. M., et al. (2018). Role of gut microbiota and oxidative stress in the progression of non-alcoholic fatty liver disease to hepatocarcinoma: Current and innovative therapeutic approaches. Redox Biol. 15, 467–479. doi:10.1016/j.redox.2018.01.009
Buda A., Jepson M. A., Martines D., Paraskeva C., PignatelliM. (2003). Butyrate downregulates alpha2beta1 integrin: A possible role in the induction of apoptosis in colorectal cancer cell lines. Gut 52 (5), 729–734. doi:10.1136/gut.52.5.729
Bullman S., Pedamallu C. S., Sicinska E., Clancy T. E., Zhang X., Cai D., et al. (2017). Analysis of Fusobacterium persistence and antibiotic response in colorectal cancer. Sci. (New York, N.Y.) 358 (6369), 1443–1448. doi:10.1126/science.aal5240
Carambia A., Schuran F. A. (2021). The aryl hydrocarbon receptor in liver inflammation. Semin. Immunopathol. 43 (4), 563–575. doi:10.1007/s00281-021-00867-8
Casero R. A., Murray Stewart T., Pegg A. E. (2018). Polyamine metabolism and cancer: Treatments, challenges and opportunities. Nat. Rev. Cancer 18 (11), 681–695. doi:10.1038/s41568-018-0050-3
Castellarin M., Warren R. L., Freeman J. D., Dreolini L., Krzywinski M., Strauss J., et al. (2012). Fusobacterium nucleatum infection is prevalent in human colorectal carcinoma. Genome Res. 22 (2), 299–306. doi:10.1101/gr.126516.111
Cervantes-Barragan L., Chai J. N., Tianero M. D., Di Luccia B., Ahern P. P., Merriman J., et al. (2017). Lactobacillus reuteri induces gut intraepithelial CD4+CD8αα+ T cells. Sci. (New York, N.Y.) 357 (6353), 806–810. doi:10.1126/science.aah5825
Cervantes-Barragan L., Colonna M. (2018). AHR signaling in the development and function of intestinal immune cells and beyond. Semin. Immunopathol. 40 (4), 371–377. doi:10.1007/s00281-018-0694-9
Chang C.-W., Lee H. C., Li L. H., Chiang Chiau J. S., Wang T. E., Chuang W. H., et al. (2020). Fecal microbiota transplantation prevents intestinal injury, upregulation of toll-like receptors, and 5-fluorouracil/oxaliplatin-induced toxicity in colorectal cancer. Int. J. Mol. Sci. 21 (2), E386. doi:10.3390/ijms21020386
Chang P. V., Hao L., Offermanns S., Medzhitov R. (2014). The microbial metabolite butyrate regulates intestinal macrophage function via histone deacetylase inhibition. Proc. Natl. Acad. Sci. U. S. A. 111 (6), 2247–2252. doi:10.1073/pnas.1322269111
Chattopadhyay I., Nandi D., Nag A. (2021). The pint- sized powerhouse: Illuminating the mighty role of the gut microbiome in improving the outcome of anti- cancer therapy. Semin. Cancer Biol. 70, 98–111. doi:10.1016/j.semcancer.2020.07.012
Chen F., Dai X., Zhou C. C., Li K. X., Zhang Y. J., Lou X. Y., et al. (2021). Integrated analysis of the faecal metagenome and serum metabolome reveals the role of gut microbiome-associated metabolites in the detection of colorectal cancer and adenoma. Gut 71, 1315–1325. doi:10.1136/gutjnl-2020-323476
Chen J., Hu S., Ji D., Gao Z., Wang H., Yang Y., et al. (2020). Hemolysin BL from novel Bacillus toyonensis BV-17 induces antitumor activity both in vitro and in vivo. Gut Microbes 12 (1), 1782158. doi:10.1080/19490976.2020.1782158
Chen S., Zhang L., Li M., Zhang Y., Sun M., Wang L., et al. (2022). Fusobacterium nucleatum reduces METTL3-mediated m6A modification and contributes to colorectal cancer metastasis. Nat. Commun. 13 (1), 1248. doi:10.1038/s41467-022-28913-5
Chen Y., Ji F., Guo J., Shi D., Fang D. (2016). Dysbiosis of small intestinal microbiota in liver cirrhosis and its association with etiology. Sci. Rep. 6, 34055. doi:10.1038/srep34055
Cheong J. E., Sun L. (2018). Targeting the Ido1/TDO2-KYN-AhR pathway for cancer immunotherapy - challenges and opportunities. Trends Pharmacol. Sci. 39 (3), 307–325. doi:10.1016/j.tips.2017.11.007
Choi S.-C., Brown J., Gong M., Ge Y., Zadeh M., Li W., et al. (2020). Gut microbiota dysbiosis and altered tryptophan catabolism contribute to autoimmunity in lupus-susceptible mice. Sci. Transl. Med. 12 (551), eaax2220. doi:10.1126/scitranslmed.aax2220
Clifford G. M., Tully S., Franceschi S. (2017). Carcinogenicity of human papillomavirus (HPV) types in HIV-positive women: A meta-analysis from HPV infection to cervical cancer. Clin. Infect. Dis. 64 (9), 1228–1235. doi:10.1093/cid/cix135
Coelho O. G. L., Cândido F. G., Alfenas R. d. C. G. (2019). Dietary fat and gut microbiota: Mechanisms involved in obesity control. Crit. Rev. Food Sci. Nutr. 59 (19), 3045–3053. doi:10.1080/10408398.2018.1481821
Coker O. O., Liu C., Wu W. K. K., Wong S. H., Jia W., Sung J. J. Y., et al. (2022). Altered gut metabolites and microbiota interactions are implicated in colorectal carcinogenesis and can be non-invasive diagnostic biomarkers. Microbiome 10 (1), 35. doi:10.1186/s40168-021-01208-5
Cremonesi E., Governa V., Garzon J. F. G., Mele V., Amicarella F., Muraro M. G., et al. (2018). Gut microbiota modulate T cell trafficking into human colorectal cancer. Gut 67 (11), 1984–1994. doi:10.1136/gutjnl-2016-313498
Cuevas-Ramos G., Petit C. R., Marcq I., Boury M., Oswald E., Nougayrede J. P. (2010). Escherichia coli induces DNA damage in vivo and triggers genomic instability in mammalian cells. Proc. Natl. Acad. Sci. U. S. A. 107 (25), 11537–11542. doi:10.1073/pnas.1001261107
Dai Z., Coker O. O., Nakatsu G., Wu W. K. K., Zhao L., Chen Z., et al. (2018). Multi-cohort analysis of colorectal cancer metagenome identified altered bacteria across populations and universal bacterial markers. Microbiome 6 (1), 70. doi:10.1186/s40168-018-0451-2
Dapito D. H., Mencin A., Gwak G. Y., Pradere J. P., Jang M. K., Mederacke I., et al. (2012). Promotion of hepatocellular carcinoma by the intestinal microbiota and TLR4. Cancer Cell 21 (4), 504–516. doi:10.1016/j.ccr.2012.02.007
Dejea C. M., Fathi P., Craig J. M., Boleij A., Taddese R., Geis A. L., et al. (2018). Patients with familial adenomatous polyposis harbor colonic biofilms containing tumorigenic bacteria. Sci. (New York, N.Y.) 359 (6375), 592–597. doi:10.1126/science.aah3648
Deuschle U., Schuler J., Schulz A., Schluter T., Kinzel O., Abel U., et al. (2012). FXR controls the tumor suppressor NDRG2 and FXR agonists reduce liver tumor growth and metastasis in an orthotopic mouse xenograft model. PloS One 7 (10), e43044. doi:10.1371/journal.pone.0043044
Dubinkina V. B., Tyakht A. V., Odintsova V. Y., Yarygin K. S., Kovarsky B. A., Pavlenko A. V., et al. (2017). Links of gut microbiota composition with alcohol dependence syndrome and alcoholic liver disease. Microbiome 5 (1), 141. doi:10.1186/s40168-017-0359-2
Dziubańska-Kusibab P. J., Berger H., Battistini F., Bouwman B. A. M., Iftekhar A., Katainen R., et al. (2020). Colibactin DNA-damage signature indicates mutational impact in colorectal cancer. Nat. Med. 26 (7), 1063–1069. doi:10.1038/s41591-020-0908-2
Fan L., Xu C., Ge Q., Lin Y., Wong C. C., Qi Y., et al. (2021). A. Muciniphila suppresses colorectal tumorigenesis by inducing TLR2/NLRP3-mediated M1-like TAMs. Cancer Immunol. Res 9 (10), 1111–1124. doi:10.1158/2326-6066.CIR-20-1019
Fedirko V., Tran H. Q., Gewirtz A. T., Stepien M., Trichopoulou A., Aleksandrova K., et al. (2017). Exposure to bacterial products lipopolysaccharide and flagellin and hepatocellular carcinoma: A nested case-control study. BMC Med. 15 (1), 72. doi:10.1186/s12916-017-0830-8
Frost F., Kacprowski T., Ruhlemann M., Pietzner M., Bang C., Franke A., et al. (2021). Long-term instability of the intestinal microbiome is associated with metabolic liver disease, low microbiota diversity, diabetes mellitus and impaired exocrine pancreatic function. Gut 70 (3), 522–530. doi:10.1136/gutjnl-2020-322753
Fukuda S., Toh H., Hase K., Oshima K., Nakanishi Y., Yoshimura K., et al. (2011). Bifidobacteria can protect from enteropathogenic infection through production of acetate. Nature 469 (7331), 543–547. doi:10.1038/nature09646
Funabashi M., Grove T. L., Wang M., Varma Y., McFadden M. E., Brown L. C., et al. (2020). A metabolic pathway for bile acid dehydroxylation by the gut microbiome. Nature 582 (7813), 566–570. doi:10.1038/s41586-020-2396-4
Gaitanis G., Magiatis P., Stathopoulou K., Bassukas I. D., Alexopoulos E. C., Velegraki A., et al. (2008). AhR ligands, malassezin, and indolo[3, 2-b]carbazole are selectively produced by Malassezia furfur strains isolated from seborrheic dermatitis. J. Invest. Dermatol. 128 (7), 1620–1625. doi:10.1038/sj.jid.5701252
Gaudier E., Jarry A., Blottiere H. M., de CoPPet P., Buisine M. P., Aubert J. P., et al. (2004). Butyrate specifically modulates MUC gene expression in intestinal epithelial goblet cells deprived of glucose. Am. J. Physiol. Gastrointest. Liver Physiol. 287 (6), G1168–G1174. doi:10.1152/ajpgi.00219.2004
Gopalakrishnan V., Spencer C. N., Nezi L., Reuben A., Andrews M. C., Karpinets T. V., et al. (2018)., 359. New York, N.Y.), 97–103. doi:10.1126/science.aan4236Gut microbiome modulates response to anti-PD-1 immunotherapy in melanoma patientsScience6371
Greenwood H. E., Witney T. H. (2021). Latest advances in imaging oxidative stress in cancer. J. Nucl. Med. 62 (11), 1506–1510. doi:10.2967/jnumed.120.256974
Guertin K. A., Li X. S., Graubard B. I., Albanes D., Weinstein S. J., Goedert J. J., et al. (2017). Serum trimethylamine N-oxide, carnitine, choline, and betaine in relation to colorectal cancer risk in the alpha tocopherol, beta carotene cancer prevention study. Cancer epidemiology, biomarkers & prevention : A publication of the American association for cancer research. Cancer Epidemiol. Biomarkers Prev. 26 (6), 945–952. doi:10.1158/1055-9965.EPI-16-0948
Han J., Zhang S., Xu Y., Pang Y., Zhang X., Hu Y., et al. (2020). Beneficial effect of antibiotics and microbial metabolites on expanded Vδ2Vγ9 T cells in hepatocellular carcinoma immunotherapy. Front. Immunol. 11, 1380. doi:10.3389/fimmu.2020.01380
Hatakeyama M. (2014). Helicobacter pylori CagA and gastric cancer: A paradigm for hit-and-run carcinogenesis. Cell Host Microbe 15 (3), 306–316. doi:10.1016/j.chom.2014.02.008
Hayes C. S., Shicora A. C., Keough M. P., Snook A. E., Burns M. R., Gilmour S. K. (2014). Polyamine-blocking therapy reverses immunosuppression in the tumor microenvironment. Cancer Immunol. Res. 2 (3), 274–285. doi:10.1158/2326-6066.CIR-13-0120-T
He J., Zhao K., Zheng L., Xu Z., Gong W., Chen S., et al. (2015). Upregulation of microRNA-122 by farnesoid X receptor suppresses the growth of hepatocellular carcinoma cells. Mol. Cancer 14, 163. doi:10.1186/s12943-015-0427-9
He Z., Gharaibeh R. Z., Newsome R. C., Pope J. L., Dougherty M. W., Tomkovich S., et al. (2019). Campylobacter jejuni promotes colorectal tumorigenesis through the action of cytolethal distending toxin. Gut 68 (2), 289–300. doi:10.1136/gutjnl-2018-317200
Hergott C. B., Roche A. M., Tamashiro E., Clarke T. B., Bailey A. G., Laughlin A., et al. (2016). Peptidoglycan from the gut microbiota governs the lifespan of circulating phagocytes at homeostasis. Blood 127 (20), 2460–2471. doi:10.1182/blood-2015-10-675173
Hong J., Guo F., Lu S. Y., Shen C., Ma D., Zhang X., et al. (2021). F. nucleatum targets lncRNA ENO1-IT1 to promote glycolysis and oncogenesis in colorectal cancer. Gut 70 (11), 2123–2137. doi:10.1136/gutjnl-2020-322780
Huang H., Ren Z., Gao X., Hu X., Zhou Y., Jiang J., et al. (2020). Integrated analysis of microbiome and host transcriptome reveals correlations between gut microbiota and clinical outcomes in HBV-related hepatocellular carcinoma. Genome Med. 12 (1), 102. doi:10.1186/s13073-020-00796-5
Hubbard T. D., Murray I. A., Bisson W. H., Lahoti T. S., Gowda K., Amin S. G., et al. (2015). Adaptation of the human aryl hydrocarbon receptor to sense microbiota-derived indoles. Sci. Rep. 5, 12689. doi:10.1038/srep12689
Hvas C. L., Dahl Jorgensen S. M., Jorgensen S. P., Storgaard M., Lemming L., Hansen M. M., et al. (2019). Fecal microbiota transplantation is superior to fidaxomicin for treatment of recurrent Clostridium difficile infection. Gastroenterology 156 (5), 1324–1332. doi:10.1053/j.gastro.2018.12.019
Irrazabal T., Thakur B. K., Kang M., Malaise Y., Streutker C., Wong E. O. Y., et al. (2020). Limiting oxidative DNA damage reduces microbe-induced colitis-associated colorectal cancer. Nat. Commun. 11 (1), 1802. doi:10.1038/s41467-020-15549-6
Jena P. K., Sheng L., Li Y., Wan Y. J. Y. (2020). Probiotics VSL#3 are effective in reversing non-alcoholic steatohepatitis in a mouse model. Hepatobiliary Surg. Nutr. 9 (2), 170–182. doi:10.21037/hbsn.2019.09.07
Jia W., Rajani C., Xu H., Zheng X. (2021). Gut microbiota alterations are distinct for primary colorectal cancer and hepatocellular carcinoma. Protein Cell 12 (5), 374–393. doi:10.1007/s13238-020-00748-0
Jia W., Xie G., Jia W. (2018). Bile acid-microbiota crosstalk in gastrointestinal inflammation and carcinogenesis. Nat. Rev. Gastroenterol. Hepatol. 15 (2), 111–128. doi:10.1038/nrgastro.2017.119
Joensuu E. I., Nieminen T. T., Lotsari J. E., Pavicic W., Abdel-Rahman W. M., Peltomaki P. (2015). Methyltransferase expression and tumor suppressor gene methylation in sporadic and familial colorectal cancer. Genes Chromosom. Cancer 54 (12), 776–787. doi:10.1002/gcc.22289
Johnson S., Lavergne V., Skinner A. M., Gonzales-Luna A. J., Garey K. W., Kelly C. P., et al. (2021). Clinical practice guideline by the infectious diseases society of America (IDSA) and society for healthcare epidemiology of America (SHEA): 2021 focused update guidelines on management of Clostridioides difficile infection in adults. Clin. Infect. Dis. 73 (5), e1029–e1044. doi:10.1093/cid/ciab549
Kaczmarek L., Kaminska B., Messina L., Spampinato G., ArcidiAcono A., MaLaguarnera L., et al. (1992). Inhibitors of polyamine biosynthesis block tumor necrosis factor-induced activation of macrophages. Cancer Res. 52 (7), 1891–1894.
Kadosh E., Snir-Alkalay I., Venkatachalam A., May S., Lasry A., Elyada E., et al. (2020). The gut microbiome switches mutant p53 from tumour-suppressive to oncogenic. Nature 586 (7827), 133–138. doi:10.1038/s41586-020-2541-0
Kartal E., Schmidt T. S. B., Molina-Montes E., Rodriguez-Perales S., Wirbel J., Maistrenko O. M., et al. (2022). A faecal microbiota signature with high specificity for pancreatic cancer. Gut 71 (7), 1359–1372. doi:10.1136/gutjnl-2021-324755
Kassi E., Kaltsas G. (2022). Plasma polyamines as an additional to imaging biomarker in MEN1 patients with duodenopancreatic neuroendocrine tumors. J. Clin. Endocrinol. Metab. 107 (2), e880–e882. doi:10.1210/clinem/dgab683
Kaźmierczak-Siedlecka K., Daca A., Fic M., van de Wetering T., Folwarski M., Makarewicz W. (2020). Therapeutic methods of gut microbiota modification in colorectal cancer management - fecal microbiota transplantation, prebiotics, probiotics, and synbiotics. Gut microbes 11 (6), 1518–1530. doi:10.1080/19490976.2020.1764309
Kendrick S. F. W., O'Boyle G., Mann J., Zeybel M., Palmer J., Jones D. E. J., et al. (2010). Acetate, the key modulator of inflammatory responses in acute alcoholic hepatitis. Hepatol. Baltim. Md.) 51 (6), 1988–1997. doi:10.1002/hep.23572
Khoruts A., Staley C., Sadowsky M. J. (2021). Faecal microbiota transplantation for Clostridioides difficile: Mechanisms and pharmacology. Nat. Rev. Gastroenterol. Hepatol. 18 (1), 67–80. doi:10.1038/s41575-020-0350-4
Kibe R., Kurihara S., Sakai Y., Suzuki H., Ooga T., Sawaki E., et al. (2014). Upregulation of colonic luminal polyamines produced by intestinal microbiota delays senescence in mice. Sci. Rep. 4, 4548. doi:10.1038/srep04548
Kim J. M., Oh Y. K., Kim Y. J., Oh H. B., Cho Y. J. (2001). Polarized secretion of CXC chemokines by human intestinal epithelial cells in response to Bacteroides fragilis enterotoxin: NF-Kappa B plays a major role in the regulation of IL-8 expression. Clin. Exp. Immunol. 123 (3), 421–427. doi:10.1046/j.1365-2249.2001.01462.x
Kong C., Yan X., Zhu Y., Zhu H., Luo Y., Liu P., et al. (2021). Fusobacterium nucleatum promotes the development of colorectal cancer by activating a cytochrome P450/epoxyoctadecenoic acid Axis via TLR4/keap1/NRF2 signaling. Cancer Res. 81 (17), 4485–4498. doi:10.1158/0008-5472.CAN-21-0453
Kostic A. D., Gevers D., Pedamallu C. S., Michaud M., Duke F., Earl A. M., et al. (2012). Genomic analysis identifies association of Fusobacterium with colorectal carcinoma. Genome Res. 22 (2), 292–298. doi:10.1101/gr.126573.111
Kühn T., Stepien M., Lopez-Nogueroles M., Damms-Machado A., Sookthai D., Johnson T., et al. (2020). Prediagnostic plasma bile acid levels and colon cancer risk: A prospective study. J. Natl. Cancer Inst. 112 (5), 516–524. doi:10.1093/jnci/djz166
Kuo C.-L., Ponneri Babuharisankar A., Lin Y. C., Lien H. W., Lo Y. K., Chou H. Y., et al. (2022). Mitochondrial oxidative stress in the tumor microenvironment and cancer immunoescape: Foe or friend? J. Biomed. Sci. 29 (1), 74. doi:10.1186/s12929-022-00859-2
Lamas B., Richard M. L., Leducq V., Pham H. P., Michel M. L., Da Costa G., et al. (2016). CARD9 impacts colitis by altering gut microbiota metabolism of tryptophan into aryl hydrocarbon receptor ligands. Nat. Med. 22 (6), 598–605. doi:10.1038/nm.4102
Lang S., Schnabl B. (2020). Microbiota and fatty liver disease-the known, the unknown, and the future. Cell Host Microbe 28 (2), 233–244. doi:10.1016/j.chom.2020.07.007
Lapidot Y., Amir A., Nosenko R., Uzan-Yulzari A., Veitsman E., Cohen-Ezra O., et al. (2020). Alterations in the gut microbiome in the progression of cirrhosis to hepatocellular carcinoma. mSystems 5 (3), 001533-e220. doi:10.1128/mSystems.00153-20
Li L., Li X., Zhong W., Yang M., Xu M., Sun Y., et al. (2019). Gut microbiota from colorectal cancer patients enhances the progression of intestinal adenoma in Apcmin/+ mice. EBioMedicine 48, 301–315. doi:10.1016/j.ebiom.2019.09.021
Li Q., Hu W., Liu W. X., Zhao L. Y., Huang D., Liu X. D., et al. (2021). Streptococcus thermophilus inhibits colorectal tumorigenesis through secreting β-galactosidase. Gastroenterology 160 (4), 1179–1193.e14. doi:10.1053/j.gastro.2020.09.003
Li X., He S., Ma B. (2020). Autophagy and autophagy-related proteins in cancer. Mol. Cancer 19 (1), 12. doi:10.1186/s12943-020-1138-4
Li Y., Innocentin S., Withers D. R., Roberts N. A., Gallagher A. R., Grigorieva E. F., et al. (2011). Exogenous stimuli maintain intraepithelial lymphocytes via aryl hydrocarbon receptor activation. Cell 147 (3), 629–640. doi:10.1016/j.cell.2011.09.025
Lindell A. E., Zimmermann-Kogadeeva M., Patil K. R. (2022). Multimodal interactions of drugs, natural compounds and pollutants with the gut microbiota. Nat. Rev. Microbiol. 20 (7), 431–443. doi:10.1038/s41579-022-00681-5
Liu L., Dong W., Wang S., Zhang Y., Liu T., Xie R., et al. (2018). Deoxycholic acid disrupts the intestinal mucosal barrier and promotes intestinal tumorigenesis. Food Funct. 9 (11), 5588–5597. doi:10.1039/c8fo01143e
Liu Q., Li F., Zhuang Y., Xu J., Wang J., Mao X., et al. (2019). Alteration in gut microbiota associated with Hepatitis B and non-hepatitis virus related hepatocellular carcinoma. Gut Pathog. 11, 1. doi:10.1186/s13099-018-0281-6
Lloyd-Price J., Abu-Ali G., Huttenhower C. (2016). The healthy human microbiome. Genome Med. 8 (1), 51. doi:10.1186/s13073-016-0307-y
Luo S., Li Z., Mao L., Chen S., Sun S. (2019). Sodium butyrate induces autophagy in colorectal cancer cells through LKB1/AMPK signaling. J. Physiol. Biochem. 75 (1), 53–63. doi:10.1007/s13105-018-0651-z
Lv L.-X., Fang D. Q., Shi D., Chen D. Y., Yan R., Zhu Y. X., et al. (2016). Alterations and correlations of the gut microbiome, metabolism and immunity in patients with primary biliary cirrhosis. Environ. Microbiol. 18 (7), 2272–2286. doi:10.1111/1462-2920.13401
Ma C., Han M., Heinrich B., Fu Q., Zhang Q., Sandhu M., et al. (2018)., 360. New York, N.Y.), eaan5931. doi:10.1126/science.aan5931Gut microbiome-mediated bile acid metabolism regulates liver cancer via NKT cellsScience6391
Malik A., Sharma D., Malireddi R. K. S., Guy C. S., Chang T. C., Olsen S. R., et al. (2018). SYK-CARD9 signaling Axis promotes gut fungi-mediated inflammasome activation to restrict colitis and colon cancer. Immunity 49 (3), 515–530. doi:10.1016/j.immuni.2018.08.024
Martin P. K., Marchiando A., Xu R., Rudensky E., Yeung F., Schuster S. L., et al. (2018). Autophagy proteins suppress protective type I interferon signalling in response to the murine gut microbiota. Nat. Microbiol. 3 (10), 1131–1141. doi:10.1038/s41564-018-0229-0
Marx J. L. (1986). Human papilloma virus and cervical cancer. Sci. (New York, N.Y.) 231 (4741), 920. doi:10.1126/science.3003916
Matson V., Fessler J., Bao R., Chongsuwat T., Zha Y., Alegre M. L., et al. (2018). The commensal microbiome is associated with anti-PD-1 efficacy in metastatic melanoma patients. Sci. (New York, N.Y.) 359 (6371), 104–108. doi:10.1126/science.aao3290
McBrearty N., Arzumanyan A., Bichenkov E., Merali S., Merali C., Feitelson M. (2021). Short chain fatty acids delay the development of hepatocellular carcinoma in HBx transgenic mice. Neoplasia (New York, N.Y.) 23 (5), 529–538. doi:10.1016/j.neo.2021.04.004
McIntosh B. E., Hogenesch J. B., Bradfield C. A. (2010). Mammalian Per-Arnt-Sim proteins in environmental adaptation. Annu. Rev. Physiol. 72, 625–645. doi:10.1146/annurev-physiol-021909-135922
Miyamoto J., Igarashi M., Watanabe K., Karaki S. I., Mukouyama H., Kishino S., et al. (2019). Gut microbiota confers host resistance to obesity by metabolizing dietary polyunsaturated fatty acids. Nat. Commun. 10 (1), 4007. doi:10.1038/s41467-019-11978-0
Modica S., Murzilli S., Salvatore L., Schmidt D. R., Moschetta A. (2008). Nuclear bile acid receptor FXR protects against intestinal tumorigenesis. Cancer Res. 68 (23), 9589–9594. doi:10.1158/0008-5472.CAN-08-1791
Montalban-Arques A., Katkeviciute E., Busenhart P., Bircher A., Wirbel J., Zeller G., et al. (2021). Commensal Clostridiales strains mediate effective anti-cancer immune response against solid tumors. Cell Host Microbe 29 (10), 1573–1588.e7. doi:10.1016/j.chom.2021.08.001
Onorati A. V., Dyczynski M., Ojha R., Amaravadi R. K. (2018). Targeting autophagy in cancer. Cancer 124 (16), 3307–3318. doi:10.1002/cncr.31335
Ou J., Carbonero F., Zoetendal E. G., DeLany J. P., Wang M., Newton K., et al. (2013). Diet, microbiota, and microbial metabolites in colon cancer risk in rural Africans and African Americans. Am. J. Clin. Nutr. 98 (1), 111–120. doi:10.3945/ajcn.112.056689
Paik D., Yao L., Zhang Y., Bae S., D'Agostino G. D., Zhang M., et al. (2022). Human gut bacteria produce Τ17-modulating bile acid metabolites. Nature 603 (7903), 907–912. doi:10.1038/s41586-022-04480-z
Pant K., Saraya A., Venugopal S. K. (2017). Oxidative stress plays a key role in butyrate-mediated autophagy via Akt/mTOR pathway in hepatoma cells. Chem. Biol. Interact. 273, 99–106. doi:10.1016/j.cbi.2017.06.001
Pant K., Yadav A. K., Gupta P., Islam R., Saraya A., Venugopal S. K. (2017). Butyrate induces ROS-mediated apoptosis by modulating miR-22/SIRT-1 pathway in hepatic cancer cells. Redox Biol. 12, 340–349. doi:10.1016/j.redox.2017.03.006
Peng Z., Chen J., Drachenberg C. B., Raufman J. P., Xie G. (2019). Farnesoid X receptor represses matrix metalloproteinase 7 expression, revealing this regulatory axis as a promising therapeutic target in colon cancer. J. Biol. Chem. 294 (21), 8529–8542. doi:10.1074/jbc.RA118.004361
Peng Z., Raufman J.-P., Xie G. (2012). Src-mediated cross-talk between farnesoid X and epidermal growth factor receptors inhibits human intestinal cell proliferation and tumorigenesis. PloS One 7 (10), e48461. doi:10.1371/journal.pone.0048461
Pérez-Cano F. J., Gonzalez-Castro A., Castellote C., Franch A., Castell M. (2010). Influence of breast milk polyamines on suckling rat immune system maturation. Dev. Comp. Immunol. 34 (2), 210–218. doi:10.1016/j.dci.2009.10.001
Peters B. A., Dominianni C., Shapiro J. A., Church T. R., Wu J., Miller G., et al. (2016). Erratum to: The gut microbiota in conventional and serrated precursors of colorectal cancer. Microbiome 4 (1), 29. doi:10.1186/s40168-017-0238-x
Pleguezuelos-Manzano C., Puschhof J., Rosendahl Huber A., van Hoeck A., Wood H. M., Nomburg J., et al. (2020). Mutational signature in colorectal cancer caused by genotoxic pks+ E. coli. Nature 580 (7802), 269–273. doi:10.1038/s41586-020-2080-8
Poland J. C., Flynn C. R. (2021). Bile acids, their receptors, and the gut microbiota. Physiol. (Bethesda, Md.) 36 (4), 235–245. doi:10.1152/physiol.00028.2020
Ponziani F. R., Bhoori S., Castelli C., Putignani L., Rivoltini L., Del Chierico F., et al. (2019). Hepatocellular carcinoma is associated with gut microbiota profile and inflammation in nonalcoholic fatty liver disease. Hepatol. Baltim. Md.) 69 (1), 107–120. doi:10.1002/hep.30036
Pothuraju R., Chaudhary S., Rachagani S., Kaur S., Roy H. K., Bouvet M., et al. (2021). Mucins, gut microbiota, and postbiotics role in colorectal cancer. Gut microbes 13 (1), 1974795. doi:10.1080/19490976.2021.1974795
Printz C. (2021). Lower cervical cancer risk associated with HPV vaccine. Cancer 127 (8), 1171. doi:10.1002/cncr.33577
Qin N., Yang F., Li A., Prifti E., Chen Y., Shao L., et al. (2014). Alterations of the human gut microbiome in liver cirrhosis. Nature 513 (7516), 59–64. doi:10.1038/nature13568
Rao J. N., Xiao L., Wang J.-Y. (2020). Polyamines in gut epithelial renewal and barrier function. Physiol. (Bethesda, Md.) 35 (5), 328–337. doi:10.1152/physiol.00011.2020
Ray K., E. (2021). E. faecalis promotes liver carcinogenesis. Nat. Rev. Gastroenterol. Hepatol. 18 (12), 832–833. doi:10.1038/s41575-021-00540-3
Ren Z., Li A., Jiang J., Zhou L., Yu Z., Lu H., et al. (2019). Gut microbiome analysis as a tool towards targeted non-invasive biomarkers for early hepatocellular carcinoma. Gut 68 (6), 1014–1023. doi:10.1136/gutjnl-2017-315084
Roberti M. P., Yonekura S., Duong C. P. M., Picard M., Ferrere G., Tidjani Alou M., et al. (2020). Chemotherapy-induced ileal crypt apoptosis and the ileal microbiome shape immunosurveillance and prognosis of proximal colon cancer. Nat. Med. 26 (6), 919–931. doi:10.1038/s41591-020-0882-8
Rooks M. G., Garrett W. S. (2016). Gut microbiota, metabolites and host immunity. Nat. Rev. Immunol. 16 (6), 341–352. doi:10.1038/nri.2016.42
Round J. L., Mazmanian S. K. (2010). Inducible Foxp3+ regulatory T-cell development by a commensal bacterium of the intestinal microbiota. Proc. Natl. Acad. Sci. U. S. A. 107 (27), 12204–12209. doi:10.1073/pnas.0909122107
Salminen S., Collado M. C., Endo A., Hill C., Lebeer S., Quigley E. M. M., et al. (2021). The International Scientific Association of Probiotics and Prebiotics (ISAPP) consensus statement on the definition and scope of postbiotics. Nat. Rev. Gastroenterol. Hepatol. 18 (9), 649–667. doi:10.1038/s41575-021-00440-6
Sánchez-Alcoholado L., Ordonez R., Otero A., Plaza-Andrade I., Laborda-Illanes A., Medina J. A., et al. (2020). Gut microbiota-mediated inflammation and gut permeability in patients with obesity and colorectal cancer. Int. J. Mol. Sci. 21 (18), E6782. doi:10.3390/ijms21186782
Sanduzzi Zamparelli M., Rocco A., Compare D., Nardone G. (2017). The gut microbiota: A new potential driving force in liver cirrhosis and hepatocellular carcinoma. United Eur. Gastroenterol. J. 5 (7), 944–953. doi:10.1177/2050640617705576
Schneider K. M., Mohs A., Gui W., Galvez E. J. C., Candels L. S., Hoenicke L., et al. (2022). Imbalanced gut microbiota fuels hepatocellular carcinoma development by shaping the hepatic inflammatory microenvironment. Nat. Commun. 13 (1), 3964. doi:10.1038/s41467-022-31312-5
Seiwert N., Neitzel C., Stroh S., Frisan T., Audebert M., Toulany M., et al. (2017). AKT2 suppresses pro-survival autophagy triggered by DNA double-strand breaks in colorectal cancer cells. Cell Death Dis. 8 (8), e3019. doi:10.1038/cddis.2017.418
Serna G., Ruiz-PaceF. , Hernando J., Alonso L., Fasani R., Landolfi S., et al. (2020). Fusobacterium nucleatum persistence and risk of recurrence after preoperative treatment in locally advanced rectal cancer. Ann. Oncol. 31 (10), 1366–1375. doi:10.1016/j.annonc.2020.06.003
Sha'fie M. S. A., Rathakrishnan S., Hazanol I. N., Dali M. H. I., Khayat M. E., Ahmad S., et al. (2020). Ethanol induces microglial cell death via the NOX/ROS/PARP/TRPM2 signalling pathway. Antioxidants (Basel, Switz. 9 (12), E1253. doi:10.3390/antiox9121253
Sheng L., Jena P. K., Hu Y., Liu H. X., Nagar N., Kalanetra K. M., et al. (2017). Hepatic inflammation caused by dysregulated bile acid synthesis is reversible by butyrate supplementation. J. Pathol. 243 (4), 431–441. doi:10.1002/path.4983
Shi Y., Zheng W., Yang K., Harris K. G., Ni K., Xue L., et al. (2020). Intratumoral accumulation of gut microbiota facilitates CD47-based immunotherapy via STING signaling. J. Exp. Med. 217 (5), e20192282. doi:10.1084/jem.20192282
Singh V., Yeoh B. S., Chassaing B., Xiao X., Saha P., Aguilera Olvera R., et al. (2018). Dysregulated microbial fermentation of soluble fiber induces cholestatic liver cancer. Cell 175 (3), 679–694. doi:10.1016/j.cell.2018.09.004
Sivan A., Corrales L., Hubert N., Williams J. B., Aquino-Michaels K., Earley Z. M., et al. (2015). Commensal Bifidobacterium promotes antitumor immunity and facilitates anti-PD-L1 efficacy. Sci. (New York, N.Y.) 350 (6264), 1084–1089. doi:10.1126/science.aac4255
Sobhani I., Bergsten E., Couffin S., Amiot A., Nebbad B., Barau C., et al. (2019). Colorectal cancer-associated microbiota contributes to oncogenic epigenetic signatures. Proc. Natl. Acad. Sci. U. S. A. 116 (48), 24285–24295. doi:10.1073/pnas.1912129116
Sobhani I., Rotkopf H., Khazaie K. (2020). Bacteria-related changes in host DNA methylation and the risk for CRC. Gut Microbes 12 (1), 1800898. doi:10.1080/19490976.2020.1800898
Song X., Sun X., Oh S. F., Wu M., Zhang Y., Zheng W., et al. (2020). Microbial bile acid metabolites modulate gut RORγ+ regulatory T cell homeostasis. Nature 577 (7790), 410–415. doi:10.1038/s41586-019-1865-0
Stepien M., Keski-Rahkonen P., Kiss A., Robinot N., Duarte-Salles T., Murphy N., et al. (2021). Metabolic perturbations prior to hepatocellular carcinoma diagnosis: Findings from a prospective observational cohort study. Int. J. Cancer 148, 609–625. doi:10.1002/ijc.33236
Sudhakar P., Jacomin A. C., Hautefort I., Samavedam S., Fatemian K., Ari E., et al. (2019). Targeted interplay between bacterial pathogens and host autophagy. Autophagy 15 (9), 1620–1633. doi:10.1080/15548627.2019.1590519
Sun L., Cai J., Gonzalez F. J. (2021). The role of farnesoid X receptor in metabolic diseases, and gastrointestinal and liver cancer. Nat. Rev. Gastroenterol. Hepatol. 18 (5), 335–347. doi:10.1038/s41575-020-00404-2
Sung H., Ferlay J., Siegel R. L., Laversanne M., Soerjomataram I., Jemal A., et al. (2021). Global cancer statistics 2020: GLOBOCAN estimates of incidence and mortality worldwide for 36 cancers in 185 countries. Ca. Cancer J. Clin. 71 (3), 209–249. doi:10.3322/caac.21660
Tao R., de Zoeten E. F., Ozkaynak E., Chen C., Wang L., Porrett P. M., et al. (2007). Deacetylase inhibition promotes the generation and function of regulatory T cells. Nat. Med. 13 (11), 1299–1307. doi:10.1038/nm1652
Taverniti V., Guglielmetti S. (2011). The immunomodulatory properties of probiotic microorganisms beyond their viability (ghost probiotics: Proposal of paraprobiotic concept). Genes Nutr. 6 (3), 261–274. doi:10.1007/s12263-011-0218-x
Thomas A. M., Manghi P., Asnicar F., Pasolli E., Armanini F., Zolfo M., et al. (2019). Metagenomic analysis of colorectal cancer datasets identifies cross-cohort microbial diagnostic signatures and a link with choline degradation. Nat. Med. 25 (4), 667–678. doi:10.1038/s41591-019-0405-7
Tian Y., Xu Q., Sun L., Ye Y., Ji G. (2018). Short-chain fatty acids administration is protective in colitis-associated colorectal cancer development. J. Nutr. Biochem. 57, 103–109. doi:10.1016/j.jnutbio.2018.03.007
Tremblay W., Mompart F., Lopez E., Quaranta M., Bergoglio V., Hashim S., et al. (2021). Cytolethal distending toxin promotes replicative stress leading to genetic instability transmitted to daughter cells. Front. Cell Dev. Biol. 9, 656795. doi:10.3389/fcell.2021.656795
van Prehn J., Reigadas E., Vogelzang E. H., Bouza E., Hristea A., Guery B., et al. (2021). European society of clinical microbiology and infectious diseases: 2021 update on the treatment guidance document for Clostridioides difficile infection in adults. Clin. Microbiol. Infect. 27, S1–S21. doi:10.1016/j.cmi.2021.09.038
Vavassori P., Mencarelli A., Renga B., Distrutti E., Fiorucci S. (2009). The bile acid receptor FXR is a modulator of intestinal innate immunity. J. Immunol. 183 (10), 6251–6261. doi:10.4049/jimmunol.0803978
Wang L., Tang L., Feng Y., Zhao S., Han M., Zhang C., et al. (2020). A purified membrane protein from Akkermansia muciniphila or the pasteurised bacterium blunts colitis associated tumourigenesis by modulation of CD8+ T cells in mice. Gut 69 (11), 1988–1997. doi:10.1136/gutjnl-2019-320105
Wang R., Pan W., Jin L., Huang W., Li Y., Wu D., et al. (2020). Human papillomavirus vaccine against cervical cancer: Opportunity and challenge. Cancer Lett. 471, 88–102. doi:10.1016/j.canlet.2019.11.039
Wang X., Yang Y., Huycke M. M. (2015). Commensal bacteria drive endogenous transformation and tumour stem cell marker expression through a bystander effect. Gut 64 (3), 459–468. doi:10.1136/gutjnl-2014-307213
Willemsen L. E. M., Koetsier M. A., van Deventer S. J. H., van Tol E. A. F. (2003). Short chain fatty acids stimulate epithelial mucin 2 expression through differential effects on prostaglandin E(1) and E(2) production by intestinal myofibroblasts. Gut 52 (10), 1442–1447. doi:10.1136/gut.52.10.1442
Wirbel J., Pyl P. T., Kartal E., Zych K., Kashani A., Milanese A., et al. (2019). Meta-analysis of fecal metagenomes reveals global microbial signatures that are specific for colorectal cancer. Nat. Med. 25 (4), 679–689. doi:10.1038/s41591-019-0406-6
Wong S. H., Zhao L., Zhang X., Nakatsu G., Han J., Xu W., et al. (2017). Gavage of fecal samples from patients with colorectal cancer promotes intestinal carcinogenesis in germ-free and conventional mice. Gastroenterology 153 (6), 1621–1633. doi:10.1053/j.gastro.2017.08.022
Wu S., Rhee K. J., Albesiano E., Rabizadeh S., Wu X., Yen H. R., et al. (2009). A human colonic commensal promotes colon tumorigenesis via activation of T helper type 17 T cell responses. Nat. Med. 15 (9), 1016–1022. doi:10.1038/nm.2015
Yachida S., Mizutani S., Shiroma H., Shiba S., Nakajima T., Sakamoto T., et al. (2019). Metagenomic and metabolomic analyses reveal distinct stage-specific phenotypes of the gut microbiota in colorectal cancer. Nat. Med. 25 (6), 968–976. doi:10.1038/s41591-019-0458-7
Yan L., Chen Y., Chen F., Tao T., Hu Z., Wang J., et al. (2022). Effect of Helicobacter pylori eradication on gastric cancer prevention: Updated report from a randomized controlled trial with 26.5 Years of follow-up. Gastroenterology 163 (1), 154–162.e3. doi:10.1053/j.gastro.2022.03.039
Yang F., Huang X., Yi T., Yen Y., Moore D. D., Huang W. (2007). Spontaneous development of liver tumors in the absence of the bile acid receptor farnesoid X receptor. Cancer Res. 67 (3), 863–867. doi:10.1158/0008-5472.CAN-06-1078
Yang Y., Du L., Shi D., Kong C., Liu J., Liu G., et al. (2021). Dysbiosis of human gut microbiome in young-onset colorectal cancer. Nat. Commun. 12 (1), 6757. doi:10.1038/s41467-021-27112-y
Yang Y., Misra B. B., Liang L., Bi D., Weng W., Wu W., et al. (2019). Integrated microbiome and metabolome analysis reveals a novel interplay between commensal bacteria and metabolites in colorectal cancer. Theranostics 9 (14), 4101–4114. doi:10.7150/thno.35186
Yano J. M., Yu K., Donaldson G. P., Shastri G. G., Ann P., Ma L., et al. (2015). Indigenous bacteria from the gut microbiota regulate host serotonin biosynthesis. Cell 161 (2), 264–276. doi:10.1016/j.cell.2015.02.047
Yi Y., Shen L., Shi W., Xia F., Zhang H., Wang Y., et al. (2021). Gut microbiome components predict response to neoadjuvant chemoradiotherapy in patients with locally advanced rectal cancer: A prospective, longitudinal study. Clin. Cancer Res. 27 (5), 1329–1340. doi:10.1158/1078-0432.CCR-20-3445
Yu L.-X., Yan H. X., Liu Q., Yang W., Wu H. P., Dong W., et al. (2010). Endotoxin accumulation prevents carcinogen-induced apoptosis and promotes liver tumorigenesis in rodents. Hepatol. Baltim. Md.) 52 (4), 1322–1333. doi:10.1002/hep.23845
Yu T., Guo F., Yu Y., Sun T., Ma D., Han J., et al. (2017). Fusobacterium nucleatum promotes chemoresistance to colorectal cancer by modulating autophagy. Cell 170 (3), 548–563. doi:10.1016/j.cell.2017.07.008
Yuan J., Chen C., Cui J., Lu J., Yan C., Wei X., et al. (2019). Fatty liver disease caused by high-alcohol-producing Klebsiella pneumoniae. Cell Metab. 30 (4), 675–688. doi:10.1016/j.cmet.2019.08.018
Zagato E., Pozzi C., Bertocchi A., Schioppa T., Saccheri F., Guglietta S., et al. (2020). Endogenous murine microbiota member Faecalibaculum rodentium and its human homologue protect from intestinal tumour growth. Nat. Microbiol. 5 (3), 511–524. doi:10.1038/s41564-019-0649-5
Zhang J., Haines C., Watson A. J. M., Hart A. R., Platt M. J., Pardoll D. M., et al. (2019). Oral antibiotic use and risk of colorectal cancer in the United Kingdom, 1989-2012: A matched case-control study. Gut 68 (11), 1971–1978. doi:10.1136/gutjnl-2019-318593
Zhang M., Wang H., Tracey K. J. (2000). Regulation of macrophage activation and inflammation by spermine: A new chapter in an old story. Crit. Care Med. 28, N60–N66. doi:10.1097/00003246-200004001-00007
Zheng Y., Wang T., Tu X., Huang Y., Zhang H., Tan D., et al. (2019). Gut microbiome affects the response to anti-PD-1 immunotherapy in patients with hepatocellular carcinoma. J. Immunother. Cancer 7 (1), 193. doi:10.1186/s40425-019-0650-9
Zhou Y., Tan W., Zou J., Cao J., Huang Q., Jiang B., et al. (2021). Metabolomics analyses of mouse retinas in oxygen-induced retinopathy. Invest. Ophthalmol. Vis. Sci. 14 (1), 9. doi:10.1167/iovs.62.10.9
Zhu Z., Huang Y., Lv L., Tao Y., Shao M., Zhao C., et al. (2018). Acute ethanol exposure-induced autophagy-mediated cardiac injury via activation of the ROS-JNK-Bcl-2 pathway. J. Cell. Physiol. 233 (2), 924–935. doi:10.1002/jcp.25934
Zmora N., Suez J., Elinav E. (2019). You are what you eat: Diet, health and the gut microbiota. Nat. Rev. Gastroenterol. Hepatol. 16 (1), 35–56. doi:10.1038/s41575-018-0061-2
Keywords: gut microbiota, dysbiosis, colorectal cancer, hepatocellular carcinoma, carcinogenesis
Citation: Mei S, Deng Z, Chen Y, Ning D, Guo Y, Fan X, Wang R, Meng Y, Zhou Q and Tian X (2022) Dysbiosis: The first hit for digestive system cancer. Front. Physiol. 13:1040991. doi: 10.3389/fphys.2022.1040991
Received: 09 September 2022; Accepted: 01 November 2022;
Published: 22 November 2022.
Edited by:
Ka Man Law, Kaiser Permanente Bernard J. Tyson School of Medicine, United StatesReviewed by:
Rajbir Singh, Amity University Gurgaon, IndiaIngeborg Klymiuk, InstitutAllergosan, Austria
Copyright © 2022 Mei, Deng, Chen, Ning, Guo, Fan, Wang, Meng, Zhou and Tian. This is an open-access article distributed under the terms of the Creative Commons Attribution License (CC BY). The use, distribution or reproduction in other forums is permitted, provided the original author(s) and the copyright owner(s) are credited and that the original publication in this journal is cited, in accordance with accepted academic practice. No use, distribution or reproduction is permitted which does not comply with these terms.
*Correspondence: Xuefei Tian, MDAzNjQwQGhudWNtLmVkdS5jbg==