- 1King’s College London, Centre of Human & Applied Physiological Sciences, London, United Kingdom
- 2Laboratory of Gravitational Physiology of the Sensorimotor System, Institute of Biomedical Problems, Russian Academy of Sciences, Moscow, Russia
- 3Space Medicine Team, HRE-OM, European Astronaut Centre, European Space Agency, Cologne, Germany
- 4KBRwyle Laboratories GmbH, Cologne, Germany
Microgravity induces spinal elongation and Low Back Pain (LBP) but the pathophysiology is unknown. Changes in paraspinal muscle viscoelastic properties may play a role. Dry Immersion (DI) is a ground-based microgravity analogue that induces changes in m. erector spinae superficial myofascial tissue tone within 2 h. This study sought to determine whether bilateral m. erector spinae tone, creep, and stiffness persist beyond 2 h; and if such changes correlate with DI-induced spinal elongation and/or LBP.
Ten healthy males lay in the DI bath at the Institute of Biomedical Problems (Moscow, Russia) for 6 h. Bilateral lumbar (L1, L4) and thoracic (T11, T9) trunk myofascial tone, stiffness and creep (MyotonPRO), and subjective LBP (0-10 NRS) were recorded before DI, after 1h, 6 h of DI, and 30min post. The non-standing spinal length was evaluated on the bath lifting platform using a bespoke stadiometer before and following DI.
DI significantly modulated m. erector spinae viscoelastic properties at L4, L1, T11, and T9 with no effect of laterality. Bilateral tissue tone was significantly reduced after 1 and 6 h DI at L4, L1, T11, and T9 to a similar extent. Stiffness was also reduced by DI at 1 h but partially recovered at 6 h for L4, L1, and T11. Creep was increased by DI at 1 h, with partial recovery at 6 h, although only T11 was significant. All properties returned to baseline 30 min following DI. Significant spinal elongation (1.17 ± 0.20 cm) with mild (at 1 h) to moderate (at 6 h) LBP was induced, mainly in the upper lumbar and lower thoracic regions. Spinal length increases positively correlated (Rho = 0.847, p = 0.024) with middle thoracic (T9) tone reduction, but with no other stiffness or creep changes. Spinal length positively correlated (Rho = 0.557, p = 0.039) with Max LBP; LBP failed to correlate with any m. erector spinae measured parameters.
The DI-induced bilateral m. erector spinae tone, creep, and stiffness changes persist beyond 2 h. Evidence of spinal elongation and LBP allows suggesting that the trunk myofascial tissue changes could play a role in LBP pathogenesis observed in real and simulated microgravity. Further study is warranted with longer duration DI, assessment of IVD geometry, and vertebral column stability.
1 Introduction
Low back pain (LBP) is the most common musculoskeletal condition affecting adults worldwide (Balagué et al., 2012). Typically, additional loading exaggerates LBP, although in the majority of individuals the specific aetiology of LBP cannot be identified (Hartvigsen et al., 2018). Acute exposure to microgravity is also associated with LBP (Kertsman et al., 2012), and whilst its aetiology is also unknown, the absence of diurnal gravitational loading on the spine presumably plays a key role (Sayson et al., 2013). In addition to LBP, microgravity induces stature increments of up to 6 cm evident in the first few days of flight (Brown, 1977). Such increments can cause operational issues including difficulties donning an Extra-Vehicular Activity (EVA) suit or fitting into the pre-moulded “Kazbek” seat pan of the Russian Soyuz capsule (Rajulu and Benson, 2009).
Microgravity-induced changes to the spinal column may also increase the risk of spinal injury due to the loading generated during in-orbit exercise on the International Space Station (ISS), descent, and landing, in addition to activity in the post-flight rehabilitation phase (Green and Scott, 2018). Such risks pose a significant health concern for space exploration missions and in particular those to the Lunar or Martian surface (Pool-Goudzwaard et al., 2015).
Astronauts frequently report LBP in the hours following insertion into microgravity that can persist for up to 4 weeks (Wing et al., 1991; Kertsman et al., 2012). The majority of astronauts (86%) report lumbar pain, although thoracic (12%) and cervical pain (2%) have also been noted (Kertsman et al., 2012). Whilst the pathophysiology of microgravity-induced LBP is unknown, supra-physiological swelling of intervertebral discs (IVD) has been suggested (Sayson et al., 2013; Belavý et al., 2016; Robin, et al., 2022). Indeed, a recent in-flight ultrasound evaluation of IVDs revealed disk desiccation, osteophytes, and qualitative changes in angle and height (Garcia et al., 2018). A further on-ground experiment has revealed an increase in IVD and elevated apparent diffusion coefficient (Treffel et al., 2020). However, magnetic resonance imaging identified no changes in IVD geometry in six NASA astronauts from pre-flight, 1–2 days, and again 2 months post-landing (Chang et al., 2016) although changes in IVD endplates and impaired spinal segment kinematics are potentially related to trunk muscle atrophy may contribute to post-flight IVD herniation risk (Bailey et al., 2018; Bailey et al., 2022).
Whilst microgravity-induced declines in muscle power, strength, and tone secondary to muscle atrophy are observed in the lower limbs (Kozlovskaya, 2007) and in paraspinal (anti-gravity) muscles (Schneider et al., 2015; Rukavishnikov et al., 2017; Amirova et al., 2021) such changes cannot underlie the rapid onset of LBP (Green and Scott, 2018). However, acute changes in paraspinal muscle and superficial myofascial tissue biomechanical and viscoelastic properties may potentially precipitate microgravity-induced LBP.
For instance, on Earth chronic LBP sufferers show a significant reduction in lower back muscle and myofascial tone (Nair et al., 2016). In fact, changes in lumbar myofascial tissue tone correlate with chronic LBP (Haladaj and Topol, 2016). Interestingly, research performed by teams from the Institute of Biomedical Problems (IBMP) in Moscow (Russia) provided evidence of rapid muscle tone change termed hypogravitational muscle syndrome following gravitational unloading (Grigor’ev et al., 2004; Rukavishnikov et al., 2017). Reductions in tone and stiffness—have also been reported in several lower limb muscles e.g., m. triceps surae: m. gastrocnemius lateralis and medialis, and m. soleus (Nemirovskaya and Shenkman, 2002; Kozlovskaya, 2007; Miller et al., 2012). Such changes may be triggered by the absence of stimuli that in gravity activate postural muscle activity (Kozlovskaya, 2007; Shenkman et al., 2021).
Indeed, a recent parabolic flight revealed rapid declines in the transverse tone of m. erector spinae (Schneider et al., 2015). Similar declines have been suggested to play a role in the development of LBP on Earth (Sung et al., 2009). Conceivably, acute reductions in paraspinal tone may contribute to IVD swelling (Chang et al., 2016) and/or spinal curvature flattening (Lee et al., 2003) and thereby spinal elongation (Rukavishnikov et al., 2017), vertebral column instability (Belavý et al., 2016) and LBP (Amirova et al., 2021). Furthermore, creep, a viscoelastic property that describes progressive elongation of tissue under tensile stress (Schneider et al., 2015) may also provide insight into changes that contribute to microgravity-induced spinal column elongation and LBP (Abboud et al., 2016).
Unfortunately, spinal assessment on the ISS has largely been restricted to pre- and post-flight measures (e.g., Chang et al., 2016; Bailey et al., 2022). Furthermore, whilst head-down tilt bed rest (HDTBR) is the most commonly employed ground-based microgravity analogue (Hargens and Vico, 2016; Vico and Hargens, 2018), its ability to induce back pain (Hutchinson et al., 1995), and other spinal column changes associated with spaceflight appears limited (Green and Scott, 2018). For instance, increments in spinal length after 3 days of HDTBR (Belavý et al., 2011) did not differ from 8 h of regular sleep on Earth (Styf et al., 1997).
Dry Immersion (DI) is an alternative ground-based microgravity analogue that involves the removal of mechanical support (supportlessness) that was developed by IBMP in the 1960s (Shulzhenko and Vil-Vilyams, 1975). DI involves the subject being “immersed” in a water-filled bath whilst laying on a waterproof flexible material allowing them to remain dry while being freely suspended (Tomilovskaya et al., 2019). DI has been reported to rapidly induce LBP of similar nature and intensity to that induced in microgravity (Rukavishnikov et al., 2017; Tomilovskaya et al., 2019). As a result, DI has been proposed as a potentially more valid analogue of microgravity-induced LBP (Kozlovskaya, 2008; Navasiolava et al., 2011; Tomilovskaya et al., 2019).
DI via resonance vibrography has been demonstrated to induce a rapid change in myofascial tissue properties, including a bilateral decrease in back extensor tone (m. longissimus dorsi in the projection of L2–L3) (Rukavishnikov et al., 2017). Furthermore, a rapid bilateral drop in m. erector spinae (in T9-T8, T12-L1, and L3-L4 cord projection) and trapezius muscle tone were recently observed during up to 2 h of DI (Amirova et al., 2021). However, whether such changes persist, and are correlated with LBP is unclear. In addition, asymmetry of paraspinal (Danneels et al., 2000; Hides et al., 2008) and pelvic (Levangie, 1999) muscle biomechanical and viscoelastic properties have been suggested to be a potential factor underlying LBP aetiology and prognosis on Earth (Hodges et al., 2006).
Amirova and co-workers (2021) employed handheld myotonometry (MyotonPRO) which has been used in parabolic flight (Schneider et al., 2015), HDTBR (Schoenrock et al., 2018), and DI (Treffel et al., 2016; de Abreu et al., 2017; Rukavishnikov et al., 2017). The MyotonPRO is reported to provide reliable paraspinal (Hu et al., 2018) resting myofascial tissue tone assessment (Kelly et al., 2018; Muckelt et al., 2022) via analysis of induced tissue oscillations (Lo et al., 2017). The MyotonPRO also provides indices of dynamic stiffness and creep (Simons and Mense, 1998). However, these measures were not reported by Amirova and co-workers (2021), although increased muscle stiffness was observed in m. erector spinae and m. lumbar multifidus following 60-days HDTBR (Schoenrock et al., 2018). Thus, this study aimed to determine whether bilateral m. erector spinae tone, in addition to creep and stiffness, persist beyond 2 h, and if such changes are associated with DI-induced spinal elongation and/or LBP.
2 Methods
Ten healthy males (Table 1) provided written informed consent to participate in the study that received ethical approval from the Physiological Section of the Biomedicine Ethics Committee at the Institute of Biomedical Problems, a part of the Russian Academy of Science (IRB protocol #401, 15.07.2015). All subjects reported no musculoskeletal (including spinal) disorder prior to inclusion in the study.
Each participant attended the laboratory at the Institute of Biomedical Problems (Moscow, Russia) on a single occasion, where they lay for 6 h in the DI bath. Lumbar and thoracic trunk myofascial tone, stiffness, and creep, as well as subjective LBP, were recorded 2 h before (PRE), after 1 (1 h DI) and 6 h (6 h DI) of DI, and 30 min after (RECOVERY) being raised out of DI. The non-standing spinal length was evaluated before (PRE) and following (POST) DI.
Subjects were passively lowered into and raised out of the DI water-filled bath (210 × 90 × 110 cm) covered with a non-elastic waterproof fabric on an in-built lifting platform (Figure 1). The water was maintained at a comfortable thermoneutral temperature of 32.5°C ± 2°C, with an ambient temperature of 24°C–26°C. Subjects were requested to remain supine, relaxed, and as still as possible throughout testing.
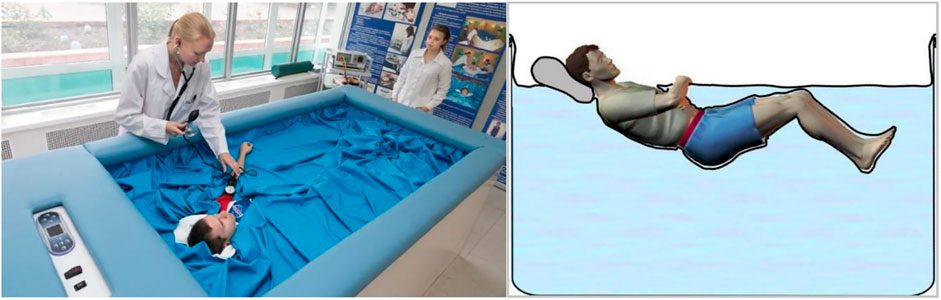
FIGURE 1. Left: Subject immersed in the Dry Immersion (DI) bath being separated from the water by non-elastic waterproof fabric (Image credit: IBMP press secretary Oleg Voloshin). Right: Schematic representation of the body posture in DI (Shulzhenko and Vil-Villiams, 1975).
Bilateral viscoelastic properties of lumbar and thoracic trunk myofascial tissues (Lower Lumbar (LL4, LR4), Upper Lumbar (LL1, LR1), Lower Thoracic (TL11, TR11), and Middle Thoracic (TL9, TR9) regions (Figure 2A) were assessed using a small handheld MyotonPRO (Myoton AS, Estonia) with subjects in the prone position with arms under the forehead, on a couch for PRE and POST DI recordings (Figure 2B). Assessment during DI required temporary lifting of the subjects with the in-built platform to allow passive turning to prone position (Figure 2C) by a team of investigators before immediately being turned back and returned to the supine DI position. Measurement points were marked on the skin above the muscle belly to ensure recording repeatability.
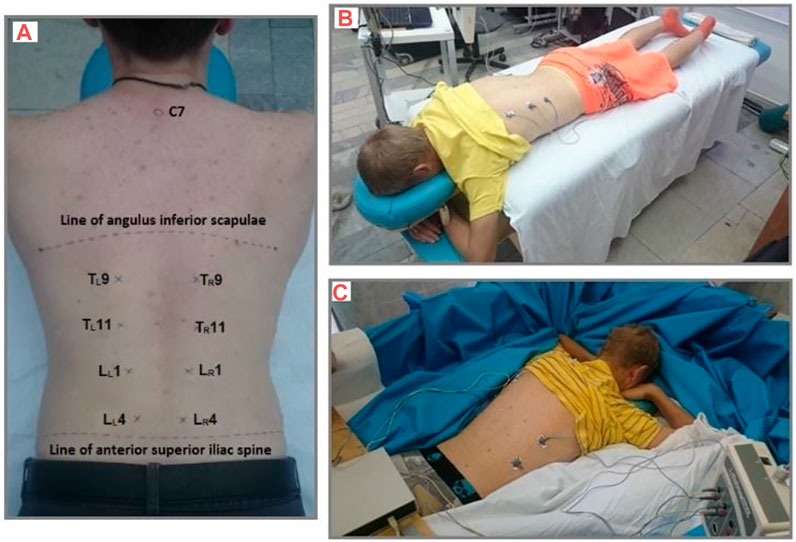
FIGURE 2. Measurement of Lower Lumbar (LL4, LR4), Upper Lumbar (LL1, LR1), Lower Thoracic (TL11, TR11), and Middle Thoracic (TL9, TR9) locations. (A) Subject remained prone for lumbar and thoracic trunk myofascial tissue assessment (B) on a couch, and (C) in the DI bath.
The MyotonPRO applies a mechanical impulse to the tissue at the application point with the probe placed perpendicularly to the skin. Consistent generation of approx. 0.18 N pre-load—to ensure subcutaneous tissue pre-compression—activates a series of five short (15 ms) 0.4 N impulses of the instrumented actuator. The following tissue properties were calculated: tone (natural oscillation frequency, Hz), biomechanical properties—dynamic stiffness (N/m), and viscoelastic properties—creep (ratio of deformation with relaxation time: Deborah Number) (Schneider et al., 2015).
The recordings were obtained at rest (confirmed by real-time EMG recording <0.1 mV (Neuro-MEP-4 device, Russia) of the measurement site following subjects being instructed to exhale normally and then hold their breath around their Functional Residual Capacity.
Spinal length (distance from cranium vertex to the point where m. gluteus maximus abuts to the angled hip support) was measured when on the DI bath lifting platform using a bespoke stadiometer (specifically designed and made for the current experiment by Dr. I. Rukavishnikov, Figure 3). The stadiometer is designed to replicate an astronaut’s body position in a Soyuz “Kazbek” seat pan whilst also eliminating axial gravitational loading.
DI-induced subjective back pain was assessed via a body map for pain localization with a numeric Rating Scale (NRS: 0–10 severe pain) employed to evaluate pain intensity (Hawker et al., 2011).
All physiological data were normally distributed (Kolmogorov—Smirnov test). The effect of laterality (Left vs. Right side) on DI-induced trunk myofascial viscoelastic properties was assessed with a two-way ANOVA during DI (PRE, 1 h DI, 6 h DI, RECOVERY). As no effect of laterality was observed, data from both sides were pooled for each location and the effect of time (DI exposure) was evaluated by a one-way ANOVA, with post-hoc Bonferroni corrected t-tests.
The effect of 6 h DI on spinal length was evaluated using paired t-tests (PRE vs. RECOVERY). DI effects on maximum pain at 1 h DI, 6 h DI, and RECOVERY were evaluated using Wilcoxon signed-rank testing.
The relationship between changes in trunk myofascial tissue tone, stiffness, creep, spinal length, and maximum-induced pain intensity was assessed via non-parametric Spearman’s correlation (Rho).
All data is represented as Mean ± Standard Error Mean (SEM). All statistical analysis was performed using the Prism8 software (GraphPad Software, Inc. United States) with statistical significance defined as p < 0.05.
3 Results
As trunk myofascial tissue tone, stiffness and creep did not significantly differ between the left and right recordings they were averaged at all levels.
Mean bilateral m. erector spinae myofascial tissue tone demonstrated a similar trend across all locations (Figure 4). Mean Lower Lumbar (L4) myofascial tone was significantly (F3,27 = 14.12, p < 0.001) reduced by DI (Figure 4A). Myofascial tone (p = 0.003) declined by 6.5% ± 0.5% after 1 h DI. However, Lower Lumbar myofascial tone was not significantly (p = 0.070) lower than PRE at 6 h DI with no difference between 1 and 6 h DI (p > 0.999). RECOVERY Lower Lumbar myofascial tone did not differ from PRE (p = 0.360).
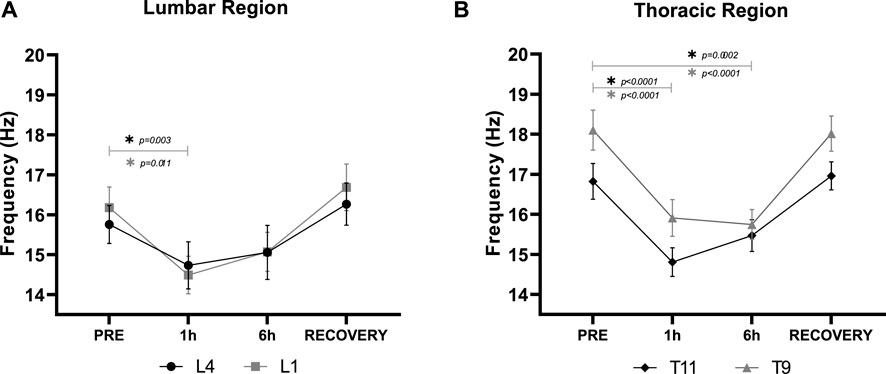
FIGURE 4. Mean (±SEM) bilateral m. erector spinae myofascial tone (Hz) in the (A): Lower Lumbar (L4) and Upper Lumbar (L1), and (B): Lower Thoracic (T11), and Middle Thoracic (T9) regions before (PRE), during Dry Immersion (DI) after 1 (1 h) and 6 (6 h) hours, and 30 min after being raised out of DI (RECOVERY). *Statistically significant difference (p < 0.05) between time points (post-hoc Bonferroni corrected t-tests).
Similarly, Upper Lumbar (L1) myofascial tone was significantly (F3,27 = 8.48, p < 0.001) reduced by DI (Figure 4A). Myofascial tone significantly (p = 0.010) declined by 10.4 ± 0.1% after 1 h DI. However, Upper Lumbar myofascial tone was not significantly (p = 0.194) lower than PRE at 6 h, nor between 1 and 6 h DI (p > 0.999). RECOVERY Upper Lumbar myofascial tone did not differ from PRE (p > 0.999).
Lower Thoracic (T11) myofascial tone was significantly (F3,27 = 30.08, p < 0.001) reduced by DI (Figure 4B). Myofascial tone significantly (p < 0.001) declined by 12.0% ± 0.7% after 1 h DI and by 8.0% ± 0.01% after 6 h (p < 0.001). No difference between 1 and 6 h DI (p > 0.999) was observed. RECOVERY Lower Thoracic myofascial tone did not differ from PRE (p > 0.99).
Middle Thoracic Region (T9) myofascial tone was significantly (F3,27 = 68.07, p < 0.001) reduced by DI (Figure 4B). Myofascial tone significantly (p < 0.001) declined by 12.1% ± 0.5% after 1 h, and by 13.0% ± 0.2% (p < 0.001) after 6 h of DI. No difference between 1 and 6 h DI (p > 0.999) was observed. RECOVERY Middle Thoracic myofascial tone did not differ from PRE (p > 0.999).
Mean bilateral m. erector spinae myofascial tissue stiffness demonstrated somewhat similar trends across all locations. Lower Lumbar (L4) myofascial stiffness was significantly (F3,27 = 14.2, p < 0.001) reduced by DI (Figure 5A). Myofascial stiffness significantly (p < 0.001) declined by 16.6% ± 2.8% after 1 h DI. However, there was a partial recovery at 6 h DI which was significantly (p = 0.005) higher than 1 h DI, and not significantly differ from PRE (p = 0.600). RECOVERY Lower Lumbar myofascial stiffness did not differ from PRE (p = 0.100).
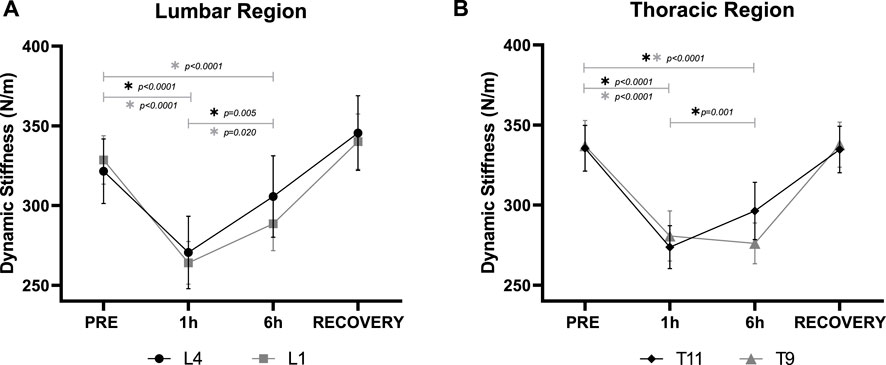
FIGURE 5. Mean (±SEM) bilateral m. erector spinae myofascial dynamic stiffness (N/m) in the (A): Lower Lumbar (L4) and Upper Lumbar (L1), and (B): Lower Thoracic (T11), and Middle Thoracic (T9) regions before (PRE), during Dry Immersion (DI) after 1 (1 h) and 6 (6 h) hours, and 30 min after being raised out of DI (RECOVERY). *Statistically significant difference (p < 0.05) between time points (post-hoc Bonferroni corrected t-tests).
Upper Lumbar (L1) myofascial stiffness was significantly (F3,27 = 43.53, p < 0.001) reduced by DI (Figure 5A). Myofascial stiffness significantly (p < 0.001) declined by 19.6% ± 2.0% after 1 h, and by 12.3% ± 2.8% (p < 0.001) after 6 h DI, although the latter was significantly (p = 0.020) weaker. RECOVERY Upper Lumbar myofascial stiffness did not differ from PRE (p = 0.850).
Lower Thoracic (T11) myofascial stiffness was also significantly (F3,27 = 68.52, p < 0.001) reduced by DI (Figure 5B). Myofascial stiffness significantly (p < 0.001) declined by 18.5% ± 1.3% after 1 h, and by 12.2 ± 2.0% (p < 0.001) after 6 h DI, although the latter was significantly (p = 0.001) weaker. RECOVERY Lower Thoracic myofascial stiffness did not differ from PRE (p > 0.999).
Middle Thoracic (T9) myofascial stiffness was significantly (F3,27 = 75.36, p < 0.001) reduced by DI (Figure 5B). Myofascial stiffness significantly (p < 0.001) declined by 16.9% ± 1.5% after 1 h (p < 0.001) and 18.0% ± 1.3% (p < 0.001) after 6 h DI. No difference between 1 and 6 h DI (p > 0.999) Middle Thoracic stiffness was observed, and RECOVERY did not differ from PRE (p > 0.999).
Mean bilateral m. erector spinae myofascial creep demonstrated a similar trend across all locations. Lower Lumbar (L4) myofascial tissue creep was significantly (F3,27 = 16.87, p < 0.001) increased by DI (Figure 6A). Creep significantly (p < 0.001) increased by 15.8% ± 3.3% after 1 h DI which was weaker at 6 h and thus not significantly different from PRE (p = 0.17) whilst tending to be lower than 1 h DI (p = 0.060). RECOVERY Lower Lumbar creep did not differ from PRE (p = 0.710).
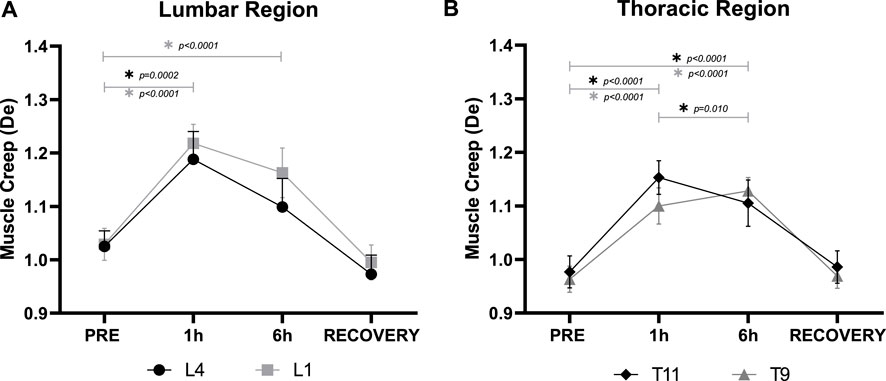
FIGURE 6. Mean (±SEM) bilateral m. erector spinae myofascial creep (De) in the (A): Lower Lumbar (L4) and Upper Lumbar (L1), and (B): Lower Thoracic (T11), and Middle Thoracic (T9) regions before (PRE), during Dry Immersion (DI) after 1 (1 h) and 6 (6 h) hours, and 30 min after being raised out of DI (RECOVERY). *Statistically significant difference (p < 0.05) between time points (post-hoc Bonferroni corrected t-tests).
Upper Lumber (L1) myofascial creep was significantly (F3,27 = 37.47, p < 0.001) increased by DI (Figure 6A). Myofascial creep significantly (p < 0.001) increased by 18.7% ± 2.8% after 1 h, that was 13.0% ± 3.0% (p < 0.001) after 6 h DI although they did not differ between 1 and 6 h (p = 0.200). RECOVERY Upper Lumber myofascial creep did not differ from PRE (p > 0.999).
Lower Thoracic (T11) myofascial creep was also significantly (F3,27 = 75.28, p < 0.001) increased by DI (Figure 6B). Myofascial creep significantly (p < 0.001) increased by 18.1% ± 1.2% after 1 h, that was 12.8% ± 1.7% (p < 0.001) after 6 h DI leading to a significant (p = 0.010) difference between 1 and 6 h DI. RECOVERY Lower Thoracic creep did not differ from PRE (p > 0.999).
Middle Thoracic (T9) creep was also significantly (F3,27 = 61.02, p < 0.001) increased by DI (Figure 6B). Creep significantly (p < 0.001) increased by 14.1% ± 1.7% after 1 h, and 17.4% ± 1.5% (p < 0.001) after 6 h DI although no difference (p = 0.500) between 1 and 6 h DI. RECOVERY Middle Thoracic creep did not differ from PRE (p > 0.999).
All participants exhibited spinal elongation resulting in a significant (p < 0.001) mean spinal length increase after 6 h DI (POST-PRE) (Table 2).
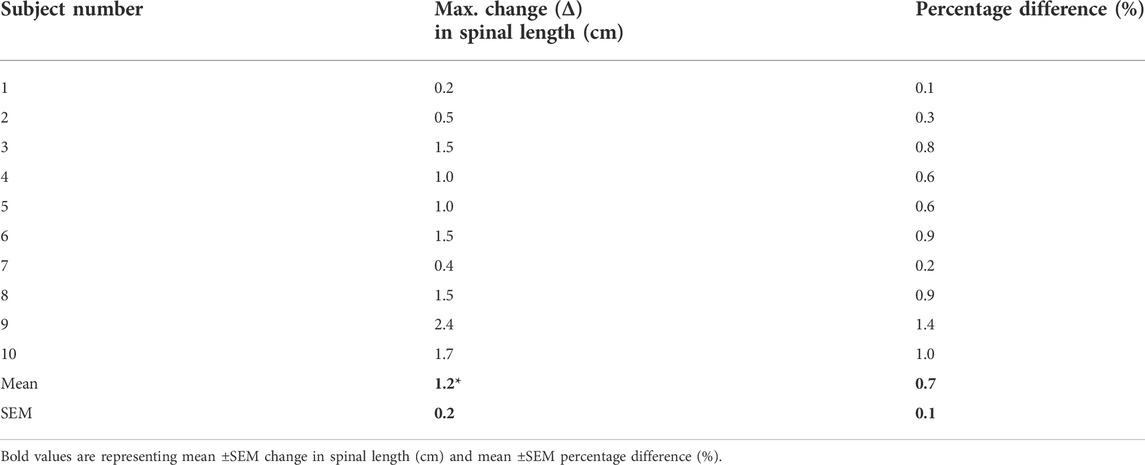
TABLE 2. Individual and mean (±SEM) (Δ) change in spinal length Post-Pre 6 h Dry Immersion (DI). * Indicates significant paired t-test (p < 0.001).
No subject reported back pain prior to DI (PRE). Seven out of ten subjects reported back pain (1.4 ± 0.9) after 1 h DI that was significantly (p = 0.031) higher at 6 h (3.4 ± 1.3) vs. 1 h DI (Figure 7A). The highest pain rating was 5 (equating to ‘moderate pain’ on the 0-10 NRS) reported by two subjects. Back pain was significantly (p = 0.016) ameliorated 30 min after DI (RECOVERY) compared to 6 h DI.
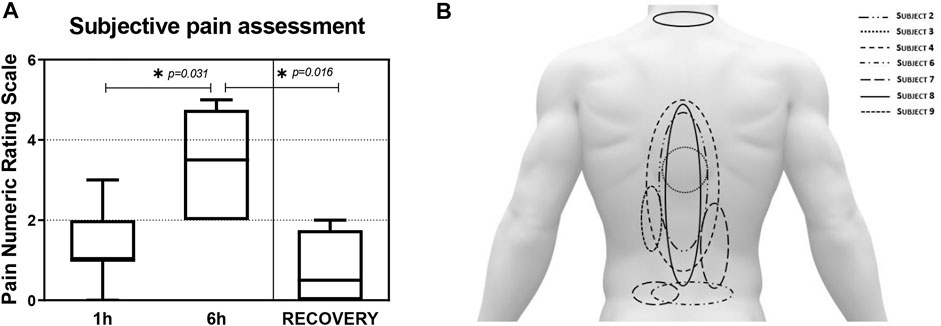
FIGURE 7. (A): Mean (±SEM) back pain intensity (Numeric Rating Scale: 0–10: Max) scores after 1 (1 h), 6 h (6 h) Dry Immersion (DI), and 30 min after DI (RECOVERY). *Statistically significant (p < 0.05) Wilcoxon signed-rank test. (B): Subjective back pain locations as drawn by the seven (out of ten) subjects that reported back pain after 6 h DI.
The seven subjects who reported back pain stated that it radiated on both sides of the spine (Figure 7B). Most subjects reported pain in the Upper Lumbar and Lower Thoracic regions, with only one subject reporting pain in the Cervical region. No radiating of pain to the gluteal region or lower limbs was reported.
Spinal elongation positively correlated with Maximal LBP (Figure 8A). Spinal elongation and Maximal LBP failed to correlate with any changes in m. erector spinae viscoelastic properties except for Middle Thoracic region. Spinal length increases induced by 6 h DI positively correlated (Rho = 0.847; p = 0.024) with Middle Thoracic (T9) myofascial tone reduction (Figure 8B).
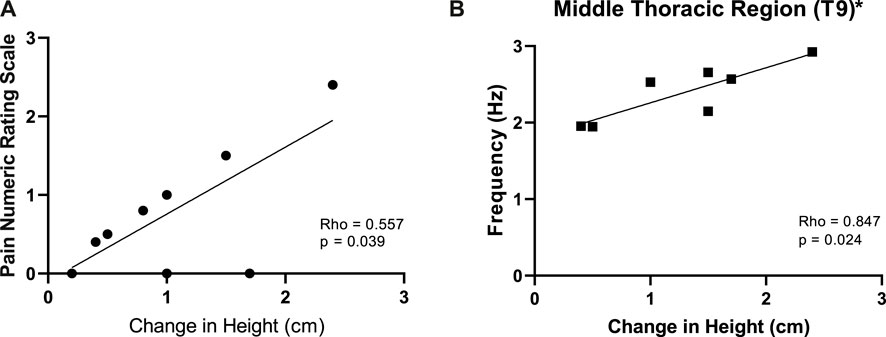
FIGURE 8. Relationship between the changes in spinal height (Post-Pre) (cm) vs. back pain (A) and changes in Middle Thoracic (T9) m. erector spinae tone (Hz) (B) induced by 6 h Dry Immersion (DI). * Statistically significant Spearman’s correlation (Rho).
4 Discussion
The main findings of the study were that DI significantly modulated m. erector spinae myofascial tissue biomechanical and viscoelastic properties at L4, L1, T11, and T9 with no effect on laterality. The bilateral myofascial tone was reduced after 1 and 6 h DI to a similar extent. Stiffness was also reduced by DI at 1 h but partially recovered at 6 h for L4, L1, and T11. Creep was increased by DI at 1 h, which also tended to partially recover at 6 h, although only T11 was significant. All measured parameters returned to baseline levels following DI. Significant (albeit mild) spinal elongation was induced by 6 h DI in all subjects, with the majority reporting LBP, mainly in the upper lumbar and lower thoracic regions that were mild at 1 h but increased to moderate after 6 h DI. Spinal length increases correlated with middle thoracic (T9) myofascial tone reduction, but with no other viscoelastic changes. Spinal length positively correlated with max LBP, but LBP failed to correlate with any m. erector spinae measured parameters.
4.1 Changes in m. erector spinae myofascial tissue properties
No effect of laterality (i.e., asymmetry) was observed on m. erector spinae viscoelastic properties recorded via myotonometry with the MyotonPRO. This contrasts with the asymmetry reported in paraspinal (Danneels et al., 2000; Hides et al., 2008) and pelvic (Levangie, 1999) muscle viscoelastic properties proposed to contribute to LBP on Earth (Hodges et al., 2006). However, as the DI exposure was only 6 h and only 10 subjects were tested further study is warranted.
DI significantly reduced bilateral m. erector spinae tone at 1 and 6 h to a similar extent—consistent with rapid declines in the transverse tone of m. erector spinae induced by acute (<22 s) unloading (Schneider et al., 2015). The study also extends findings of rapid bilateral reductions in m. erector spinae at the T9-T8, T12-L1, and L3-L4 cord projections and trapezius myofascial tone during up to 2 h of DI (Amirova et al., 2021).
Bilateral m. erector spinae stiffness was also reduced by DI at 1 h, but that partially recovered at 6 h for L4, L1, and T11. Thus, the present study suggests that short exposure to DI can trigger an acute reduction in myofascial tissue tone and stiffness, indicating possible changes in motor neuron activation patterns, and suppression of tonic motor neuron activities (Kozlovskaya and Kirenskaya, 1987; Kozlovskaya, 2002; Kozlovskaya et al., 2006; Shenkman et al., 2007), sensory and motor ataxia (Tomilovskaya et al., 2014; Shigueva et al., 2015), and significant hypersensitivity of the proprioceptive pathways (Grigor’ev et al., 2004).
Reductions in m. erector spinae stiffness contrast with increases observed in m. erector spinae and m. lumbar multifidus following 60-days HDTBR (Schoenrock et al., 2018). However, HDTBR is not associated with LBP (see Green and Scott, 2018). Thus, the DI-induced changes in myofascial tone may relate to the withdrawal of support (Kozlovskaya et al., 2007) and may be associated with LBP development. However, LBP was increased at 6 vs. 1 h, in contrast to attenuation of stiffness reductions at L4, L1, and T11. Yet, it may be that differential changes in stiffness around the vertebral column contribute to the development of LBP (Sung et al., 2009).
According to Grigor’ev and co-workers (Grigor’ev et al., 2004), DI promotes partial ‘deafferentation’ of the nervous system due to the withdrawal of support stimuli, accompanied by reduced muscle proprioceptive input. An association between muscle tone changes and support-related afferentation has been observed in the lower limbs (Kozlovskaya et al., 1984; Kozlovskaya, 2002) and our data suggests similar effects may be manifest in trunk musculature.
Myofascial creep was increased by DI at 1 h, that also tended to partially recover at 6 h, although only T11 was significant. To the authors’ knowledge, this study is the first to examine the association of LBP induced by support unloading with myofascial creep. The increase in viscoelastic tissue creep could be partially explained by the opposition of gravitational force withdrawal (Guo, 2002; Solomonow et al., 2003) and the diminishing protection from spine instability (Solomonow, 2012; Treffel et al., 2017).
DI induces a “flexed” spinal curvature similar to that observed in microgravity (NASA–STD-3000, 2014). As the pelvis is relatively heavy, it tends to sink during DI; the thorax, in contrast, tends to rise due to the buoyancy of the chest cavity and the fact that the head and neck are elevated on a pillow. Despite this, the withdrawal of gravitational loading reduces axial loads on the torso, and may significantly modulate lumbar spine stabilization by eliciting viscoelastic changes in paraspinal muscles (LeBlanc et al., 1994). Furthermore, an increase in myofascial creep could be associated with activation of the trunk muscles in response to viscoelastic changes, which could modulate dynamic intervertebral disc strain (Hayward and Dolan, 2018) that can precipitate LBP development. However, further study is required to investigate this hypothesis.
Interestingly, 30 min after DI all measured parameters recovered to PRE levels—confirming that whilst DI induces viscoelastic and biomechanical changes, they are rapidly reversible. Such findings support the proposition that post-flight testing should be rapid—to avoid failure to observe significant spinal geometric changes (Chang et al., 2016) despite small increases in IVD height observed in flight (Garcia et al., 2018), due to recompression (Green and Scott, 2018).
It is important to acknowledge that MyotonPRO is a highly sensitive device whose measurement accuracy may be affected by the differences in subjects’ positions. The current study has tried to maintain a similar position of the subjects through all measurement stages whenever possible, although the positions of their hand on the couch and in the bath differed slightly due to bath design.
4.2 Spinal elongation
Whilst significant albeit mild (1.2 ± 0.2 cm) spinal elongation was induced by 6 h DI. However, the largest increase in a single participant was 2.4 cm. Such increments, whilst substantial are lower than some spinal length changes reported inflight (i.e., 1.5–6.1 cm; Brown, 1977; Kimura et al., 2001; Kertsman et al., 2012; Young and Rajulu, 2020). Whilst the underlying mechanisms are unknown spinal elongation may be associated with a reduction of lumbar and thoracic curvature, and/or an increase in vertebral disc height (Sayson & Hargens, 2008; Sayson et al., 2013; Belavy et al., 2016). Declines in postural myofascial tissue tone and stiffness may potentially promote increases in IVD height. Whether this is in a case in DI warrants further study.
4.3 Low back pain
6 h of DI induced LBP in 7 out of 10 participants, mainly in the upper lumbar and lower thoracic regions that was mild at 1 h but increased to moderate at 6 h. The LBP was described as dull and deep, which are prominent features of pain originating from lower lying tissues (Mannion et al., 2007) not assessed in this study. During DI, visceral mass is drawn towards the diaphragm muscle, which may explain the prevalence of pain in the lumbar region and visceral subcostal pain. Moreover, the flexed lower limb position in DI can lead to retroversion of the pelvis, which could facilitate the decrease in lumbar curvature (Treffel et al., 2016).
Data obtained from previous DI experiments (3–21 days) demonstrated that back pain sensations - described as blunt aching, mainly in the upper lumbar spine region (Rukavishnikov et al., 2017; Tomilovskaya et al., 2020) were observed during the first 36 h in DI (Rukavishnikov et al., 2017; Treffel et al., 2017)—again broadly consistent with that reported by some crew (Pool-Goudzwaard et al., 2015) during early spaceflight (Kertsman et al., 2012). A decrease in myofascial tissue tone, spinal elongation, and the development of LBP does not necessarily correlate in time. Pain develops as a consequence of reduced muscle tone and an increase in spinal length. Thus, the sensation of pain requires a longer time to develop than physical changes. LBP observed in previous studies (Rukavishnikov et al., 2017; Tomilovskaya et al., 2020) has only peaked after 12–24 h h of unloading. This could suggest that time spent in the bath was insufficient for triggering sufficient physical changes to provoke more intense pain in the back. Nevertheless, DI appears to mimic the level, type, and distribution of LBP experienced in space to some extent.
4.4 Correlations
Spinal length increases correlated with middle thoracic (T9) myofascial tone reduction, but with no other m. erector spinae biomechanical and viscoelastic changes. However, the reduction in viscoelastic effects at 6 h may have contributed to the failure to observe other significant correlations. It should also be noted that such trends are at odds with the increase in LBP severity seen at 6 h compared to 1 h. However, the relationship with the evolution of stature is unknown.
Interestingly, a positive correlation was observed between changes in spinal length and LBP. The relationship between LBP and spinal lengthening reported by Hutchinson et al. (1995) was taken to suggest that stretching of the spinal and paraspinal muscles may lead to the development of LBP sensation in simulated and actual microgravity. However, no significant correlation between changes in creep and spinal elongation or reported pain was observed.
Muscle atrophy is a common sequelae of microgravity (LeBlanc et al., 1995; Sayson & Hargens, 2008; Sayson et al., 2013). Previous research has observed atrophy of the lumbar paraspinal muscles (m. multifidus, m. erector spinae, m. quadratus lumborum) in astronauts (LeBlanc et al., 1995; Chang et al., 2016). The spinal postural muscle atrophy likely becomes a primary musculoskeletal response to unloading due to a predominance of gravity-sensitive Type I muscle fibres in the deep and superficial m. erector spinae and m. multifidus (MacDonald et al., 2006). However, 6 h in DI was not sufficient to induce trunk muscle atrophy. Thus, further longer-term investigations of m. erector spinae (and other muscle) biomechanical and viscoelastic properties and their relationship to LBP development and vertebral column functionality are warranted.
5 Conclusion
This study demonstrates that DI-induced bilateral m. erector spinae tone, creep, and stiffness changes persist beyond 2 h—albeit tending to attenuate. Thus, whilst spinal elongation and LBP were induced, its relationship with the trunk myofascial tissue changes is complex and its role in LBP pathogenesis observed in real and simulated microgravity is yet to be determined. Further study is warranted with longer DI duration and additional assessment of IVD geometry and vertebral column stability. Correct quantitative assessment of trunk myofascial tissue changes will likely enhance the determination of astronauts’ predisposition to back pain or strong muscle atony in prolonged space flight. Experiments performed with DI may benefit the design and validation of specific countermeasures against the deterioration of back muscle biomechanical and viscoelastic properties induced by microgravity and hypokinesia.
Data availability statement
The original contributions presented in the study are included in the article/Supplementary Material, further inquiries can be directed to the corresponding author.
Ethics statement
All the subjects gave written informed consent according to principles of the Declaration of Helsinki, were reviewed and approved by the Physiological Section of the Biomedicine Ethics Committee at the Institute of Biomedical Problems, a part of the Russian Academy of Science (protocols #401, 15.07.2015). Written informed consent was obtained from the individuals for the publication of any identifiable images or data included in this article.
Author contributions
AP designed the study, collected the data, and wrote the draft of the manuscript. DG made a major revision of the manuscript and proofread it. LA and IR helped with the study design, organized medical support, and contributed to the revision of the manuscript. ET and IK were the supervisors of the study and contributed to the revision of the manuscript. All authors contributed to the article and approved the submitted version.
Funding
This work was supported by the Russian Science Foundation (project 19-15-00435).
Acknowledgments
This article is dedicated to the memory of Inessa Kozlovskaya. We would like to thank IBMP colleagues: Inna Nosikova, Inna Zelenskaya, Tatyana Shigueva, Konstantin Zelenskiy, and Vitaliy Brykov for organizing the Dry Immersion protocol and excellent work. A personal thank you to the Centre of Human and Applied Physiological Sciences at King’s College London for cooperation and providing support towards this study.
Conflict of interest
Author DG is employed by KBRwyle Laboratories GmbH.
The remaining authors declare that the research was conducted in the absence of any commercial or financial relationships that could be construed as a potential conflict of interest.
Publisher’s note
All claims expressed in this article are solely those of the authors and do not necessarily represent those of their affiliated organizations, or those of the publisher, the editors and the reviewers. Any product that may be evaluated in this article, or claim that may be made by its manufacturer, is not guaranteed or endorsed by the publisher.
Supplementary material
The Supplementary Material for this article can be found online at: https://www.frontiersin.org/articles/10.3389/fphys.2022.1039924/full#supplementary-material
References
Abboud J., Nougarou F., Descarreaux M. (2016). Muscle activity adaptations to spinal tissue creep in the presence of muscle fatigue. PLoS One 11 (2), e0149076. doi:10.1371/journal.pone.0149076
Amirova L. E., Plehuna A., Rukavishnikov I. V., Saveko A. A., Peipsi A., Tomilovskaya E. S. (2021). Sharp changes in muscle tone in humans under simulated microgravity. Front. Physiol. 12, 661922. doi:10.3389/fphys.2021.661922
Bailey J. F., Miller S. L., Khieu K., O’Neill C. W., Healey R. M., Coughlin D. G., et al. (2018). From the international space station to the clinic: How prolonged unloading may disrupt lumbar spine stability. Spine J. 18, 7–14. doi:10.1016/j.spinee.2017.08.261
Bailey J. F., Nyayapati P., Johnson G. T. A., Dziesinski L., Scheffler A. W., Crawford R., et al. (2022). Biomechanical changes in the lumbar spine following spaceflight and factors associated with postspaceflight disc herniation. Spine J. 22, 197–206. doi:10.1016/j.spinee.2021.07.021
Balagué F., Mannion A. F., Pellisé F., Cedrasch i. C. (2012). Non-specific low back pain. Lancet 4, 482–491. doi:10.1016/S0140-6736(11)60610-7
Belavý D. L., Adams M., Brisby H., Cagnie B., Danneels L., Fairbank J., et al. (2016). Disc herniations in astronauts: What causes them, and what does it tell us about herniation on Earth? Eur. Spine J. 25, 144–154. doi:10.1007/s00586-015-3917-y
Belavý D. L., Armbrecht G., Richardson C. A., Felsenberg D., Hides J. A. (2011). Muscle atrophy and changes in spinal morphology: Is the lumbar spine vulnerable after prolonged bed-rest? Spine 36, 137–145. doi:10.1097/BRS.0b013e3181cc93e8
Brown J. W. (1977). Crew height measurements, the Apollo-Soyuz test project medical report. Washington DC: NASA SP-411.
Chang D. G., Healey R. M., Snyder A. J., Sayson J. V., Macias B. R., Coughlin D. G., et al. (2016). Lumbar spine paraspinal muscle and intervertebral disc height changes in astronauts after long-duration spaceflight on the international space station. Spine 41, 1917–1924. doi:10.1097/BRS.0000000000001873
Danneels L. A., Vanderstraeten G. G., Cambier D. C., Witvrouw E. E., De Cuyper H. J. (2000). CT imaging of trunk muscles in chronic low back pain patients and healthy control subjects. Eur. Spine J. 9, 4. doi:10.1007/s005860000190
de Abreu S., Amirova L., Murphy R., Wallace R., Twomey L., Gauquelin-Koch G., et al. (2017). Multi-system deconditioning in 3-day dry immersion without daily raise. Front. Physiol. 8, 799. doi:10.3389/fphys.2017.00799
Garcia K. M., Harrison M. F., Sargsyan A. E., Ebert D., Dulchavsky S. A. (2018). Real-time ultrasound assessment of astronaut spinal anatomy and disorders on the international space station. J. Ultrasound Med. 37, 987–999. doi:10.1002/jum.14438
Green D. A., Scott J. R. (2018). Spinal health during unloading and reloading associated with spaceflight. Front. Physiol. 8, 1126. doi:10.3389/fphys.2017.01126
Grigor’ev A. I., Kozlovskaya I. B., Shenkman B. S. (2004). [The role of support afferents in organisation of the tonic muscle system]. Ross. Fiziol. Zh. Im. I. M. Sechenova 90, 508–521. [In Russian, English summary]
Guo H. R. (2002). Working hours spent on repeated activities and prevalence of back pain. Occup. Environ. Med. 59, 680–688. doi:10.1136/oem.59.10.680
Haladaj R., Topol M. (2016). Multiple impulse therapy in the assessment of paraspinal muscle tone in patients with low back pain. Ortop. Traumatol. Rehabil. 18, 537–547. doi:10.5604/15093492.1230520
Hargens A. R., Vico L. (2016). Long-duration bed rest as an analog to microgravity. J. Appl. Physiol. 120, 891–903. doi:10.1152/japplphysiol.00935.2015
Hartvigsen J., Hancock M. J., Kongsted A., Louw Q., Ferreira M. L., Genevay S., et al. (2018). What low back pain is and why we need to pay attention. Lancet 391, 2356–2367. doi:10.1016/S0140-6736(18)30480-X
Hawker G. A., Mian S., Kendezerska T., French M. (2011). Measures of adult pain: Visual analog Scale for pain (VAS pain), numeric rating Scale for pain (NRS pain), McGill pain questionnaire (MPQ), short-form McGill pain questionnaire (SF-mpq), chronic pain grade Scale (CPGS), short form-36 bodily pain Scale (SF-36 BPS), and measure of intermittent and constant osteoarthritis pain (ICOAP). Arthritis Care Res. 63, S240–S252. doi:10.1002/acr.20543
Hayward T., Dolan P. (2018). How does creep of spinal tissues affect reflex activation of the back muscles? Orthopaedic Proceedings. 84.
Hides J. A., Gilmore C., Stanton W., Bohlscheid E. (2008). Multifidus size and symmetry among chronic LBP and healthy asymptomatic subjects. Man. Ther. 13, 43–49. doi:10.1016/j.math.2006.07.017
Hodges P., Holm A. K., Hansson T., Holm S. (2006). Rapid atrophy of the lumbar multifidus follows experimental disc or nerve root injury. Spine 31, 2926–2933. doi:10.1097/01.brs.0000248453.51165.0b
Hu X., Lei D., Li L., Leng Y., Yu Q., Wei X., et al. (2018). Quantifying paraspinal muscle tone and stiffness in young adults with chronic low back pain: A reliability study. Sci. Rep. 8, 14343. doi:10.1038/s41598-018-32418-x
Hutchinson K. J., Waterpaugh D. E., Murthy G., Convertino V. A., Hargens A. R. (1995). Back pain during 6 degrees head-down tilt approximates that during actual microgravity. Aviat. Space Environ. Med. 66, 256–259.
Kelly J. P., Koppenhaver S. L., Michener L. A., Proulx L., Bisagni F., Cleland J. A. (2018). Characterization of tissue stiffness of the infraspinatus, erector spinae, and gastrocnemius muscle using ultrasound shear wave elastography and superficial mechanical deformation. J. Electromyogr. Kinesiol. 38, 73–80. doi:10.1016/j.jelekin.2017.11.001
Kertsman E. L., Scheuring R. A., Barnes M. G., DeKorse T.B., Saile L.G. (2012). Space adaptation back pain: a retrospective study. Aviat. Space Environ. 83, 1. doi:10.3357/asem.2876.2012
Kimura S., Steinbach G. C., Watenpaugh D. E., Hargens A. R. (2001). Lumbar spine disc height and curvature responses to an axial load generated by a compression device compatible with magnetic resonance imaging. Spine 26, 2596–2600. doi:10.1097/00007632-200112010-00014
Kozlovskaya I. B. (2002). “Dry immersion as a powerful tool in studies of sensory-motor effects of microgravity,” in 5th international head-out water immersion symposium: Research simulations to model microgravity (Houston, Texas, 8–9.
Kozlovskaya I. B. (2008). Fundamental and applied objectives of investigations in immersion. Aviakosm. Ekol. Med. 42, 3–7. [In Russian, English summary].
Kozlovskaya I. B. (2007). “Gravitational mechanisms in the motor system,” in Contemporary course of classical physiology. Editors Y. V. Natochin, and V. A. Tkatchuk (Moscow: GEOTAR-Media), 115–134.
Kozlovskaya I. B., Grigor’eva L. S., Gevlich G. I. (1984). Comparative analysis of the effects of weightlessness and its ground models on the speed-strength properties and the tone of human skeletal muscle. Aviakosm. Ekol. Med. 18, 22–26. [In Russian, English summary].
Kozlovskaya I. B., Kirenskaya A. V. (1987). “Gravitational mechanisms in motor system,” in Studies in real and simulated weightlessness. New concepts of motor control. Editor J. Massion (Moscow: Plenum Press), 345–350.
Kozlovskaya I. B., Sayenko I. V., Miller T. F., Khusnutdinova D. R., Melnik K. A., Popov D. V., et al. (2006). New approaches to counter measures of the negative effects of micro-gravity in long-term space flights. Acta Astronaut. 60, 8–9. doi:10.1016/j.actaastro.2006.09.038
Kozlovskaya I. B., Sayenko I. V., Sayenko D. G., Miller T. F., Khusnutdinova D. R., Melnik K. A. (2007). Role of support afferentation in control of the tonic muscle activity. Acta Astronaut. 60, 285–294. doi:10.1016/j.actaastro.2006.08.010
LeBlanc A., Evans H. J., Schneider V. S., Wendt R. E., Hedrick T. D. (1994). Changes in intervertebral disc cross-sectional area with bed rest and space flight flight. Spine 19, 812–817. doi:10.1097/00007632-199404000-00015
LeBlanc A., Rowe R., Schneider V., Evans E., Hedrick T. (1995). Regional muscle loss after short duration spaceflight. Aviat. Space Environ. Med. 66 (12), 1151–1154.
Lee S. U., Hargens A. R., Fredericson M., Lang P. (2003). Lumbar spine disc heights and curvature: Upright posture vs. supine compression harness. Aviat. Space Environ. Med. 74 (5), 512–516.
Levangie P. K. (1999). The association between static pelvic asymmetry and low back pain. Spine 24, 1234–1242. doi:10.1097/00007632-199906150-00011
Lo W. L. A., Zhao J. L., Li L., Mao Y. R., Huang D. F. (2017). Relative and Absolute Interrater Reliabilities of a Hand-Held Myotonometer to Quantify Mechanical Muscle Properties in Patients with Acute Stroke in an Inpatient Ward Biomed Res. Int. 4294028. doi:10.1155/2017/4294028
MacDonald D. A., Mosley G. L., Hodges P. W. (2006). The lumbar multifidus: Does the evidence support clinical beliefs? Man. Ther. 11, 254–263. doi:10.1016/j.math.2006.02.004
Mannion A. F., Balagué F., Pellisé F., Cedraschi C. (2007). Pain measurement in patients with low back pain. Nat. Clin. Pract. Rheumatol. 3, 610–618. doi:10.1038/ncprheum0646
Miller T. F., Saenko I. V., Popov D. V., Vinogradova O. L., Kozlovskaya I. B. (2012). The effects of support deprivation and stimulation of the foot support zones on the lateral stiffness and electromyogram of the resting calf muscles. Hum. Physiol. 38, 763–767. doi:10.1134/s0362119712070146
Muckelt P. E., Warner M. B., Cheliotis-James T., Muckelt R., Hastermann M., Schoenrock B., et al. (2022). Protocol and reference values for minimal detectable change of MyotonPRO and ultrasound imaging measurements of muscle and subcutaneous tissue. Sci. Rep. 12, 13654. doi:10.1038/s41598-022-17507-2
Nair K., Masi A. T., Andonian B. J., Barry A. J., Coates B. A., Dougherty J., et al. (2016). Stiffness of resting lumbar myofascia in healthy young subjects quantified using a handheld myotonometer and concurrently with surface electromyography monitoring. J. Bodyw. Mov. Ther. 20, 388–396. doi:10.1016/j.jbmt.2015.12.005
Navasiolava N. M., Custaud M. A., Tomilovskaya E. S., Larina I. M., Mano T., Koch G. G., et al. (2011). Long-term dry immersion: Review and prospects. Eur. J. Appl. Physiol. 111, 1235–1260. doi:10.1007/s00421-010-1750-x
Nemirovskaya T. L., Shenkman B. S. (2002). Effect of support stimulation on unloaded soleus in rat. Eur. J. Appl. Physiol. 87, 120–126. doi:10.1007/s00421-002-0603-7
Pool-Goudzwaard A. L., Belavy D. L., Hides J. A., Richardson C. A., Snijders C. J. (2015). Low back pain in microgravity and bed rest studies. Aerosp. Med. Hum. Perform. 86, 541–547. doi:10.3357/AMHP.4169.2015
Rajulu S., Benson E. (2009). JSC-17798: Complexity of sizing for space suit applications. Houston, TX: National Aeronautics and Space Administration.
Robin A., Navasiolava N., Gauquelin-Koch G., Gharib C., Custaud M-A., Treffel L. (2022). Spinal changes after 5-day dry immersion as shown by magnetic resonance imaging (DI-5-CUFFS). Am. J. Physiol. Regul. Integr. Comp. Physiol. 323, R310–R318. doi:10.1152/ajpregu.00055.2022
Rukavishnikov I. V., Amirova L. E., Kukoba T. B., Tomilovskaya E. S., Kozlovskaya I. B. (2017). Effects of gravitational unloading on back muscles tone. Hum. Physiol. 43, 291–300. doi:10.1134/s0362119717030173
Sayson J. V., Hargens A. R. (2008). Pathophysiology of low back pain during exposure to microgravity. Aviat. Space Environ. Med. 79, 365–373. doi:10.3357/asem.1994.2008
Sayson J. V., Lotz J., Parazynski S., Hargens A. R. (2013). Back pain in space and post-flight spine injury: Mechanisms and countermeasure development. Acta Astronaut. 86, 24–38. doi:10.1016/j.actaastro.2012.05.016
Schneider S., Peipsi A., Stokes M., Knicker A., Abeln V. (2015). Feasibility of monitoring muscle health in microgravity environments using Myoton technology. Med. Biol. Eng. Comput. 53, 57–66. doi:10.1007/s11517-014-1211-5
Schoenrock B., Zander V., Dern S., Limper U., Mulder E., Veraksitš A., et al. (2018). Bed rest, exercise countermeasure and reconditioning effects on the human resting muscle tone system. Front. Physiol. 9, 810. doi:10.3389/fphys.2018.00810
Shenkman B. S., Nemirovskaya T. L., Shapovalova K. B., Podlubnaya Z. A., Vikhliantsev I. M., Moukhina A. M., et al. (2007). Afferent control mechanisms involved in the development of soleus fiber alterations in simulated hypogravity. Acta Astronaut. 60, 307–312. doi:10.1016/j.actaastro.2006.08.012
Shenkman B. S., Tsaturyan A. K., Vikhlyantsev I. M., Kozlovskaya I. B., Grigoriev A. I. (2021). Molecular mechanisms of muscle tone impairment under conditions of real and simulated space flight. Acta Naturae 13, 85–97. doi:10.32607/actanaturae.10953
Shigueva T. A., Zakirova A. Z., Tomilovskaya E. S., Kozlovskaya I. B. (2015). Effect of support deprivation on the order of motor unit recruitment. Hum. Physiol. 41, 813–816. doi:10.1134/S036211971507021X
Shulzhenko E. B., Vil-Vilyams I. F. (1975). Simulation of the human body deconditioning with the method of ‘‘dry’’ immersion. Moscow, Russia: 10th K.E. Tsiolkovsky readings, 39–47. [In Russian, English summary].
Simons D. G., Mense S. (1998). Understanding and measurement of muscle tone as related to clinical muscle pain. Pain 75, 1–17. doi:10.1016/S0304-3959(97)00102-4
Solomonow M., Baratta R. V., Banks A., Freudenberger C., Zhou B. H. (2003). Flexion-relaxation response to static lumbar flexion in males and females. Clin. Biomech. (Bristol, Avon) 18, 273–279. doi:10.1016/s0268-0033(03)00024-x
Solomonow M. (2012). Neuromuscular manifestations of viscoelastic tissue degradation following high and low risk repetitive lumbar flexion. J. Electromyogr. Kinesiol. 22, 155–175. doi:10.1016/j.jelekin.2011.11.008
Styf J. R., Ballard R., Fechner K., Watenpaugh D., Kahan N. J., Hargens A. R. (1997). Height increase, neuromuscular function, and back pain during 6 degrees head-down tilt with traction. Aviat. Space Environ. Med. 68, 24–29.
Sung P. S., Lammers A. R., Danial P. (2009). Different parts of erector spinae muscle fatigability in subjects with and without low back pain. Spine J. 9, 115–120. doi:10.1016/j.spinee.2007.11.011
Tomilovskaya E., Shigueva T., Sayenko D., Rukavishnikov I., Kozlovskaya I. (2019). Dry immersion as a ground-based model of microgravity physiological effects. Front. Physiol. 10, 284. doi:10.3389/fphys.2019.00284
Tomilovskaya E. S., Rukavishnikov I. V., Amirova L. E., Shigueva T. A., Saveko А. А., Kitov V. V., et al. (2020). 21-day dry immersion: Design and primary results. Aviakosmicheskaya i Ekol. Meditsina Russ. 54, 5–14. doi:10.21687/0233-528X-2020-54-4-5-14
Tomilovskaya E. S., Rukavishnikov I. V., Kofman I. S., Kitov V. V., Grishin A. P., Yu N., et al. (2014). “Functional sensory-motor performance following long term space flight: The first results of "field test" experiment,” in 65th international astronautical congress (Ontario, Canada.
Treffel L., Massabuau N., Zuj K., Custaud M. A., Gauquelin-Koch G., Blanc S., et al. (2017). Pain and vertebral dysfunction in dry immersion: A model of microgravity simulation different from bed rest studies. Pain Res. Manag. 2017, 9602131. doi:10.1155/2017/9602131
Treffel L., Mkhitaryan K., Gellee S., Gauquelin-Koch G., Gharib C., Blanc S., et al. (2016). Intervertebral disc swelling demonstrated by 3D and water content magnetic resonance analyses after a 3-day dry immersion simulating microgravity. Front. Physiol. 7, 605. doi:10.3389/fphys.2016.00605
Treffel L., Navasiolava N., Mkhitaryan K., Jouan E., Zuj K., Gauquelin-Koch G., et al. (2020). DI-5-Cuffs: Lumbar intervertebral disc proteoglycan and water content changes in humans after five days of dry immersion to simulate microgravity. Int. J. Mol. Sci. 21, 3748. doi:10.3390/ijms21113748
Vico L., Hargens A. (2018). Skeletal changes during and after spaceflight. Nat. Rev. Rheumatol. 14, 229–245. doi:10.1038/nrrheum.2018.37
Wing P. C., Tsang I. K., Susak L., Gagnon F., Gagnon R., Potts J. E. (1991). Back pain and spinal changes in microgravity. Orthop. Clin. North Am. 22, 255–262. doi:10.1016/s0030-5898(20)31651-5
Keywords: low back pain, muscle tone, myotonpro, dry immersion, space flight, myofascial tissue properties
Citation: Plehuna A, Green DA, Amirova LE, Tomilovskaya ES, Rukavishnikov IV and Kozlovskaya IB (2022) Dry immersion induced acute low back pain and its relationship with trunk myofascial viscoelastic changes. Front. Physiol. 13:1039924. doi: 10.3389/fphys.2022.1039924
Received: 08 September 2022; Accepted: 26 September 2022;
Published: 13 October 2022.
Edited by:
Katsumasa Goto, Toyohashi Sozo University, JapanReviewed by:
Tatsuro Egawa, Kyoto University, JapanLoïc Treffel, Lab INMG—PNMG University of Lyon; IRF'O-ITO Toulouse, France
Copyright © 2022 Plehuna, Green, Amirova, Tomilovskaya, Rukavishnikov and Kozlovskaya. This is an open-access article distributed under the terms of the Creative Commons Attribution License (CC BY). The use, distribution or reproduction in other forums is permitted, provided the original author(s) and the copyright owner(s) are credited and that the original publication in this journal is cited, in accordance with accepted academic practice. No use, distribution or reproduction is permitted which does not comply with these terms.
*Correspondence: Anastasija Plehuna, YS5wbGVodW5AZ21haWwuY29t
†Deceased